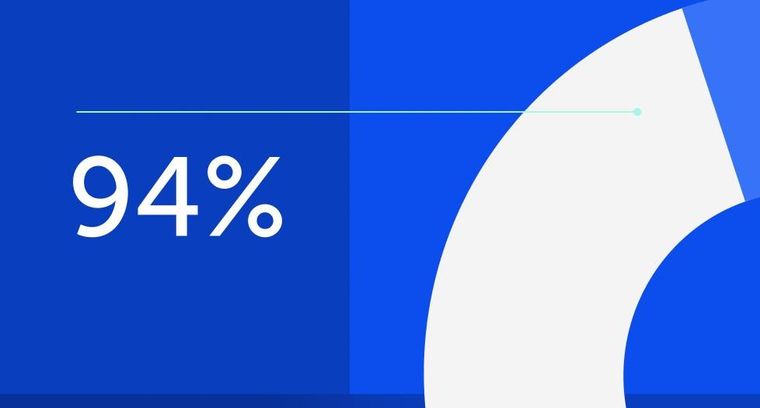
94% of researchers rate our articles as excellent or good
Learn more about the work of our research integrity team to safeguard the quality of each article we publish.
Find out more
MINI REVIEW article
Front. Mater., 24 April 2024
Sec. Colloidal Materials and Interfaces
Volume 11 - 2024 | https://doi.org/10.3389/fmats.2024.1376865
This article is part of the Research Topic10 years of Frontiers in Materials: past discoveries, current challenges and future perspectivesView all 8 articles
Aqueous Zinc Ion Batteries (ZIBs), characterized by their high theoretical capacity, cost-effectiveness, and robust safety profile, stand out as one of the most promising contenders for the next-generation of electrochemical energy storage applications. Nevertheless, the commercialization of ZIBs encounters obstacles of unsatisfactory energy density and suboptimal cycling stability, which are related to the unstable interfaces of Zn anodes and cathodes. Herein, the research advances in Zn anodes and cathode materials and corresponding interface engineering in recent years are systematically reviewed. The rationalization of these research can guide further investigations in the design of cathode/anode materials in ZIBs.
With the rapid depletion of fossil fuel resources and the exacerbation of the greenhouse effect, the challenges of energy shortages and environmental pollution have emerged as pivotal impediments to the sustainable development of human society (Zhang et al., 2021; Lv et al., 2023). Renewable energy sources such as wind power, solar energy, and tidal energy are gaining widespread attention. However, the intermittence and instability of renewable energy restricts their practical large-scale applications. In recent years, lithium-ion batteries have progressively asserted their dominance in the commercial rechargeable battery market, driven by attributes like high energy density, prolonged lifespan, and well-established manufacturing technologies (Schmuch et al., 2018). Notwithstanding their commercial success, lithium-ion batteries confront several challenges, including the constrained availability of lithium resources, elevated costs, safety apprehensions, and environmental pollution stemming from flammable and toxic organic electrolytes (Zhang et al., 2023). These issues significantly impede the continued advancement and widespread deployment of lithium-ion batteries. In contrast to lithium-ion batteries, which raise safety concerns, aqueous zinc-ion batteries exhibit a multitude of advantages, including higher safety levels, lower costs, and a more convenient manufacturing process. Consequently, ZIBs are positioned to emerge as the next-generation of environmentally friendly rechargeable batteries (Zhang et al., 2023). Nevertheless, the commercial adoption of ZIBs encounters substantial challenges attributed to two prevalent issues of limited energy density and suboptimal cycling stability.
The storage mechanism in ZIBs primarily revolves the zinc plating/stripping processes on the anode interface and the reversible insertion/extraction of cations on the cathode. Currently, the zinc anode in ZIBs encounters challenges associated with zinc dendrite growth, low Coulombic efficiency (CE) due to side reactions, and inadequate lifespan (Cao et al., 2020). Specifically, irregular Zn2+ tip effects in ZIBs may lead to the uncontrolled growth of zinc dendrites, breaching the separator and causing internal short circuits. Furthermore, side reactions, including zinc corrosion and hydrogen evolution reactions (HER), induce the irreversible zinc plating/stripping and electrolyte consumption. On the other hand, cathode materials significantly influence the cost and electrochemical performance of ZIBs. The most commonly reported cathode materials mainly include manganese-based oxides, vanadium-based oxides, prussian blue analogs (PBAs), and transition metal dichalcogenides (TMDs). However, all of these cathode materials have their corresponding inherent drawbacks (Wang et al., 2021b). For instance, manganese-based oxides and vanadium-based oxides are susceptible to the dissolution of active materials, while PBAs and TMDs usually exhibit relatively low capacity and working voltage. Additionally, strong interactions between Zn2+ and the cathode lattice impede the diffusion kinetics of Zn2+, resulting in the irreversible embedding of Zn2+ in the lattice of the cathode materials.
In this review, we have consolidated the most recent efforts and developments on the electrode materials and interface engineering, aiming at attaining high energy density and prolonged lifespan in ZIBs. First, the challenges confronting zinc anode and recent advancements in establishing stable zinc anode interfaces are delineated. Subsequently, a brief overview of research progress of cathode materials is provided. This review would serve as a valuable resource for both current and future researchers, fostering advancements in the field of and catalyzing research and applications in ZIBs.
In the neutral or slightly acidic electrolyte, such as in ZnSO4 electrolyte, Zn metal undergoes the redox reaction at the anode/electrolyte interface to generate Zn2+ through the donation of electrons, and then Zn (OH)42− forms through the incorporation of Zn2+ with OH− (Figure 1A). The continuous dissolution of Zn anode in ZnSO4 aqueous electrolyte ultimately results in the formation of Zn4SO4(OH)6·xH2O (ZSH) on the anode surface (Zhu et al., 2023). Additionally, given the fact that the standard electrode potential of Zn2+/Zn (−0.76 V) is lower than that of H+/H2 (0 V), HER would occur in advance of the Zn deposition reaction (Figure 1B). In aqueous ZnSO4 electrolyte, the main side reactions possibly occurred on the Zn anode are as following.
Figure 1. (A) Schematic diagram of ZHS formation on anode surface (Li et al., 2020) (B) In situ investigations of the HER (Qiu et al., 2019) (C) The mechanisms of “tip effect” (Li et al., 2023c) (D) Simulation results of dendrite growth (Wang et al., 2023).
In addition to HER and ZHS, the unstable thermodynamics also lead to spontaneous corrosion reactions on the Zn surface. Both chemical corrosion and electrochemical corrosion occur simultaneously upon the direct contact of the electrolyte and the electrode (Tian et al., 2023).
Dendrite growth is another important issue in the development of ZIBs. The dendrite growth would potentially compromise battery life span, Coulombic efficiency (CE) and specific capacity, thereby hampering practical applications (Hong et al., 2023). Dendrite growth is mainly originated from the nonuniform distribution of electric field on the Zn electrode surface, associating with the irregular surface imperfections that act as nucleation sites for growth (Figure 1C). In addition, the inhomogeneous distribution of zinc ions in the electrolyte near the electrode would also contribute to the non-uniform deposition of Zn atoms (Figure 1D), leading to uncontrolled dendrite formation and the subsequent risk of short circuits (Cao et al., 2022). Dendrite growth would be promoted by the tip effect, Zn2+ prefers to deposit on the tip of the dendrite formed at the initial nucleation site. Consequently, the dendrite growth increases the risk of penetrating the separators, leading to a short circuit. Furthermore, zinc dendrite would be detached from the electrode surface upon cycling, consequently contributing to the formation of “dead” zinc (Qian et al., 2022).
Therefore, the metallic Zn anode encounters issues such as dendrite growth, HER, surface corrosion, and the formation of by-products in ZIBs. Importantly, these associated issues would create a close and intricate impact on the anode performance (Zhang et al., 2020). The growth of dendrites increases would increase the active surface area of Zn anode, accelerating both hydrogen evolution and corrosion reactions. The formation of “dead Zn” would further intensify the consumption of electrolyte, leading to the generation of more by-products. Therefore, in order to improve the performance of Zn anode, these issues should be considered thoroughly and well addressed.
Many efforts have been devoted to mitigating the issues of zinc anode mentioned above, and many effective strategies have been proposed in the literature. In this section, effective strategies based on the interface engineering for the protection of Zn anode, including polymers, inorganic substance, alloying, and electrolyte additives, are summarized.
Polymers with properties of flexibility, abundant polar groups, and eco-friendly are prospected to be a promising agents to modify the surface of Zn anodes (Hong et al., 2022b). Adsorption sites induced by the functional groups of polymers play a crucial role in transferring Zn2+ along the polymer chains to the reaction interface, ensuring the uniform distribution of Zn2+ at the molecular scale through fast ion transport rates. For example, an ion-selective polymer glue was designed and coated on the Zn surface (Jiao et al., 2021). This polymer layer suppresses the contact of the Zn anode with the electrolyte, effectively blocking water diffusion while allowing rapid Zn2+ ion migration and thereby facilitating the uniform electrodeposition. A multifunctional polymeric interphase composed of polyamide (PA) and zinc trifluoromethane sulfonate (Zn (TfO)2) was also developed (Zhao et al., 2019). The PA layer with abundant hydrogen bonding effectively hinders the direct contact of water molecule with Zn, suppressing the side reactions and dendrite growth. A semi-interpenetrating network (s-IPN) structure with hydrophilic and robust polymer network was generated for the protection of Zn anode (Ye et al., 2023). Benefiting from epoxide and amine groups in s-IPN, the symmetric batteries with modified anode showed high cycling stability of over 2,000 h.
Usually, the single unamended polymer protective layers are not sufficient to significantly improve the cycling performance and mechanical property of Zn anodes. Therefore, the modification of single polymers was proposed to enhance Zn anode property. For example, the self-adaptable poly (dimethylsiloxane) (PDMS)/TiO2−x coated Zn anode (Figure 2A). (PDMS)/TiO2−x was designed to dynamically adapt to volume changes and inhibited dendrite growth, consequently stabilizing the Zn surface (Guo et al., 2022). Rapid and uniform transfer of Zn2+ were induced by the synergistic effect of oxygenvacancy-rich TiO2−x and PDMS.
Figure 2. (A) Schematic illustrations of (PDMS)/TiO2−x coating and protective mechanism (Guo et al., 2022), (B) Zn deposition process on Zn@Ca-Mont and Zn@Zn-Mont anodes (Hong et al., 2021) (C) grain boundary engineering (Zhao et al., 2023a) (D) the EDL structure and Zn deposition behavior regulation in PMCNA (Feng et al., 2023).
Inorganic nonmetallic materials have been employed as non-conductive coatings on metallic Zn anode, which benefits from their rapid ion conductivity and chemical inertess (Hong et al., 2021), thus effectively stabilizing the anode interface and increasing anode performance (Figure 2B). Liu et al. (2021) proposed a Zn-metal oxide Ohmic contact interface model to improve the reversibility of Zn anodes. Zn anodes modified by the metal oxides with high work function, such as CeO2, TiO2, WO3, and MoO3, distinctly attract the cations to diffuse inward. The interface modified Zn anode with CeO2 layers exhibited rapid Zn2+ diffusion kinetic, enhanced Coulombic efficiency, and dendrite-free Zn2+ deposition.
In recent years, inorganic salts have emerged as a viable option for interface protection of Zn metals. Amorphous zinc phosphate (AZP) nanofilm with flexible thickness was generated as a solid electrolyte interphase (SEI) for Zn anode (Li et al., 2023b). Consequently, numerous shortened pathways for rapid Zn2+ transportation were constructed by the amorphous layer. The nanofilm with hydrophobic propterty effectively prevents the direct contact of water with the Zn anode, thereby retarding the interfacial HER and suppressing associated corrosion and passivation.
A robust inorganic interface with strong adhesion on the Zn surface can also be fabricated by a simple acid etching method. Four types acids, including oxalic acid, phytic, citric, and phosphoric acid were used to regulate the interfaces of Zn anodes (Wang et al., 2021a). With more exposing (002) plane, high corrosion-resistance and suppressed dendritic deposition were achieved. The zinc phosphate@Zn (PPZ@Zn) electrode with high extent of texturing and compact coating endowed stable Zn stripping/plating interfaces.
Alloying with one or more metal elements provides an effective method for enhancing the protection of the Zn electrode. Mechanical strength, corrosion resistance, and electrical conductivity would be improved by these alloy anodes (Hong et al., 2022a). The involvement of the electrochemically inactive metal elements, such as Cu, Ag, and Mn in the alloys reduces the atomic defects in the Zn anodes. This reduction in defects not only hinders the formation of by-products and inhibits corrosion on the Zn surface but also significantly lowers the nucleation energy barrier.
Metallic Cu, characterized by excellent chemical/physical stability and cost-effectiveness, is considered an effective element for the generation of alloy anodes. Zhou et al. (2022) demonstrated a strategy to address issues on Zn surface by electrochemically depositing Zn onto the Cu surface. Xu et al. (2021) generated a robust protective layer by immersing Zn plate into an aqueous solution of CuSO4. Owing to the strong zincophilicity and inert nature of Cu, alleviated interface corrosion and inhibited dendrite growth were realized. Simultaneously, the structural features of Zn-Cu could tune uniform nucleation and deposition of Zn2+ to suppress dendrite growth, thereby endowing alloying anode with lowered nucleation overpotential and polarization, prolonged cycling stability and increased CE. The reduction potential of Sn is −0.14 V, higher than that of Zn (−0.76 V vs. SHE). Sn was selected to generate a protective layer on the Zn foil (Li et al., 2021a). The alloy anode fabricated increases the hydrogen precipitation energy barrier and lowers the deposition energy barrier, promoting the Zn2+ deposition kinetics.
Recently, Zhao et al. (2023a) developed a conventional metallurgical process for the construction of Zn-Ti alloy anode. The grain boundary of metallic Zn was modified by the construction of the Zn-Ti dual-phase alloy (Figure 2C). In this alloy, Ti-containing solid solutions and intermetallic compounds was formed and thermodynamically stabilized at grain boundaries. Moreover, the Ti-containing alloy anode with high interface zincophilicity substantially inhibited intergranular corrosion and HER during cycling, diminished nucleation energy barrier, and promoted favorable nucleation mode.
Apart from the direct interface engineering of the Zn anodes, the incorporation of additives into the electrolyte is regarded as another effective strategy for the in-situ construction of stable interface. For instance, polyacrylamide (PAM), a low-cost polymer containing ketone units, was employed as an effective electrolyte additive (Zhang et al., 2019). The overpotential of zinc nucleation was significantly reduced and the stability of zinc deposition as enhanced. Liu et al. (2023) found that cetyltrimethyl ammonium bromide (CTAB) not only adsorbed onto the zinc anode surface, thereby regulating Zn2+ deposition orientation and inhibiting dendrite formation, but also modified the solvation structure of Zn2+ to reduce HER. Feng et al. (2023) designed a polymer additive (PMCNA) engineered by copolymerizing 2-methacryloyloxyethyl phosphorylcholine (MPC) and N-acryloyl glycinamide (NAGA) (Figure 2D). PMCNA adsorbed onto the Zn anode surface, forming a interface protective layer in situ to resist side reactions and guided Zn nucleation depositing along the (002) plane.
In addition to organic molecules, metal ions have been used as additives added into liquid electrolytes to enhance the anode stability. For instance, Hu et al. (2022) reported that CeCl3, when used as an additive, could effectively inhibit the growth of Zn dendrites. They revealed that Ce3+can be preferentially adsorb on the surface of the Zn anode, forming H2O-poor electrical double layer that inhibits side reaction through the electrostatic shielding effect. Specifically, the Ce3+ ions accumulated around the Zn protrusions, forming an interfacial “electrostatic shield” with a regional positive electric field. The electric field prevented the further accumulation of Zn2+ ions through the electrostatic force, impeding the deposition of Zn2+ ions and rendering the even Zn plating upon cycling.
Anode interface engineering aims to impede the direct interaction between zinc and the electrolyte, thereby effectively mitigating dendrite formation, corrosion, and side reactions (Wu et al., 2024). Polymer and Inorganic nonmetallic materials as coatings exhibit sufficient conductivity and compatibility with Zn metal. Nonetheless, thinner coatings may be prone to cracking and shedding, compromising anode stability, while excessively thick coatings could elevate impedance and hinder ionic conductivity.
For the Zn alloy anodes, it is significant to optimize the deposition time, solution concentration, and voltage to dictate alloy property (Du et al., 2023). The researchers predominantly focus on binary alloys rather than ternary alloys. Therefore, further exploration of binary alloys is warranted.
Electrolyte additives is regarded as an effective strategy for the in-situ construction of stable interface (Zhao et al., 2023b). However, the presence of additives in the electrolyte may impact Zn ion migration, leading to decreased ionic conductivity and complicating the interface of the anode. Therefore, optimizing the concentration of additives poses a challenge for this strategy.
The energy storage mechanisms of lithium and sodium ion batteries have been well elucidated, which generally include intercalation, alloying, and conversion mechanisms. For ZIBs, six mechanisms have been delineated (Figure 3A), such as Zn2+ insertion/extraction, reversible chemical conversion reactions, Zn2+ and H+ co-insertion/extraction, dissolution/deposition reactions, ion coordination and multiple-ion insertion/extraction (Xu et al., 2012; Pan et al., 2016; Li et al., 2020; Li et al., 2023).
Figure 3. (A) Schematic diagrams of the storage mechanism for cathodes in ZIBs (Li et al., 2023) (B) Illustration of synthesis process of MnO2@CFP (Sun et al., 2017) (C) Illustration of the synthesis of AMO (Yao et al., 2023) (D) Schematic illustration of the energy storage mechanism in the Zn||ZVO and Zn||Ov-ZVO batteries (Ye et al., 2023) (E) TEM images of CuMn-PBA DSNBs (Zeng et al., 2022) (F) Illustration of the preparation process and the crystal structure of D-MoS2-O (Li et al., 2021b).
The electrochemical performance of a battery system is significantly contingent upon the type, structure, and surface properties of the electrode material. The charge storage process hinges on the migration of Zn2+ ions between two electrodes for ZIBs, hence, it is imperative to develop high-performance of cathodes for ZIBs. Recently, manganese-based oxides, vanadium-based oxides, prussian blue analogs and transition metal dichalcogenides were received considerable research interest for ZIBs.
Due to the advantages of cost-effectiveness, abundant availability, relatively low toxicity and multivalent states (Mn2+, Mn3+, Mn4+, Mn7+), manganese-based oxides cathodes have been extensively investigated as the cathode materials for ZIBs over the past decade (Fang et al., 2018; Liu et al., 2021; Hong et al., 2023). MnO2, as one of the most important manganese-based oxides materials, has a variety of crystal structures, including α-MnO2, β-MnO2, δ-MnO2 and so on. These structures consist of [MnO6] octahedra as the basic structural units, interconnected by shared corners or edges into chain-, tunnel-, and layered-type configurations.
As the most extensively studied of MnO2, α-MnO2 is composed of octahedral MnO6 in a double chain style, possessing a 2 × 2 tunnel in its structure. Due to the substantial tunnels in its structural framework, α-MnO2 could serve as a host material for foreign cations, including Zn2+ ions. For instance, Pan et al. (Pan et al., 2016) demonstrated a reversible Zn/MnO2 system where aqueous ZnSO4 based solution was used as the electrolyte, and α-MnO2 nanofibers were used as the cathode materials (Figure 3B). For the α-MnO2 nanofiber cathode, it exhibited an operating voltage of 1.44 V, a specific capacity of 210 mA h g−1 at 0.2C and a capacity retention of 92% over 5,000 cycles. Sun et al. (2017) proposed an in-situ electrodeposition method to prepare a binder-free nanocrystal α-MnO2 cathode, donated as MnO2@CFP. Stable cycling performance up to 10,000 cycles at 6.5C with a low capacity decay rate of 0.007% per cycle was achieved. Meanwhile, the consequent H+ and Zn2+ insertion mechanism in Zn/MnO2 battery chemistry was revealed.
β-MnO2 is characterized by the most stable 1 × 1 tunnel-type structure constructed by MnO6 octahedra single chains through the corner sharing. Recent studies have confirmed the excellent zinc storage performance of β-MnO2. Liu et al. (2019) synthesized β-MnO2 nanorods through a microwave hydrothermal method. A high-capacity delivery of 288 mA h g−1 at 0.05C and cycling stability with capacity retentions of 84.3% after 1000 cycles at 2C were achieved. The capacity fading of the β-MnO2 cathode was primarily attributed to the formation of inactive ZnMn2O4 after long-term charge/discharge cycling. Zhang et al. (2017) reported a high-performance rechargeable aqueous ZIBs system with β-MnO2 cathode, which delivered a high capacity of 225 mA h g−1 at 0.65C. The tunnel-structured manganese dioxide polymorphs underwent a phase transition to layered zinc-buserite on first discharging, enabling subsequent intercalation of zinc cations in the latter structure.
δ-MnO2 is constructed by co-angled [MnO6] octahedrons, forming a two-dimensional infinite layer. Recently, layered δ-MnO2 was also received consierable interest as cathode materials for ZIBs. Remarkably high battery performances was demonstrated and attributed to the large interlayer distance (approximately 7.0 Å) of δ-MnO2. Yao et al. (2023) reported a two-step strategy to prepare pre-intercalation of ammonium ions in layered δ-MnO2 nanosheets, denoted as AMO, as the cathode material for ZIBs (Figure 3C). The AMO delivered a reversible Zn storage capacity of 128.7 mA h g−1 over 10000 cycles at 4 A g−1 without significant capacity decay. Furthermore, the intercalated ammonium ions serve as the interlayer pillars to expand the lattice spacing of AMO and form hydrogen-bond networks, thus relieving the Jahn–Teller effect and enabling fast Zn2+ ion diffusion kinetics.
Vanadium-based oxides are widely investigated as cathode materials for ZIBs due to the manifold vanadium oxidation states (Vx+ (x = 2, 3, 4, 5)) and diverse redox properties. The fundamental V–O coordination polyhedra assemble into different frameworks of vanadium oxides, with V coordination polyhedra transformed from tetrahedron through square pyramid, trigonal bipyramid and regular octahedra. The changes in both complex polyhedra and V oxidation states can be systematically tuned to accommodate Zn2+ ions, resulting in outstanding electrochemical performance (Wang et al., 2023; Wang et al., 2023).
V2O5 exhibits a layered structure bound together by weak van der Waals forces, showing a square-pyramidal coordination of V5+ linked to five oxygen atoms. The layered structure of V2O5 could facilitate the intercalation of Zn2+ and thus has been extensively investigated as an intercalation host for ZIBs. Ye et al. (2023) fabricated oxygen vacancy-enriched V2O5 structures, denoted as Ov-ZVO, by hydrothermal method followed by further annealing. It was revealed that the presence of oxygen vacancies reduced Zn2+ diffusion barriers and weakened the interaction between Zn and O atoms in Ov-ZVO (Figure 3D), thus leading to the excellent electrochemical performance. A remarkably high specific capacity of 402 mA h g−1 at 0.1 A g−1 and impressive energy output of 193 W h kg−1 at 2673 W kg−1 were demonstrated. In another investigation by Qi et al. (2023), a conductive polypyrrole (PPy) was incorporated with V2O5, resulting in PPy-intercalated V2O5 (PPy-V2O5). The intercalated PPy served to prevent structure collapse, contributing to the structure and cycling stability. A high reversible capacity of 450.6 mA h g−1 at 0.5 A g−1 and excellent capacity retention of 90.0% at 10 A g−1 after 8500 cycles were achieved.
V2O3 is recognized as a promising electrochemical energy storage material with high capacity. V2O3 has a 3D structure-like tunnels formed by the edge-sharing of the adjacent octahedron with two common VO6 octahedra. These tunnels would facilitate the intercalation of cations. The V-V chain allows for the transfer of vanadium 3 days electrons, inducing high electronic conductivity. The high electronic conductivity is an important property for application as a cathode material in ZIBs. Zhu et al. (2021) designed a vanadium defective V2O3 (Vd–V2O3) by a hydrothermal method and an ensuing annealing process. The vanadium vacancies created permanent sites for the preoccupation of a small amount of Zn2+, enhancing the stability of lattice and mitigating deterioration during the process of Zn2+ insertion/extraction. The Vd–V2O3 exhibited a capacity of 196 mA h g−1 at 0.1 A g−1 and achieved 30,000 stable cycles with a capacity retention rate of 81%.
Prussian blue analogs with features of substantial open structures, versatile ion-storage capabilities and abundant redox-active sites have garnered attention for application in ZIBs (Hurlbutt et al., 2018; Li et al., 2022). The general formula of PBAs is AxM′y [M″(CN)6]z∙nH2O, where M′ and M″ represent transition-metal ions in the divalent or trivalent state, and A can be Li+, Na+, or Zn2+ ions. In the PBAs structure, M′-coordinated and M″-coordinated transition metal ions are interconnected by C≡N, forming a rigid open framework with a large interlayer for hosting A ions or water molecules, as well as 3D diffusion tunnels for A ions.
In a study conducted by Zeng et al. (2022), Cu-substituted Mn-PBAs double-shelled nanoboxes (CuMn-PBA DSNBs) were prepared by using efficient tannic acid etching and cation exchange methods (Figure 3E). The distinctive hollow structures, Cu substitution and Mn vacancies in the CuMn-PBA DSNBs electrode contributed to the improved Zn ion storage properties. A notable rate capability (116.8 mA h g−1 at 0.1 A g−1) and superior cycling stability (96.8% retention after 2000 cycles) were achieved. Yang et al. (2023) proposed the epitaxial growth of Fe on Mn-hexacyanoferrate to construct a core–shell double-atom-redox PBA (Mn@FeHCF). The in-situ surface reorganization effectively suppressed Jahn–Teller distortion and prevented Mn dissolution into the electrolyte in the core Mn-PBA. Mn@FeHCF delivered a high discharge capacity of 166 and 117 mA h g–1 and a capacity retention of 72.4% and 83.9% over 400 and 4800 cycles at 0.1 and 2 A g–1, respectively.
Transition metal dichalcogenides (TMDs) are typically 2D layered structures constructed by weak van der Waals forces. Transition metal dichalcogenides are denoted as MX2, where M represents transition metals (e.g., V, Mo, Ti and W) and X denotes chalcogen atoms (S, Se and Te). Generally, TMDs can be classified into three crystal phases of 1T, 2H, and 3R with the different arrangements of the TMDs monolayers. The different arrange of the monolayers would lead to different properties (Lee et al., 2021; Yang et al., 2022; Shuai et al., 2023). Due to their sufficient spacing layer, large theoretical capacity and fast ion transfer kinetics, TMDs have been investigated as ZIB cathode. For example, He et al. (2017) demonstrated that nanosheets of VS2 can accommodate 0.23 Zn2+ per formula units, giving a specific capacity of 190.3 mA h g−1 at 0.05 A g−1. Tan et al. (2022) employed a one-step hydrothermal method to fabricate 1T-VS2 nanospheres assembled from nanosheets. The distinctive hierarchitectures of 1T-VS2 delivered a high reversible capacities of 212.9 and 102.1 mA h g−1 at 0.1 and 5 A g−1, respectively. An ultralong cycling life with 86.7% capacity retention over 2000 cycles at 2 A g−1was also achieved.
In addition to VS2, MoS2 has also been explored as a cathode material for ZIBs. Li et al. (2021b) reported a molecular engineering strategy (Figure 3F) which involves structure defects manufacturing and O-doping to enlarge interlayers and unlock basal planes of MoS2 (donated as D-MoS2-O). It was demonstrated that the incorporation of defects and O-doping in MoS2 reduced Zn2+ migration barriers, leading the improved electrochemical performance. Superior rate performance (261 mA h g−1 at 0.1 A g−1 with 102.4 mA h g−1 at 10 A g−1) and cycling stability (90.5% capacity retention after 1000 cycles) were achieved.
The cathode materials for ZIBs are plagued by active materials dissolution during cycling, resulting in significant capacity decay. Consequently, preventing cathode dissolution is a critical step in enhancing the electrochemical performance of ZIBs. For instance, the dissolution of Mn arises from disproportionation and irreversible phase changes for the Mn-Based oxides, resulting in the structural collapse and the rapid decay of battery capacity. To address the issue of Mn dissolution, various strategies have been investigated, including material doping, structural modifications, and coating (Figure 4A) (Nolis et al., 2018; Venkatkarthick et al., 2020). As for vanadium compound, soluble intermediate and the intimate contact between crystal water in V-based oxides and electrolyte contribute to vanadium dissolution (Figure 4B). This challenge can be alleviated by incorporating V2O5 sol into the electrolyte. Moreover, approaches such as ion pre-intercalation and ion-exchange induced phase transitions have demonstrated efficacy in further fortifying the crystal structure of the material, thereby enhancing its stability in aqueous solutions.
Figure 4. (A) TEM images of carbon-coated MnO2 (Islam et al., 2017) (B) The crystal structures of V2O5·nH2O (Yan et al., 2018) (C) Schematic illustration of the fabrication process of a pristine Zn3V2O7(OH)2·2H2O (ZVO) cathode and the HfO2-coated ZVO by ALD (Guo et al., 2019) (D) Schematic illustration of reversible Zn2+ intercalation/deintercalation during the electrochemical discharge/charge process in Co0.247V2O5·0.944H2O (Ma et al., 2019).
The intercalation of H+ ions during discharge initiates the creation of basic zinc salts (BZS) on the surface of the cathode material, which are a type of layered double hydroxide (LDH). The precise composition and characteristics of the resulting BZS precipitate are contingent upon the electrolytes employed in battery operation. The emergence of by-products during battery cycling can give rise to several issues (Jia et al., 2020; Wang et al., 2021b). For instance, the by-products formed on the positive electrode surface hinder charge transfer at the electrolyte/cathode interface. Besides, irreversible charging and discharging processes resulting from the detachment and incomplete reversibility of ZSH formed by H+ intercalation. Therefore, building an artificial solid electrolyte interphase on the surface of the cathode materials can be effectively reduce the generation of by-products (Figure 4C).
As a type of multivalent battery, ZIBs faces challenges stemming from the strong electrostatic interaction between the cathode and Zn2+ ions, despite its inherent high specific capacity attributed to the involvement of multiple electrons in redox reactions. Compared with monovalent ions, Zn2+ ions own higher charge density, leading to electrostatic attraction with cathode materials, resulting in lower diffusion kinetics (Yong et al., 2020). Moreover, during deep charge/discharge cycles, a portion of Zn2+ ions tend to become trapped by electronegative atoms within the host lattice, inducing structural instability and reduced capacity in subsequent cycles. Introducing metal ions or water molecules into the cathode material layer offers a potential solution to alleviate the electrostatic interaction between multivalent Zn2+ ions and the host framework (Figure 4D). The method serves to decrease the high charge density of Zn2+ ions and reduce the activation energy required for charge transfer at the electrode interface. Furthermore, defect engineering can effectively decrease the electron density surrounding Zn2+ ions, thereby facilitating both ion diffusion and electron transfer processes.
ZIBs have garnered significant research attention due to their high safety, cost-effectiveness, material abundance, and superior ionic conductivity, showing immense promise for large-scale energy storage. In this review, we have summarized the significant protection methods of the Zn surface, including polymer interface, inorganic interface, alloying anode, and electrolyte additives. In addition, we have underscored the pivotal scientific challenges related to electrode materials and provided an overview of recent advancements in electrode design strategies for cathode materials.
Significant efforts have been devoted to engineering interfaces to protect Zn anodes. Firstly, the anode/electrolyte interface should be hydrophobic, acting as a waterproof coating. Additionally, it is crucial that the anode/electrolyte interface includes a rapid ion channel to facilitate ion diffusion. Therefore, moving forward, our focus should be on constructing an ideal interface with a thinner thickness, adequate mechanical strength, and stable chemical properties.
For the cathode, the fundamental mechanisms underlying electrochemical reactions in ZIBs, particularly with Mn-based cathodes, require further elucidation. As mentioned earlier, the Zn2+ storage mechanisms of Mn-based materials are currently contentious and subject to debate. Ambiguous interpretations may arise due to the lack of rigorous protocols and standardized characterization techniques, impeding the effective design and investigation of high-performance electrodes and electrolytes for ZIBs. There is an urgent need to employ multiple electrochemical approaches, advanced characterization techniques, and precise theoretical calculations to address this challenge.
We hope that this review serves as a catalyst for the dissemination of insights garnered from prior studies, fostering momentum towards bridging the disparity between the current state of ZIBs and the impending requirements for practical applications in the future.
JNY: Writing–original draft. HT: Writing–original draft. KXW: Writing–review and editing. JSC: Writing–review and editing.
The author(s) declare that financial support was received for the research, authorship, and/or publication of this article. This work was financially supported by Shanghai Municipal Science and Technology Major Project and the National Natural Science Foundation of China (22133005 and 21931005).
The authors declare that the research was conducted in the absence of any commercial or financial relationships that could be construed as a potential conflict of interest.
The author(s) declared that they were an editorial board member of Frontiers, at the time of submission. This had no impact on the peer review process and the final decision.
All claims expressed in this article are solely those of the authors and do not necessarily represent those of their affiliated organizations, or those of the publisher, the editors and the reviewers. Any product that may be evaluated in this article, or claim that may be made by its manufacturer, is not guaranteed or endorsed by the publisher.
Cao, J., Zhang, D., Zhang, X., Zeng, Z., Qin, J., Huang, Y. J. E., et al. (2022). Strategies of regulating Zn2+ solvation structures for dendrite-free and side reaction-suppressed zinc-ion batteries. Energy and Environ. Sci. 15, 499–528. doi:10.1039/D1EE03377H
Cao, Z., Zhuang, P., Zhang, X., Ye, M., Shen, J., and Ajayan, P. M. (2020). Strategies for dendrite-free anode in aqueous rechargeable zinc ion batteries. Adv. Energy Mater. 10, 2001599. doi:10.1002/aenm.202001599
Du, Y., Feng, Y., Li, R., Peng, Z., Yao, X., Duan, S., et al. (2023). Zinc-bismuth binary alloy enabling high-performance aqueous zinc ion batteries. Small, 2307848. doi:10.1002/smll.202307848
Fang, G., Zhou, J., Pan, A., and Liang, S. (2018). Recent advances in aqueous zinc-ion batteries. ACS Energy Lett. 3, 2480–2501. doi:10.1021/acsenergylett.8b01426
Feng, D., Jiao, Y., and Wu, P. J. A. C. (2023). Guiding Zn uniform deposition with polymer additives for long-lasting and highly utilized Zn metal anodes. Angew. Chem. Int. Ed. 62, e202314456. doi:10.1002/anie.202314456
Guo, J., Ming, J., Lei, Y., Zhang, W., Xia, C., Cui, Y., et al. (2019). Artificial solid electrolyte interphase for suppressing surface reactions and cathode dissolution in aqueous zinc ion batteries. ACS Energy Lett. 4, 2776–2781. doi:10.1021/acsenergylett.9b02029
Guo, Z., Fan, L., Zhao, C., Chen, A., Liu, N., Zhang, Y., et al. (2022). A dynamic and self-adapting interface coating for stable Zn-metal anodes. Adv. Mater. 34, 2105133. doi:10.1002/adma.202105133
He, P., Yan, M., Zhang, G., Sun, R., Chen, L., An, Q., et al. (2017). Layered VS2 nanosheet-based aqueous Zn ion battery cathode. Adv. Energy Mater. 7, 1601920. doi:10.1002/aenm.201601920
Hong, L., Wang, L.-Y., Wang, Y., Wu, X., Huang, W., Zhou, Y., et al. (2022a). Toward hydrogen-free and dendrite-free aqueous zinc batteries: formation of zincophilic protective layer on Zn anodes. Adv. Sci. 9, 2104866. doi:10.1002/advs.202104866
Hong, L., Wu, X., Liu, Y.-S., Yu, C., Liu, Y., Sun, K., et al. (2023a). Self-adapting and self-healing hydrogel interface with fast Zn2+ transport kinetics for highly reversible Zn anodes. Adv. Funct. Mater. 33, 2300952. doi:10.1002/adfm.202300952
Hong, L., Wu, X., Ma, C., Huang, W., Zhou, Y., Wang, K.-X., et al. (2021). Boosting the Zn-ion transfer kinetics to stabilize the Zn metal interface for high-performance rechargeable Zn-ion batteries. J. Mater. Chem. A 9, 16814–16823. doi:10.1039/D1TA03967A
Hong, L., Wu, X., Wang, L.-Y., Zhong, M., Zhang, P., Jiang, L., et al. (2022b). Highly reversible zinc anode enabled by a cation-exchange coating with Zn-ion selective channels. ACS Nano 16, 6906–6915. doi:10.1021/acsnano.2c02370
Hong, S., Jin, S., Deng, Y., Garcia-Mendez, R., Kim, K.-i., Utomo, N., et al. (2023b). Efficient scalable hydrothermal synthesis of MnO2 with controlled polymorphs and morphologies for enhanced battery cathodes. ACS Energy Lett. 8, 1744–1751. doi:10.1021/acsenergylett.3c00018
Hu, Z., Zhang, F., Zhao, Y., Wang, H., Huang, Y., Wu, F., et al. (2022). A self-regulated electrostatic shielding layer toward dendrite-free Zn batteries. Adv. Mater. 34, 2203104. doi:10.1002/adma.202203104
Hurlbutt, K., Wheeler, S., Capone, I., and Pasta, M. (2018). Prussian blue analogs as battery materials. Joule 2, 1950–1960. doi:10.1016/j.joule.2018.07.017
Islam, S., Alfaruqi, M. H., Song, J., Kim, S., Pham, D. T., Jo, J., et al. (2017). Carbon-coated manganese dioxide nanoparticles and their enhanced electrochemical properties for zinc-ion battery applications. J. Energy Chem. 26, 815–819. doi:10.1016/j.jechem.2017.04.002
Jia, X., Liu, C., Neale, Z. G., Yang, J., and Cao, G. (2020). Active materials for aqueous zinc ion batteries: synthesis, crystal structure, morphology, and electrochemistry. Chem. Rev. 120, 7795–7866. doi:10.1021/acs.chemrev.9b00628
Jiao, Y., Li, F., Jin, X., Lei, Q., Li, L., Wang, L., et al. (2021). Engineering polymer glue towards 90% zinc utilization for 1000 hours to make high-performance Zn-ion batteries. Adv. Funct. Mater. 31, 2107652. doi:10.1002/adfm.202107652
Lee, W. S. V., Xiong, T., Wang, X., and Xue, J. (2021). Unraveling MoS2 and transition metal dichalcogenides as functional zinc-ion battery cathode: a perspective. Small Methods 5, 2000815. doi:10.1002/smtd.202000815
Li, C., Xie, X., Liang, S., and Zhou, J. (2020a). Issues and future perspective on zinc metal anode for rechargeable aqueous zinc-ion batteries. Energy and Environ. Mater. 3, 146–159. doi:10.1002/eem2.12067
Li, G., Sun, L., Zhang, S., Zhang, C., Jin, H., Davey, K., et al. (2023a). Developing cathode materials for aqueous zinc ion batteries: challenges and practical prospects. Adv. Funct. Mater. 34, 2301291. doi:10.1002/adfm.202301291
Li, J., Yin, X., Duan, F., Ba, J., Wu, M., Zhao, K., et al. (2023b). Pure amorphous and ultrathin phosphate layer with superior ionic conduction for zinc anode protection. ACS Nano 17, 20062–20072. doi:10.1021/acsnano.3c05640
Li, J., Zhou, S., Chen, Y., Meng, X., Azizi, A., He, Q., et al. (2023c). Self-smoothing deposition behavior enabled by beneficial potential compensating for highly reversible Zn-metal anodes. Adv. Funct. Mater. 33, 2307201. doi:10.1002/adfm.202307201
Li, S., Fu, J., Miao, G., Wang, S., Zhao, W., Wu, Z., et al. (2021a). Toward planar and dendrite-free Zn electrodepositions by regulating Sn-crystal textured surface. Adv. Mater. 33, 2008424. doi:10.1002/adma.202008424
Li, S., Liu, Y., Zhao, X., Cui, K., Shen, Q., Li, P., et al. (2021b). Molecular engineering on MoS2 enables large interlayers and unlocked basal planes for high-performance aqueous Zn-ion storage. Angew. Chem. Int. Ed. 60, 20286–20293. doi:10.1002/anie.202108317
Li, W., Wang, K., and Jiang, K. (2020b). A low cost aqueous Zn–S battery realizing ultrahigh energy density. Adv. Sci. 7, 2000761. doi:10.1002/advs.202000761
Li, Y., Zhao, J., Hu, Q., Hao, T., Cao, H., Huang, X., et al. (2022). Prussian blue analogs cathodes for aqueous zinc ion batteries. Mater. Today Energy 29, 101095. doi:10.1016/j.mtener.2022.101095
Liu, H., Wang, J. G., Hua, W., Sun, H., Huyan, Y., Tian, S., et al. (2021a). Building ohmic contact interfaces toward ultrastable Zn metal anodes. Adv. Sci. 8, 2102612. doi:10.1002/advs.202102612
Liu, M., Zhao, Q., Liu, H., Yang, J., Chen, X., Yang, L., et al. (2019). Tuning phase evolution of β-MnO2 during microwave hydrothermal synthesis for high-performance aqueous Zn ion battery. Nano Energy 64, 103942. doi:10.1016/j.nanoen.2019.103942
Liu, W., Zhang, X., Huang, Y., Jiang, B., Chang, Z., Xu, C., et al. (2021b). β-MnO2 with proton conversion mechanism in rechargeable zinc ion battery. J. Energy Chem. 56, 365–373. doi:10.1016/j.jechem.2020.07.027
Liu, Z., Wang, R., Gao, Y., Zhang, S., Wan, J., Mao, J., et al. (2023). Low-cost multi-function electrolyte additive enabling highly stable interfacial chemical environment for highly reversible aqueous zinc ion batteries. Adv. Funct. Mater. 33, 2308463. doi:10.1002/adfm.202308463
Lv, T., Peng, Y., Zhang, G., Jiang, S., Yang, Z., Yang, S., et al. (2023). How about vanadium-based compounds as cathode materials for aqueous zinc ion batteries? Adv. Sci. 10, 2206907. doi:10.1002/advs.202206907
Ma, L., Li, N., Long, C., Dong, B., Fang, D., Liu, Z., et al. (2019). Achieving both high voltage and high capacity in aqueous zinc-ion battery for record high energy density. Adv. Funct. Mater. 29, 1906142. doi:10.1002/adfm.201906142
Nolis, G. M., Adil, A., Yoo, H. D., Hu, L., Bayliss, R. D., Lapidus, S. H., et al. (2018). Electrochemical reduction of a spinel-type manganese oxide cathode in aqueous electrolytes with Ca2+ or Zn2+. J. Phys. Chem. C 122, 4182–4188. doi:10.1021/acs.jpcc.7b12084
Pan, H., Shao, Y., Yan, P., Cheng, Y., Han, K. S., Nie, Z., et al. (2016). Reversible aqueous zinc/manganese oxide energy storage from conversion reactions. Nat. Energy 1, 16039. doi:10.1038/nenergy.2016.39
Qi, Y., Liao, M., Xie, Y., Chen, J., Dong, X., Wang, Y., et al. (2023). Long-life vanadium oxide cathode for zinc battery enabled by polypyrrole intercalation and concentrated electrolyte. Chem. Eng. J. 470, 143971. doi:10.1016/j.cej.2023.143971
Qian, S., Zhou, J., Peng, M., Qian, Y., Meng, Y., Jiang, Y., et al. (2022). A lewis acidity adjustable organic ammonium cation derived robust protecting shield for stable aqueous zinc-ion batteries by inhibiting the tip effect. Mater. Chem. Front. 6, 901–907. doi:10.1039/D1QM01604K
Qiu, H., Du, X., Zhao, J., Wang, Y., Ju, J., Chen, Z., et al. (2019). Zinc anode-compatible in-situ solid electrolyte interphase via cation solvation modulation. Nat. Commun. 10, 5374. doi:10.1038/s41467-019-13436-3
Schmuch, R., Wagner, R., Hörpel, G., Placke, T., and Winter, M. (2018). Performance and cost of materials for lithium-based rechargeable automotive batteries. Nat. Energy 3, 267–278. doi:10.1038/s41560-018-0107-2
Shuai, H., Liu, R., Li, W., Yang, X., Lu, H., Gao, Y., et al. (2023). Recent advances of transition metal sulfides/selenides cathodes for aqueous zinc-ion batteries. Adv. Energy Mater. 13, 2202992. doi:10.1002/aenm.202202992
Sun, W., Wang, F., Hou, S., Yang, C., Fan, X., Ma, Z., et al. (2017). Zn/MnO2 battery chemistry with H+ and Zn2+ coinsertion. J. Am. Chem. Soc. 139, 9775–9778. doi:10.1021/jacs.7b04471
Tan, Y., Li, S., Zhao, X., Wang, Y., Shen, Q., Qu, X., et al. (2022). Unexpected role of the interlayer “dead Zn2+” in strengthening the nanostructures of VS2 cathodes for high-performance aqueous Zn-ion storage. Adv. Energy Mater. 12, 2104001. doi:10.1002/aenm.202104001
Tian, H., Yang, J. L., Deng, Y., Tang, W., Liu, R., Xu, C., et al. (2023). Steel anti-corrosion strategy enables long-cycle Zn anode. Adv. Energy Mater. 13, 2202603. doi:10.1002/aenm.202202603
Venkatkarthick, R., Rodthongkum, N., Zhang, X., Wang, S., Pattananuwat, P., Zhao, Y., et al. (2020). Vanadium-based oxide on two-dimensional vanadium carbide MXene (V2Ox@V2CTx) as cathode for rechargeable aqueous zinc-ion batteries. ACS Appl. Energy Mater. 3, 4677–4689. doi:10.1021/acsaem.0c00309
Wang, D., Liang, W., He, X., Yang, Y., Wang, S., Li, J., et al. (2023a). V2O3@C microspheres as the high-performance cathode materials for advanced aqueous zinc-ion storage. ACS Appl. Mater. Interfaces 15, 20876–20884. doi:10.1021/acsami.2c21763
Wang, H., Zhou, A., Hu, X., Hu, Z., Zhang, F., Huang, Y., et al. (2023b). Bifunctional dynamic adaptive interphase reconfiguration for zinc deposition modulation and side reaction suppression in aqueous zinc ion batteries. ACS Nano 17 (12), 11946–11956. doi:10.1021/acsnano.3c04155
Wang, T., Li, S., Weng, X., Gao, L., Yan, Y., Zhang, N., et al. (2023c). Ultrafast 3D hybrid-ion transport in porous V2O5 cathodes for superior-rate rechargeable aqueous zinc batteries. Adv. Energy Mater. 13, 2204358. doi:10.1002/aenm.202204358
Wang, X., Meng, J., Lin, X., Yang, Y., Zhou, S., Wang, Y., et al. (2021a). Stable zinc metal anodes with textured crystal faces and functional zinc compound coatings. Adv. Funct. Mater. 31, 2106114. doi:10.1002/adfm.202106114
Wang, X., Zhang, Z., Xi, B., Chen, W., Jia, Y., Feng, J., et al. (2021b). Advances and perspectives of cathode storage chemistry in aqueous zinc-ion batteries. ACS Nano 15, 9244–9272. doi:10.1021/acsnano.1c01389
Wu, P., Xu, L., Xiao, X., Ye, X., Meng, Y., and Liu, S. (2024). An industrially applicable passivation strategy for significantly improving cyclability of zinc metal anodes in aqueous batteries. Adv. Mater. 36, 2306601. doi:10.1002/adma.202306601
Xu, C., Li, B., Du, H., and Kang, F. (2012). Energetic zinc ion chemistry: the rechargeable zinc ion battery. Angew. Chem. Int. Ed. 51, 933–935. doi:10.1002/anie.201106307
Xu, X., Chen, Y., Zheng, D., Ruan, P., Cai, Y., Dai, X., et al. (2021). Ultra-fast and scalable saline immersion strategy enabling uniform Zn nucleation and deposition for high-performance Zn-ion batteries. Small 17, 2101901. doi:10.1002/smll.202101901
Yan, M., He, P., Chen, Y., Wang, S., Wei, Q., Zhao, K., et al. (2018). Water-lubricated intercalation in V2O5·nH2O for high-capacity and high-rate aqueous rechargeable zinc batteries. Adv. Mater. 30, 1703725. doi:10.1002/adma.201703725
Yang, G., Liang, Z., Li, Q., Li, Y., Tian, F., and Wang, C. (2023). Epitaxial core–shell MnFe prussian blue cathode for highly stable aqueous zinc batteries. ACS Energy Lett. 8, 4085–4095. doi:10.1021/acsenergylett.3c01603
Yang, J., Xu, Q., Zheng, Y., Tian, Z., Shi, Y., Ma, C., et al. (2022). Phase engineering of metastable transition metal dichalcogenides via ionic liquid assisted synthesis. ACS Nano 16 (9), 15215–15225. doi:10.1021/acsnano.2c06549
Yao, H., Yu, H., Zheng, Y., Li, N. W., Li, S., Luan, D., et al. (2023). Pre-intercalation of ammonium ions in layered δ-MnO2 nanosheets for high-performance aqueous zinc-ion batteries. Angew. Chem. Int. Ed. 62, e202315257. doi:10.1002/anie.202315257
Ye, J.-J., Li, P.-H., Zhang, H.-R., Song, Z.-Y., Fan, T., Zhang, W., et al. (2023a). Manipulating oxygen vacancies to spur ion kinetics in V2O5 structures for superior aqueous zinc-ion batteries. Adv. Funct. Mater. 33, 2305659. doi:10.1002/adfm.202305659
Ye, P., Li, X., He, K., Dou, A., Wang, X., Naveed, A., et al. (2023b). A semi-interpenetrating network polymer coating for dendrite-free Zn anodes. J. Power Sources 558, 232622. doi:10.1016/j.jpowsour.2022.232622
Yong, B., Ma, D., Wang, Y., Mi, H., He, C., and Zhang, P. (2020). Understanding the design principles of advanced aqueous zinc-ion battery cathodes: from transport kinetics to structural engineering, and future perspectives. Adv. Energy Mater. 10, 2002354. doi:10.1002/aenm.202002354
Zeng, Y., Xu, J., Wang, Y., Li, S., Luan, D., and Lou, X. W. (2022). Formation of CuMn prussian blue analog double-shelled nanoboxes toward long-life Zn-ion batteries. Angew. Chem. Int. Ed. 61, e202212031. doi:10.1002/anie.202212031
Zhang, N., Cheng, F., Liu, J., Wang, L., Long, X., Liu, X., et al. (2017). Rechargeable aqueous zinc-manganese dioxide batteries with high energy and power densities. Nat. Commun. 8, 405. doi:10.1038/s41467-017-00467-x
Zhang, N., Ji, Y.-R., Wang, J.-C., Wang, P.-F., Zhu, Y.-R., and Yi, T.-F. (2023). Understanding of the charge storage mechanism of MnO2-based aqueous zinc-ion batteries: reaction processes and regulation strategies. J. Energy Chem. 82, 423–463. doi:10.1016/j.jechem.2023.03.052
Zhang, Q., Luan, J., Fu, L., Wu, S., Tang, Y., Ji, X., et al. (2019). The three-dimensional dendrite-free zinc anode on a copper mesh with a zinc-oriented polyacrylamide electrolyte additive. Angew. Chem. Int. Ed. 58, 15841–15847. doi:10.1002/anie.201907830
Zhang, Q., Luan, J., Tang, Y., Ji, X., and Wang, H. J. A. C. I. E. (2020). Interfacial design of dendrite-free zinc anodes for aqueous zinc-ion batteries. Angew. Chem. Int. Ed. 59, 13180–13191. doi:10.1002/anie.202000162
Zhang, S., Liu, Y., Fan, Q., Zhang, C., Zhou, T., Kalantar-Zadeh, K., et al. (2021). Liquid metal batteries for future energy storage. Energy and Environ. Sci. 14, 4177–4202. doi:10.1039/D1EE00531F
Zhao, Y., Guo, S., Chen, M., Lu, B., Zhang, X., Liang, S., et al. (2023a). Tailoring grain boundary stability of zinc-titanium alloy for long-lasting aqueous zinc batteries. Nat. Commun. 14, 7080. doi:10.1038/s41467-023-42919-7
Zhao, Y., Hong, H., Zhong, L., Zhu, J., Hou, Y., Wang, S., et al. (2023b). Zn-rejuvenated and SEI-regulated additive in zinc metal battery via the iodine post-functionalized zeolitic imidazolate framework-90. Adv. Energy Mater. 13, 2300627. doi:10.1002/aenm.202300627
Zhao, Z., Zhao, J., Hu, Z., Li, J., Li, J., Zhang, Y., et al. (2019). Long-life and deeply rechargeable aqueous Zn anodes enabled by a multifunctional brightener-inspired interphase. Energy and Environ. Sci. 12, 1938–1949. doi:10.1039/C9EE00596J
Zhou, L., Yang, F., Zeng, S., Gao, X., Liu, X., Cao, X., et al. (2022). Zincophilic Cu sites induce dendrite-free Zn anodes for robust alkaline/neutral aqueous batteries. Adv. Funct. Mater. 32, 2110829. doi:10.1002/adfm.202110829
Zhu, C., Li, P., Xu, G., Cheng, H., and Gao, G. J. C. C. R. (2023). Recent progress and challenges of Zn anode modification materials in aqueous Zn-ion batteries. Coord. Chem. Rev. 485, 215142. doi:10.1016/j.ccr.2023.215142
Keywords: aqueous zinc-ion battery, Zn anode, cathodes, optimization strategy, anode protection
Citation: Yang J-N, Tian H, Wang K-X and Chen J-S (2024) 10 Years of Frontiers in materials: interface engineering for aqueous zinc-ion batteries. Front. Mater. 11:1376865. doi: 10.3389/fmats.2024.1376865
Received: 26 January 2024; Accepted: 10 April 2024;
Published: 24 April 2024.
Edited by:
Nicola Maria Pugno, University of Trento, ItalyReviewed by:
Jian Wang, University of Science and Technology Liaoning, ChinaCopyright © 2024 Yang, Tian, Wang and Chen. This is an open-access article distributed under the terms of the Creative Commons Attribution License (CC BY). The use, distribution or reproduction in other forums is permitted, provided the original author(s) and the copyright owner(s) are credited and that the original publication in this journal is cited, in accordance with accepted academic practice. No use, distribution or reproduction is permitted which does not comply with these terms.
*Correspondence: Kai-Xue Wang, ay53YW5nQHNqdHUuZWR1LmNu; Jie-Sheng Chen, Y2hlbWNqQHNqdHUuZWR1LmNu
†These authors have contributed equally to this work
Disclaimer: All claims expressed in this article are solely those of the authors and do not necessarily represent those of their affiliated organizations, or those of the publisher, the editors and the reviewers. Any product that may be evaluated in this article or claim that may be made by its manufacturer is not guaranteed or endorsed by the publisher.
Research integrity at Frontiers
Learn more about the work of our research integrity team to safeguard the quality of each article we publish.