- 1Aramco Research Center, Moscow, Russia
- 2Emanuel Institute of Biochemical Physics, Russian Academy of Sciences, Moscow, Russia
- 3Plekhanov Russian University of Economics, Moscow, Russia
- 4Laboratory of Inorganic Nanomaterials, National University of Science and Technology MISIS, Moscow, Russia
- 5Reservoir Engineering Technology Division at Exploration and Petroleum Engineering Center - Advanced Research Center (EXPEC ARC) ARC, Dhahran, Saudi Arabia
The development of hydrogen energy is capable of solving a number of important issues that modern society is facing, including global warming and various environmental impacts. Currently, there is an intensive search for natural sources of hydrogen as well as low-carbon techniques for mass production of hydrogen from natural gas, associated petroleum gas, and water. In parallel, efforts to develop technologies for the subsequent management of hydrogen are underway, and the creation of its safe and efficient storage is one of the highest priority goals. For the transportation and storage of hydrogen today, a number of solutions are offered, each of which has both positive and negative aspects. The boron nitride family of materials with high thermal and chemical stability, variability of morphologies, and flexibility of structure has been considered as a candidate for efficient hydrogen storage. This review offers to familiarize readers with the progress in the research and application of hexagonal boron nitride (h-BN), as well as BN-based materials in comparison with other materials, as promising hydrogen storage. Experimental and theoretical data obtained for different morphologies and internal structures were reviewed in relevance to the material`s sorption capacity with respect to hydrogen. Various approaches to improve the efficiency of hydrogen storage were analyzed, and the highest storage capabilities published were mentioned. Thus, BN-based materials are very promising as hydrogen storage, even for an automotive application, but the development of new mass production technologies should be carried out.
1 Introduction
Nowadays, industrial sectors are working hard to find ways to reduce greenhouse gas (GHG) emissions and stop climate change. In 2022, the production, transportation, and processing of oil and gas released 5.1 billion tons (Gt) of CO2-eq. These emissions, categorized as “scopes 1 and 2,” contributed to almost 15% of the overall energy-related greenhouse gas (GHG) emissions. Furthermore, the utilization of oil and gas resulted in an additional 40% of emissions (IEA, 2023). The IEA’s Net Zero Emissions by 2050 (NZE) Scenario assumes an immediate, concerted effort by all the oil and gas industry to limit emissions from its activities. GHG emissions can be cut down in industries where hydrogen is used as an energy source. When burned, it emits no carbon dioxide or other carbon-based gases, and when produced via electrolysis using renewable energy, it has a comparatively low carbon footprint. The majority of hydrogen is currently produced using fossil fuels, but a rapid transition to renewable hydrogen is ongoing, and it is projected that both the overall amount of hydrogen produced and the share of renewable hydrogen will expand considerably over the next several decades (IEA, 2019).
As it was demonstrated in Figure 1A, there are five basic approaches used to achieve a reduction in emissions intensities: tackling methane emissions, eliminating all non-emergency flaring, electrifying upstream facilities with low-emissions electricity, equipping oil and gas processes with carbon capture utilization and storage (CCUS), and expanding the use of low-emission hydrogen in refineries (IEA, 2023).
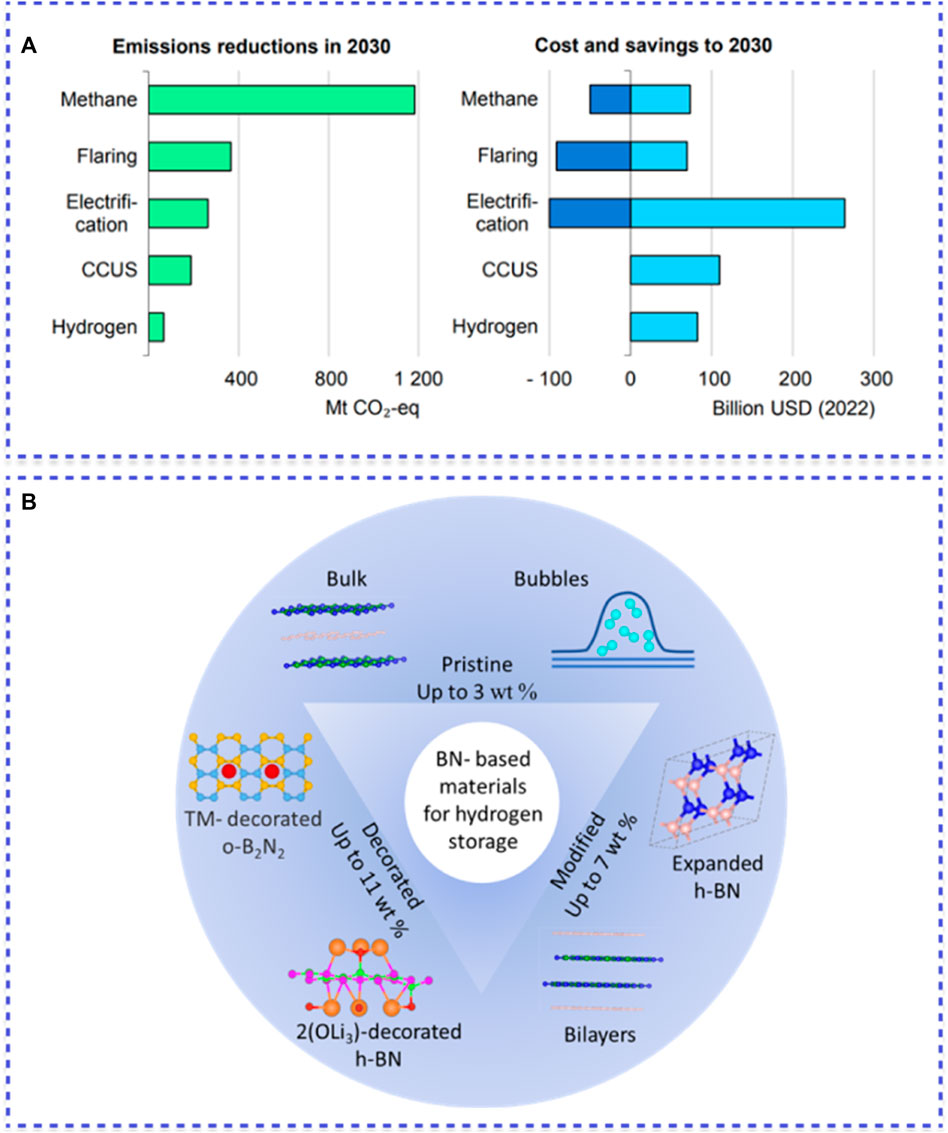
Figure 1. (A) Estimated reduction in emissions and total cost and savings accrued resulting from the adoption of measures to curtail emissions from oil and gas operations in the NZE scenario by 2030 [1]; (B) Key trends in the development of hydrogen storage in BN-based materials.
Thus, the development of hydrogen energy is one of the most promising solutions to the issue of massive GHG emissions. The difficulties of hydrogen energy distribution can be solved by developing methods for its production, transport, and storage at a reasonably low cost. One of the most important and unsolved problems in the scaling of the application of hydrogen energy is the development of attractive options to store hydrogen. In general, there are two types of hydrogen storage methods: physical methods that involve the compression of hydrogen and possibly converting it into a liquid form, and chemical methods that rely on strong interactions between hydrogen molecules or atoms and the accumulating materials. In particular, for the second-type methods, solid adsorbing materials are common, along with liquid organic hydrogen carriers (LOHC). It was demonstrated that the adsorption energy of H2 molecules must be between 0.1 and 0.8 eV in order to create a solid material with the high-density capability of storing hydrogen under standard pressure and temperature conditions (Jena, 2011).
Storing efficiency is especially important for facilities used in transport. For automotive applications, hydrogen storage capacity of about 6.5 wt% of the mass of the storage system is set as the ultimate goal by the U.S. Department of Energy (DOE) (U.S. Department of Energy, 2022). Even though LaNi5H6 is employed as a hydrogen storage material, it is capable of accumulating only 1 wt% of H2 molecules due to the extremely high atomic weights of La and Ni (Schlapbach and Züttel, 2001). On the other hand, carbon-based materials, which are made of light elements such as boron, carbon, and nitrogen, might be able to store a significantly higher amount of hydrogen than metal hydrides. Several studies focused on the usage of carbon nanotubes, fullerenes, and nanomaterials for hydrogen storage have been shown in the past decade. For example, it has been reported that multi-walled carbon nanotubes can absorb hydrogen in amounts up to 4.6 wt% (Schlapbach and Züttel, 2001).
Carbon-based materials, such as porous graphene (Wang et al., 2019; Huo et al., 2021), carbon-nitrogen composites (Bafekry et al., 2019; Varunaa and Ravindran, 2019), covalent organic frameworks (COF) (Cao et al., 2009; Zhao et al., 2020; Shangguan et al., 2021), and metal-organic frameworks (MOF) (Rosi et al., 2003; Sengupta et al., 2023), according to their sorption capacity for hydrogen, show promising characteristics and were previously studied as potential hydrogen storage materials. However, some physicochemical properties and peculiarities of hydrogenation, dehydrogenation, and cyclization processes significantly limit the prospects of their use. Moreover, many of these materials are not stable in ambient conditions in the presence of moisture (Hamilton et al., 2009).
Recently, h-BN structures have also been considered as promising materials for hydrogen storage applications (Tozzini and Pellegrini, 2013). Hexagonal boron nitride is an isostructural graphene counterpart, and the polarity of B-N bonds and partially ionic bond nature make it efficient for hydrogen storage (Li et al., 2022). Complementary modification of h-BN-based structures can additionally extend the capability of the materials in hydrogen storage applications. An extensive review of the theoretical and experimental studies presenting the possibilities of h-BN in relation to hydrogen sorption is presented in this paper. Our manuscript first focuses on the main results, representing the topical developments and achievements in this field. Then a comprehensive comparison and analysis of the mentioned results are presented, and conclusions are made.
2 Outstanding features of hexagonal boron nitride-based materials
H-BN-based nanomaterials, like their carbon counterparts, exhibit an extended surface area and high porosity. Due to the high chemical inertia and thermodynamic stability of h-BN (up to 1273 K in air), as well as the high specific surface area of nanoparticles and B-N polar covalent bonds, this material is of great interest in the issue of its various applications, including usage as hydrogen storage. Traditionally, h-BN materials are considered efficient hydrogen storage operating via chemisorption mechanisms, but recent theoretical studies increasingly show the promise of BN-based structures for high hydrogen physisorption (Cheng et al., 2007; Salazar-Aparicio et al., 2014; Azimirad et al., 2016). As a highly stable hydrogen storage material, h-BN can provide improved performance, cycling, and regeneration. But one of the main limitations in the industrial application of hexagonal boron nitride is the lack of developed techniques for cheap mass production of nanostructures of this material, as well as simple and effective approaches for their modification to increase sorption capacity. Outstanding properties of this material, along with the capacity of its structure with respect to hydrogen, are described in (Lim et al., 2007; Ebrahimi-nejad and Shokuhfar, 2013; Wang et al., 2013; Moussa et al., 2014a; Moussa et al., 2014b; Lei et al., 2014; Liu et al., 2014; Weng et al., 2014; Wang et al., 2017).
For instance, boron nitride nanotubes (BNNT) obtained by annealing ball-milled boron-nickel catalyst in a nitrogen/hydrogen gas mixture at 1298 K demonstrated the ability to sorb hydrogen at room temperature, with a predictable storage capacity up to 2.2 wt% under 6 MPa (Lim et al., 2007). The improved hydrogen storage of this material can be attributed to its nanoscale morphology and the presence of heteropolar B-N bonding. Through ionic B-N bonding, an extra dipole moment is generated, which aids in stronger hydrogen adsorption.
Nanostructured BN has unique physical and chemical properties when compared to bulk and microsized materials. For example, BN nanoparticles (BNNP) can be synthesized via a vapor-phase pyrolysis precursor, then tailored to the nanostructures by annealing at high temperatures (2273 K) (Moussa et al., 2014b). Then ball-milling of these nanostructures can be applied to improve the specific surface area. As a result, hollow-cored BNNP with a porous shell structure showing a specific surface area of 200.5 m2g−1 and a total pore volume of 0.287 cm3g−1, which is conducive to advanced hydrogen accumulation, can be obtained.
Another study (Lei et al., 2014) focuses on oxygen-doped BN nanosheets, which were synthesized through a sol-gel process. The obtained material demonstrated a storage capacity of 5.7 wt% under 5 MPa at room temperature, with each 3 × 3 supercell of BN monolayer capable of absorbing up to 6 H2 molecules. A theoretical study revealed that the enhanced hydrogen storage capability can be attributed to the oxygen-doping of BN nanosheets, with decreased adsorption distance between H2 molecules and O atoms compared with pure BN. Additional calculations (Liu et al., 2014) examined the structural durability of metal/BN and investigated metal adsorption sites on the layers. Specifically, the study explored the Na-doped BN layer and found that the electric field generated between the positively charged Na and negatively charged N played a crucial role in enhancing the binding energy between hydrogen and the complex, creating the polarization of hydrogen. As a result, the hydrogen storage ability of this layer was approximately 5.84 wt%.
The key trends in the development of hydrogen storage in BN-based materials are schematically presented in Figure 1B.
To estimate the sorption capacity of h-BN, Wang and colleagues (Wang et al., 2002) milled a commercial powder of BN in a hydrogen atmosphere at 10 bars of pressure and obtained 3-nm crystallites. These crystallites were able to uptake hydrogen in an amount less than 0.5 wt% for a milling time of 5 h. For a much longer milling time of about 80 h, the sorption capacity of h-BN increased up to 2.6 wt%. Indeed, this is quite an impressive result for such a simple method of obtaining material.
In addition to the highlighted material, porous h-BN is also considered for the physical storage of H2 molecules and cryo-adsorption. Such material was synthesized at 873 K according to a chlorine-assisted method and showed a specific surface area of 960 m2/g and a specific total pore volume of 0.68 cm3/g. Thanks to these textural properties, such material is capable of reversibly storing 1.01 wt% of hydrogen at 77 K and 1 bar (Kim et al., 2013). A higher specific surface area was reported for porous BN microbelts. The reversible hydrogen uptake of 2.3 wt% was achieved at 77 K and 10 bars by a sample with a specific surface area of 1,144 m2/g and a specific pore volume of 0.88 cm3/g (Weng et al., 2013). Shahsavari and Zhao (Shahsavari and Zhao, 2018) demonstrate a maximum gravimetric uptake of h-BN of 8.65 wt% at 300 K via ab initio multiscale simulations. This one has the highest hydrogen uptake on sorbents at room temperature without doping, confirming the positive effect of the specific surface area.
3 Main regularities in accumulating of hydrogen
The hydrogen storage capacities of materials are related to their specific surface areas and, respectively, morphologies. Higher specific surface areas favor faulty architectures with numerous open-edge layers on the external surface. And one of the high specific area promising morphologies of hexagonal boron nitride nanostructures for hydrogen absorption at room temperature is the nanotube. It was shown that with the BN nanotubes, chemisorption of hydrogen predominates (Ma et al., 2002a; Tang et al., 2002; Lim et al., 2007; Leela et al., 2010; Okan et al., 2012) over physisorption almost in all cases. As a result, higher energies are required to remove hydrogen (in the reverse dehydrogenation process). According to Ma et al. (2002a), the hydrogen adsorption on the multiwalled BN nanotubes with a specific surface area of 150 m2/g and on bamboo-like BN nanotubes with a specific surface area of 210 m2/g at 293 K and 100 bars was 1.8 wt% of hydrogen and 2.6 wt% of hydrogen, respectively. It has also been experimentally demonstrated that the hydrogen storage capacity of h-BN nanostructures increases with increasing hydrogen pressure. Thus, flower-type BN nanostructures (specific surface area of 180 m2/g) show a maximum hydrogen storage capacity of 2.5 wt% at around 100 bar hydrogen pressure, while straight-walled BNNTs (specific surface area of 210 m2/g) show an increased storage capacity of 2.7 wt% at the same conditions. In turn, the bamboo-type nanotubes with the maximum specific surface area of 230 m2/g have the highest hydrogen uptake capacity of 3.0 wt%.
Tang et al. (2002) looked at the capability to accumulate hydrogen by different BN structures at 10 MPa and room temperature. They found that collapsed BN nanotubes were better at increasing hydrogen adsorption capacity than conventional multiwalled nanotubes. Because of this, there were more dangling bonds, and the specific area of BN nanotubes dramatically increased from 254.2 m2/g to 789.1 m2/g. The amount of hydrogen adsorbed by the material consequently goes from 0.9 to 4.2 wt%.
The chemical functionalization of nanomaterials may also play a great role in their hydrogen sorption capacity. For example, oxygen-doped h-BN nanosheets with 2–6 layers that were made using the sol-gel method can accumulate up to 5.7 wt% of hydrogen at room temperature under 5 MPa (Lei et al., 2014). This material exhibits high cycling stability, up to 15 cycles in hydrogen uptake-release. Another paper (Zhang et al., 2015) shows the advantage of using one additional morphology of material based on hexagonal boron nitride - porous boron nitride structures (p-BN). Quantum-chemical calculations based on the first principle show that hydrogen storage of C-doped p-BN led to a maximum of 5.1 wt% hydrogen taken, and Li-doped p-BN adsorbs up to 7.5 wt% hydrogen. In addition, similar calculations can be performed for promising materials like lithium-functionalized BC2N monolayers that have been doped with boron and carbon (Qiu et al., 2014). Moreover, using the dispersion-corrected semi-empirical method, the hydrogen storage capability of BmNm nanocages was investigated, and the highest uptake of 12.01% was demonstrated for B96N96 (Kinal and Sayhan, 2016).
It is worth paying attention to the new structures based on boron nitride, the proposed theoretical ones. The theoretically proposed novel structure B20N24 (Zhao et al., 2023) developed through the CALYPSO code can be seen as a candidate for hydrogen storage. Results show that 19 H2 molecules absorbed by the structure correspond to 6.8 wt% of storing capacity. The theoretical insights into the possible use of pristine bilayer h-BN as a potential hydrogen storage medium by means of first-principles calculations (Rai et al., 2021) show the maximum storing ability up to 3.4 wt% with the calculated desorption temperature in the range from 1399 K to 279 K, depending on the specific number of H2 molecules absorbed. The theoretically predicated by DFT calculations orthorhombic diboron dinitride (o-B2N2) monolayer decorated by Ti atoms demonstrates 11.21 wt% capacity with a desorption temperature of 396 K (Chodvadiya et al., 2024). The novel theoretically designed TM-decorated B24N24 (TM = Sc, Ti) fullerenes have a hydrogen gravimetric density equal to 7.74 wt% and 7.50 wt% for Sc6B24N24 and Ti6B24N24, respectively, with a hydrogen release temperature in the range of 243–408 K (Ma et al., 2020).
Of course, such results obtained both by theoretical calculations and experimental studies encourage some optimism about the resolution of the issue of creating an effective hydrogen storage facility based on nanostructured h-BN.
With the use of the pseudopotential density functional theory (DFT) method, the effect of substitutional doping and structural defects on the hydrogen adsorption capability of boron nitride nanotubes was studied. As a result, a substantial enhancement of the binding energy compared to the perfect boron nitride structure was revealed. It was also shown that BN nanotubes have about 40% larger binding energy compared to carbon nanotubes, the diffusion of hydrogen is slower in small-diameter BN nanotubes than in larger-diameter ones, and the desorption temperature is expected to be about 123 K (Jhi and Kwon, 2004). The effect of Pt modification of the BN nanotubes, resulting in a high average adsorption energy of −0.365 eV for hydrogen molecules, was studied via DFT calculations in (Wu et al., 2006). It was shown that the adsorption of a hydrogen molecule weakens the interaction between the Pt atom and the BN nanotube. While two Pt atoms are doped on the BN tube, the creation of a Pt dimer weakens the Pt-BN connection and reduces the adsorption energy of a hydrogen molecule on the Pt dimer. In addition, the structure, stability, and hydrogen storage efficiency of hydrogenated h-BN sheets doped with lithium have been theoretically explored in (Banerjee et al., 2016). Li atoms on the h-BN sheets have been found to act as binding sites to absorb up to 6 wt% of hydrogen at a lower temperature (<198 K). At the same time, the ab initio simulation indicates a favorable hydrogen desorption temperature of ∼398 K. In addition to pure lithium, its compounds also show themselves to be good modifiers for increasing the hydrogen-storing capacity of h-BN. The calculated hydrogen gravimetric density of 2(OLi3)-decorated h-BN for H2 molecules storage can reach 9.67 wt% (Zhang et al., 2023). The average adsorption energy per H2 molecule is −0.175 eV, which falls in the ideal window for reversible uptake-release at ambient temperatures.
However, as theoretical studies of carbon-based materials have shown, metal additives can also lead to the aggregation of metal clusters, which can ultimately reduce the adsorption capacity of the materials with respect to hydrogen (Kim et al., 2008; Panigrahi et al., 2021). The first-principle quantum calculations presented in (Venkataramanan et al., 2009) demonstrate favorable absorption sites for Ni and Rh atoms on the BN sheets and the related hydrogen adsorption rate. In Ni-doped BN sheets, a strong chemical bond develops between the nitrogen and Ni atoms; however, in Rh-doped BN sheets, both boron and nitrogen bonds with the Rh atoms were discovered. Both Ni and Rh atoms can chemically absorb up to three hydrogen molecules, and the distance between the metal atom and the BN sheet increases along with the increase in the number of hydrogen molecules.
The capacity of hydrogen adsorption of 4.2 wt% was achieved on composites of Ti powder mechanically milled with h-BN at a weight ratio of 1:1. This value is equal to the theoretically calculated hydrogen absorption of Ti, which can testify that in the tested mixture, Ti mainly absorbs hydrogen (Kondo et al., 2005). In another study (Chen et al., 2012), the adsorption of transition metals such as Sc, Ti, V, Cr, Mn, Fe, Co, and Ni on carbon-doped h-BN sheets and the cage B12N12 was investigated using DFT calculations. Sc, V, Cr, and Mn were energetically favorable to be dispersed on the CN-BN sheet and cage and could uptake up to 6 wt% of hydrogen. As was demonstrated, the carbon dopants in h-BN served as potential wells for trapping metal atoms and preventing their clustering.
The novel approach for hydrogen storage in bubbles of pure h-BN (appeared bulging structures on the h-BN surface) via plasma treatment was proposed (He et al., 2019). Such bubble-type structures produced by the methane plasma have a diameter ranging from 2 to 4 μm and a height of up to 8.5 nm. The stability of the hydrogen bubbles based on geometric evolutions shows the diameters of all the bubbles tended to be stable after about 30 weeks under ambient conditions. This is a promising method for preserving hydrogen, but its extraction remains unsolved.
A comparison of different BN-based materials in their ability to accumulate hydrogen is presented in Table 1.
4 Hydrogen release and recycling
There are few data on the hydrogenation and dehydrogenation of hexagonal boron nitride available in the literature, and these are mostly molecular modeling studies. Especially, it makes sense to perform additional experimental modeling devoted to the investigation of hydrogen release processes from h-BN-based materials. Most publications, though, do not disclose the information about whether the BN materials can be used again after the dehydrogenation/desorption process or how their properties change after keeping and releasing hydrogen at the corresponding desorption temperatures. Also, there are some reports stating that the thermal release of hydrogen from BN materials is slow and inefficient and that catalysts are required to speed up the process. Since the value of a material’s ability to hold hydrogen is the most important parameter, there is an abundance of information in the literature related to changes in the structure of hexagonal boron nitride to increase the effectiveness of hydrogen accumulation more than release.
The destruction of strong bonds between atoms of BN-based materials and chemisorbed hydrogen (including hydrogen from hydrogenated functional groups) sometimes requires additional energy to release hydrogen after storage. Since then, high-temperature treatment should be applied to storage materials to emit stored hydrogen. For example, nanostructured h-BN powder, which is able to accumulate about 2.6 wt% of hydrogen, can release all hydrogen only at a temperature of 570 K (Wang et al., 2002). At the same time, chemisorbed hydrogen can be released from boron nitride nanotubes at temperatures above 623 K. But triflic acid can catalyze this process, making the dehydrogenation process effective at a slightly elevated temperature (313–323 K) (Roy et al., 2014). Additionally, the proposed mechanism leads to triflic acid regeneration during the process, ensuring total accumulated hydrogen release after some time. In comparison, collapsed Pt-catalyzed BN nanotubes release 5% of adsorbed hydrogen in the temperature range from 353 to 413 K and another 95% in the temperature range from 573 to 723 K (Tang et al., 2002). BN nanofibers with diameters ranging from 30 to 100 nm and lengths of several µm and a hydrogen storage capacity of about 2.9 wt% (at 293 K and 100 bars) are capable of releasing only 20 wt% of accumulated hydrogen at room temperature (physisorbed fraction) (Ma et al., 2002b). A temperature of 573 K is required to release chemisorbed hydrogen. Similar findings were presented in (Ma et al., 2002b), where BN bamboo-like nanotubes retained about 70% of the accumulated hydrogen after returning to ambient pressure, indicating predominantly chemical adsorption. The adsorbed hydrogen was fully released only when the sample was heated to 573 K. In subsequent cycles, the hydrogen uptake capacity remained stable, indicating reversible adsorption-desorption processes. This shows that bad architecture makes it easier for chemicals to interact with hydrogen, forming chemical bonds.
At the same time, additional experiments showed that about 89% of stored hydrogen can be released from oxygen-doped h-BN nanosheets at room temperature due to the decreasing pressure to the ambient value (Lei et al., 2014). Moreover, hydrogen uptake ability slightly changed from 5.7 to 4.79 wt% by fifteen cycles of hydrogen adsorption-desorption. However, molecular modeling performed in the same study showed only 5.5 wt% for maximum hydrogen absorption by an ideal O-doped BN structure. Quantum-chemical calculations based on the first principle predict that pristine boron nitride has a maximum reversible hydrogen storage capacity of 1.5 wt% under 5 MPa pressure and at room temperature (Tokarev et al., 2016). The same characteristic for O-doped boron nitride is a bit higher and equals 1.9 wt%.
5 Conclusion and perspectives
As can be seen from the literature analysis, both experimental and theoretical modeling data show the high potential of h-BN-based materials for effective hydrogen storage. In addition, theoretical modeling based on first-principles and DFT calculations demonstrates better results in hydrogen storage performance compared to the experimentally reproduced results. Nevertheless, it can be concluded that the best ways to improve the ability of h-BN materials to absorb hydrogen are to increase the effective specific surface area of the materials, correct the structure by making structural defects, possibly change the interplanar distance of layers, dope boron nitride with impurity elements (such as C and O), and form metal clusters on the surface (functionalization by Li, Ti, and others). Reviewed studies mention the hydrogen accumulation capabilities of h-BN-based materials in the range of 0.1–9.67 wt% at room temperature and increased pressure. Moreover, theoretical results are usually slightly overestimated compared to experimental ones. These numbers confirm that currently developed structures are suitable even for automotive applications. Thus, the best way to develop h-BN-based hydrogen storage systems with strong characteristics is to perform more experimental studies on the optimization of mass production of enhanced h-BN nanostructures. But to make the h-BN-based materials commercially attractive, further intensive research work devoted to the hydrogen release procedure and reuse of the materials should be proceed.
Author contributions
AK: Writing–original draft, Writing–review and editing. AMM: Writing–original draft, Writing–review and editing. PA: Writing–original draft, Writing–review and editing. ZP: Writing–original draft, Writing–review and editing. ATM: Writing–review and editing. AA-Q: Writing–review and editing.
Funding
The author(s) declare that no financial support was received for the research, authorship, and/or publication of this article.
Conflict of interest
The authors declare that the research was conducted in the absence of any commercial or financial relationships that could be construed as a potential conflict of interest.
Publisher’s note
All claims expressed in this article are solely those of the authors and do not necessarily represent those of their affiliated organizations, or those of the publisher, the editors and the reviewers. Any product that may be evaluated in this article, or claim that may be made by its manufacturer, is not guaranteed or endorsed by the publisher.
References
Azimirad, R., Bayani, A. H., and Safa, S. (2016). The effect of concentration of H 2 physisorption on the current – voltage characteristic of armchair BN nanotubes in CNT – BNNT – CNT set. Pramana - J. Phys. 87 (2), 46–47. doi:10.1007/s12043-016-1238-2
Bafekry, A., Ghergherehchi, M., Farjami Shayesteh, S., and Peeters, F. M. (2019). Adsorption of molecules on C3N nanosheet: a first-principles calculations. Chem. Phys. 526 (July 2019), 110442. doi:10.1016/j.chemphys.2019.110442
Banerjee, P., Pathak, B., Ahuja, R., and Das, G. P. (2016). First principles design of Li functionalized hydrogenated h-BN nanosheet for hydrogen storage. Int. J. Hydrogen Energy 41 (32), 14437–14446. doi:10.1016/j.ijhydene.2016.02.113
Cao, D., Lan, J., Wang, W., and Smit, B. (2009). Lithium-doped 3D covalent organic frameworks: high-capacity hydrogen storage materials. Angew. Chem. - Int. Ed. 48 (26), 4730–4733. doi:10.1002/anie.200900960
Chen, M., Zhao, Y. jun, Liao, J. hai, and bao, Y. X. (2012). Transition-metal dispersion on carbon-doped boron nitride nanostructures: applications for high-capacity hydrogen storage. Phys. Rev. B 86, 045459–45467. doi:10.1103/physrevb.86.045459
Cheng, J., Zhang, L., Ding, R., Ding, Z., Wang, X., and Wang, Z. (2007). Grand canonical Monte Carlo simulation of hydrogen physisorption in single-walled boron nitride nanotubes. Int. J. Hydrogen Energy 32, 3402–3405. doi:10.1016/j.ijhydene.2007.02.037
Chettri, B., Patra, P. K., Srivastava, S., Laref, A., and Rai, D. P. (2021). Enhanced H 2 Storage Capacity of Bilayer Hexagonal Boron Nitride (h-BN) Incorporating van der Waals Interaction under an Applied External Electric Field. ACS Omega 6, 22374–22382. doi:10.1021/acsomega.1c03154
Chodvadiya, D., Kanabar, S., Chakraborty, B., and Jha, P. K. (2024). Exploring the hydrogen storage possibility of the pristine, defected and metals decorated o-B2N2 monolayers: insights from DFT simulations. Int. J. Hydrogen Energy 53 (October 2023), 958–968. doi:10.1016/j.ijhydene.2023.11.326
Ebrahimi-nejad, S., and Shokuhfar, A. (2013). Compressive buckling of open-ended boron nitride nanotubes in hydrogen storage applications. Phys. E Low-dimensional Syst. Nanostructures. 50, 29–36. doi:10.1016/j.physe.2013.02.021
Fu, P., Wang, J., Jia, R., Bibi, S., Eglitis, R. I., and Zhang, H. xing (2017). Theoretical study on hydrogen storage capacity of expanded h-BN systems. Comput. Mater Sci. 139, 335–340. doi:10.1016/j.commatsci.2017.08.015
Hamilton, C. W., Baker, R. T., Staubitz, A., and Manners, I. (2009). B – N compounds for chemical hydrogen storage. Chem. Soc. Rev. 38, 279–293. doi:10.1039/b800312m
He, L., Wang, H., Chen, L., Wang, X., Xie, H., Jiang, C., et al. (2019). Isolating hydrogen in hexagonal boron nitride bubbles by a plasma treatment. Nat. Commun. 10 (1), 2815–2819. doi:10.1038/s41467-019-10660-9
Huo, Y., Zhang, Y., Wang, C., Fang, Y., Li, K., and Chen, Y. (2021). Boron-doping effect on the enhanced hydrogen storage of titanium-decorated porous graphene: a first-principles study. Int. J. Hydrogen Energy 46 (80), 40301–40311. doi:10.1016/j.ijhydene.2021.09.243
IEA (2023) Emissions from oil and gas operations in Net Zero transitions. A world energy outlook special and COP28.
Jena, P. (2011). Materials for hydrogen storage: past, present, and future. J. Phys. Chem. Lett. 2 (3), 206–211. doi:10.1021/jz1015372
Jhi, S. H., and Kwon, Y. K. (2004). Hydrogen adsorption on boron nitride nanotubes: a path to room-temperature hydrogen storage. Phys. Rev. B - Condens Matter Mater Phys. 69 (24), 245407–245414. doi:10.1103/physrevb.69.245407
Kim, G., Jhi, S. H., Park, N., Louie, S. G., and Cohen, M. L. (2008). Optimization of metal dispersion in doped graphitic materials for hydrogen storage. Phys. Rev. B - Condens Matter Mater Phys. 78 (8), 085408–85415. doi:10.1103/physrevb.78.085408
Kim, J., Han, J., Seo, M., Kang, S., Kim, D., and Ihm, J. (2013). High-surface area ceramic-derived boron-nitride and its hydrogen uptake properties. J. Mater Chem. A 1 (4), 1014–1017. doi:10.1039/c2ta00904h
Kinal, A., and Sayhan, S. (2016). Accurate prediction of hydrogen storage capacity of small boron nitride nanocages by dispersion corrected semi-empirical PM6-DH2 method. Int. J. Hydrogen Energy 41 (1), 392–400. doi:10.1016/j.ijhydene.2015.10.076
Kondo, T., Shindo, K., and Sakurai, Y. (2005). Comparison of hydrogen absorption properties of Ti mechanically milled with hexagonal boron nitride (h-BN) and with graphite. J. Alloys Compd. 386, 202–206. doi:10.1016/j.jallcom.2004.04.147
Leela, A., Reddy, M., Tanur, A. E., and Walker, G. C. (2010). Synthesis and hydrogen storage properties of different types of boron nitride nanostructures. Int. J. Hydrogen Energy 35 (9), 4138–4143. doi:10.1016/j.ijhydene.2010.01.072
Lei, W., Zhang, H., Wu, Y., Zhang, B., Liu, D., Qin, S., et al. (2014). Oxygen-doped boron nitride nanosheets with excellent performance in hydrogen storage. Nano Energy 6, 219–224. doi:10.1016/j.nanoen.2014.04.004
Li, J., Lin, J., Xu, X., Zhang, X., Xue, Y., Mi, J., et al. (2013). Porous boron nitride with a high surface area: hydrogen storage and water treatment. Nanotechnology 24 (15), 155603. doi:10.1088/0957-4484/24/15/155603
Li, M., Huang, G., Chen, X., Yin, J., Zhang, P., Yao, Y., et al. (2022). Perspectives on environmental applications of hexagonal boron nitride nanomaterials. Nano Today 44, 101486. doi:10.1016/j.nantod.2022.101486
Lim, S. H., Luo, J., Ji, W., and Lin, J. (2007). Synthesis of boron nitride nanotubes and its hydrogen uptake. Catal. Today 120, 346–350. doi:10.1016/j.cattod.2006.09.016
Liu, Y., Liu, W., Wang, R., Hao, L., and Jiao, W. (2014). Hydrogen storage using Na-decorated graphyne and its boron nitride analog. Int. J. Hydrogen Energy 39 (24), 12757–12764. doi:10.1016/j.ijhydene.2014.06.107
Ma, L. juan, Rong, Y., Hao, W., Jia, J., and Wu, H. shun (2020). Investigation of hydrogen storage on Sc/Ti- decorated novel B 24 N 24. Int. J. Hydrogen Energy 45 (58), 33740–33750. doi:10.1016/j.ijhydene.2020.09.019
Ma, R., Bando, Y., Sato, T., Golberg, D., Zhu, H., Ma, R., et al. Synthesis of boron nitride nanofibers and measurement of their hydrogen uptake capacity Synthesis of boron nitride nanofibers and measurement of their hydrogen uptake capacity. 2002b;5225.
Ma, R., Bando, Y., Zhu, H., Sato, T., Xu, C., and Wu, D. (2002a). Hydrogen uptake in boron nitride nanotubes at room temperature. J. Am. Chem. Soc. 124 (26), 7672–7673. doi:10.1021/ja026030e
Moussa, G., Demirci, U. B., Malo, S., Bernard, S., and Miele, P. (2014a). Hollow core mesoporous shell boron nitride nanopolyhedron-confined ammonia borane: a pure B–N–H composite for chemical hydrogen storage. J. Mater Chem. A 2, 7717–7722. doi:10.1039/c3ta13611f
Moussa, G., Salameh, C., Bruma, A., Malo, S., Demirci, U. B., Bernard, S., et al. (2014b). Nanostructured boron nitride: from molecular design to hydrogen storage application. Inorganics 2, 396–409. doi:10.3390/inorganics2030396
Okan, B. S., Kocabas, O., Nalbant, A., Baysal, M., Letofsky-papst, I., and Yu, Y. (2012). E ff ect of reaction temperature and catalyst type on the formation of boron nitride nanotubes by chemical vapor deposition and measurement of their hydrogen storage capacity. Ind. Eng. Chem. Res. 51, 11341–11347. doi:10.1021/ie301605z
Panigrahi, P., Desai, M., Krishna, M., Bae, H., Lee, H., Ahuja, R., et al. (2021). Selective decoration of nitrogenated holey graphene (C2N) with titanium clusters for enhanced hydrogen storage application. Int. J. Hydrogen Energy 46 (10), 7371–7380. doi:10.1016/j.ijhydene.2020.11.222
Qiu, N. X., Zhang, C. H., and Xue, Y. (2014). Tuning hydrogen storage in lithium-functionalized BC2N sheets by doping with boron and carbon. ChemPhysChem 15 (14), 3015–3025. doi:10.1002/cphc.201402246
Rai, D. P., Chettri, B., Patra, P. K., and Sattar, S. (2021). Hydrogen storage in bilayer hexagonal boron nitride: a first- principles study. ACS Omega 6, 30362–30370. doi:10.1021/acsomega.1c03443
Ren, J., Zhang, N., Zhang, H., and Peng, X. (2015). First-principles study of hydrogen storage on Pt (Pd)-doped boron nitride sheet. Struct. Chem. 26 (3), 731–738. doi:10.1007/s11224-014-0531-2
Rosi, N. L., Eckert, J., Eddaoudi, M., Vodak, D. T., Kim, J., O’Keeffe, M., et al. (2003). Hydrogen storage in microporous metal-organic frameworks. Science 300 (5622), 1127–1129. doi:10.1126/science.1083440
Roy, L., Bhunya, S., and Paul, A. (2014). A metal-free strategy to release chemisorbed H2 from hydrogenated boron nitride nanotubes. Angew. Chem. - Int. Ed. 53 (46), 12430–12435. doi:10.1002/anie.201403610
Salazar-Aparicio, R. V., Vazquez-Nava, R. A., Arzate, N., and Mendoza, B. S. (2014). Molecular hydrogen physisorption on boron-nitride nanotubes probed by second harmonic generation. Phys. Rev. B 90 (155403), 1–5. doi:10.1103/PhysRevB.90.155403
Schlapbach, L., and Züttel, A. (2001). Hydrogen-storage materials for mobile applications. Nature 414 (6861), 353–358. doi:10.1038/35104634
Sengupta, D., Melix, P., Bose, S., Duncan, J., Wang, X., Mian, M. R., et al. (2023). Air-stable Cu(I) metal–organic framework for hydrogen storage. J. Am. Chem. Soc. 145 (37), 20492–20502. doi:10.1021/jacs.3c06393
Shahsavari, R., and Zhao, S. (2018). Merger of energetic affinity and optimal geometry provides new class of boron nitride based sorbents with unprecedented hydrogen storage capacity. Small 14 (15), 17028633–e1702910. doi:10.1002/smll.201702863
Shangguan, W., Zhao, H., Dai, J. qing, Cai, J., and Yan, C. (2021). First-principles study of hydrogen storage of Sc-modi fi ed semiconductor covalent organic framework - 1. ACS Omega 6, 21985–21993. doi:10.1021/acsomega.1c02452
Tang, C., Bando, Y., Ding, X., Qi, S., and Golberg, D. (2002). Catalyzed collapse and enhanced hydrogen storage of BN nanotubes. J. Am. Chem. Soc. 124 (49), 14550–14551. doi:10.1021/ja028051e
Tokarev, A., Kjeang, E., Cannon, M., and Bessarabov, D. (2016). Theoretical limit of reversible hydrogen storage capacity for pristine and oxygen-doped boron nitride. Int. J. Hydrogen Energy 41 (38), 16984–16991. doi:10.1016/j.ijhydene.2016.07.010
Tozzini, V., and Pellegrini, V. (2013). Prospects for hydrogen storage in graphene. Phys. Chem. Chem. Phys. 15, 80–89. doi:10.1039/c2cp42538f
U.S. Department of Energy (2022). DOE technical targets for onboard hydrogen storage for light-duty vehicles. Available at: https://www.energy.gov/eere/fuelcells/doe-technical-targets-onboard-hydrogen-storage-light-duty-vehicles.
Varunaa, R., and Ravindran, P. (2019). Potential hydrogen storage materials from metal decorated 2D-C2N: an: ab initio study. Phys. Chem. Chem. Phys. 21 (45), 25311–25322. doi:10.1039/c9cp05105h
Venkataramanan, N. S., Khazaei, M., Sahara, R., Mizuseki, H., and Kawazoe, Y. (2009). First-principles study of hydrogen storage over Ni and Rh doped BN sheets. Chem. Phys. 359 (1–3), 173–178. doi:10.1016/j.chemphys.2009.04.001
Wang, J., Chen, Y., Yuan, L., Zhang, M., and Zhang, C. (2019). Scandium decoration of boron doped porous graphene for high-capacity hydrogen storage. Molecules 24 (13), 2382. doi:10.3390/molecules24132382
Wang, J., Ma, F., and Sun, M. (2017). Graphene, hexagonal boron nitride, and their heterostructures: properties and applications. RSC Adv. 7, 16801–16822. doi:10.1039/C7RA00260B
Wang, P., Orimo, S., Matsushima, T., Fujii, H., and Majer, G. (2002). Hydrogen in mechanically prepared nanostructured h-BN: a critical comparison with that in nanostructured graphite. Appl. Phys. Lett. 80 (2), 318–320. doi:10.1063/1.1432447
Wang, T., Wang, C., Huo, Y., and Liu, M. (2022). Hydrogen storage in C-doped h-BN decorated with titanium atom: a computational study. Chem. Phys. Lett. 803 (April), 139853. doi:10.1016/j.cplett.2022.139853
Wang, Y., Wang, F., Xu, B., Zhang, J., Sun, Q., Wang, Y., et al. (2013). Theoretical prediction of hydrogen storage on Li-decorated boron nitride atomic chains. J. Appl. Phys. 113 (May), 064309. doi:10.1063/1.4790868
Weng, Q., Wang, X., Bando, Y., and Golberg, D. (2014). One-step template-free synthesis of highly porous boron nitride microsponges for hydrogen storage. Adv. Energy Mat. 4 (7), 1–8. doi:10.1002/aenm.201301525
Weng, Q., Wang, X., Zhi, C., Bando, Y., and Golberg, D. (2013). Boron nitride porous microbelts for hydrogen storage. ACS Nano. 7 (2), 1558–1565. doi:10.1021/nn305320v
Wu, X., Yang, J. L., and Zeng, X. C. (2006). Adsorption of hydrogen molecules on the platinum-doped boron nitride nanotubes. J. Chem. Phys. 125 (4), 44704. doi:10.1063/1.2210933
Zhang, H., Tong, C. J., Zhang, Y., Zhang, Y. N., and Liu, L. M. (2015). Porous BN for hydrogen generation and storage. J. Mater Chem. A 3 (18), 9632–9637. doi:10.1039/C5TA01052G
Zhang, N., Shi, Y., Li, J., Zhang, Y., Guo, J., Fu, Z., et al. (2023). Potential applications of OLi3-decorated h-BN monosheet for high hydrogen storage. Appl. Phys. Lett. 123 (8), 83902. doi:10.1063/5.0159978
Zhao, H., Guan, Y., Guo, H., Du, R., and Yan, C. (2020). Hydrogen storage capacity on Li-decorated covalent organic framework-1: a first-principles study. Mater Res. Express 7, 035506. doi:10.1088/2053-1591/ab7fe0
Keywords: hydrogen storage, sustainability, boron nitride, structure design, functionalization, theoretical modeling, experimental investigation
Citation: Kovalskii AM, Manakhov AM, Afanasev PA, Popov ZI, Matveev AT and Al-Qasim AS (2024) Hydrogen storage ability of hexagonal boron nitride. Front. Mater. 11:1375977. doi: 10.3389/fmats.2024.1375977
Received: 24 January 2024; Accepted: 28 May 2024;
Published: 13 June 2024.
Edited by:
Jingbo Louise Liu, Texas A&M University Kingsville, United StatesReviewed by:
Ernest Chiedoziam Agwamba, Clifford University, NigeriaQi Li, Amazon, United States
Anoop Uchagawkar, University of Kansas, United States
Copyright © 2024 Kovalskii, Manakhov, Afanasev, Popov, Matveev and Al-Qasim. This is an open-access article distributed under the terms of the Creative Commons Attribution License (CC BY). The use, distribution or reproduction in other forums is permitted, provided the original author(s) and the copyright owner(s) are credited and that the original publication in this journal is cited, in accordance with accepted academic practice. No use, distribution or reproduction is permitted which does not comply with these terms.
*Correspondence: Andrey M. Kovalskii, YW5kcmV5LmtvdmFsc2tpaUBhcmFtY29pbm5vdmF0aW9ucy5jb20=