- 1Department of Materials Science and Engineering, University of Tennessee, Knoxville, TN, United States
- 2National Institute of Physics, College of Science, University of the Philippines Diliman, Quezon, Philippines
- 3Department of Physics and Institute of Quantum Convergence Technology, Kangwon National University, Chuncheon, Republic of Korea
- 4Department of Chemistry, University of Tennessee, Knoxville, TN, United States
- 5Materials Science and Technology Division, Oak Ridge National Laboratory, Oak Ridge, TN, United States
van der Waals antiferromagnets with chemical formula MPX3 (M = V, Mn, Fe, Co, Ni, Cd; X = S, Se) are superb platforms for exploring the fundamental properties of complex chalcogenides, revealing their structure-property relations and unraveling the physics of confinement. Pressure is extremely effective as an external stimulus, able to tune properties and drive new states of matter. In this review, we summarize experimental and theoretical progress to date with special emphasis on the structural, magnetic, and optical properties of the MPX3 family of materials. Under compression, these compounds host inter-layer sliding and insulator-to-metal transitions accompanied by dramatic volume reduction and spin state collapse, piezochromism, possible polar metal and orbital Mott phases, as well as superconductivity. Some responses are already providing the basis for spintronic, magneto-optic, and thermoelectric devices. We propose that strain may drive similar functionality in these materials.
1 Introduction
Transition metal phosphorous trichalcogenides, MPX3, where M represents a transition metal ion and X is either S or Se, are a family of semiconducting van der Waals magnets that were originally discovered in 1894 (Friedel, 1894) but were not studied intensively until the 1970s (Klingen et al., 1973). Originally, interest in these materials was sparked by their low dimensional magnetic properties (Le Flem et al., 1982) and ability to form intercalation compounds (Jophnson and Jacobson, 1982), although more recently these materials have attracted attention as single-layer magnets (Burch et al., 2018) and as highly tunable systems that undergo bandwidth-controlled Mott transitions under pressure (Kim et al., 2019).
MPX3 materials typically crystallize in two space groups, C2/m (No. 12) for the sulfur analogs and R
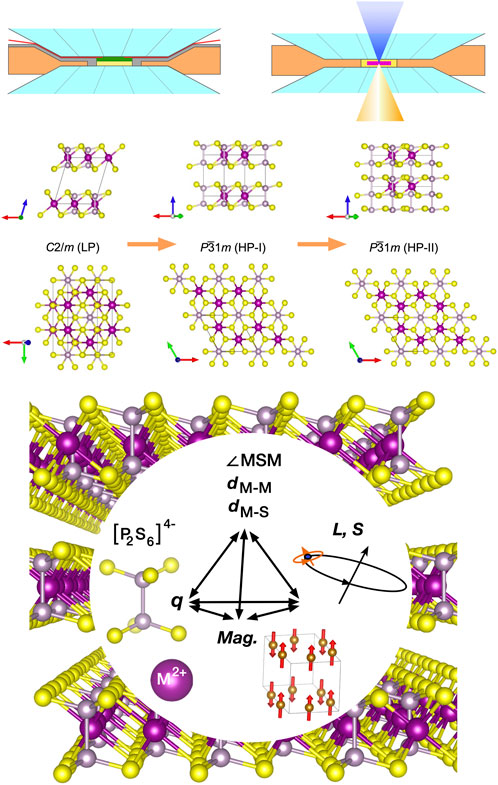
Figure 1. (Top) Schematic diagrams of diamond anvil cells (DAC) applying hydrostatic (left) and non-hydrostatic (right) pressure to MPX3 compounds. (Middle) An example of pressure-induced structural transition involving layer sliding and phosphorous chain formation in MPX3. (Bottom) An Illustration of structural (metal-metal distance dM−M, metal-sulfur distance dM−S, and bond angle ∠MSM), orbital (L), spin (S), charge (q)), and magnetic (Mag.) degrees of freedom, which together interact to give rise to novel pressure-induced physical properties of MPX3.
In general, MPS3 materials with M = V, Mn, Fe, Co, and Ni are reported to be antiferromagnetic Mott insulators with variable semiconducting energy gaps and magnetic structures. CdPS3, in contrast, has a filled d shell and is semiconducting and nonmagnetic. The Mottness and magnetism of MPS3 compounds originates from their partially-filled d-orbital shells; this, in addition to the presence of a van der Waals gap, suggests that the MPS3 series is an excellent platform for the study of two-dimensional correlated electron phenomena and magnetism (Figure 1).
Tuning the physical properties of these compounds is a highly contemporary research subject, allowing for the exploration of new states of matter and functionalities. Toward this end, researchers are using chemical substitution, doping and intercalation, as well as various external perturbations such as electric field, light, strong magnetic fields, and strain (Wang et al., 2018a; Pei et al., 2022). For instance, chemical substitution on the M and X sites provides the control of d-orbital filling and bandwidth, respectively, which are both important parameters to control Mottness, magnetism, and spin-orbital-lattice coupling (Lu et al., 2022; Peng et al., 2023). Even better, many MPS3 compounds have both X = S and Se limits as stable systems, which allows continuous tuning of lattice parameters and bandwidth via synthesizing MPSxSe3−x samples (Basnet et al., 2022b). Even differences in X-site chemistry sometimes gives rise to interesting contrasts; for example, the presence and absence of superconductivity in pressurized FePSe3 and FePS3, respectively. At the same time, doping and intercalation control carrier concentration and impart new properties (Basnet et al., 2022a; Tezze et al., 2022).
Among these external stimuli, pressure is a highly effective tool for tuning the physical properties of MPX3 materials. The interlayer van der Waals (vdW) interaction is weak; thus, the interlayer spacing can be effectively modulated by pressure. As the layers are forced closer together, layers slide with respect to each other, changing their relative orientation. Furthermore, pressure modifies the c/a ratio, reflecting the more compressible interlayer distance than the ab plane. Bond lengths and angles within the chalcogenide layers are also modified under compression (Figure 1). With the collapse of the van der Waals gap under pressure, interlayer orbital overlap increases, and the materials become more three-dimensional with enhanced bandwidth. With varying d-orbital occupancies and the resulting different orbital degrees of freedom (i.e., t2g vs. eg), pressure can induce drastically different responses of lattice structure, as well as electronic and magnetic properties, depending on the chemistry of the metal center and chalcogen anion.
In addition to bandwidth and dimensionality tuning, the sliding of adjacent layers and change of stacking order can create contrasting responses in electronic and magnetic properties, as recently suggested in 1T-TaS2 with the “star of David” charge density wave formation. In MPX3, this effect appears prominently since even hydrostatic pressure can create shear strain in the monoclinic structure of many of them (Butler et al., 2020). As we will see below, the presence of this layer-sliding in the MPS3 series under pressure (in V0.9PS3, MnPS3, FePS3, CoPS3, and NiPS3) creates complicated yet rich structural, electronic, and magnetic behaviors. For example, it has been reported that the layer sliding in MnPS3 expedites the pressure-induced Mott transition (Harms et al., 2020). At this point it is uncertain why systems show different layer-sliding responses under compression, still it is clear that pressure is a valuable tool in unlocking tremendously rich physical characteristics hidden within the MPX3 series.
Over the past few years the evolution of the structural, magnetic, and electronic properties of MPX3 compounds under compression have been extensively studied. Given the rapid progress in this field, the time is ripe for a short review. In the sections below, we first discuss the M = Mn, Fe, and Ni, which have been the topics of the most number of studies. We then discuss V0.9PS3 and CoPS3, which have been much less studied. Lastly, we cover the non-magnetic compounds, CdPS3 and CdPSe3, and the structure-property relations that can be unraveled in these systems.
In addressing ongoing research topics in each system, discussions involving the following themes occur in most of the compounds: strong electron correlations from the partially-filled d-orbital shells, pressure-induced Mott insulator-to-metal transitions, the role of p-d hybridization in ground-state and optical properties via the comparison between MPS3 and MPSe3 systems, and the role of layer-sliding and the changes in of P-P bonding at the Mott metallization transition. These issues are discussed at appropriate points in the manuscript.
Pressure is an effective tool for tuning the properties of complex chalcogenides, but the following considerations should be kept in mind when interpreting experimental data. Below 6 or 7 GPa, pressure in the diamond anvil cell is reliably hydrostatic, although tests should be performed to confirm this expectation. Above 6 or 7 GPa, it’s very challenging to assure perfectly hydrostatic conditions. Even in a well-tested and characterized system, there may be deviations on the order of 1% or more—especially at low temperatures. Non-hydrostatic conditions can impact the properties - for instance, broadening a spectroscopic response. These signatures are most easily monitored by ruby fluorescence and are discussed in a number of references including (Musfeldt et al., 2023).
2 Properties of MPX3 materials under pressure
2.1 MnPS3 and MnPSe3
MnPS3 is the prototype in this family of materials and is therefore extensively investigated. This system crystallizes in a monoclinic C2/m structure whereas MnPSe3 displays a trigonal R

Table 1. Inter-layer thicknesses distances and van der Waals (vdW) gaps of MPS3 and MPSe3. Data are from (Ouvrard et al., 1985b; Ouvrard et al., 1985a; Wiedenmann et al., 1981).
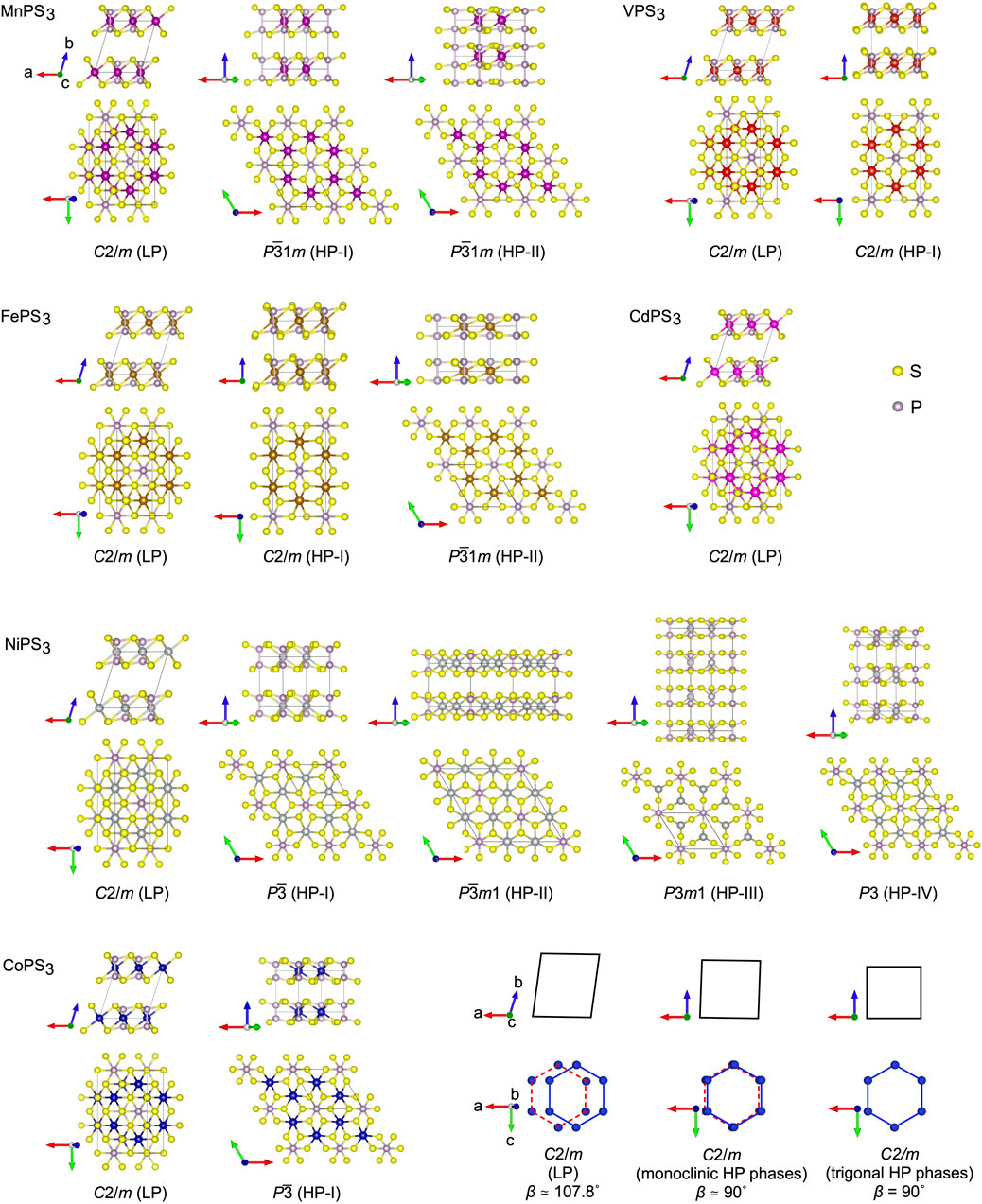
Figure 2. Crystal structures of MPS3 (M = V, Mn, Fe, Ni, Co, Cd) family of materials in the low-pressure (LP) and high-pressure (HP) phases visualized using VESTA3 (Momma and Izumi, 2011) based upon reported experimental and theoretical results (Ouvrard et al., 1985a; Haines et al., 2018; Coak et al., 2019; Harms et al., 2020; Harms et al., 2022a; Matsuoka et al., 2023). The bottom right shows layer sliding in C2/m symmetry with decreasing monoclinic angle β under pressure. When β = 90°, the honeycomb pattern of metal ions overlaps between adjacent layers, and the system transforms to a trigonal high pressure phase.
Early neutron diffraction studies of MnPS3 uncovered collinear Néel-type AFM order at TN = 78 K (Le Flem et al., 1982; Kurosawa et al., 1983b; Joy and Vasudevan, 1992; Wildes et al., 1994), although there were discrepancies as to the direction of the magnetic propagation vector. A recent study revisited this problem using single crystal neutron diffraction and polarimetry (Ressouche et al., 2010). This work revealed a propagation vector k = [000] as well as magnetoelectric coupling and ferrotoroidicity (Ressouche et al., 2010). By comparison, TN = 74 K for MnPSe3 (Wiedenmann et al., 1981; Le Flem et al., 1982). A similar reduction of the transition temperature is observed for FePX3 when the X is modified from sulfur to selenium. Neutron scattering of MnPSe3 reveals an identical propagation vector (k = [000]) (Wiedenmann et al., 1981; Bhutani et al., 2020). Photoemission and x-ray absorption spectroscopy confirms a high spin Mn2+ configuration in MnPSe3 (Fujii et al., 2022). Each Mn center is antiferromagnetically coupled to its three nearest neighbors (Kurosawa et al., 1983a). One surprising finding is the strong magnetic interaction between third nearest neighbors. The spin wave excitations have also been studied by Raman and neutron scattering in these materials. Major findings include: hybridization of the two-magnon excitation with phonons (which impacts the spin wave decoherence pathway) and absence of nonreciprocity in zone boundary magnons (which provides experimental limits that can be tested against theoretical predictions) (Sun et al., 2019; Vaclavkova et al., 2020; Mai et al., 2021; Wildes et al., 2021).
Several different but complementary efforts were required to unravel the sequence of events that takes place under pressure. In the first experimental work, Wang et al. uncovered a simultaneous spin-state crossover and metal-insulator transition in both MnPS3 and MnPSe3 (Figure 3) as well as zigzag chain formation of the Mn atoms in the metallic phase (Wang et al., 2016). The critical pressures (Pc’s) for these processes were reported to be 30 and 25 GPa for MnPS3 and MnPSe3, respectively. First-principles electronic structure calculations using DFT + U and DFT + DMFT techniques reproduced the insulator-to-metal transition with simultaneous spin-state transition but with a Mn-Mn dimerization at 64 GPa (Kim et al., 2019), although it was hinted that some structural components might be missing. This early experimental and theoretical work did not consider layer sliding, which turns out to modify the picture in important ways.
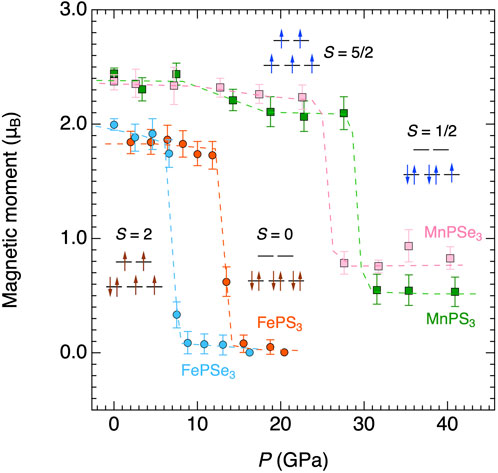
Figure 3. Magnetic moment and spin state as a function of pressure for MnPS3, MnPSe3, FePS3, and FePSe3 relative to properties at ambient conditions. Data taken from (Wang et al., 2016; Wang Y. et al., 2018).
In a significant conceptual advance, Coak et al. discovered an intermediate pressure transition in the series of MPS3 (M = V0.9, Mn, Fe, Ni) materials (Coak et al., 2020). This transition involves coherent layer sliding to create an alignment of P–P dimers along the layer-normal direction. This insight enabled a number of subsequent advancements. For instance, infrared, Raman scattering, and optical measurements as a function of pressure combined with first-principles calculations reveal that MnPS3 undergoes a C2/m (LP) → P
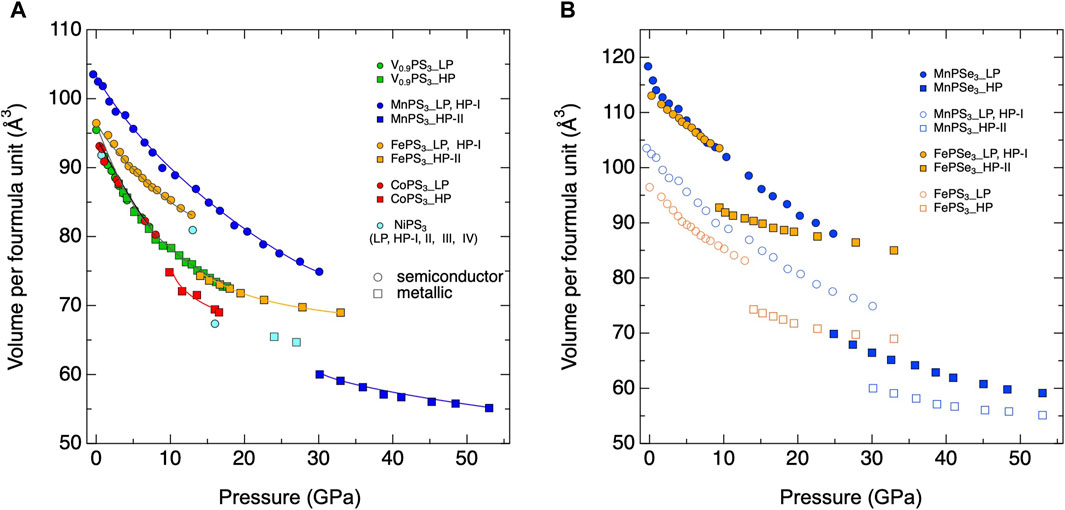
Figure 4. Volume per formula unit as a function of pressure. (A) MPS3 (M = V, Mn, Fe, Co, Ni). (B) MnPS3 and FePS3 with MnPSe3 and FePSe3 for comparison. The circles denote the low (or ambient) pressure phase, which is semiconducting, including the HP-I phases for MnPS3 and FePS3. The squares indicate high pressure metallic phases. The solid lines guide the eye.
We note in passing that theory tends to over-estimate the band gap and pressure of the insulator-to-metal transition in these materials (Grasso et al., 1991; Harms et al., 2020; Yan et al., 2023). Gap estimation is a well-known challenge for first-principles density functional theory-based approaches, and weak interactions with shallow potentials are notoriously difficult to calculate with accuracy. Nevertheless, these techniques nicely reveal the semiconducting behavior of the aligned phosphorous dimer phase and the metallic character of the aligned phosphorous chain phase (Figure 5A).
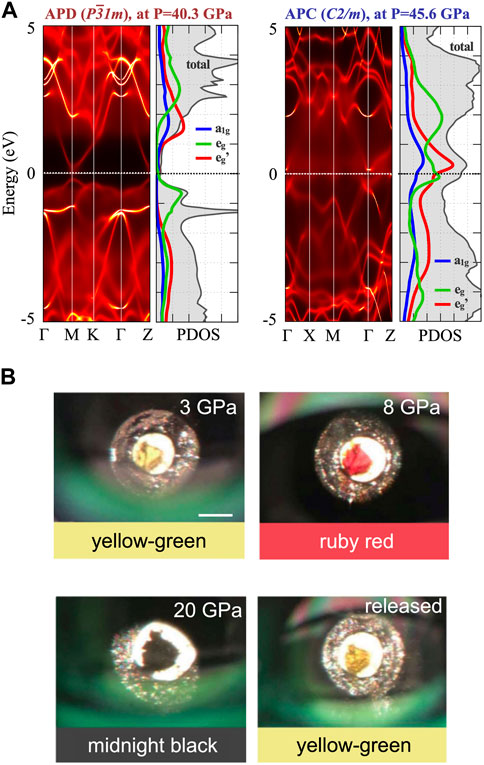
Figure 5. MnPS3 under pressure puncture. (A) Spectral functions from DFT + DMFT calculations showing the k-resolved and orbital-projected spectral functions calculated with the aligned phosporous dimer (APD) and aligned phosphorous chain (APC), respectively (Harms et al., 2020). (B) Photographs of piezochromic MnPS3 inside the diamond anvil cell at several characteristic pressures and also after release at room temperature. The scale bar (left panel) is 200 μm. (Harms et al., 2020).
From the optical properties point of view, MnPS3 is a semiconductor with a 2.7 eV band gap. It hosts spin-charge coupling across the magnetic ordering transition and a set of crystal field excitations that have been widely investigated (Boerio-Goates et al., 1981; Grasso et al., 1991; Harms et al., 2020; Yan et al., 2023; Park et al., 2024). There is strong p-d hybridization which activates the d-to-d on-site excitations of the Mn2+ centers. There is also a broad near infrared emission band assigned as 4T1 →6A1 with a half life of approximately 50 μs at 77 K (Boerio-Goates et al., 1981). The ratio of the crystal field splitting to the Racah parameter (10Dq/B) is unusually large and may explain the strongly red-shifted light emission in this system. That the band gap of MnPS3 decreases systematically under pressure suggests that this system hosts piezochromism (Harms et al., 2020). It turns out that MnPS3 is green at ambient conditions, and as shown in Figure 5B, it is yellowish-green at 3 GPa, bright red at 8 GPa, and black by 20 GPa. The latter occurs because the band gap shifts out of the visible wavelength range, moving systematically toward closure at a rate of approximately −50 meV/GPa. This effect is quenched by the appearance of the insulator–metal transition. This high level of linear responsivity under pressure is unusual, and piezochromism of this type has applications in pressure sensing, actuation, as well as artificial skin. At this time, it is not known whether strain can drive similar effects in the bulk material, although in few- and single-sheet MnPS3 there is a C2/m to P
Thermal properties of these materials are also of interest from a fundamental point of view and for heat management purposes in a device. Kargar et al. find in- and out-of-plane thermal conductivities of 6.3 ± 1.7 and 1.1 ± 0.2 W/m⋅K, respectively (Kargar et al., 2019). These values are higher than what is found in the Fe analog.
2.2 FePS3 and FePSe3
FePS3 and FePSe3 are layered antiferromagnets with TN around 120 K (Le Flem et al., 1982; Joy and Vasudevan, 1992). FePS3 has gained significant interest recently for its Ising-like antiferromagnetic ordering in the single-layer limit (Lee et al., 2016). The d6 configuration of the Fe2+ charge status shows a high-spin configuration S = 2 with the partially-filled t2g complex, implying anisotropic nature of magnetism as revealed by experimental and theoretical observations (Nauman et al., 2021; Zhang et al., 2021). In the presence of external pressure, like Mn-based siblings, spin-state transition and the resulting complex electronic and structural responses are expected in FePS3 and isostructural FePSe3. Accordingly, the responses of magnetic, electronic, and structural properties of FePS3 and FePSe3 have been actively investigated.
Recent x-ray, neutron scattering, and optical spectroscopy studies on the effect of hydrostatic pressure on FePS3 reveal a sequence of structural phase transitions, (Haines et al., 2018; Coak et al., 2021; Harms et al., 2022b) analogous to MnPS3 and V1−xPS3 (Coak et al., 2019; Harms et al., 2020); a gradual layer-sliding crossover from the monoclinic LP (C2/m space group) to the pseudo-hexagonal intermediate phase (HP-I, C2/m) around 2–5 GPa, and the following C2/m → P
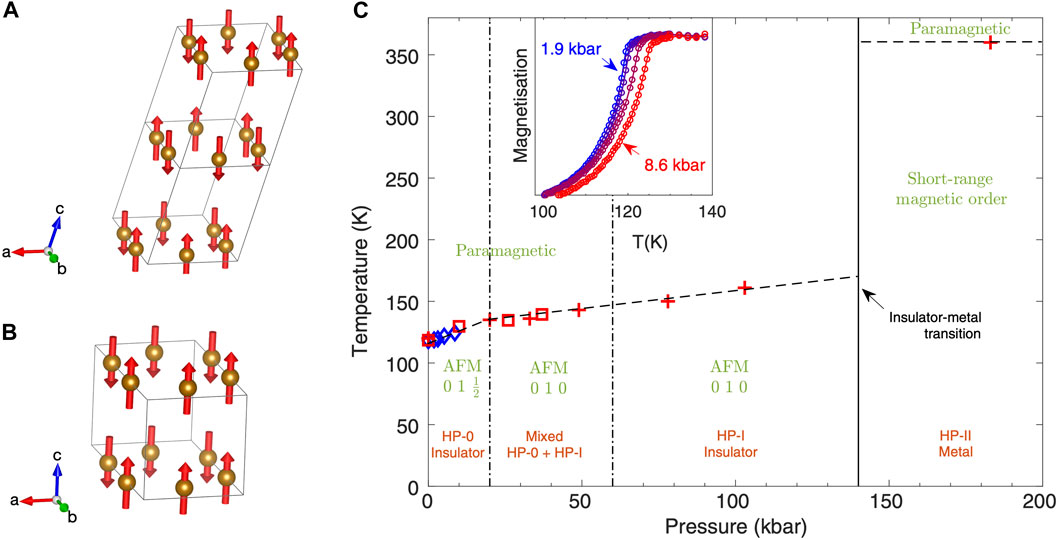
Figure 6. Crystal and magnetic structures of the low-pressure (HP-0) and the first high-pressure (HP-I) phases of FePS3. (A) The magnetic cell of FePS3 with the k = [0 1 1/2] propagation vector - overall antiferromagnentic order, consisting of a zig-zag chain antiferromagnetically coupled to their neighbors along a-axis and between the planes (Lançon et al., 2016). (B) The magnetic unit cell with k = [0 1 0] propagation vector, where the planes are ferromagnetically aligned (Coak et al., 2021). (C) Pressure-temperature phase diagram of FePS3 from magnetization measurements and neutron scattering experiments. Blue circles, red crosses (and squares) are from magnetization measurements and integration of neutron spectra, respectively. Dashed lines are fits to the data points, and vertical lines mark structural transitions. Inset shows normalized magnetization versus temperature with increasing pressure. The plot is from (Coak et al., 2021).
The second transition (HP-I → HP-II) accompanies the breaking of the P-P dimer structure and the formation of interlayer P-chain perpendicular to the Fe layers, in addition to a sudden collapse of the interlayer distance and volume, (Haines et al., 2018) similar to the case of MnPS3. Harms et al. (2020) note that these observations are consistent with separate first-principles electronic structure calculation results, (Zheng et al., 2019; Deng et al., 2023) where the origin of the coexistence of the LP and HP-I phases around 2–5 GPa is suggested to be the result of the shear-induced energy barrier between the LP and HP-I phases (see Figure 7A), (Deng et al., 2023).
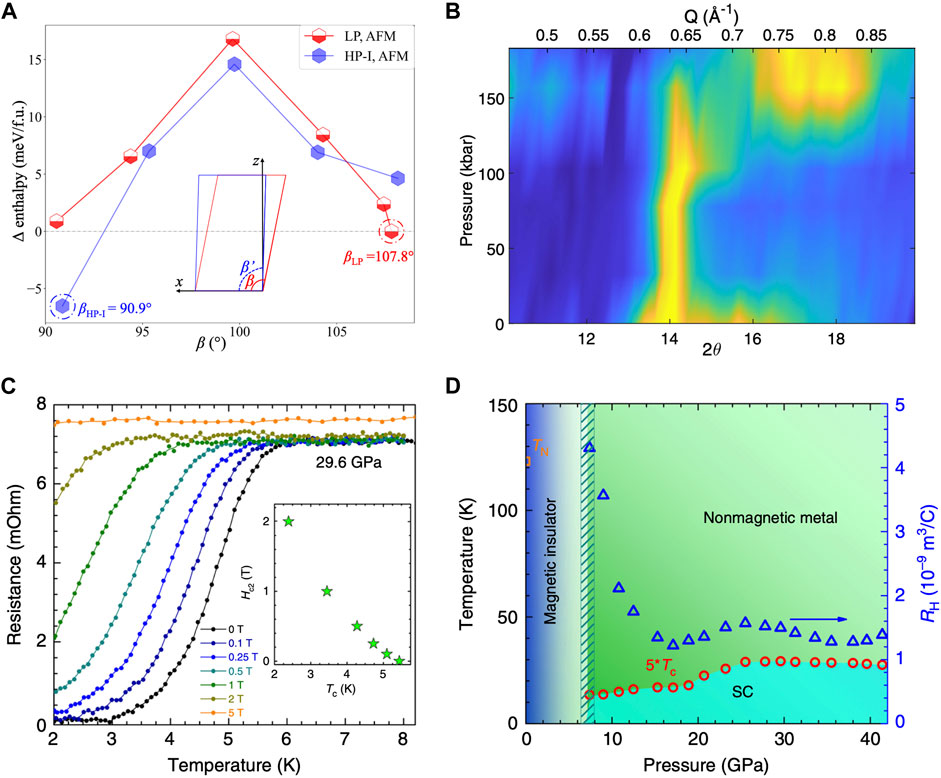
Figure 7. High-pressure electronic states of FePS3 and superconducting FePSe3 (A) Enthalpy difference from DFT calculations as a function of the monoclinic β angle in the C2/m structure for the ambient (LP) and HP-I AFM phases of FePS3 at 1.0 GPa. Panel from Deng et al. (2023). (B) Heat map of magnetic peaks at 80 K in FePS3, showing the presence of magnetic signals in the HP-II (P
From both experimental and theoretical studies, the Mott-insulating antiferromagnetic phase is reported to remain robust in the LP and HP-I phases, reflecting the quasi-two-dimensional nature (Haines et al., 2018; Zheng et al., 2019). With the formation of the interlayer P-chain in the HP-II phase this two-dimensional character breaks down, and so does the insulating behavior. The sudden collapse of the interlayer distance and cell volume at the HP-I → II transition implies a spin-state transition (see Figure 4). Indeed, an early study on pressurized phases of FePS3 and FePSe3 reported collapses of magnetic moments and transitions to low-spin phases around 13 and 8 GPa, respectively, Wang et al. (2018b) and additionally, the DFT study of Zheng et al. (2019) also reports the spin-state transition from the high-spin S = 2 to the low-spin S = 0 configuration of the Fe ions at the HP-I → II transition. Interestingly, on the other hand, a high-pressure neutron diffraction data by Coak et al. (2021) suggests the presence of short-range magnetic order in the HP-II phase, persisting even up to room temperature (see Figure 6C, 7B). From a detailed electronic structure calculation study, Deng et al. (2023) argues that this puzzling behavior may be explained by the potential coexistence of two metastable metallic states; the high-spin one with the intralayer P2 dimer structure remains intact comparing the short-range order (2D-like), while the P2 dimer breaks to form the out-of-plane P chain structure (3D-like) in the other low-spin state. A separate dynamical mean-field theory study on FePS3 also suggests the coexistence of the two structural phases, each one hosting the normal Fermi liquid-like character (with the interlayer P-chain) and the incoherent “Hund’s metal” character (with the intralayer P-dimer) in the high-pressure regime, where the Hund’s metal phase keeps the local moments intact and induces bad-metallic behavior due to magnetic fluctuations (Kim et al., 2022).
It is interesting to note that, in Kim et al., (2022), the Hund’s metal phase with the orbital-selective correlation effects occurs only with a non-hydrostatic pressure, especially when the pressure perpendicular to the Fe layers are stronger than the in-plane counterparts. This originates from the direction-selective orbital effect under pressure, where the t2g orbitals of Fe ions becomes more susceptible to the out-of-plane stress compared to eg. This implies a subtle role of anisotropy and inhomogeneity in pressure, which may often occur in high-pressure experimental situations depending on the choices of pressure-transmitting medium (Matsuoka et al., 2021).
Lastly we comment on FePSe3. This system shares a crystal structure and magnetic ground state with FePS3, (Wiedenmann et al., 1981; Le Flem et al., 1982) and expected from theoretical calculations to show many common properties, such as layer-sliding transition to HP-I, volume collapse at the HP-I → II transition accompanying the simultaneous spin-state and insulator-metal transitions (Zheng et al., 2019). Experimentally the second transition is observed to occur at a lower pressure of around 8 GPa than that of FePS3, which can be attributed to the larger hybridization effects from Se ions (Wang et al., 2018b). Unlike FePS3, superconductivity of unknown origin has been reported in the HP-II metallic phase of FePSe3 below 2.5K (see Figures 7C, D), (Wang et al., 2018b). Structural refinement in the same study suggests the formation of the zigzag chains within the Fe honeycomb layer in the HP-II phase of FePSe3, hinting the presence of orbital physics from high-spin configuration at Fe ions and potential unconventional superconductivity.
2.3 NiPS3 and NiPSe3
NiPS3 and the Se analog provide another exciting platform for exploring new states of matter under external stimuli and developing different types of structure-property relationships. The magnetic ordering temperatures are TN = 155 and 206 K, respectively (Le Flem et al., 1982; Joy and Vasudevan, 1992; Chandrasekharan and Vasudevan, 1994), so we see that in this case, heavier anions act to raise the Neél temperature. In the low temperature phase of NiPS3, the magnetic propagation vector k = [010]. Analysis of the integrated intensity of the (010) magnetic Bragg peak as a function of temperature reveals a critical exponent for the magnetization 2β = 0.30 away from the transition temperature, consistent with a two-dimensional universality class, and 0.30 near TN, suggesting the importance of three-dimensional interactions in this regime (Wildes et al., 2015). There are a number of low energy excitations in this system from which competing exchange interactions can be extracted (Mehlawat et al., 2022; Wildes et al., 2023), and active pumping of orbital resonances can also manipulate magnetic order in this quasi-two-dimensional van der Waals antiferromagnet (Afanasiev et al., 2021). Solid solutions in the form of Ni1−xMnxPS3 (0 ≤ x ≤ 1) can also tune the spin flop transition from approximately 7 to 2 T due to modulation of the magnetic anisotropy (Basnet et al., 2021). Intercalation is also very effective in this regard. It can even drive the ground state ferrimagnetic (Basnet et al., 2022a; Tezze et al., 2022). The magnetic properties of NiPS3 are clearly very sensitive to chemical pressure, suggesting that there is more to learn under physical pressure. The latter is obviously a much cleaner technique as well. Light is also an important external stimuli in these materials. NiPS3, for instance, sports laser-induced demagnetization with responsivity (lifetime) on the order of 10 ps (Kuntu et al., 2024). Photo-induced quenching of magnetic order is likely to be very sensitive to pressure.
Early high pressure x-ray diffraction work combined with first-principles calculations suggested strong similarities between NiPS3 and the M = Mn and V analogs, with LP-to-HP-I and the HP-I-to-HP-II transitions near 15 and 27 GPa with an insulator-to-metal transition near 20 GPa (Ma et al., 2021). More recent efforts combining transport and x-ray diffraction reveal a series of different crystal structures under pressure, time-dependent and somewhat sluggish character to several of the transitions, and an insulator-to-metal transition near 30 GPa (Matsuoka et al., 2021). Systematic studies of hydrostatic pressure vs. strain demonstrates that the critical pressure of the insulator-to metal transition (as identified by transport measurements) is reduced from 30 to 12.5 GPa under uniaxial strain (Cui et al., 2021) - an observation that again emphasizes the need for hydrostatic pressure conditions. This confusing and somewhat contradictory picture was resolved by bringing together synchrotron-based infrared absorption, Raman scattering, x-ray diffraction, and first-principles calculations under pressure to show that in fact NiPS3 is quite different from the other MPS3 materials (Harms et al., 2022a) with a series of five different critical pressures. The set of symmetry progressions in this material is complex (involving C2/m → P
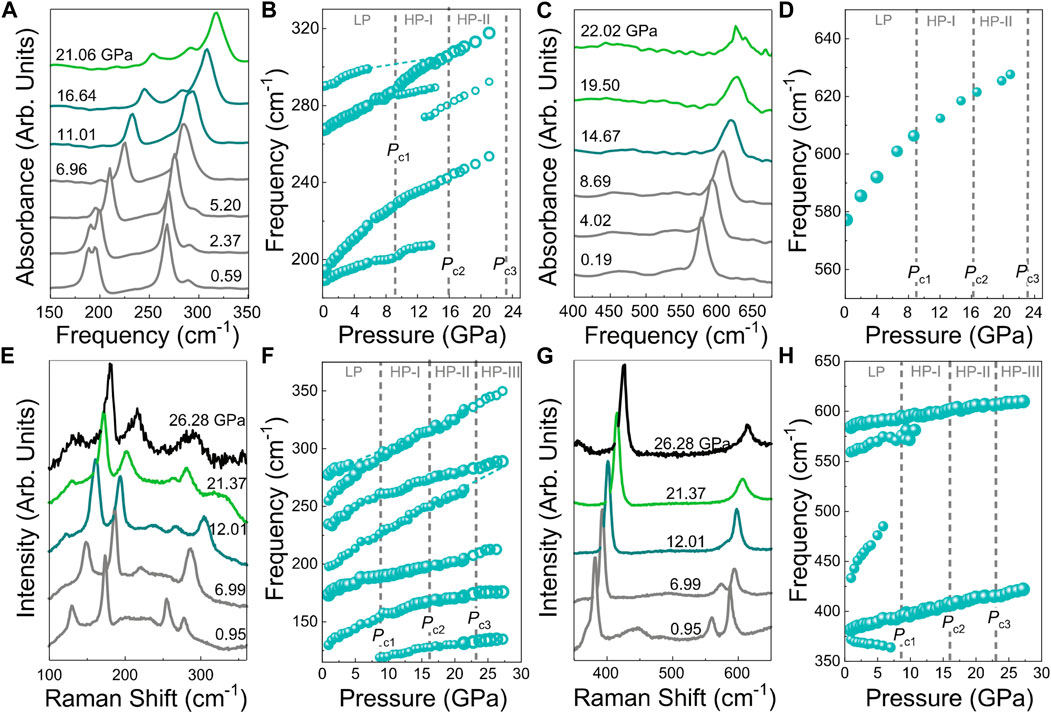
Figure 8. Vibrational properties of NiPS3 under compression. (A–D) Close-up view of the infrared absorption and frequency vs. pressure plots. (E–H) Close-up view of the Raman scattering response and frequency vs. pressure plots. Spectra for the low pressure (LP) phase are shown in gray; those for the high pressure phases (HP-I, HP-II, and HP-III) are shown in teal, green, and black, respectively. Closed and open circles represent two independent runs. Note that infrared spectra in the HP-III phase are not included because the phonons are screened by the metallic response. The critical pressures (PC’s) separate the high pressure phases and are indicated with dashed vertical gray lines. Panels (A–H) from (Harms et al., 2022a).
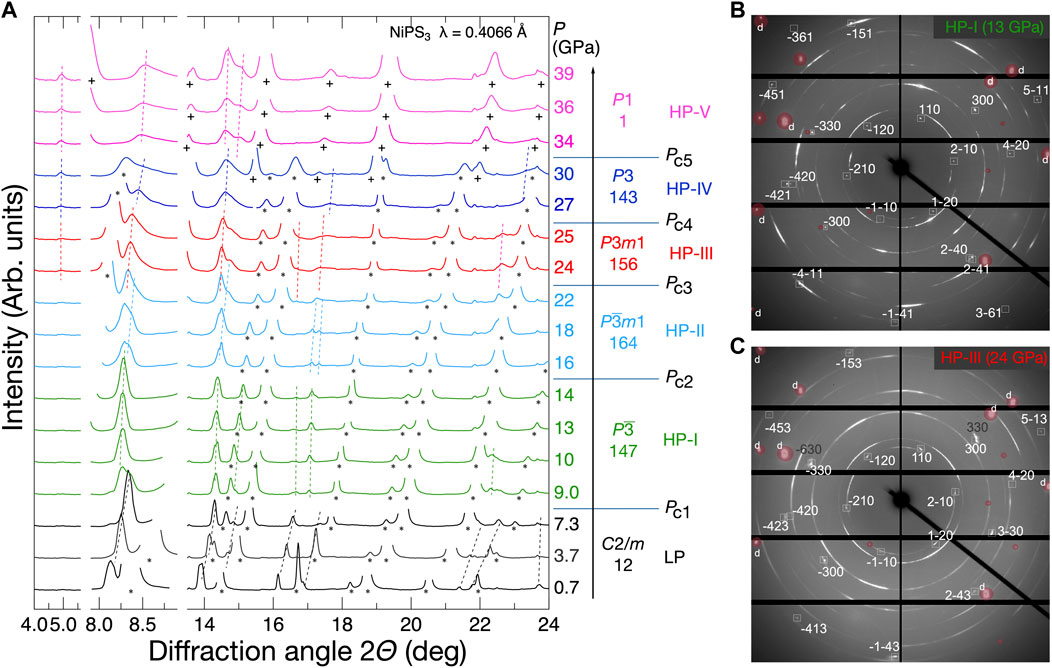
Figure 9. High pressure x-ray diffraction results of NiPS3 up to 39 GPa. (A) Integrated x-ray diffraction profiles at indicated pressures. The appearance of x-ray diffraction peaks at the given angle are indicated, alongside dashed lines to guide the eye. Critical pressures, dividing individual pressure phases, are indicated by solid blue horizontal lines. The space groups of individual high pressure phases are labeled. The asterisk (*) and plus (+) marks correspond to the diffraction response from NaCl in B1-type structure (below 30 GPa) and B2-type (above 30 GPa). (B) and (C) The x-ray diffraction images on the detector for HP-I (13 GPa) and HP-III (24 GPa). The peaks from NiPS3 are enclosed with squares alongside the hkl indices for each phase. The gray labels in the panel (C) indicate the diffraction from HP-II. The Bragg diffraction from the diamond anvils is masked with red circles.Panels (A–C) from (Harms et al., 2022a).
NiPSe3 is different, primarily because it does not have a complicated set of symmetry progressions under compression. Instead, there is a relatively simple structural sequence as shown in Figure 10 and Table 3, (Harms et al., 2022a; Sun et al., 2023). More recent efforts involving combined synchrotron x-ray diffraction and first-principles theory confirm these two structural transitions: LP to HP-I (where the honeycomb layers slide relative to each other) and HP-I to HP-II (where the structure has more three-dimensional character) (Sun et al., 2023). There is also a concomitant magnetic crossover at PC1 where the direction of the antiferromagnetic moment switches from out-of-plane to in-plane. Complementary resistance measurements indicate a bandwidth-controlled Mott insulator-metal transition near 8.0 GPa. The latter coincides with the emergence of superconductivity with Tc ≈ 4.8 K (Sun et al., 2023). This leads to a remarkable and complex pressure-temperature phase diagram hosting coexisting zigzag antiferromagnetic order and superconductivity (Figure 11).
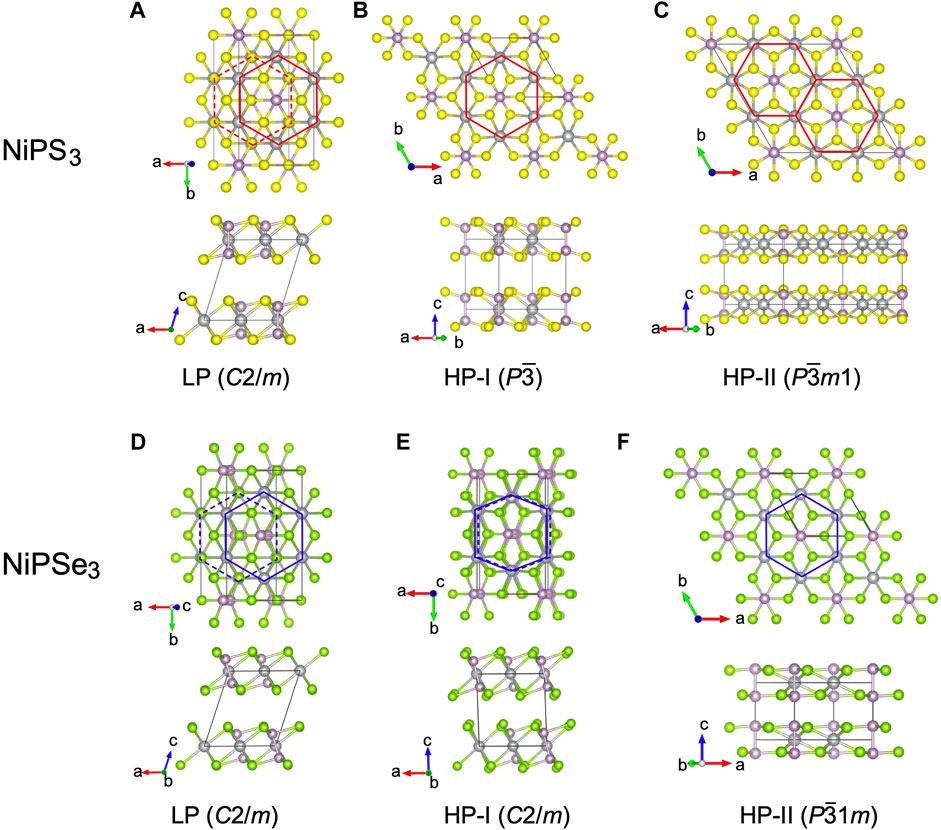
Figure 10. Crystal structures of NiPS3 and NiPSe3 in LP ((A) and (D)), HP-I ((B) and (E)), and HP-II ((C) and (F)) phases visualized using VESTA3 (Momma and Izumi, 2011) based on the experimental reports (Harms et al., 2022a; Sun et al., 2023). As discussed in the text, NiPS3 displays an unusual sequence under pressure: C2/m with β ∼ 108°→ P
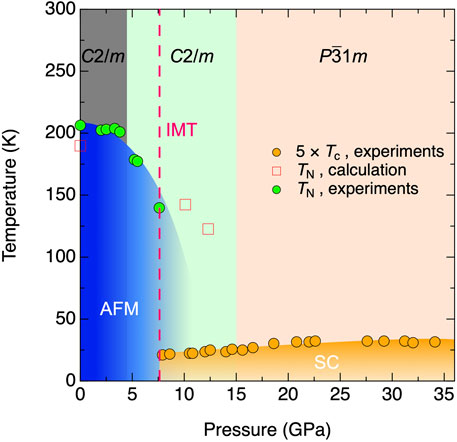
Figure 11. Phase diagram of NiPSe3. Structural phase boundaries are from x-ray diffraction experiments at room temperature. TN for AFM order and TC for superconductivity are from electrical resistance measurements and DFT calculations. Created based on data from (Sun et al., 2023).
Finally, we point out that transition metal dichalcogenides such as MoS2 and WS2 and related nanostructures are employed for solid state lubrication due to their ultra-low friction coefficients - especially under high shear conditions (Musfeldt et al., 2020). In an important initiative, Deng et al. (2024) are started to explore the mechanical properties of terenary chalcogenides like NiPS3 using lateral force microscopy (Deng et al., 2024). These measurements reveal a friction coefficient of approximately 4.5 × 10−3 under 5 × 10−3 Pa with load up to 768 nN. These findings are discussed in terms of the extremely weak interlayer interactions. Table 1 summarizes other candidates with low layer thickness/van der Waals gap ratios including CdPS3 and FePSe3 that should be tested.
2.4 V1−xPS3 (x < 1)
The vanadium phosphate synthesis has been achieved with the vanadium deficiency in V1−xPS3 which can be explained as due to valence mixing on the vanadium site between V2+ and V3+ states (Ouvrard et al., 1985b; Ichimura and Sano, 1991). The LP-phase has an electrical conductivity higher than 10–5 Ω(−1)cm(−1), while MnPS3, NIPS3 and ZnPS3 crystals lower than 10–9 Ω(−1)cm(−1) at room temperature. The high conductivity of the former crystals is attributed to their mixed valency on the metal and/or the S and P sites, which has been revealed by x-ray photoelectron spectroscopy (Ichimura and Sano, 1991). The LP → HP-I transition starts at 2.6 GPa followed by the gradual transition to HP-I completing near 8.0 GPa (Coak et al., 2019). The layers of V0.9PS3 shift relative to each other in a sliding motion of ∼ a/3 along the a-axis such that the S atoms become arranged in a hexagonal closed packing layout between the layers (Table 2; Figure 2), (Coak et al., 2019). In HP-I, P atoms are slightly distorted along the a-axis (the x coordinate value is 0.0074 at 17.7 GPa) of the unit cell, and this distortion results in the same C2/m symmetry (Coak et al., 2019). The volume per formula unit decreases continuously without a sudden or discontinuous changes across the gradual LP → HP-I transformation (Figure 4). Coak et al. (2019) The electrical resistance decreases significantly and continuously with increasing pressure, and then the insulator-to-metal transition occurs near 12 GPa above the pressure where the LP → HP-I transformation completes (Coak et al., 2019). Thus, the isostructural insulator-to-metal transition is concluded, suggesting a second-order transition (Coak et al., 2019). From the magnetoresistance data, Coak et al. claim the insulator-to-metal transition involves the antiferromagnetic to paramagnetic transition (Coak et al., 2019). The R vs. T curve in the metallic phase shows an upturn at low temperatures. Coak et al. (2019) suggest that Kondo effect due to the vanadium deficiency and disordered valence mixing on the vanadium sites (Coak et al., 2019). The synthesis of VPSe3 has not been reported to the knowledge of the authors.
2.5 CoPS3 and CoPSe3
Like other structural siblings, CoPS3 has gained significant interest recently for the study of two-dimensional magnetism. Since Co2+ ion is in the d7 configuration with a hole in the t2g shell, significant effects of spin-orbit coupling on magnetism is expected. Indeed, recent theoretical studies suggested an interesting way to realize the so-called Kitaev’s exchange interactions in cobalt-based compounds, (Kitaev, 2006; Liu and Khaliullin, 2018; Sano et al., 2018) which were originally considered in heavier transition metal systems (Jackeli and Khaliullin, 2009). Several Co-based systems including CoPS3 have been studied for the search of the Kitaev-induced frustrated magnetism (Kim et al., 2020; Kim et al., 2021; Lin et al., 2021; Kim et al., 2023; Zhang et al., 2023). Considering the presence of metastable spin states and significant spin-orbit coupling in Co ions, (Yamaguchi et al,. 1996; Zobel et al., 2002). It is expected that pressure may induce intriguing evolution of electronic and magnetic properties of CoPS3.
CoPS3 crystallizes in C2/m structure at ambient pressure as other MPS3 family at ambient pressure. CoPS3 exhibits AFM at ambient pressure with a TN = 122 K and a Weiss temperature of θ = −166 K (Ouvrard et al., 1982; Wildes et al., 2017). Single-crystal neutron diffraction shows that the magnetic propagation vector is k = [010] with the moments mostly along the a-axis and with a small component along the c-axis (Ouvrard et al., 1982; Wildes et al., 2017). As observed for NiPS3, this magnetic structure is in sharp contrast to MnPS3 and FePS3 whose moments are mostly along the c-axis. Its effective moment is 4.9 μB, slightly larger than the expected value for a pure spin moment of a Co2+ cation (Ouvrard et al., 1982), implying orbital contribution to the magnetization as mentioned above. In addition, an inelastic neutron scattering study suggests a XXZ-type antiferromagneic order in CoPS3, signalling the presence of the spin-orbit coupling-induced magnetic anisotropy (Kim et al., 2020).
High pressure properties of CoPS3 have not been experimentally reported until recently, probably due to the significant difficulty in the synthesis and single crystal growth of this compound. A first-principles calculation from an article dedicated to the pressure effects in CoPS3 predicts a pressure-driven isostructural Mott transition accompanied by a spin crossover (Gu et al. 2021). x-ray diffraction and Raman scattering measurements reveal that CoPS3 exhibits C2/m (LP) → P
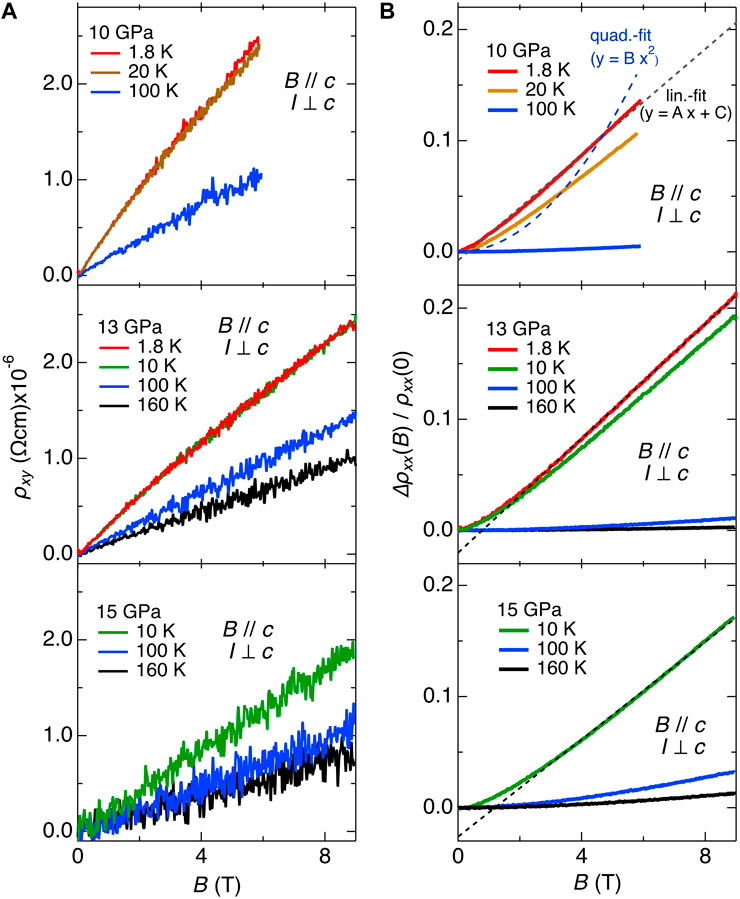
Figure 12. Transverse magnetotransport data from metallic CoPS3. (A) The ρxy at different temperatures. The data at 1.8 K and 20 K at 10 GPa overlap (top panel). The data at 1.8 K and 10 K at 13 GPa overlap (middle panel). (B) The magnetoresistance. The dotted (black) and dashed (blue) lines in the top panel indicate the linear fit, y = Ax + C and quadratic fits y = Bx2 to the data at 10 GPa and 1.8 K (Matsuoka et al., 2023). Reprinted (Figure 5) with permission from [Matsuoka, T., Rao, R., Susner, M. A., Conner, B. S., Zhang, D., and Mandrus, D., Pressure-induced insulator-to-metal transition in the van der Waals compound CoPS3, 107, 165125 (2023)).] Copyright (2023) by the American Physical Society.
2.6 CdPS3 and CdPSe3
CdPS3 crystallizes in the C2/m structure with the monoclinic angle β = 107.27°, possessing an insulating electrical character like other MPX3 family members (Ouvrard et al., 1985a; Niu et al., 2022). The Raman scattering measurements combined with ab initio calculation reveal that CdPS3 exhibits two structural transformations the LP-phase (C2/m) → HP-I phase (R
3 Summary and future prospects
Complex chalcogenides display properties and states of matter that are incredibly sensitive to external stimuli. This particular review is inspired by opportunities to employ pressure to unveil elusive states of matter and uncover charge-structure-function relationships. Compression is particularly well-suited to tuning the properties of chalcogenides because, in addition to changing bond lengths and angles, it provides deterministic control of the c/a structural ratio and the van der Waals gap. By controlling the environment around the metal center, compression also controls spin. As emphasized throughout this review, strain is likely to offer complementary advantages. At the same time, expanding the chemical phase space of the MPX3 system to include solid solutions like (Zn,Cd)PS3 or (Ni,Co)PS3, heavy 4- and 5d-metal centers to enhance spin-orbit coupling, the CrPS4 member of this family, and dual sublattice analogs such as bimetallic CuInP2S6 and AgInP2S6 provides exciting new platforms for exploring ferroelectricity, new types of photoinduced behavior, ionic motion, and even charge pumping under compression.
Author contributions
TM: Funding acquisition, Visualization, Writing–original draft, Writing–review and editing. H-SK: Visualization, Writing–original draft, Writing–review and editing, Funding acquisition. SS: Writing–original draft, Writing–review and editing. JM: Funding acquisition, Visualization, Writing–original draft, Writing–review and editing. DM: Funding acquisition, Visualization, Writing–original draft, Writing–reviewand editing.
Funding
The authors declare that financial support was received for the research, authorship, and/or publication of this article. TM is supported by the Air Force Office of Scientific Research under award number FA2386-23-1-4112, Dr. Emerlinda Roman Professorial Chair Grant awarded from University of the Philippines Foundation (2023–2024), and the NIP research contract (2023). HSK and SS are funded by the Korea Research Fellow (KRF) Program and the Basic Science Research Program through the National Research Foundation of Korea funded by the Ministry of Education (Grant No. NRF-2019H1D3A1A01102984, 424 NRF-2020R1C1C1005900, and RS-2023-00220471). JLM is funded by Physical Behavior of Materials, Basic Energy Sciences, U.S. Department of Energy (Contract number DE-SC00023144). DGM is funded by the Gordon and Betty Moore Foundation’s EPiQS Initiative, Grant No. GBMF9069.
Acknowledgments
The authors thank our collaborators for valuable discussions: Dr. Nathan C. Harms, Dr. Amanda Haglund, Dr. Amanda J. Clune, Dr. Rui Xue, Dr. Kevin A. Smith, Dr. Kenneth R. O’Neal, and Dr. Maik Lang from The University of Tennesee Knoxville, Dr. Jesse S. Smith and Dr. Dongzhou Zhang from Argonne National Laboratory, Dr. Erxi Feng, Dr. Huibo Cao, and Dr. Antonio M. dos Santos from Oak Ridge National Laboratory, Dr. Rahul Rao, Dr. Michael A. Susner, and Dr. Benjamin S. Conner from Air Force Research Laboratory, Dr. Zhenxian Liu from The University of Illinois Chicago, Dr. Kristjan Haule and Dr. David Vanderbilt from Rutgers University.
Conflict of interest
The authors declare that the research was conducted in the absence of any commercial or financial relationships that could be construed as a potential conflict of interest.
Publisher’s note
All claims expressed in this article are solely those of the authors and do not necessarily represent those of their affiliated organizations, or those of the publisher, the editors and the reviewers. Any product that may be evaluated in this article, or claim that may be made by its manufacturer, is not guaranteed or endorsed by the publisher.
References
Afanasiev, D., Hortensius, J. R., Matthiesen, M., Mañas-Valero, S., Šiškins, M., Lee, M., et al. (2021). Controlling the anisotropy of a van der Waals antiferromagnet with light. Sci. Adv. 7, eabf3096. doi:10.1126/sciadv.abf3096
Aoyama, T., Yamauchi, K., Iyama, A., Picozzi, S., Shimizu, K., and Kimura, T. (2014). Giant spin-driven ferroelectric polarization in TbMnO3 under high pressure. Nat. Commun. 5, 4927. doi:10.1038/ncomms5927
Basnet, R., Ford, D., TenBarge, K., Lochala, J., and Hu, J. (2022a). Emergence of ferrimagnetism in Li-intercalated NiPS3. J. Phys. Condens. Matter 34, 434002. doi:10.1088/1361-648X/ac8a81
Basnet, R., Kotur, K. M., Rybak, M., Stephenson, C., Bishop, S., Autieri, C., et al. (2022b). Controlling magnetic exchange and anisotropy by nonmagnetic ligand substitution in layered MPX3 (M= Ni, Mn; X= S, Se). Phys. Rev. Res. 4, 023256. doi:10.1103/physrevresearch.4.023256
Basnet, R., Wegner, A., Pandey, K., Storment, S., and Hu, J. (2021). Highly sensitive spin-flop transition in antiferromagnetic van der Waals material MPS3 (M = Ni and Mn). Phys. Rev. Mater. 5, 064413. doi:10.1103/PhysRevMaterials.5.064413
Bhutani, A., Zuo, J. L., McAuliffe, R. D., dela Cruz, C. R., and Shoemaker, D. P. (2020). Strong anisotropy in the mixed antiferromagnetic system Mn1−xFexPSe3. Phys. Rev. Mater. 4, 034411. doi:10.1103/PhysRevMaterials.4.034411
Boerio-Goates, J., Lifshitz, E., and Francis, A. H. (1981). Electronic spectroscopy of nearly octahedrally coordinated manganese in manganese phosphide sulfide (MnPS3) and cadmium phosphide sulfide (CdPS3) lattices. Inorg. Chem. 20, 3019–3023. doi:10.1021/ic50223a052
Burch, K. S., Mandrus, D., and Park, J.-G. (2018). Magnetism in two-dimensional van der waals materials. Nature 563, 47–52. doi:10.1038/s41586-018-0631-z
Butler, C., Yoshida, M., Hanaguri, T., and Iwasa, Y. (2020). Mottness versus unit-cell doubling as the driver of the insulating state in 1T-TaS2. Nat. Commun. 11, 2477. doi:10.1038/s41467-020-16132-9
Chandrasekharan, N., and Vasudevan, S. (1994). Magnetism and exchange in the layered antiferromagnet NiPS3. J. Phys. Condens. Matter 6, 4569–4579. doi:10.1088/0953-8984/6/24/017
Coak, M. J., Jarvis, D. M., Hamidov, H., Haines, C. R. S., Alireza, P. L., Liu, C., et al. (2020). Tuning dimensionality in van-der-Waals antiferromagnetic Mott insulators TMPS3. J. Phys. Condens. Matter 32, 124003. doi:10.1088/1361-648X/ab5be8
Coak, M. J., Jarvis, D. M., Hamidov, H., Wildes, A. R., Paddison, J. A. M., Liu, C., et al. (2021). Emergent magnetic phases in pressure-tuned van der Waals antiferromagnet FePS3. Phys. Rev. X 11, 011024. doi:10.1103/PhysRevX.11.011024
Coak, M. J., Son, S., Daisenberger, D., Hamidov, H., Haines, C. R. S., Alireza, P. L., et al. (2019). Isostructural Mott transition in 2D honeycomb antiferromagnet V0.9PS3. npj Quantum Mater. 4, 38. doi:10.1038/s41535-019-0178-8
Cui, H., Yun, S., Lee, K. J., Lee, C., Chang, S. H., Lee, Y., et al. (2021). Quasihydrostatic versus nonhydrostatic pressure effects on the electrical properties of NiPS3. Phys. Rev. Mater. 5, 124008. doi:10.1103/PhysRevMaterials.5.124008
Dedkov, Y., Guo, Y., and Voloshina, E. (2023). Progress in the studies of electronic and magnetic properties of layered MPX3 materials (M: transition metal, X: chalcogen). Electron. Struct. 5, 043001. doi:10.1088/2516-1075/acfa4e
Deng, H., Yu, T., Du, C., Shen, R., Zhao, Y., He, X., et al. (Friction, under review, (2024)). NiPS3: a ternary 2D material with an ultra-low friction coefficient.
Deng, S., Chen, S., Monserrat, B., Artacho, E., and Saxena, S. S. (2023). Pressure-induced transitions in FePS3: structural, magnetic and electronic properties. SciPost Phys. 15, 020. doi:10.21468/SciPostPhys.15.1.020
Friedel, M. C. (1894). Sur une nouvelle série de sulfophosphures, les thiohypophosphates. Comptes rendus l’Académie Sci. 119, 260–264.
Fujii, M., Yamaguchi, T., Ohkochi, T., De, C., Cheong, S.-W., and Mizokawa, T. (2022). Bulk and surface electronic structure of MnPSe3 revealed by photoemission and x-ray absorption spectroscopy. Phys. Rev. B 106, 035118. doi:10.1103/PhysRevB.106.035118
Grasso, V., Neri, F., Perillo, P., Silipigni, L., and Piacentini, M. (1991). Optical-absorption spectra of crystal-field transitions in MnPS3 at low temperatures. Phys. Rev. B 44, 11060–11066. doi:10.1103/PhysRevB.44.11060
Gu, Y., Zhang, S., and Zou, X. (2021). Tunable magnetism in layered CoPS3 by pressure and carrier doping. Sci. China Mater. 64, 673–682. doi:10.1007/s40843-020-1453-0
Haines, C. R. S., Coak, M. J., Wildes, A. R., Lampronti, G. I., Liu, C., Nahai-Williamson, P., et al. (2018). Pressure-induced electronic and structural phase evolution in the van der Waals compound FePS3. Phys. Rev. Lett. 121, 266801. doi:10.1103/PhysRevLett.121.266801
Harms, N. C., Kim, H.-s., Clune, A. J., Smith, K. A., O’Neal, K. R., Haglund, A. V., et al. (2020). Piezochromism in the magnetic chalcogenide MnPS3. npj Quantum Mater. 5, 56. doi:10.1038/s41535-020-00259-5
Harms, N. C., Matsuoka, T., Samanta, S., Clune, A. J., Smith, K. A., Haglund, A. V., et al. (2022a). Symmetry progression and possible polar metallicity in NiPS3 under pressure. npj 2D Mater. Appl. 6, 40. doi:10.1038/s41699-022-00313-9
Harms, N. C., Smith, K. A., Haglund, A. V., Mandrus, D. G., Liu, Z., Kim, H.-s., et al. (2022b). Metal site substitution and role of the dimer on symmetry breaking in FePS3 and CrPS4 under pressure. ACS Appl. Electron. Mater. 4, 3246–3255. doi:10.1021/acsaelm.2c00563
Hiroi, Z. (2008). A brief comment on the chemical formulae of the rare earth iron arsenide oxide superconductors. arXiv0805.4668. doi:10.48550/arXiv.0805.4668
Ichimura, K., and Sano, M. (1991). Electrical conductivity of layered transition-metal phosphorus trisulfide crystals. Synth. Met. 45, 203–211. doi:10.1016/0379-6779(91)91804-J
Jackeli, G., and Khaliullin, G. (2009). Mott insulators in the strong spin-orbit coupling limit: from Heisenberg to a quantum compass and Kitaev models. Phys. Rev. Lett. 102, 017205. doi:10.1103/PhysRevLett.102.017205
Jophnson, J. W., and Jacobson, A. (1982). “Intercalation chemistry of metal phosphorus trichalcogenides,” in Intercalation chemistry (Academic Press Inc New York), 267–283.
Joy, P. A., and Vasudevan, S. (1992). Magnetism in the layered transition-metal thiophosphates MPS3 (M =Mn, Fe, and Ni). Phys. Rev. B 46, 5425–5433. doi:10.1103/PhysRevB.46.5425
Kang, S., Kim, K., Kim, B. H., Kim, J., Sim, K. I., Lee, J.-U., et al. (2020). Coherent many-body exciton in van der Waals antiferromagnet NiPS3. Nature 583, 785–789. doi:10.1038/s41586-020-2520-5
Kargar, F., Aytan, E., Ghosh, S., Lee, J., Gomez, M., Liu, Y., et al. (2019). Phonon and thermal properties of quasi-two-dimensional FePS3 and MnPS3 antiferromagnetic semiconductor materials. ACS Nano 14, 2424–2435. doi:10.1021/acsnano.9b09839
Kim, C., Jeong, J., Lin, G., Park, P., Masuda, T., Asai, S., et al. (2021). Antiferromagnetic kitaev interaction in Jeff = 1/2 cobalt honeycomb materials Na3Co2SbO6 and Na2Co2TeO6. J. Phys. Condens. Matter 34, 045802. doi:10.1088/1361-648X/ac2644
Kim, C., Jeong, J., Park, P., Masuda, T., Asai, S., Itoh, S., et al. (2020). Spin waves in the two-dimensional honeycomb lattice xxz-type van der waals antiferromagnet CoPS3. Phys. Rev. B 102, 184429. doi:10.1103/PhysRevB.102.184429
Kim, C., Kim, S., Park, P., Kim, T., Jeong, J., Ohira-Kawamura, S., et al. (2023). Bond-dependent anisotropy and magnon decay in cobalt-based Kitaev triangular antiferromagnet. Nat. Phys. 19, 1624–1629. doi:10.1038/s41567-023-02180-7
Kim, H.-S., Haule, K., and Vanderbilt, D. (2019). Mott metal-insulator transitions in pressurized layered trichalcogenides. Phys. Rev. Lett. 123, 236401. doi:10.1103/PhysRevLett.123.236401
Kim, M., Kim, H.-S., Haule, K., and Vanderbilt, D. (2022). Orbital-selective mott phase and non-Fermi liquid in FePS3. Phys. Rev. B 105, L041108. doi:10.1103/PhysRevB.105.L041108
Kim, S. Y., Kim, T. Y., Sandilands, L. J., Sinn, S., Lee, M. C., Son, J., et al. (2018). Charge-spin correlation in van der Waals antiferromagnet NiPS3. Phys. Rev. Lett. 120, 136402. doi:10.1103/PhysRevLett.120.136402
Kitaev, A. (2006). Anyons in an exactly solved model and beyond. Ann. Phys. 321, 2–111. January Special Issue. doi:10.1016/j.aop.2005.10.005
Klingen, W., Ott, R., and Hahn, H. (1973). Uber die darstellung und eigenschaften von hexathio-und hexaselenohypodiphosphaten. Z. für Anorg. Allg. Chem. 396, 271–278. doi:10.1002/zaac.19733960305
Kuntu, D. V., Arkhipova, E. A., Shelukhin, L. A., Mertens, F., Prosnikov, M. A., Eliseyev, I. A., et al. (2024). Laser-induced demagnetization in van der Waals XY- and ising-like antiferromagnets NiPS3 and FePS3. Phys. Rev. Mat. 8, 014408. doi:10.1103/PhysRevMaterials.8.014408
Kurosawa, K., Saito, S., and Yamaguch, Y. (1983a). Neutron diffraction study on MnPS3 and FePS3. J. Phys. Soc. Jpn. 52, 3919–3926. doi:10.1143/JPSJ.52.3919
Kurosawa, K., Saito, S., and Yamaguchi, Y. (1983b). Neutron diffraction study on MnPS3 and FePS3. J. Phys. Soc. Jpn. 52, 3919–3926. doi:10.1143/JPSJ.52.3919
Lançon, D., Walker, H. C., Ressouche, E., Ouladdiaf, B., Rule, K. C., McIntyre, G. J., et al. (2016). Magnetic structure and magnon dynamics of the quasi-two-dimensional antiferromagnet FePS3. Phys. Rev. B 94, 214407. doi:10.1103/PhysRevB.94.214407
Lane, C., and Zhu, J.-X. (2020). Thickness dependence of electronic structure and optical properties of a correlated van der Waals antiferromagnetic NiPS3 thin film. Phys. Rev. B 102, 075124. doi:10.1103/PhysRevB.102.075124
Lee, J.-U., Lee, S., Ryoo, J. H., Kang, S., Kim, T. Y., Kim, P., et al. (2016). Ising-type magnetic ordering in atomically thin FePS3. Nano Lett. 16, 7433–7438. doi:10.1021/acs.nanolett.6b03052
Le Flem, G., Brec, R., Ouvard, G., Louisy, A., and Segransan, P. (1982). Magnetic interactions in the layer compounds MPX3 (M = Mn, Fe, Ni; X = S, Se). J. Phys. Chem. Solids 43, 455–461. doi:10.1016/0022-3697(82)90156-1
Lin, G., Jeong, J., Kim, C., Wang, Y., Huang, Q., Masuda, T., et al. (2021). Field-induced quantum spin disordered state in spin−1/2 honeycomb magnet Na2Co2TeO6. Nat. Commun. 12, 5559. doi:10.1038/s41467-021-25567-7
Liu, B., Yang, Y., Fu, Y., and He, J. (2022). Study on physical properties of two-dimensional layered magnetic semiconductor material FePSe3. Adv. Condens. Matter Phys. 11, 21–27. doi:10.12677/CMP.2022.112003
Liu, H., and Khaliullin, G. (2018). Pseudospin exchange interactions in d7 cobalt compounds: possible realization of the Kitaev model. Phys. Rev. B 97, 014407. doi:10.1103/PhysRevB.97.014407
Lu, Z., Yang, X., Huang, L., Chen, X., Liu, M., Peng, J., et al. (2022). Evolution of magnetic phase in two-dimensional van der Waals Mn1−xNixPS3 single crystals. J. Phys. Condens. Matter 34, 354005. doi:10.1088/1361-648X/ac7a80
Ma, X., Wang, Y., Yin, Y., Yue, B., Dai, J., Cheng, J., et al. (2021). Dimensional crossover tuned by pressure in layered magnetic NiPS3. Sci. China Phys. Mech. I& Astronomy 64, 297011. doi:10.1007/s11433-021-1727-6
Mai, T. T., Garrity, K. F., McCreary, A., Argo, J., Simpson, J. R., Doan-Nguyen, V., et al. (2021). Magnon-phonon hybridization in 2D antiferromagnet MnPSe3. Sci. Adv. 7, eabj3106–7. doi:10.1126/sciadv.abj3106
Matsuoka, T., Haglund, A., Xue, R., Smith, J. S., Lang, M., dos Santos, A. M., et al. (2021). Pressure-induced insulator–metal transition in two-dimensional mott insulator NiPS3. J. Phys. Soc. Jpn. 90, 124076. doi:10.7566/JPSJ.90.124706
Matsuoka, T., Rao, R., Susner, M. A., Conner, B. S., Zhang, D., and Mandrus, D. (2023). Pressure-induced insulator-to-metal transition in the van der Waals compound CoPS3. Phys. Rev. B 107, 165125. doi:10.1103/PhysRevB.107.165125
Mehlawat, K., Alfonsov, A., Selter, S., Shemerliuk, Y., Aswartham, S., Büchner, B., et al. (2022). Low-energy excitations and magnetic anisotropy of the layered van der Waals antiferromagnet Ni2P2S6. Phys. Rev. B 105, 214427. doi:10.1103/PhysRevB.105.214427
Momma, K., and Izumi, F. (2011). VESTA3 for three-dimensional visualization of crystal, volumetric and morphology data. J. Appl. Crystallogr. 44, 1272–1276. doi:10.1107/S0021889811038970
Musfeldt, J. L., Iwasa, Y., and Tenne, R. (2020). Nanotubes from layered transition metal dichalcogenides. Phys. Today 73, 42–48. doi:10.1063/PT.3.4547
Musfeldt, J. L., Mandrus, D. G., and Liu, Z. (2023). Insulator–metal transition in CrSiTe3 triggered by structural distortion under pressure. npj 2D Mater. Appl. 7, 28–36. doi:10.1038/s41699-023-00389-x
Nauman, M., Kiem, D. H., Lee, S., Son, S., Park, J.-G., Kang, W., et al. (2021). Complete mapping of magnetic anisotropy for prototype ising van der waals FePS3. 2D Mater. 8, 035011. doi:10.1088/2053-1583/abeed3
Neal, S. N., Kim, H.-S., O’Neal, K. R., Haglund, A. V., Smith, K. A., Mandrus, D. G., et al. (2020). Symmetry crossover in layered MPS3 complexes (M = Mn, Fe, Ni). Phys. Rev. B 102, 085408. doi:10.1103/PhysRevB.102.085408
Neal, S. N., Kim, H.-S., Smith, K. A., Haglund, A. V., Mandrus, D. G., Bechtel, H. A., et al. (2019). Near-field infrared spectroscopy of monolayer MnPS3. Phys. Rev. B 100, 075428. doi:10.1103/PhysRevB.100.075428
Niu, M., Cheng, H., Li, X., Yu, J., Yang, X., Gao, Y., et al. (2022). Pressure-induced phase transitions in weak interlayer coupling CdPS3. Appl. Phys. Lett. 120, 233104. doi:10.1063/5.0089478
Ouvrard, G., Brec, R., and Rouxel, J. (1982). Synthesis and physical characterization of the lamellar compound CoPS3. Chem. Inf. 13, 971. doi:10.1002/chin.198235037
Ouvrard, G., Brec, R., and Rouxel, J. (1985a). Structural determination of some MPS3 layered phases (M = Mn, Fe, Co, Ni and Cd). Mater. Res. Bull. 20, 1181–1189. doi:10.1016/0025-5408(85)90092-3
Ouvrard, G., Fréour, R., Brec, R., and Rouxel, J. (1985b). A mixed valence compound in the two dimensional MPS3 family: V0.78PS3 structure and physical properties. Mater. Res. Bull. 20, 1053–1062. doi:10.1016/0025-5408(85)90204-1
Pei, Q., Wang, X.-C., Zou, J.-J., and Mi, W.-B. (2018). Tunable electronic structure and magnetic coupling in strained two-dimensional semiconductor MnPSe3. Front. Phys. 13, 137105. doi:10.1007/s11467-018-0796-9
Pei, S., Wang, Z., and Xia, J. (2022). High pressure studies of 2D materials and heterostructures: a review. Mater. Des. 213, 110363. doi:10.1016/j.matdes.2021.110363
Peng, J., Yang, X., Lu, Z., Huang, L., Chen, X., He, M., et al. (2023). Ferromagnetism induced by magnetic dilution in van der Waals material metal thiophosphates. Adv. Quantum Technol. 6, 2200105. doi:10.1002/qute.202200105
Ressouche, E., Loire, M., Simonet, V., Ballou, R., Stunault, A., and Wildes, A. (2010). Magnetoelectric MnPS3 as a candidate for ferrotoroidicity. Phys. Rev. B 82, 100408. doi:10.1103/PhysRevB.82.100408
Sano, R., Kato, Y., and Motome, Y. (2018). Kitaev-Heisenberg Hamiltonian for high-spin d7 mott insulators. Phys. Rev. B 97, 014408. doi:10.1103/PhysRevB.97.014408
Shannon, R. D. (1976). Revised effective ionic radii and systematic studies of interatomic distances in halides and chalcogenides. Acta Crystallogr. Sect. A 32, 751–767. doi:10.1107/S0567739476001551
Sun, H., Qiu, L., Han, Y., Yi, E., Li, J., Huo, M., et al. (2023). Coexistence of zigzag antiferromagnetic order and superconductivity in compressed NiPSe3. Mater. Today Phys. 36, 101188. doi:10.1016/j.mtphys.2023.101188
Sun, Y. J., Tan, Q. H., Liu, X. L., Gao, Y. F., and Zhang, J. (2019). Probing the magnetic ordering of antiferromagnetic MnPS3 by Raman spectroscopy. J. Phys. Chem. Lett. 10, 3087–3093. doi:10.1021/acs.jpclett.9b00758
Tezze, D., Pereira, J. M., Asensio, Y., Ipatov, M., Calavalle, F., Casanova, F., et al. (2022). Tuning the magnetic properties of NiPS3 through organic-ion intercalation. Nanoscale 14, 1165–1173. doi:10.1039/D1NR07281A
Vaclavkova, D., Delhomme, A., Faugeras, C., Potemski, M., Bogucki, A., Suffczyński, J., et al. (2020). Magnetoelastic interaction in the two-dimensional magnetic material MnPS3 studied by first principles calculations and Raman experiments. 2D Mater. 7, 035030. doi:10.1088/2053-1583/ab93e3
Wang, F., Shifa, T. A., Yu, P., He, P., Liu, Y., Wang, F., et al. (2018a). New Frontiers on van der Waals layered metal phosphorous trichalcogenides. Adv. Funct. Mater. 28, 1802151. doi:10.1002/adfm.201802151
Wang, Y., Ying, J., Zhou, Z., Sun, J., Wen, T., Zhou, Y., et al. (2018b). Emergent superconductivity in an iron-based honeycomb lattice initiated by pressure-driven spin-crossover. Nat. Commun. 9, 1914. doi:10.1038/s41467-018-04326-1
Wang, Y., Zhou, Z., Wen, T., Zhou, Y., Li, N., Han, F., et al. (2016). Pressure-driven cooperative spin-crossover, large-volume collapse, and semiconductor-to-metal transition in manganese(II) honeycomb lattices. J. Am. Chem. Soc. 138, 15751–15757. doi:10.1021/jacs.6b10225
Wiedenmann, A., Rossat-Mignod, J., Louisy, A., Brec, R., and Rouxel, J. (1981). Neutron diffraction study of the layered compounds MnPSe3 and FePSe3. Solid State Commun. 40, 1067–1072. doi:10.1016/0038-1098(81)90253-2
Wildes, A. R., Fåk, B., Hansen, U. B., Enderle, M., Stewart, J. R., Testa, L., et al. (2023). Spin wave spectra of single crystal CoPS3. Phys. Rev. B 107, 054438. doi:10.1103/PhysRevB.107.054438
Wildes, A. R., Kennedy, S. J., and Hicks, T. J. (1994). True two-dimensional magnetic ordering in MnPS3. J. Phys. Condens. Matter 6, L335–L341. doi:10.1088/0953-8984/6/24/002
Wildes, A. R., Okamoto, S., and Xiao, D. (2021). Search for nonreciprocal magnons in MnPS3. Phys. Rev. B 103, 024424. doi:10.1103/PhysRevB.103.024424
Wildes, A. R., Simonet, V., Ressouche, E., Ballou, R., and McIntyre, G. J. (2017). The magnetic properties and structure of the quasi-two-dimensional antiferromagnet CoPS3. J. Phys. Condens. Matter 29, 455801. doi:10.1088/1361-648X/aa8a43
Wildes, A. R., Simonet, V., Ressouche, E., McIntyre, G. J., Avdeev, M., Suard, E., et al. (2015). Magnetic structure of the quasi-two-dimensional antiferromagnet NiPS3. Phys. Rev. B 92, 224408. doi:10.1103/PhysRevB.92.224408
Yamaguchi, S., Okimoto, Y., Taniguchi, H., and Tokura, Y. (1996). Spin-state transition and high-spin polarons in LaCoO3. Phys. Rev. B 53, R2926–R2929. doi:10.1103/PhysRevB.53.R2926
Yan, Y., Feng, D., Zhu, J., Zhou, Q., Tian, F., Li, F., et al. (2023). Lattice and electronic structural evolutions in compressed multilayer MnPS3. J. Phys. Chem. C 127, 17186–17193. doi:10.1021/acs.jpcc.3c03501
Zhang, Q., Hwangbo, K., Wang, C., Jiang, Q., Chu, J.-H., Wen, H., et al. (2021). Observation of giant optical linear dichroism in a zigzag antiferromagnet FePS3. Nano Lett. 21, 6938–6945. doi:10.1021/acs.nanolett.1c02188
Zhang, X., Xu, Y., Halloran, T., Zhong, R., Broholm, C., Cava, R. J., et al. (2023). A magnetic continuum in the cobalt-based honeycomb magnet BaCo2(AsO4)2. Nat. Mater. 22, 58–63. doi:10.1038/s41563-022-01403-1
Zheng, Y., Jiang, X.-x., Xue, X.-x., Dai, J., and Feng, Y. (2019). Ab initio study of pressure-driven phase transition in FePS3 and FePSe3. Phys. Rev. B 100, 174102. doi:10.1103/PhysRevB.100.174102
Keywords: van der Waals solids, complex chalcogenides, external stimuli, antiferromagnetic ordering, insulator-metal transition, structural transition, magnetic transition, pressure
Citation: Matsuoka T, Kim H-S, Samanta S, Musfeldt JL and Mandrus DG (2024) MPX3 van der Waals magnets under pressure (M = Mn, Ni, V, Fe, Co, Cd; X = S, Se). Front. Mater. 11:1362744. doi: 10.3389/fmats.2024.1362744
Received: 28 December 2023; Accepted: 23 February 2024;
Published: 03 June 2024.
Edited by:
Leszek A. Majewski, The University of Manchester, United KingdomCopyright © 2024 Matsuoka, Kim, Samanta, Musfeldt and Mandrus. This is an open-access article distributed under the terms of the Creative Commons Attribution License (CC BY). The use, distribution or reproduction in other forums is permitted, provided the original author(s) and the copyright owner(s) are credited and that the original publication in this journal is cited, in accordance with accepted academic practice. No use, distribution or reproduction is permitted which does not comply with these terms.
*Correspondence: Takahiro Matsuoka, dG1hdHN1b2thQHVwLmVkdS5waA==; Heung-Sik Kim, aGV1bmdzaWtpbUBrYW5nd29uLmFjLmty; Janice L. Musfeldt, bXVzZmVsZHRAdXRrLmVkdQ==; David G. Mandrus, ZG1hbmRydXNAdXRrLmVkdQ==