- Department of Molecular Biology, University of Wyoming, Laramie, WY, United States
Water is essential for all active life processes. Despite this, there are a number of organisms that can survive prolonged desiccation. The vitrification hypothesis posits that such organisms survive desiccation by forming non-crystalline amorphous (vitrified) solids, often through the accumulation of protective disaccharides. In line with this theory, vitrification has been shown to be essential for desiccation tolerance in many organisms that survive extreme drying. However, it is known that not all vitrified materials are protective and that certain physio-chemical properties correlate with the protection in the glassy state. Furthermore, recent evidence suggests that the physio-chemical properties that correlate with protection can vary depending on the chemical nature of similarly sized protectants. While the chemistry of protectants has been probed in relation to the protective properties they induce when vitrified, the effect of protectant size on glassy properties and protection during drying has not been investigated. Here, we study the effect of the polymer size of sucrose on glassy properties associated with protection in the vitrified state. The monomer sucrose, and the polymers polysucrose 70 and polysucrose 400 (70 and 400 refer to the molecular weight of the polymers in kDa). Using these three different-sized sucrose polymers, we find that each of the glassy properties we investigated including; enzyme protection, water content, glass transition temperature, and glass former fragility, were affected by polymer size. However, only one vitrified property, glass transition temperature, correlated with protection during drying. This correlation is heavily dependent on sucrose polymer size. Increased glass transition midpoint temperature correlated positively with protection conferred by monomeric sucrose (p-value = 0.009, R2 = 0.840), whereas this correlation was bi-phasic for polysucrose 70, and had an inverse relationship for polysucrose 400 (p-value = 0.120, R2 = 0.490). Our results indicate that the size of vitrifying protectants can have a profound effect on glassy properties as well as on how these properties correlate with protection in the dry state. Beyond desiccation tolerance, these findings provide insights for the development of new technologies for the stabilization of biological material in the dry state.
Introduction
The vitrification hypothesis is one of the major hypotheses with regard to how desiccation-tolerant organisms survive extreme drying (Crowe et al., 1992; Crowe et al., 1998; Pan et al., 2008; Hibshman et al., 2020). This hypothesis posits that mediators of anhydrobiosis (Greek for “life without water”), such as trehalose and sucrose, work through the induction of highly viscous states which ultimately form non-crystalline, amorphous glass-like (vitrified) solids. The viscosity induced by these vitrifying mediators is thought to slow detrimental processes, such as protein unfolding and aggregation as well as membrane fusion (Crowe et al., 1997; Jain and Roy, 2009; Hesgrove and Boothby, 2020). Ultimately, the formation of non-crystalline solids is thought to be protective in that it limits crystallization, which if left unchecked has been shown to damage intracellular components and cellular superstructure (Aguilera and Karel, 1997; Han and Bischof, 2004). Through empirical studies, vitrification has been established as an essential mechanism for desiccation tolerance in a number of systems (Sun and Leopold, 1997; Crowe et al., 1998; Sakurai et al., 2008). However, while vitrification is considered essential, it is not sufficient for desiccation tolerance. This is illustrated by the simple logic that any sufficiently heterogeneous system, such as a typical non-desiccation tolerant cell, will vitrify when dried, but not all cells are anhydrobiotic (Crowe et al., 1998; Sakurai et al., 2008; Boothby et al., 2017; Boothby, 2021). This implies that there must be some property or properties that distinguishes protective from non-protective glasses.
Previous work has identified several properties of vitrified systems that are linked to their protective capacity during desiccation (Crowe et al., 1998; Ballesteros and Walters, 2019; Ramirez et al., 2024). The first of these is the glass transition temperature (Tg), the temperature at which a vitrified material transitions from a glass-like state to a more rubbery phase (Angell, 1995; Ito et al., 1999; Grasmeijer et al., 2013). Additionally, other studies have demonstrated that increasing the Tg (anti-plasticization) of many dry systems has a stabilizing effect, whereas decreasing Tg (plasticization) of a vitrified system in many situations has a negative impact on protection (Lim and Hoag, 2013; Mascia et al., 2020).
Glass former fragility, which was classically described by Angell as log viscosity as a function of the temperature as it approaches Tg, can more intuitively be thought of as how a material increases in viscosity as its temperature approaches the glass transition (Angell, 2002). With regard to desiccation, a strong glass former would increase in viscosity as water is lost and continue to do so to the point of vitrification. Conversely, a fragile glass former would not increase in viscosity dramatically in the initial stages of drying, but rather would increase in viscosity only close to the point at which it vitrifies (Angell, 1995; Ballesteros and Walters, 2019). Glass former fragility has been mostly studied in the context of cryogenic vitrification, but has been seen to correlate with protection in a limited number of studies involving dry vitrification (Walters, 2004; Ballesteros and Walters, 2019; Ramirez et al., 2024).
Water content is an additional property of a vitrified material that has been considered with regard to glassy properties that could influence protection (Ramirez et al., 2024). Water within a vitrified system has been shown to be protective in and of itself, providing hydrogen bonds that can stabilize proteins and maintain membrane organization (Wright, 1989; Hibshman et al., 2020). Water within a vitrified system can also influence other glassy properties, and is typically considered a plasticizer of vitrified systems, with the addition of water causing a decrease in Tg. Contrary to that, water has also been shown to have anti-plasticizing effects on some vitreous systems (Seow et al., 1999; Ruiz et al., 2017). Thus, while water can act to stabilize biological macromolecules as well as a plasticizer/anti-plasticizer of vitreous systems, its direct correlation with protection in the glassy state is mysterious.
In a recent report, the properties of vitrified systems that promote protection were investigated using a series of mixtures comprising one of three different disaccharides (trehalose, maltose, and sucrose) and increasing amounts of glycerol (Ramirez et al., 2024). It showed that the glassy property(s); glass former fragility, glass transition temperature, and water content associated with protection varied from disaccharide to disaccharide. Protection granted by mixtures containing maltose correlated strongly with increased water content compared to the other two properties, while the protection of glasses formed with sucrose best correlates with increased glass transition temperature and the protection conferred by trehalose glasses correlates with reduced glass former fragility. Thus, protective sugar-glasses made up of similarly sized components, but with distinct chemical properties, modulate distinct properties that correlate with protection. However, what has not been investigated is the effect of protectant size on glassy properties, and how these properties correlate with protection.
Here, we investigate this question using a series of mixtures composed of distinct sucrose species (monomeric, polysucrose 70, and polysucrose 400) and increasing glycerol content. Sucrose, a disaccharide made of glucose and fructose connected through the C1-C2 glycosidic linkage, is a naturally occurring sugar found in many desiccation-tolerant plants, and its contributions during vitrification are believed to be the reasons for cell survival during desiccation (Illing et al., 2005; Chemistry LibreTexts, 2023). Because of its protective properties, sucrose is commonly used in the food and pharmaceutical industry to stabilize food and drugs in the dry state (Beneke et al., 2009). Polysucroses are sucrose polymers formed by a highly branched structure by copolymerization of sucrose with epichlorohydrin (Jugdawa et al., 2017). In contrast to sucrose monomers, polysucroses are not found as naturally occurring excipients. Instead, polysucroses are commonly used in the biological field for purposes such as separation technology and to study glomerular physiology (Quach and Ferrante, 2017; Königshausen et al., 2019). Polysucrose 70 and polysucrose 400 were selected to provide an extended range of size difference relative to sucrose and one another. Polysucrose 400 is the largest commonly available polymer size of sucrose and thus was selected as an upper limit of polymer size. Polysucrose 70 was selected as an intermediate size between the monomer and polysucrose 400. The nomenclature polysucrose 70 and polysucrose 400 is used to indicate molecular weights of 70 kDa and 400 kDa respectively.
We find that the polymer size of sucrose affects each glassy property investigated here, however, only Tg correlated with protection. Interestingly, the correlation between Tg and protection varied between samples composed of different-sized sucrose species. Tg and protection had a positive correlation for mixtures formulated with monomeric sucrose, but this correlation was negative for mixtures composed of polysucrose 400, and bi-phasic for samples containing polysucrose 70.
These results provide insights into how polymer-size affects the properties and protection conferred by glassy systems.
Methodology
Disaccharide-glycerol mixtures
The three species of sucrose were commercially obtained: D-sucrose (342 g/mol) from Sigma-Aldrich (S0389-500G), Ficoll 70 (polysucrose 70; 70 kDa) and Ficoll 400 (polysucrose 400; 400 kDa) from Sigma-Aldrich (SC-257529A and 26873-85-8 respectively). Glycerol was sourced from Biobasic (GB0232).
To prepare mixtures a 50 mL stock solution of 0.25 mM polysucrose 400 was made. Polysucrose 70 and monomeric sucrose solutions were made in reference to the polysucrose 400 solution such that each solution contained the same number of sucrose monomers.
From these stock solutions, a series of solutions were prepared via the addition of glycerol. Sucrose content was varied from 100.0% to 87.5% by adding glycerol in weight by weight ratio (sugar 100.0%, sugar 97.5%+glycerol 2.5%, sugar 95.0%+glycerol 5.0%, sugar 92.5%+glycerol 7.5% sugar 90.0%+glycerol 10.0%, and sugar 87.5%+glycerol 12.5%) (Supplementary Table S1).
Sample desiccation
2 mL from each aqueous sample was dispensed into individual plastic weigh boats and desiccated using a speedvac (Savant SpeedVac SC110 with a Thermo OFP400 vacuum pump) for 16 h. Each experiment was performed with triplicate. After the 16 h of desiccation, known weights of dried samples were loaded to pre-massed differential scanning calorimetry (DSC) aluminum hermetic pan (TA 900793.901) and aluminum hermetic lids (901684.901) while dried samples were loaded to pre-tared thermogravimetric analysis (TGA) platinum crucibles (TA 957207.904). Glass transition temperature (Tg) and glass former fragility (m index) were derived from DSC thermograms (Supplementary Figure S1) and percentage of remaining water content was calculated using TGA (Supplementary Figure S2).
Calculation of glass transition temperature
Dried samples (3–4 mg) were placed in pre-weighed DSC pans and loaded in the DSC machine. The glassy state of sugars was obtained experimentally via quench-cooling. The sample was heated up from 30°C to 200°C at a rate of 10°C/min and then cooled to 0°C immediately. Subsequently, the sample was heated again by raising the temperature to 250°C at a rate of 10°C/min. Tg for each of the three sugar species was obtained as the midpoint of the onset and offset (endset) temperatures using Trios software. Average midpoint values were taken from the triplicates from each type of sucrose-glycerol mixtures.
Calculation of water content in vitrified samples
Samples were run on a TGA (TGA5500 instrument) in 100 μL platinum crucibles (TA 952018.906). Crucibles were tared prior to each run and prior to loading the samples. Then the analysis was performed to determine a material’s thermal stability and its fraction of volatile components by monitoring the weight changes along with the temperature. The dried samples (10–11 mg) were placed in TGA plates and loaded in the TGA machine. The program was started at 30°C and then heated to 250°C at 10°C/min rate. Water loss from each sample of three species of sugars was calculated in order to determine the remaining percentage of water content in the dried samples. Thermograms were used to calculate mass differences that occur after ∼100°C but before the thermal denaturation at ∼180°C. The Trios software “Smart Analysis” tool was used to identify the inflection point between mass loss events occurring from starting masses of samples and the mass of samples at the plateau. The average values were taken from the triplicates from each species of sucrose-glycerol mixtures.
Lactate dehydrogenase (LDH) enzyme protection assay
LDH assays were performed according to the methodology described in Boothby et al., 2017. The stock solutions of 25 mM Tris HCl (pH 7.0), 100 mM sodium phosphate (pH 6.0), 2 mM pyruvate, and 10 mM NADH were prepared in bulk and stored at 4°C prior to the experiment. L-Lactate Dehydrogenase (LDH) was received from Sigma (SKU #10127230001). Prior to assay, LDH was diluted to a working concentration of 1 g/L using a pre-prepared stock solution of 25 mM Tris HCl (pH 7.0). Experimental sugar-glycerol mixtures were formulated with LDH at a 1:10 (LDH:sugar-glycerol) ratio. For all samples, 90 μL from each sample was added with 10 μL of LDH into a 1.5 mL microcentrifuge tube and a total volume of 100 μL was split in half as a control and an experimental set. The experimental set was kept in the vacuum desiccator and the control set was kept in the refrigerator (4°C) for 16 h. Experiment was performed with triplicates from each sample for each of the three different species of sucrose. After vacuum desiccation, control and experimental excipient mixtures were rehydrated using molecular grade water and brought to 250 μL total volume. A nanodrop instrument using quartz cuvettes was first blanked against the 980 μL of 100 mM sodium phosphate and 2 mM pyruvate solution. The nanodrop instrument was used to take absorbance readings every 2 seconds at the 340 nm wavelength (A340) for 60 s. Absorbance reading was taken by 10 μL of rehydrated control mixture combined with 10 μL of NADH in 980 μL of 100 mM sodium phosphate and 2 mM pyruvate solution. Then the absorbance reading was taken for the experimental mixture using the same procedure as the controls. A ratio of experimental over control, multiplied by one hundred, will produce the percent protection of the experimental mixture. Likewise percent protection was calculated for each mixture of three species of sucrose. The average protection percentages were taken from the triplicates from each type of sucrose-glycerol mixtures.
Fragility (m index) calculation
Trios software (TA Instruments TRIOS version #5.0.0.44608) was used to perform analysis of onset and endset points in order to calculate the glass former fragility (m index). Calculations of the glass m index were performed based on equations 10 and 14) proposed by Crowley and Zografi (Crowley and Zografi, 2001). On a thermogram with a completed heating ramp to 250°C, the degradation peak, melt peak, and glass transition were identified. The Trios software built-in onset and endset analysis was used to determine the glass transition onset and endset and m index was calculated using Crowley and Zografi’s equations 10 where m is the alternative fragility parameter, ΔETg is the activation enthalpy of structural relaxation at Tg, R is the gas constant, Tg is the experimental glass transition temperature onset, and equation 14 where ΔE𝜂 is the activation enthalpy for viscosity, R is the gas constant, Tg is the experimental glass transition onset temperature, Tgoff is the experimental glass transition endset temperature, and constant is an empirical constant of 5 (Crowley and Zografi, 2001). A mean of each set of replicates was obtained.
Statistical methods
One-Way ANOVA was utilized to compare the means in independent groups of sucrose- and polysucrose-glycerol mixtures in order to determine whether there is statistical difference between one or more mixtures. The Tukey post hoc test was used to assess the significance of differences between pairs of individual group means. Rstudio (R 4.2.2) and the package ‘ggpubr’ was used to calculate the One-Way ANOVA, Tukey post hoc test, p-value, and R2 results. A p-value of less than 0.05 being one level of statistical significance (*), less than 0.01 being two levels of statistical significance (**), and less than 0.001 being three levels of statistical significance (***). All error values in bar graphs represent one standard error (SE) and 95% confidence interval (CI) levels in correlation graphs. Regression analysis was performed using the simple linear model for monomeric sucrose and polysucrose 400 correlations. Since in each case examining polysucrose 70 produced a bi-phasic correlation, and for such relationships fitting data is less exacting and accurate, we opted not to fit these data.
Results
Disaccharide-glycerol mixtures have varying levels of protection during desiccation
To begin to address the question of how protectant size influences glassy properties and protection, we generated a series of mixtures, each utilizing one of three sucrose species; monomeric sucrose, polysucrose 70, and polysucrose 400, and increasing amounts of glycerol (0.0, 2.5, 5.0, 7.5, 10.0, and 12.5% glycerol in weight by weight ratio) (Supplementary Table S1). Samples were prepared such that the total number of sucrose monomers in each sample was 5 × 10−3 mol in 50 mL. We next assessed the ability of each of these 18 mixtures to preserve the function of the labile enzyme lactate dehydrogenase (LDH) during desiccation (Figures 1A, B). Previous reports have shown that LDH is sensitive to desiccation and that drying and rehydration of this enzyme results in loss in functionality. This functionality can be preserved by coincubation with protective vitrifying agents, including sucrose, prior to drying (Goyal et al., 2005).
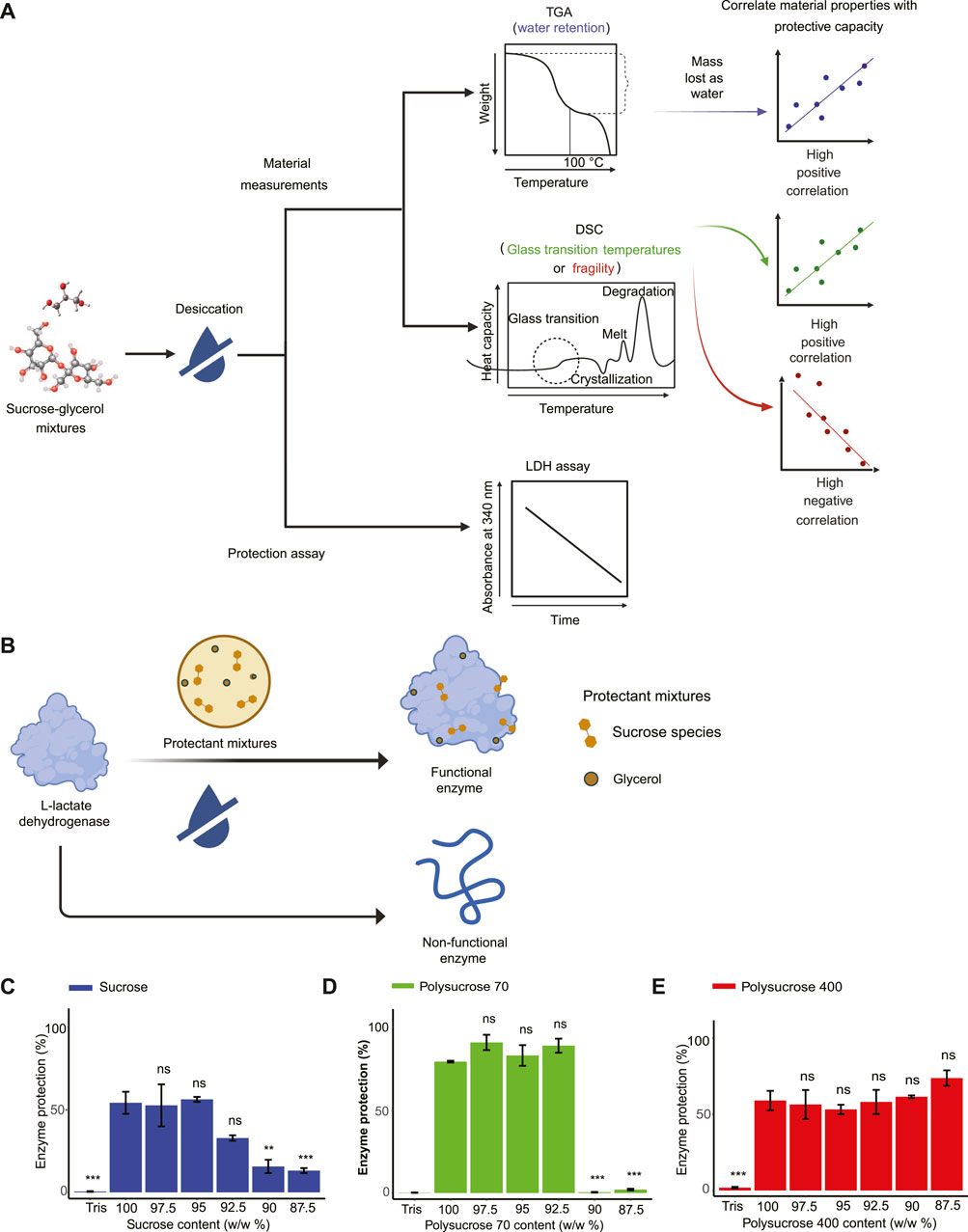
FIGURE 1. Vitrified sucrose-glycerol mixtures display distinct enzyme-protective properties. (A) Schematic of workflow to produce correlation values between material properties and enzyme protection capacity. (B) Schematic of Lactate dehydrogenase (LDH) enzyme assay. (C) LDH enzyme protection values for monomeric sucrose-glycerol mixtures. (D) LDH enzyme protection values for polysucrose 70-glycerol mixtures. (E) LDH enzyme protection values for polysucrose 400-glycerol mixtures. Statistics calculated using a one-way ANOVA and Tukey post hoc test: p-value ≤0.05 (*) p-value ≤0.01 (**), p-value ≤0.001 (***), pairwise comparison are shown only from 100% sucrose to each mixture, error bars represent 1*standard error in barcharts. Statistics on all other pairwise comparisons are provided in Supplementary Table S3.
Pure monomeric sucrose provided 54.243% protection to LDH (Figure 1C). A decrease in protection in monomeric sucrose mixtures was observed with the addition of 7.5% glycerol, though this was not significant, and with each step-wise addition beyond that, significant decreases in protection were observed relative to pure monomer sucrose (Figure 1C). Pure polysucrose 70, which provides the same number of sucrose monomers (5 × 10−3 mol/50 mL) as our pure sucrose monomer sample, provided 79.883% protection to LDH during drying. For mixtures containing polysucrose 70, protection did not decrease with additions of glycerol up to 7.5%, after which there was a dramatic drop-off in protection (Figure 1D). It was noted that the polysucrose 70 biphasic result was different from the sucrose and polysucrose 400 linear results. As a result, we have repeated these assays multiple times (3 technical replicates and 3 biological replicates) on different days. In all cases we obtained the similar results. Thus, given our experience with the assay and our repeated and replicable trials with polysucrose 70 we concluded that while the result is surprising but none-the-less real. For pure polysucrose 400, which provides the same number of sucrose molecules as our pure monomer sample and our polysucrose sample, provided 59.037% protection to LDH subjected to desiccation. Mixtures containing polysucrose 400 differed further from mixtures containing monomeric sucrose or polysucrose 70, in that protection was not affected by the addition of glycerol at any levels used in this study (Figure 1E). Apart from that, there was an increase of protection with addition of 10.0% and 12.5% glycerol to the mixture, but this was not statistically significant relative to pure polysucrose 400 (Figure 1E).
These data demonstrate that the polymer size of sucrose affects its protective capacity, with pure polysucrose 70 providing more protection to LDH than mixtures of pure monomeric sucrose or polysucrose 400 that are composed of the same number of sucrose monomers. Furthermore, these results indicate additions of glycerol affect the protective capacity of different sized sucrose polymers in distinct ways.
Water content varies between glasses composed of different sucrose species, but does not correlate with the protective capacity
After observing that the protective capacity of sucrose in different polymerized states differs during drying, we were curious if there are any glassy properties of these sugars that correlate with their ability to provide protection from desiccation. To begin to assess the correlation between properties of vitrified systems composed of different sized sucrose species and the protection, we first assessed whether the water content could account for these differences or not.
In the field of desiccation tolerance, it has been suggested that desiccation tolerant organisms may be able to retain more water than their desiccation sensitive organisms (Holzinger and Karsten, 2013; Hibshman et al., 2020). A higher amount of water could help increase the proportion of proteome, membranes, and other vital cellular components that remain solvated within the system. Conversely, retaining too much water in a dry/drying state might also be deleterious, by allowing for reduced viscosity and increased alpha- and/or beta-relaxations (Fan and Roos, 2016). Thus, desiccation tolerant organisms likely need to precisely tune the amount of water retained within their cells to maintain optimal viability. Therefore, water content could have an important impact on in vitro stabilization of biological material in a dry state.
To assess whether there are differences in water retention in vitrified systems composed of different sucrose species and whether or not these differences correlate with differences in protection, we tested each of our mixtures using thermogravimetric analysis (TGA) (Figure 1A). TGA produced thermograms with two distinct areas of sample mass loss, the first centered around 100°C and the second around 200°C (Supplementary Figure S2). These mass losses correspond to the evaporation of water from our samples and the degradation of the sample at high temperature, respectively (Hurtta et al., 2004). The water contents of our 18 dry mixtures ranged from 3.141% to 12.172% (Figures 2A–C). Pure dry monomeric sucrose contained 5.636% retained water, while pure dry polysucrose 70 and 400 showed significantly higher water content (12.172% and 7.585%, respectively) (Figures 2A–D; Supplementary Table S2). The water content of the dried sucrose monomer mixtures did not change significantly with the addition of glycerol into the mixtures (Figure 2A). For polysucrose 70, water content significantly decreases upon addition of 12.5% glycerol (Figure 2B). Polysucrose 400 displayed a non-monotonic response to glycerol addition, with an initial decrease, followed by an increase, a decrease, and then a further increase (Figure 2C).
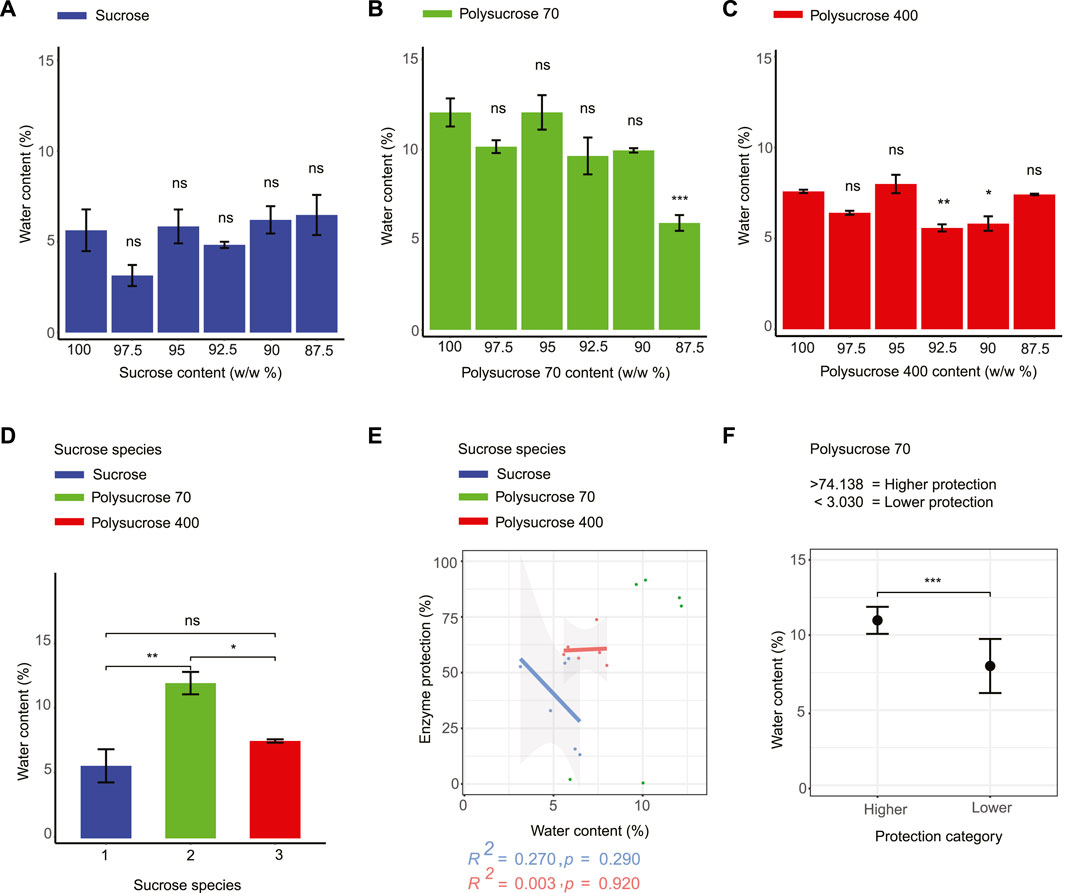
FIGURE 2. Water content does not correlate with the enzyme-protective capacity of any sucrose-glycerol glasses while water content strongly correlates with Tg. (A) Water content values for monomeric sucrose-glycerol mixtures. (B) Water content values for polysucrose 70-glycerol mixtures. (C) Water content values for polysucrose 400-glycerol mixtures. (D) Water content values for all types of pure sucrose monomers and polymers. (E) Correlation plot of water content versus enzyme protection for all sugar-glycerol mixtures. (F) Average value of bi-phasic pattern of higher and lower protection level of polysucrose 70-glycerol mixtures and average water content and error bars represent 95% confidence intervals. Statistics calculated using a one-way ANOVA and Tukey post hoc test: p-value ≤0.05 (*) p-value ≤0.01 (**), p-value ≤0.001 (***), pairwise comparison are shown only from 100% sucrose to each mixture, error bars represent 1*standard error in barcharts and 95% confidence intervals in scatterplots. Statistics on all other pairwise comparisons are provided in Supplementary Table S3.
After observing distinct water retentive behaviors in glasses composed of different sucrose species, we assessed the relationship between water content and LDH enzyme protection. For monomeric sucrose mixtures we observed a negative, albeit statistically insignificant, relationship between protection and water content (Figure 2E). This differed from our series of mixtures composed with polysucrose 70, which showed a statistically significant bi-phasic relationship between water retention and protection, with samples at low average water contents (7.940%) being the least protective and samples with the most retained water (10.979%) conferring the most protection (Figure 2F). Finally, mixtures containing polysucrose 400 differed further, displaying a slightly positive, but statistically insignificant, trend between retained water and protection (Figure 2E).
Taken together these data demonstrate that water is retained in vitrified sucrose samples differently depending on the polymer state of sucrose. However, these data indicate that retained water is not a strong predictor of protection for vitrified sucrose at any size of the species tested.
Glass transition temperature correlates with the protective capacity of sucrose species-glycerol mixtures in distinct ways
After observing that water retention is a poor indicator of the protective capacity of sucrose species-glycerol glasses, we were curious if glass transition temperature (Tg) might be a good predictor. Tg is the temperature at which a glassy material will begin to transition into a more rubbery phase. Previous work has established that even small amounts of additives, such as glycerol, can lead to changes in the Tg of a vitrifying material, which can correlate with changes to protective capacity (Weng and Elliott, 2015; Ramirez et al., 2024). With this in mind, we were curious if the Tg differs between sucrose-glycerol mixtures composed of different sized sucrose species. Furthermore, we were curious if Tg, which has previously been observed to be a good predictor of protection conferred by sucrose, is also a good predictor of protection conferred by sucrose species (Ramirez et al., 2024). To this end, we performed differential scanning calorimetry (DSC) on dried sucrose species-glycerol mixtures to obtain Tg onset, midpoint, and endset values (Supplementary Figure S1).
The Tg of pure sucrose was observed to be significantly lower than that of pure polysucrose 70 and polysucrose 400. For example, the midpoint Tg for monomeric sucrose was measured to be 73.310°C, while the Tg of polysucrose 70 and 400 were 126.603°C and 130.205°C, respectively (Figures 3A–C; Supplementary Table S2). The addition of glycerol to all three sucrose species tested had a similar plasticizing effect, however the range of observed plasticization varied (Figures 3A–C). For example, addition of glycerol decreased the Tg of monomeric sucrose from 73.310°C (pure) to 40.907°C (87.5%), a range of 32.403°C (Figure 3A; Supplementary Table S2). This same range for polysucrose 70 and 400 was 64.183°C and 69.282°C, respectively (Figures 3B, C; Supplementary Table S2).
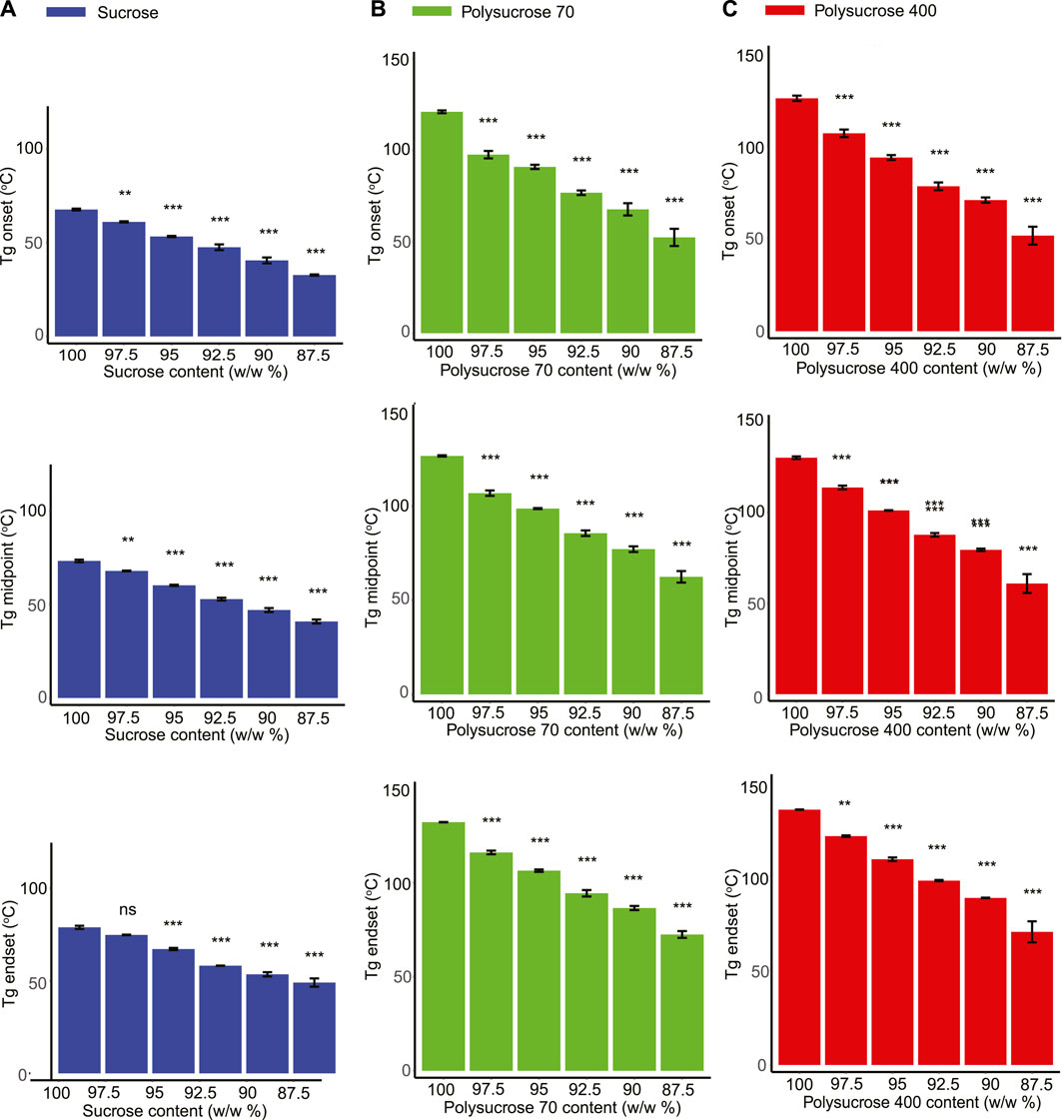
FIGURE 3. Glycerol shows a plasticization effect in all three species of sucrose. (A) Glass transition onset, midpoint and endset values for monomeric sucrose-glycerol mixtures. (B) Glass transition onset, midpoint and endset values for polysucrose 70-glycerol mixtures. (C) Glass transition onset, midpoint and endset values for polysucrose 400-glycerol mixtures. Statistics calculated using a one-way ANOVA and Tukey post hoc test: p-value ≤0.05 (*) p-value ≤0.01 (**), p-value ≤0.001 (***), pairwise comparison are shown only from 100.0% sucrose to each mixture, error bars represent 1*standard error. Statistics on all other pairwise comparisons are provided in Supplementary Table S3.
Next, we evaluated the relationship between protective capacity and the Tg onset, midpoint, and endset for each mixture (Figures 4A–C). Regardless of whether onset, midpoint, or endset was considered, the same trends were observed (Figures 4A–C). For monomeric sucrose there was a statistically significant, strong positive correlation between protection and Tg. This is in stark contrast to polysucrose 400, which showed a statistically insignificant negative correlation between protection and Tg. For polysucrose 70, we observed a statistically significant bi-phasic relationship between protection and Tg, where mixtures with a Tg average onset lower than 60.010°C, Tg average midpoint lower than 69.771°C, and Tg average endset lower than 79.532°C provided essentially no protection, but for mixtures with a Tg average onset higher than 97.052°C, Tg average midpoint higher than 104.942°C and Tg average endset higher than 112.834°C we observed the highest levels of protection (Figures 4D–F).
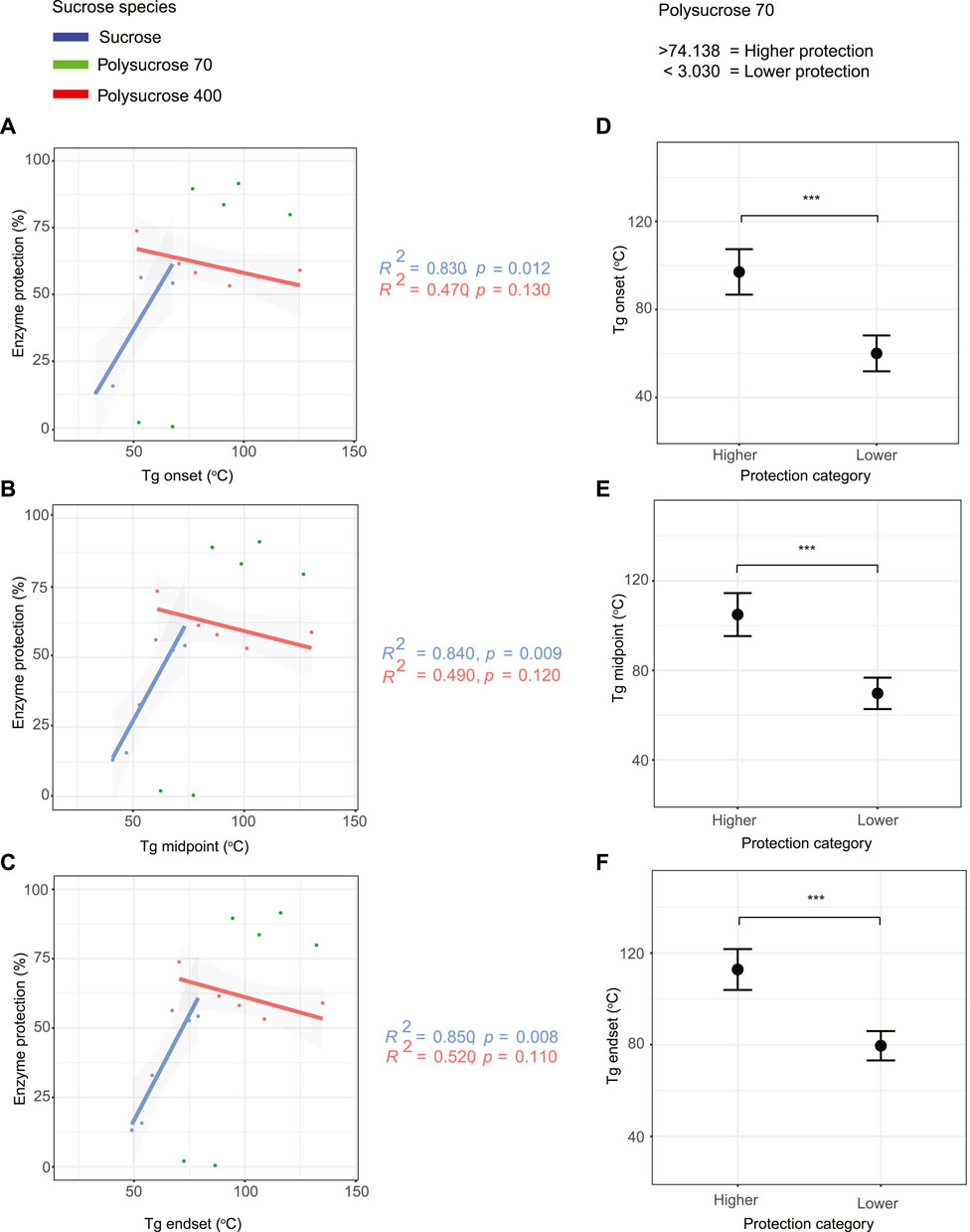
FIGURE 4. Monomeric sucrose strongly correlates with the glass transition temperature (Tg). (A) Correlation plot of glass transition onset values versus protection for all sucrose-glycerol mixtures. (B) Correlation plot of glass transition midpoint values versus protection for all sucrose-glycerol mixtures. (C) Correlation plot of glass transition endset values versus protection for all sucrose-glycerol mixtures. (D) Average value of bi-phasic pattern of higher and lower protection level of polysucrose 70-glycerol mixtures and average glass transition onset. (E) Average value of bi-phasic pattern of higher and lower protection level of polysucrose 70-glycerol mixtures and average glass transition midpoint. (F) Average value of bi-phasic pattern of higher and lower protection level of polysucrose 70-glycerol mixtures and average glass transition endset. Error bars represent 95% confidence intervals.
Taken together, these results indicate that the size of the sucrose species influences the Tg of mixtures, with monomeric sucrose transitioning at much lower temperature than either polysucrose 70 or 400. Furthermore, while all size species of sucrose were observed to plasticize with the addition of glycerol, monomeric sucrose plasticized over a much smaller range than either polymer. Finally, and most strikingly, there were correlations between Tg and protection. However, protection provided by sucrose in different polymerized states correlated with Tg in dramatically distinct ways (e.g., positive versus negative correlations).
Glass former fragility of sucrose-glycerol mixtures does not correlate with enzyme protection
Since changes to Tg can influence the glass former fragility (m index) of a material, we were interested in whether glass former fragility also differed between our samples as well as if there is a correlation between glass former fragility and protection during drying for mixtures composed of different sized sucrose species. For pure samples lacking glycerol we observed an increase in glass former fragility as the size of sucrose species increased (Figures 5A–C). For each of our three species of sucrose, we observed a decrease in glass former fragility with the addition of 2.5% glycerol, which was statistically insignificant for monomeric sucrose, but statistically significant for both polymers (Figures 5A–C). With the rest of the additions of glycerol (up to 12.5%) there were mild decreases in glass former fragility observed in polysucrose 400, but these were slight and almost always statistically insignificant. Monomeric sucrose and polysucrose 70 however, showed non-monotonic behavior regardless of size of the sucrose species (Figures 5A–C).
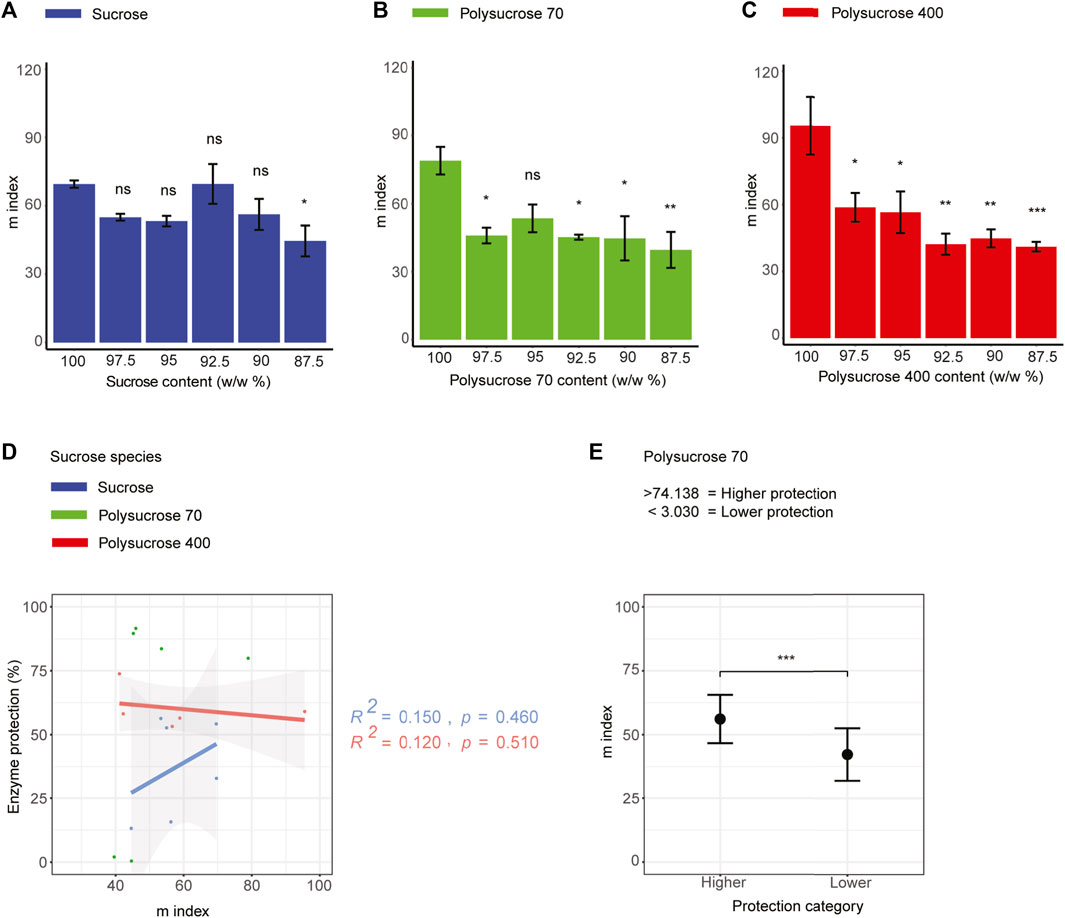
FIGURE 5. Glass forming fragility does not correlate with enzyme protection in all three types of sucrose-glycerol mixtures. (A) Glass former fragility (m index) values for monomeric sucrose-glycerol mixtures. (B) Glass former fragility (m index) values for polysucrose 70-glycerol mixtures. (C) Glass former fragility (m-index) values for polysucrose 400-glycerol mixtures. (D) Correlation plot of glass former fragility (m index) versus protection for all sucrose-glycerol mixtures. (E) Average value of bi-phasic pattern of higher and lower protection level of polysucrose 70-glycerol mixtures and average m index. Statistics calculated using a one-way ANOVA and Tukey post hoc test: p-value ≤0.05 (*) p-value ≤0.01 (**), p-value ≤0.001 (***), pairwise comparison are shown only from 100.0 sucrose to each mixture, error bars represent 1*standard error in barcharts and 95% confidence intervals in scatterplots. Statistics on all other pairwise comparisons are provided in Supplementary Table S3.
Next, we assessed the relationship between the glass former fragility of our mixtures and protection of LDH. This correlative analysis produced only statistically insignificant trends (Figure 5D), indicating that glass former fragility is not a strong predictor of protection. Polysucrose 70, which still showed a statistically significant bi-phasic relationship between glass former fragility and protection, with samples with low average m index (42.142) being the least protective and samples with higher average m index value (56.126) conferring the most protection (Figure 5E).
Taken together these results suggest that glass former fragility is not a mechanistic driver of desiccation protection conferred by sucrose, since the glass former fragility of pure monomeric sucrose is significantly lower than that of pure polysucrose 70 and 400, but monomeric sucrose does not offer more protection than polysucrose 70 or 400 (Figures 1C–E). Furthermore, the addition of glycerol decreased glass former fragility in all cases, but again, there was no strong trend between this decrease in fragility and augmented protection.
Discussion
Anhydrobiotic organisms survive during near complete water loss through different physiological and behavioral adaptations against the extreme environmental conditions (Watanabe, 2006; Kaczmarek et al., 2019). The vitrification hypothesis, a central hypothesis in the desiccation tolerance field, posits that the accumulation of sugars and other mediators of desiccation tolerance induce highly viscous states which ultimately form into amorphous solids during dehydration in order to protect the cells via the reduction of molecular motion and relaxations. While vitrification is considered to be essential for living systems to survive during desiccation, the field has known for decades that this is not sufficient for survival as virtually all sufficiently heterogeneous systems that are not desiccation tolerant will vitrify. A mixture that is not sufficiently heterogeneous can undergo crystallization rather than become vitrified (Kuwayama et al., 2015). It is known that crystallinity within a vitrified material can influence its protective capacity (Cardona et al., 1997; Hoekstra et al., 2001). A previous study demonstrated that similarly sized sugars dried in an identical fashion result in a similar lack of microcrystallinity within the glasses they form (Ramirez et al., 2024). In this study the effect of polymer size on sugar glass microcrystallinity has not been probed but will be an interesting parameter to assess in the future. In general, it would be expected that polymers of different sizes would potentially create differing degrees of microcrystalline, which we would hypothesize would be detrimental to protection.
Much effort has been spent in attempting to identify properties that distinguish protective from non-protective vitrifying systems. Chief among these properties are Tg, glass former fragility, and water content (Crowley and Zografi, 2001; Truong et al., 2004). While at the organismal level, both an increase in Tg and a decrease in glass former fragility are commonly observed in desiccation tolerant versus sensitive life stages of anhydrobiotic organisms. However, in vitro disaccharides of similar size, but with distinct chemical properties confer protection which correlates with either Tg or glass former fragility (Ramirez et al., 2024). However, when considering how the physics and material properties of a vitrified system are augmented, it is important to consider not just the chemical properties of the system’s constituents, but also their size.
Here we have used a series of vitrifying mixtures composed of three different sizes of sucrose species (monomeric, polysucrose 70, and polysucrose 400). We see that with regard to enzyme protection, water content, Tg, and glass former fragility, the size of sucrose has an influence on all three of these properties. However, consistent with previous findings (Ramirez et al., 2024), only Tg correlated with protection in the monomeric sucrose system (Ramirez et al., 2024). Importantly, we find that while the correlation between the Tg of mixtures composed of monomeric sucrose had a positive correlation with protection, this relationship was bi-phasic for polysucrose 70, and there was an inverse or negative correlation between Tg and protection conferred by mixtures containing polysucrose 400.
Water plays critical roles in all active life processes as well as stabilizing diverse biological macromolecules. The optimal water content for stabilizing different biological macromolecules remains to be determined, but one could envision that retaining too little water would be detrimental in that this would limit the number of stabilizing hydrogen bonds that could be made with a protein or other cellular materials. However, there might also be a penalty for retaining too much water, where excess residual water could be insufficient to fully stabilize a protein, while still allowing for some degree of molecular motion (alpha- and beta-relaxations) which could promote protein unfolding and/or aggregation.
While water content did not correlate with protection for any of our three sucrose species, the total amount of retained water did vary, with polysucrose 70 retaining the most water, followed by polysucrose 400, and finally monomeric sucrose. Our results therefore support the hypothesis that size of sucrose species influences water retention, but do not support the notion that water content is a property strongly predictive of sucrose-mediated protection during drying. These results do not rule out the possibility that water content influenced by species of sucrose-size might correlate with protection for other vitrifying mediators of desiccation tolerance.
Beyond stabilizing biological macromolecules, water is known to act as a plasticizing agent for many vitrified systems. However, here we have observed that water levels are positively correlated with the Tg of polysucrose 70 mixtures, but not with the Tg of mixtures composed of monomeric sucrose or polysucrose 400 (Supplementary Figure S3). This indicates an anti-plasticization effect of water on the polysucrose 70 system, which is rarely observed.
Interestingly, there were correlations between Tg and LDH protection for our sucrose-glycerol mixtures. The surprising result here is that the correlations were different between each size species of sucrose, with the Tg of monomeric sucrose correlating positively with protection, being bi-phasic for polysucrose 70, and being negatively correlated for polysucrose 400.
In general, an increase in cross-linking decreases the mobility of the polymer and the free volume leading to an increase in Tg. However, here increasing the Tg of a glassy system composed of monomers enhances protection, while increasing the Tg of a vitrified system composed of larger polymers (polysucrose 400) decreases protection. This might be explained by the higher water content in polysucrose 70 and polysucrose 400 compared to monomeric sucrose.
Previous work had established that there is no correlation between glass former fragility and protections conferred by monomeric sucrose. However, the effect of polymer size on glass former fragility and the correlation of glass former fragility and protection for sucrose species has not been investigated. We observe differences in glass former fragility between samples of pure monomeric sucrose, polysucrose 70, and polysucrose 400. However, there are no clear and significant trends with regard to protection and glass former fragility among any of our series of mixtures.
Overall, our study provides insights into the influence of size of sucrose species on protection, water retention, Tg, and glass former fragility. Size of the sucrose influenced all these properties, but in terms of importance in predicting protection during drying only Tg was found to be significant in sucrose monomer. The fact that the type of correlation (e.g., positive versus negative correlation) that Tg and protection had for mixtures composed of different sized sucrose species adds additional evidence to our conclusions that size of the sucrose species influences glassy properties and that at least some of these properties are important for conferring protection.
These results provide insights into how organisms may help to stabilize their cells and sensitive cellular components during desiccation. Beyond desiccation, understanding how vitrification properties can be tuned to enhance protection has important implications in the biomedical and pharmaceutical field for storage of sensitive biologics without the need for the cold-chain.
Data availability statement
The original contributions presented in the study are included in the article/Supplementary Material, further inquiries can be directed to the corresponding author.
Author contributions
UK: Data curation, Formal Analysis, Investigation, Methodology, Visualization, Writing–original draft, Writing–review and editing. JR: Conceptualization, Writing–original draft, Writing–review and editing, Formal Analysis, Investigation, Methodology. TB: Conceptualization, Writing–original draft, Writing–review and editing, Funding acquisition, Supervision, Project administration.
Funding
The author(s) declare financial support was received for the research, authorship, and/or publication of this article. This work was supported by the Water and Life Integration Institute (WALII) which is supported by NSF award #2213983. This work was partially funded through a fellowship to JFR administered by Wyoming NASA EPSCoR (NASA Grant #80NSSC19M0061). UGVSSK and JFR are supported by the USDA National Institute of Food and Agriculture, Hatch project #1012152.
Acknowledgments
The authors are thankful to all the members of the TB Lab for discussions and reading of this manuscript. The authors gratefully acknowledge financial support from the Wyoming Institutional Development Award (IDeA) from the National Institute of General Medical Sciences of the National institute of Health (Grant #2P20GM103432).
Conflict of interest
The authors declare that the research was conducted in the absence of any commercial or financial relationships that could be construed as a potential conflict of interest.
Publisher’s note
All claims expressed in this article are solely those of the authors and do not necessarily represent those of their affiliated organizations, or those of the publisher, the editors and the reviewers. Any product that may be evaluated in this article, or claim that may be made by its manufacturer, is not guaranteed or endorsed by the publisher.
Supplementary material
The Supplementary Material for this article can be found online at: https://www.frontiersin.org/articles/10.3389/fmats.2024.1351671/full#supplementary-material
References
Aguilera, J. M., and Karel, M. (1997). Preservation of biological materials under desiccation. Crit. Rev. Food Sci. Nutr. 37, 287–309. doi:10.1080/10408399709527776
Angell, C. A. (1995). The old problems of glass and the glass transition, and the many new twists. Proc. Natl. Acad. Sci. U. S. A. 92, 6675–6682. doi:10.1073/pnas.92.15.6675
Angell, C. A. (2002). Liquid fragility and the glass transition in water and aqueous solutions. Chem. Rev. 102, 2627–2650. doi:10.1021/cr000689q
Ballesteros, D., and Walters, C. (2019). Solid-state biology and seed longevity: a mechanical analysis of glasses in pea and soybean embryonic axes. Front. Plant Sci. 10, 920. doi:10.3389/fpls.2019.00920
Beneke, C. E., Viljoen, A. M., and Hamman, J. H. (2009). Polymeric plant-derived excipients in drug delivery. Molecules 14, 2602–2620. doi:10.3390/molecules14072602
Boothby, T. C. (2021). Water content influences the vitrified properties of CAHS proteins. Mol. Cell 81, 411–413. doi:10.1016/j.molcel.2020.12.009
Boothby, T. C., Tapia, H., Brozena, A. H., Piszkiewicz, S., Smith, A. E., Giovannini, I., et al. (2017). Tardigrades use intrinsically disordered proteins to survive desiccation. Mol. Cell 65, 975–984.e5. doi:10.1016/j.molcel.2017.02.018
Cardona, S., Schebor, C., Buera, M. P., Karel, M., and Chirife, J. (1997). Thermal stability of invertase in reduced-moisture amorphous matrices in relation to glassy state and trehalose crystallization. J. Food Sci. 62, 105–112. doi:10.1111/j.1365-2621.1997.tb04378.x
Chemistry LibreTexts (2023). 24.8: disaccharides and glycosidic bonds. Available at : https://chem.libretexts.org/Bookshelves/Organic_Chemistry/Map%3A_Organic_Chemistry_(Wade)_Complete_and_Semesters_I_and_II/Map%3A_Organic_Chemistry_(Wade)/24%3A_Carbohydrates/24.08%3A_Disaccharides_and_Glycosidic_Bonds.
Crowe, J. H., Carpenter, J. F., and Crowe, L. M. (1998). The role of vitrification in anhydrobiosis. Annu. Rev. Physiol. 60, 73–103. doi:10.1146/annurev.physiol.60.1.73
Crowe, J. H., Hoekstra, F. A., and Crowe, L. M. (1992). Anhydrobiosis. Annu. Rev. Physiol. 54, 579–599. doi:10.1146/annurev.ph.54.030192.003051
Crowe, J. H., Oliver, A. E., Hoekstra, F. A., and Crowe, L. M. (1997). Stabilization of dry membranes by mixtures of hydroxyethyl starch and glucose: the role of vitrification. Cryobiology 35, 20–30. doi:10.1006/cryo.1997.2020
Crowley, K. J., and Zografi, G. (2001). The use of thermal methods for predicting glass-former fragility. Thermochim. Acta 380, 79–93. doi:10.1016/s0040-6031(01)00662-1
Fan, F., and Roos, Y. H. (2016). Crystallization and structural relaxation times in structural strength analysis of amorphous sugar/whey protein systems. Food Hydrocoll. 60, 85–97. doi:10.1016/j.foodhyd.2016.03.019
Goyal, K., Walton, L. J., and Tunnacliffe, A. (2005). LEA proteins prevent protein aggregation due to water stress. Biochem. J. 388, 151–157. doi:10.1042/bj20041931
Grasmeijer, N., Stankovic, M., de Waard, H., Frijlink, H. W., and Hinrichs, W. L. J. (2013). Unraveling protein stabilization mechanisms: vitrification and water replacement in a glass transition temperature controlled system. Biochim. Biophys. Acta 1834, 763–769. doi:10.1016/j.bbapap.2013.01.020
Han, B., and Bischof, J. C. (2004). Direct cell injury associated with eutectic crystallization during freezing. Cryobiology 48, 8–21. doi:10.1016/j.cryobiol.2003.11.002
Hesgrove, C., and Boothby, T. C. (2020). The biology of tardigrade disordered proteins in extreme stress tolerance. Cell Commun. Signal 18, 178. doi:10.1186/s12964-020-00670-2
Hibshman, J. D., Clegg, J. S., and Goldstein, B. (2020). Mechanisms of desiccation tolerance: themes and variations in brine shrimp, roundworms, and tardigrades. Front. Physiol. 11, 592016. doi:10.3389/fphys.2020.592016
Hoekstra, F. A., Golovina, E. A., and Buitink, J. (2001). Mechanisms of plant desiccation tolerance. Trends Plant Sci. 6, 431–438. doi:10.1016/s1360-1385(01)02052-0
Holzinger, A., and Karsten, U. (2013). Desiccation stress and tolerance in green algae: consequences for ultrastructure, physiological and molecular mechanisms. Front. Plant Sci. 4, 327. doi:10.3389/fpls.2013.00327
Hurtta, M., Pitkänen, I., and Knuutinen, J. (2004). Melting behaviour of D-sucrose, D-glucose and D-fructose. Carbohydr. Res. 339, 2267–2273. doi:10.1016/j.carres.2004.06.022
Illing, N., Denby, K. J., Collett, H., Shen, A., and Farrant, J. M. (2005). The signature of seeds in resurrection plants: a molecular and physiological comparison of desiccation tolerance in seeds and vegetative tissues. Integr. Comp. Biol. 45, 771–787. doi:10.1093/icb/45.5.771
Ito, K., Moynihan, C. T., and Angell, C. A. (1999). Thermodynamic determination of fragility in liquids and a fragile-to-strong liquid transition in water. Nature 398, 492–495. doi:10.1038/19042
Jain, N. K., and Roy, I. (2009). Effect of trehalose on protein structure. Protein Sci. 18, 24–36. doi:10.1002/pro.3
Jugdawa, Y., Bhaw-Luximon, A., Wesner, D., Goonoo, N., Schönherr, H., and Jhurry, D. (2017). Polysucrose-based hydrogels for loading of small molecules and cell growth. React. Funct. Polym. 115, 18–27. doi:10.1016/j.reactfunctpolym.2017.03.012
Kaczmarek, Ł., Roszkowska, M., Fontaneto, D., Jezierska, M., Pietrzak, B., Wieczorek, R., et al. (2019). Staying young and fit? Ontogenetic and phylogenetic consequences of animal anhydrobiosis. J. Zool. 309, 1–11. doi:10.1111/jzo.12677
Königshausen, E., Potthoff, S. A., Woznowski, M., Stegbauer, J., Rump, L. C., and Sellin, L. (2019). Highly sensitive measurement of glomerular permeability in mice with fluorescein isothiocyanate-polysucrose 70. J. Vis. Exp. doi:10.3791/59064
Kuwayama, M., Gandhi, G., Kagalwala, S., and Ramani, R. (2015). “Vitrification: an overview,” in Vitrification in assisted reproduction: a user’s manual. Editors G. Allahbadia, M. Kuwayama, and G. Gandhi (New Delhi: Springer India), 1–7.
Lim, H., and Hoag, S. W. (2013). Plasticizer effects on physical–mechanical properties of solvent cast Soluplus® films. AAPS PharmSciTech 14, 903–910. doi:10.1208/s12249-013-9971-z
Mascia, L., Kouparitsas, Y., Nocita, D., and Bao, X. (2020). Antiplasticization of polymer materials: structural aspects and effects on mechanical and diffusion-controlled properties. Polymers 12, 769. doi:10.3390/polym12040769
Pan, D., Inoue, A., Sakurai, T., and Chen, M. W. (2008). Experimental characterization of shear transformation zones for plastic flow of bulk metallic glasses. Proc. Natl. Acad. Sci. U. S. A. 105, 14769–14772. doi:10.1073/pnas.0806051105
Quach, A., and Ferrante, A. (2017). The application of dextran sedimentation as an initial step in neutrophil purification promotes their stimulation, due to the presence of monocytes. J. Immunol. Res. 2017, 1–10. doi:10.1155/2017/1254792
Ramirez, J. F., Kumara, U. G. V. S., Arulsamy, N., and Boothby, T. C. (2024). Water content, transition temperature and fragility influence protection and anhydrobiotic capacity. BBA Advances 2024. doi:10.1016/j.bbadva.2024.100115
Ruiz, G. N., Romanini, M., Hauptmann, A., Loerting, T., Shalaev, E., Tamarit, J. L., et al. (2017). Genuine antiplasticizing effect of water on a glass-former drug. Sci. Rep. 7, 7470. doi:10.1038/s41598-017-07643-5
Sakurai, M., Furuki, T., Akao, K.-I., Tanaka, D., Nakahara, Y., Kikawada, T., et al. (2008). Vitrification is essential for anhydrobiosis in an African chironomid, Polypedilum vanderplanki. Proc. Natl. Acad. Sci. U. S. A. 105, 5093–5098. doi:10.1073/pnas.0706197105
Seow, C. C., Cheah, P. B., and Chang, Y. P. (1999). Antiplasticization by water in reduced-moisture food systems. J. Food Sci. 64, 576–581. doi:10.1111/j.1365-2621.1999.tb15088.x
Sun, W. Q., and Leopold, A. C. (1997). Cytoplasmic vitrification and survival of anhydrobiotic organisms. Comp. Biochem. Physiol. A Physiol. 117, 327–333. doi:10.1016/s0300-9629(96)00271-x
Truong, V., Bhandari, B. R., Howes, T., and Adhikari, B. (2004). Glass transition behaviour of fructose. Int. J. Food Sci. Technol. 39, 569–578. doi:10.1111/j.1365-2621.2004.00817.x
Walters, C. (2004). Temperature dependency of molecular mobility in preserved seeds. Biophys. J. 86, 1253–1258. doi:10.1016/s0006-3495(04)74199-5
Watanabe, M. (2006). Anhydrobiosis in invertebrates. Appl. Entomol. Zool. 41, 15–31. doi:10.1303/aez.2006.15
Weng, L., and Elliott, G. D. (2015). Local minimum in fragility for trehalose/glycerol mixtures: implications for biopharmaceutical stabilization. J. Phys. Chem. B 119, 6820–6827. doi:10.1021/acs.jpcb.5b01675
Keywords: vitrification, sucrose, polysucrose, glycerol, glass transition, glass former fragility, water content
Citation: Kumara UGVSS, Ramirez JF and Boothby TC (2024) The effect of sucrose polymer-size on glass transition temperature, glass former fragility, and water retention during drying. Front. Mater. 11:1351671. doi: 10.3389/fmats.2024.1351671
Received: 06 December 2023; Accepted: 22 January 2024;
Published: 07 February 2024.
Edited by:
Ceren Karaman, Akdeniz University, TürkiyeReviewed by:
Shanshan Shi, University of South Carolina, United StatesHiroshi Sekiguchi, Japan Synchrotron Radiation Research Institute, Japan
Copyright © 2024 Kumara, Ramirez and Boothby. This is an open-access article distributed under the terms of the Creative Commons Attribution License (CC BY). The use, distribution or reproduction in other forums is permitted, provided the original author(s) and the copyright owner(s) are credited and that the original publication in this journal is cited, in accordance with accepted academic practice. No use, distribution or reproduction is permitted which does not comply with these terms.
*Correspondence: Thomas C. Boothby, dGJvb3RoYnlAdXd5by5lZHU=