- 1Department of Oral Medicine, Infection, and Immunity, Harvard School of Dental Medicine, Boston, MA, United States
- 2Department of Biologic and Materials Science, University of Michigan School of Dentistry, Ann Arbor, MI, United States
- 3John A. Paulson School of Engineering and Applied Sciences, Harvard University, Cambridge, MA, United States
- 4Wyss Institute for Biologically Inspired Engineering, Harvard University, Boston, MA, United States
Cell-instructive biomaterials are an essential component in tissue engineering and regenerative medicine. In the past three decades since the term “Tissue Engineering” was coined, researchers have made significant progress towards regenerating disease or damage tissues and organs by combining innovations in biomaterials, signaling molecules and cell therapies. However, challenges persist including limitations in properties of cell-instructive biomaterials, lack of advanced manufacturing technologies for precise spatiotemporal control of key players in tissue engineering, and hurdles in clinical translation and regulatory process. In this perspective article, we briefly review the current state of the field including the evolution in our understanding of the role biomaterial mechanics and scaffolding architecture, development of self-healing and modular biomaterials, and progress in advanced manufacturing technologies such as 3D bioprinting. In addition, we discuss about how innovation in research technologies including multi-omics and spatial biology, and advanced imaging modalities may pave the way for enhancing our understanding about cell-biomaterial interactions. Finally, we present our perspective as early career clinicians and researchers on the key role and potential impact that clinician-scientists can generate in the development, validation, clinical translation and adoption of the next-generation of cell-instructive biomaterials for application in engineering tissues and organs to impact human health.
1 Advances in biomaterial mechanics and mechanoregeneration
Biomaterial mechanics are key determinants of their suitability for biomedical applications. Since the birth of the field of tissue engineering in the 1990s (Langer and Vacanti, 1993), scientists focused largely towards designing biomaterials for tissue regeneration. In these approaches, biomaterials commonly served as temporary scaffolds that mimic the native extracellular matrix (ECM), and provided a favorable microenvironment for cells to form of tissue(s) of interest (Drury and Mooney, 2003).
In native tissues, resident cells exist within a complex and physically confining three-dimensional (3D) ECM, which provides key signals directing cellular behavior (Ingber et al., 1994; Vining and Mooney, 2017; Yamada and Sixt, 2019). In addition to biochemical signals such as growth factors and chemokines, cells have the ability to sense and respond to biophysical signals within their microenvironment (Discher et al., 2009). The cell-ECM mechanotransduction is facilitated through binding between cell surface receptors to peptide epitopes conjugated to the ECM, such as arginine–glycine–aspartate (RGD) ligands (Rowley et al., 1999). Recent advances in material sciences have expanded the arsenal of cell adhesion peptides that can directly interact with surface receptors, such as integrins or cadherins, to alter downstream signaling and cellular response (Hamley, 2017; Ligorio and Mata, 2023). For instance, phage display technology has been successfully employed to identify and further expand the library of small peptides applicable for biomaterial functionalization (Martins et al., 2016). By conjugating these cell adhesion molecules to biomaterials, researchers have significantly improved cell-material interactions leading to enhanced cell response and behavior when encapsulated including sensing the mechanical cues provided by the materials (Rowley and Mooney, 2002; Ligorio and Mata, 2023). In fact, mechanical forces can direct stem cell behavior in development and regeneration (Vining and Mooney, 2017). As cells interact with the surrounding ECM, a cascade of mechanosensing and mechanotransduction signaling pathways work together to mediate change in gene and protein expression leading to alteration in cell behavior (Vining and Mooney, 2017; Wu et al., 2022b), this has been discussed in a number of review articles.
In the past two decades, research in mechanobiology, studying mechanisms by which cells sense and respond to mechanical signals, has uncovered the fundamental role of biomaterial mechanical properties in tuning cell function. In the late 1990s and early 2000s, researchers elucidated the role of ECM stiffness on tuning cell behaviour both in two-dimension (2D) and 3D (Discher et al., 2005; Engler et al., 2006). Particularly, elasticity or stiffness (Discher et al., 2005; Vining and Mooney, 2017) has been demonstrated to control many important cell behaviors in tissue regeneration, such as migration, proliferation, differentiation, and matrix deposition (Alsberg et al., 2001). In the 2010s, further research demonstrated the role of ECM stiffness in tissue regeneration including the promotion of craniofacial bone regeneration in vivo when mesenchymal stem cells are encapsulated in hydrogels with a favorable stiffness (Huebsch et al., 2015; Huebsch et al., 2010) (Figure 1).
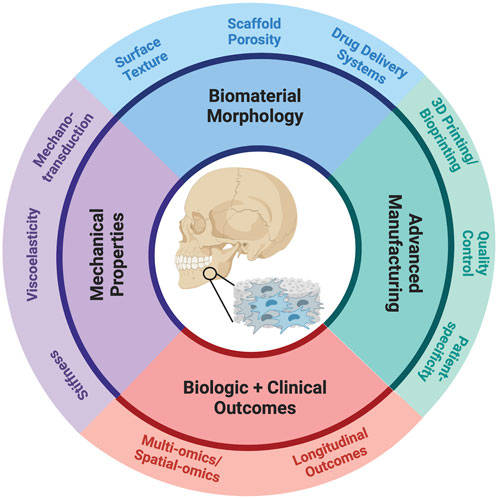
FIGURE 1. Schematic of key considerations in the design and fabrication of cell-instructive biomaterials.
However, all living tissues are viscoelastic. In the recent years, researchers have identified matrix viscoelasticity as a key mechanical property capable of modulating 2D and 3D cell and tissue behavior (Chaudhuri et al., 2020; Chaudhuri et al., 2016; Chaudhuri et al., 2015; Vining and Mooney, 2017) for in vitro tissue engineering and in vivo regeneration (Wu et al., 2022b). Recent research has elucidated the role of viscoelasticity on tuning stem cell spheroid and organoid behavior (Wu et al., 2022a; Elosegui-Artola et al., 2023). In addition, the viscoelasticity has been demonstrated to impact in vivo tissue regeneration as biomaterials with rapid stress relaxation enhanced bone regeneration in a rodent model (Darnell et al., 2017; Whitehead et al., 2021) (Figure 1).
As research progresses in silico, with the development of spheroid and organoid systems and organ-on-chip technologies, researchers have additional tools to study the effect of biomaterial mechanics in a more physiologically relevant model while reducing the need for animal studies (Ingber, 2022; Leung et al., 2022). These systems can recapitulate aspects of human biology, enhance the high throughput of experiments, and enable a more ethical conduct of biomaterial research to continue to advance our field. Research and development teams may also choose to use finite element analysis models (FEA) or other computational models to analyze the behavior of complex structures and materials prior to beginning animal studies. FEA aids in material selection process, design optimization, and failure analysis (Wu and Briant, 2012; Hendrikson et al., 2017).
2 Advances in scaffold architecture and morphology
In the 1940s, medical devices highlighted the importance of textured and porous features as precursors to today’s tissue engineering techniques in biomaterial fabrication. Biomaterial surfaces are crucial as interfaces between the host and implants, influencing various biological processes (Liu et al., 2022). Different applications require unique biomaterial structures, and there is no one-size-fits-all approach to fabrication methods. Textured and porous materials with open systems have been proven to foster positive interactions between the host and implant across different disciplines, ensuring successful long-term integration (Swanson and Ma, 2020). Recent literature and commercial products underscore the significance of biomaterial architectural features, such as surface texture and porosity, backed by reliable fabrication methods.
For the next-generation of tissue engineering biomaterials, engineers must develop a toolkit of fabrication strategies encompassing nano, micro, and macro-level features (Anderson, 2006). Advancements in materials fabrication technology are pivotal in fostering collaboration between materials science, engineering, and biomedical sciences. “Scaffold architecture” pertains to how bulk material is distributed at macro, micro, and nano-scales, aligning with tissue, cellular, and molecular levels of organization (Muschler et al., 2004). Customizability at each architectural level is vital for influencing biological outcomes because there is no universal construct, and scaffolds are designed for specific applications. Tissue engineering scaffold design involves selecting biodegradable and biocompatible polymers, architectural features, and mechanical properties tailored to the target tissue, facilitating cell adhesion, proliferation, differentiation, and nutrient exchange. In particular, scaffold texture, such as topography and surface patterning, and porosity and pore sizes, play critical roles in addressing these needs (Loh and Choong, 2013; Swanson and Ma, 2020; Adhikari et al., 2023) (Figure 1).
Texture is vital. Textured surfaces promote integration by aiding cell infiltration and reducing inflammation, making them advantageous for host-guest interactions (Ito, 1999). Tissue engineering scaffolds aim to mimic the collagen extracellular matrix (ECM) found in most tissues (Langer and Vacanti, 1993). Collagen, the body’s most abundant extracellular protein, has a triple helix macro-structure and sub-micrometer fiber diameter. Processing technologies like electrospinning, self-assembly, and thermally induced phase separation are well-established for achieving an ECM-like nano-architecture and surface texture in synthetic biomaterials (Ma and Zhang, 1999). Each of these methods has unique properties and applications, which we have previously described in detail elsewhere (Swanson and Ma, 2020).
Replicating the 3D structure and role of the native ECM is crucial in tissue engineering (Ma and Zhang, 1999). Nanofibers resulting from thermally-induced phase separation create a biomimetic fiber network that enhances protein adsorption (Woo et al., 2003) and promotes cell adhesion and proliferation (Smith et al., 2010), affecting cell differentiation (Smith et al., 2009). In various contexts, 3D nanofibrous matrices facilitate osteoblast cell adhesion, stem cell differentiation (Liu et al., 2009), and tissue regeneration (Gupte and Ma, 2012) (Figure 1).
Pores are equally important. Native tissues have hierarchical nutrient, waste, and signaling molecule transport channels (Nunes et al., 1997). In synthetic prostheses or tissue engineering scaffolds, mirroring this native porous architecture is beneficial (Ryan et al., 2006). Pore size requirements vary by scaffold and tissue type, with a minimum pore size needed for vascularization (Gupte et al., 2018; Swanson et al., 2021), tissue ingrowth, and uniform cell distribution. Pore curvature influences early differentiation of mesenchymal cells (Swanson et al., 2022). A porous 3D construct offers a high surface area and void space for crucial cell-matrix interactions, and synthetic polymers are advantageous for their reproducible fabrication with pre-designed macropores. Macropores are particularly important in cell-free constructs, where endogenous cells fill defects (Swanson et al., 2020). Such 3D tissue engineering scaffolds with interconnected macropores have shown regenerative potential in various tissues and physiological systems within a short timeframe (Figure 1).
3 Advances in self-healing and modular materials
Technologies developed during the nuanced eras of tissue engineering utilized a “top-down” approach in which variations cells, growth factors, and scaffolds were tested together until an appropriate engineered tissue was produced (Nichol and Khademhosseini, 2009). These traditional approaches often resulted in non-homogenous cell distributions (Sittinger et al., 2004) and were time intensive. Recent advances in the fabrication and manufacturing of self-healing and modular materials with promising applications in tissue engineering and regenerative medicine.
Hydrogels are commonly used in biomaterials due to their ability to maintain their structural integrity, emulate an ideal environment to mimic native tissues (Mantha et al., 2019), and seamlessly transition between liquid and solid states (Ho et al., 2022). Their ability to rapidly transition between solid-like and liquid-like states makes them ideal injectable and extrudable materials in surgical procedures and 3D printing, respectively (Zhang et al., 2018; Salzlechner et al., 2020). Self-healing biomaterials have shown potential for regenerative medicine applications (Riley et al., 2019; Uman et al., 2020) and therapeutics due to controlled delivery into patient-specific tissue defects (Li and Mooney, 2016; Choe et al., 2018; Li et al., 2023).
The modularity of granular hydrogels can induce an appropriate host response. For example, researchers can modify rheological properties to control the rate of infiltration of biological structures and restrict excessive deposition of ECM by invading cells, or in the case of severe tissue damage maximize cell response (Qazi and Burdick, 2021).
Granular hydrogels can self-heal through a process called “jamming transition”, where the particles in the material rearrange themselves to fill the void left by the damage (Daly et al., 2019; Riley et al., 2019; Muir et al., 2021). The jamming phenomenon results in microporous properties that enable injectability. Additionally, the shear-thinning characteristic of granular materials makes it a great candidate for extrusion bioprinting as they can be customized to emulate the biophysical and biochemical environment of the extracellular matrix. The fabrication of different shapes of the microgels, granular bioinks using spheroid and organoid building blocks, and application of multimaterial bioprinting are all recent areas of interests by researchers (Daly, 2023). Several proof-of-concept models have been published and show evidence of feasibility (Chaji et al., 2020; McCormack et al., 2020; Flégeau et al., 2022; Guo and Longaker, 2022).
These multifunctional materials offer enormous potential for complex biomimicry in regenerative applications. Researchers are currently challenged by synthesis and characterization techniques of injectable hydrogels at a scalable level and various regulatory aspects (Alonso et al., 2021). There is no consensus in the fabrication process of microgels, and variations in key rheological parameters influence bioprinting quality outcomes. Development of synthetic polymers is crucial to achieving consistent quality bioink prior to translating this technology into clinics (Bian, 2020). Animal studies and biocompatibility research is needed to address the challenges associated with these self-healing hydrogels and to fully realize their potential in clinical applications.
4 Advances in 3D bioprinting and manufacturing
3D bioprinting is a key manufacturing method that allows researchers to mimic native tissue structures consistently, automate complex manufacturing processes, and create patient-specific and organ-specific tissue-engineered constructs. Recently, exciting advances are paving the way to expand the future of biomaterials research and provide an important avenue to translate materials innovations into biomedical applications. These developments include aspiration-assisted freeform bioprinting (Kim et al., 2022), combinatorial multimaterial bioprinting (Hassan et al., 2022), high cell density and high-resolution bioprinting (Skylar-Scott et al., 2019; You et al., 2023). In addition, four-dimensional (4D) bioprinting has emerged to integrate time as the fourth dimension where printed constructs can change their shape or function when an external stimulus is introduced, or when cell fusion and self-assembly occur post-printing (Gao et al., 2016). Together, these developments will enhance precise spatiotemporal control of the manufacturing of tissues and organs adaptable to individual patients and specific clinical needs.
One significant challenge in bioprinting is tuning the intricate balance between cell functions and printability. With recent progress in the development of granular hydrogel bioinks, bulk microporosity in bioprinted constructs enhances the diffusion of nutrients and allows for better cell growth (Tuftee et al., 2023). The modular nature of granular hydrogels allows researchers to gain control over printability, resolution, porosity, scaffold degradation, cell loading, and heterogeneity, all contributing to enhancing the versatility of 3D bioprinting technology to address unmet clinical needs.
Another significant challenge in 3D bioprinting is the maintenance of bioprinted tissue viability. The inclusion of complex vascular networks for nutrient transport and waste disposal is critical to generating viable tissues suitable for clinical application (Chen et al., 2021). For instance, large bone defects are difficult to regenerate due to the lack of effective vascularization in the biomaterial scaffold (Shen et al., 2022). Recent advances in 3D bioprinting pave the way for fabrication of tissue constructs with enhanced vascularization with strategies such as the incorporation of pro-angiogenic factors, for example vascular endothelial growth factors (VEGF), platelet-derived growth factors (PDGF) or fibroblast growth factor (FGF), to bioinks induce host vascular in growth into the implanted tissue, the utilization of sacrificial printing techniques to create perfusable tissues, or the fabrication of scaffolds with their own vasculature that can be directly microsurgically connected to the host vasculature to promote tissue integration (Skylar-Scott et al., 2019; Chen et al., 2021; Joshi et al., 2022).
3D bioprinting has the potential to revolutionize the future of healthcare by fabricating organ replacement options to overcome donor organ supply shortage, providing physiologically relevant in vitro models for drug testing to reduce the use of animals in research and enabling personalized treatments in regenerative medicine. For clinical translation, recent progress highlights the potential of intraoperative bioprinting of heterogeneous hard and soft tissues during craniomaxillofacial reconstructive surgery (Moncal et al., 2021; Kang et al., 2023). However, future work is needed to address the challenges of 3D bioprinting, including high costs associated with expensive technology, regulatory hurdles of cell therapy, quality control of manufacturing, and ethical concerns (Datta et al., 2023).
5 Advances in multi-omics and spatial biology for understanding cell-material interactions
Understanding cell-material interactions such as inflammation, degradation, and remodeling is necessary to develop new biomaterials. Recent advances in multi-omics and spatial biology pave the way to enhance our understanding of these complex interactions at a single-cell resolution with spatial information. These methods allow researchers to gather valuable data and refine material design with high throughput screening of cell-material interactions (Sari et al., 2022). For instance, macrophages play key roles in host response to biomaterials upon implantation. Our understanding of biomaterial degradation-related macrophage subpopulations around implanted biomaterials has evolved from the classic M1/M2 profiles (Mills, 2012). Single-cell RNA-seq enable the comprehensive analysis of transcriptome profiles around biomaterial scaffolds, and mapping of scaffold induced cell response at high resolution.
In addition, new advances in spatial biology pave the way to precisely identify the biogeography of cellular activity, gene and protein expression, providing spatial and temporal information in cell-biomaterial interaction. Both mammalian and bacterial cellular activities can be studied at a single-cell level putting their behavior in the context of their microenvironment. For example, novel technologies such as spatial phenotyping have the potential to combine multi-omics data and relate with specific tissue, organ and biomaterial architecture to create a “Google Map” of cell-material interactions (Shi et al., 2020; Hickey et al., 2022). These powerful tools will pave the way for researchers to refine the development of cell-instructive biomaterials.
6 Advanced imagine modalities in tissue engineering
Advanced imaging techniques are essential for noninvasive, longitudinal, and consistent monitoring of tissue-engineered constructs, thus overcoming the limitations of conventional analysis tools and formalin-fixed-paraffin-embedded (FFPE) histologic analysis (Nam et al., 2015). Confocal, Raman, and holotomography are advanced imaging modalities used in tissue engineering. Confocal microscopy can provide exact 3D imaging and correctly measure subcellular structure and dynamic processes. Confocal microscopy is widely used to examine surface and near-surface features in biomaterials such as bone, dentin, and enamel, imaging cells on scaffolds, and monitoring the function of tissue-engineered constructs (Wang and Larina, 2017). Raman spectroscopy is a label-free vibrational technique that offers unique insights into the structure and composition of tissues and cells at the molecular level (Bergholt et al., 2019). Raman spectroscopy has been a growing tool in tissue engineering, providing a wealth of quantitative information about the molecular structure of a tissue’s extracellular matrix (ECM). Holotomography is a new imaging technique that uses X-ray synchrotron radiation to investigate angio- and microvasculogenesis without using contrast agents (Appel et al., 2013). Holotomography has been used to evaluate artificial tissues, and it can reveal details down to subcellular length scales. Currently, these methods are limited in capturing complex structures at z-lengths greater than 100–500 um, depending on the modality. This area of active investigation and engineering optimization will hold great significance in evaluating the cell-biomaterial interface and regenerative outcomes in the future. Each imaging method has its range of applications and provides information based on the specific properties of the imaging technique. Therefore, according to the requirements of the tissue engineering studies, the most appropriate tool should be selected among various imaging modalities.
7 The role of clinician-scientists in translation and adoption
Despite advances in material sciences and advanced manufacturing technologies, the clinical adoption of innovation is confronted by numerous challenges, including strict regulatory policies surrounding biologics and cell therapies, resource-intensive clinical trials, complex reimbursement and payer structure, and Roger’s bell curve of technology adoption. Clinician-scientists who have completed formal bioengineering research and clinical practice training can provide valuable perspectives and insights into translation innovation and adoption to the clinical arena (Roberts et al., 2012).
First, clinician-scientists may be in the ideal position to identify unmet clinical needs and develop innovative solutions to address these needs with the support of their research labs and engineering team. Rather than developing a medical device or technology first and finding a clinical application second, a solution should be reverse engineered with a clinical indication in mind. Once the clinical need and solution has been identified and designed, the clinician-scientist can provide clinical validation and assist in human factors design parameters (Herr, 2010). Clinician-led innovation can lead to increased surgical quality and value, and can decrease product development timelines (Augustin et al., 2020; Schwartz et al., 2016).
Second, clinician-scientists can lead pivotal clinical trials with their knowledge of the technology, experience in trial design and execution, and clinical skills to carry out the interventions (Roberts et al., 2012). To strengthen the pipeline of clinician-scientists contributing to the process of translational medicine, it is necessary to develop strong training programs, provide quality mentoring, and robust funding (Herzog et al., 2018).
Third, clinician-scientists may have experience with regulatory pathways and are well-positioned to communicate with regulators such as the FDA. Understanding the different regulatory pathways available prior to technology development is key to alleviating some of the difficulties in attaining FDA approval for biologics. As defined in the Code of Federal Regulations Title 21 Section 3.2(e), a combination product is comprised of two regulated components. By developing a biologic/device combination product rather than a sole biologic, researchers can apply through the FDA medical device approval process, a notably shorter approval process compared to the FDA biologic approval process.
Fourth, clinician-scientists may have deep insights into the reimbursement process and identify the appropriate reimbursement strategy, associated Current Procedural Terminology (CPT) codes (i.e., medical insurance billing codes), and product pricing, all of which are key to successful adoption. Many of these technologies are new and do not have existing CPT codes. During the research translation process, conversation with the Center for Medicare and Medicaid Services should begin to request a new CPT code or modification of an existing code. Having an approved CPT code not only helps with negotiating insurance reimbursement but generates interest from an investor’s perspective, can increase market size, and accelerate clinician adoption rate. Ability to quantify the proposed value of the product when negotiating reimbursement with insurance is crucial to this step. Collaborating with a biotechnology analyst who is comfortable with gathering primary and secondary market research in fields with limited information is key. Clinician-scientists can help analysts understand the value of the technology in these discussions. The Michigan-Pittsburgh-Wyss Regenerative Medicine (MPWRM) Resource Center and Center for Dental, Oral, and Craniofacial Tissue & Organ Regeneration (C-DOCTOR) are centers from the National Institute of Dental and Craniofacial Research with expert scientists, engineers, clinicians, regulatory and technology commercialization teams specialized in regenerative medicine available to researchers accepted into the programs (Taylor et al., 2021).
Last, clinician-scientists may also be excellent educators to provide training to the clinical community to enhance patient outcomes by communicating about the scientific basis of the technologies, and clinical tips.
In sum, advances in biomaterials and bioengineering will revolutionize the future of regenerative medicine. Clinician-scientists are well-positioned to lead the adoption of innovation into the clinical arena.
Data availability statement
The original contributions presented in the study are included in the article/supplementary material, further inquiries can be directed to the corresponding author.
Author contributions
RR: Conceptualization, Visualization, Writing–original draft, Writing–review and editing. WS: Conceptualization, Visualization, Writing–original draft, Writing–review and editing. DW: Conceptualization, Supervision, Visualization, Writing–original draft, Writing–review and editing.
Funding
The author(s) declare financial support was received for the research, authorship, and/or publication of this article. Osteology Foundation (OF-21032), NIH/NIDCR (F30DE029359).
Conflict of interest
The authors declare that the research was conducted in the absence of any commercial or financial relationships that could be construed as a potential conflict of interest.
Publisher’s note
All claims expressed in this article are solely those of the authors and do not necessarily represent those of their affiliated organizations, or those of the publisher, the editors and the reviewers. Any product that may be evaluated in this article, or claim that may be made by its manufacturer, is not guaranteed or endorsed by the publisher.
References
Adhikari, J., Roy, A., Chanda, A., Gouripriya, D. A., Thomas, S., Ghosh, M., et al. (2023). Effects of surface patterning and topography on the cellular functions of tissue engineered scaffolds with special reference to 3D bioprinting. Biomater. Sci. 11, 1236–1269. doi:10.1039/D2BM01499H
Alonso, J. M., Andrade Del Olmo, J., Perez Gonzalez, R., and Saez-Martinez, V. (2021). Injectable hydrogels: from laboratory to industrialization. Polymers 13, 650. doi:10.3390/polym13040650
Alsberg, E., Anderson, K. W., Albeiruti, A., Franceschi, R. T., and Mooney, D. J. (2001). Cell-interactive alginate hydrogels for bone tissue engineering. J. Dent. Res. 80, 2025–2029. doi:10.1177/00220345010800111501
Anderson, J. M. (2006). The future of biomedical materials. J. Mater Sci. Mater Med. 17, 1025–1028. doi:10.1007/s10856-006-0439-5
Appel, A. A., Anastasio, M. A., Larson, J. C., and Brey, E. M. (2013). Imaging challenges in biomaterials and tissue engineering. Biomaterials 34, 6615–6630. doi:10.1016/j.biomaterials.2013.05.033
Augustin, D. A., Yock, C. A., Wall, J., Lucian, L., Krummel, T., Pietzsch, J. B., et al. (2020). Stanford’s biodesign innovation program: teaching opportunities for value-driven innovation in surgery. Surgery 167, 535–539. doi:10.1016/j.surg.2019.10.012
Bergholt, M. S., Serio, A., and Albro, M. B. (2019). Raman spectroscopy: guiding light for the extracellular matrix. Front. Bioeng. Biotechnol. 7, 303. doi:10.3389/fbioe.2019.00303
Bian, L. (2020). Functional hydrogel bioink, a key challenge of 3D cellular bioprinting. Apl. Bioeng. 4, 030401. doi:10.1063/5.0018548
Chaji, S., Al-Saleh, J., and Gomillion, C. (2020). Bioprinted three-dimensional cell-laden hydrogels to evaluate adipocyte-breast cancer cell interactions. Gels 6, 10. doi:10.3390/gels6010010
Chaudhuri, O., Cooper-White, J., Janmey, P. A., Mooney, D. J., and Shenoy, V. B. (2020). Effects of extracellular matrix viscoelasticity on cellular behaviour. Nature 584, 535–546. doi:10.1038/s41586-020-2612-2
Chaudhuri, O., Gu, L., Darnell, M., Klumpers, D., Bencherif, S. A., Weaver, J. C., et al. (2015). Substrate stress relaxation regulates cell spreading. Nat. Commun. 6, 6365. doi:10.1038/ncomms7365
Chaudhuri, O., Gu, L., Klumpers, D., Darnell, M., Bencherif, S. A., Weaver, J. C., et al. (2016). Hydrogels with tunable stress relaxation regulate stem cell fate and activity. Nat. Mater 15, 326–334. doi:10.1038/nmat4489
Chen, E. P., Toksoy, Z., Davis, B. A., and Geibel, J. P. (2021). 3D bioprinting of vascularized tissues for in vitro and in vivo applications. Front. Bioeng. Biotechnol. 9, 664188. doi:10.3389/fbioe.2021.664188
Choe, G., Park, J., Park, H., and Lee, J. Y. (2018). Hydrogel biomaterials for stem cell microencapsulation. Polym. (Basel) 10, 997. doi:10.3390/polym10090997
Daly, A. C. (2023). Granular hydrogels in biofabrication: recent advances and future perspectives. Adv. Healthc. Mater., e2301388. doi:10.1002/adhm.202301388
Daly, A. C., Riley, L., Segura, T., and Burdick, J. A. (2019). Hydrogel microparticles for biomedical applications. Nat. Rev. Mater 5, 20–43. doi:10.1038/s41578-019-0148-6
Darnell, M., Young, S., Gu, L., Shah, N., Lippens, E., Weaver, J., et al. (2017). Substrate stress-relaxation regulates scaffold remodeling and bone formation in vivo. Adv. Healthc. Mater 6. doi:10.1002/adhm.201601185
Datta, P., Cabrera, L. Y., and Ozbolat, I. T. (2023). Ethical challenges with 3D bioprinted tissues and organs. Trends Biotechnol. 41, 6–9. doi:10.1016/j.tibtech.2022.08.012
Discher, D. E., Janmey, P., and Wang, Y. (2005). Tissue cells feel and respond to the stiffness of their substrate. Science 310, 1139–1143. doi:10.1126/science.1116995
Discher, D. E., Mooney, D. J., and Zandstra, P. W. (2009). Growth factors, matrices, and forces combine and control stem cells. Science 324, 1673–1677. doi:10.1126/science.1171643
Drury, J. L., and Mooney, D. J. (2003). Hydrogels for tissue engineering: scaffold design variables and applications. Biomaterials 24, 4337–4351. doi:10.1016/S0142-9612(03)00340-5
Elosegui-Artola, A., Gupta, A., Najibi, A. J., Seo, B. R., Garry, R., Tringides, C. M., et al. (2023). Matrix viscoelasticity controls spatiotemporal tissue organization. Nat. Mater 22, 117–127. doi:10.1038/s41563-022-01400-4
Engler, A. J., Sen, S., Sweeney, H. L., and Discher, D. E. (2006). Matrix elasticity directs stem cell lineage specification. Cell 126, 677–689. doi:10.1016/j.cell.2006.06.044
Flégeau, K., Puiggali-Jou, A., and Zenobi-Wong, M. (2022). Cartilage tissue engineering by extrusion bioprinting utilizing porous hyaluronic acid microgel bioinks. Biofabrication 14, 034105. doi:10.1088/1758-5090/ac6b58
Gao, B., Yang, Q., Zhao, X., Jin, G., Ma, Y., and Xu, F. (2016). 4D bioprinting for biomedical applications. Trends Biotechnol. 34, 746–756. doi:10.1016/j.tibtech.2016.03.004
Guo, J. L., and Longaker, M. T. (2022). Bioprinted hydrogels for fibrosis and wound healing: treatment and modeling. Gels 9, 19. doi:10.3390/gels9010019
Gupte, M. J., and Ma, P. X. (2012). Nanofibrous scaffolds for dental and craniofacial applications. J. Dent. Res. 91, 227–234. doi:10.1177/0022034511417441
Gupte, M. J., Swanson, W. B., Hu, J., Jin, X., Ma, H., Zhang, Z., et al. (2018). Pore size directs bone marrow stromal cell fate and tissue regeneration in nanofibrous macroporous scaffolds by mediating vascularization. Acta Biomater. 82, 1–11. doi:10.1016/j.actbio.2018.10.016
Hamley, I. W. (2017). Small bioactive peptides for biomaterials design and therapeutics. Chem. Rev. 117, 14015–14041. doi:10.1021/acs.chemrev.7b00522
Hassan, S., Gomez-Reyes, E., Enciso-Martinez, E., Shi, K., Campos, J. G., Soria, O. Y. P., et al. (2022). Tunable and compartmentalized multimaterial bioprinting for complex living tissue constructs. ACS Appl. Mat. Interfaces 14, 51602–51618. doi:10.1021/acsami.2c12585
Hendrikson, W. J., van Blitterswijk, C. A., Rouwkema, J., and Moroni, L. (2017). The use of finite element analyses to design and fabricate three-dimensional scaffolds for skeletal tissue engineering. Front. Bioeng. Biotechnol. 5, 30. doi:10.3389/fbioe.2017.00030
Herr, G. L. (2010). Biodesign: the process of innovating medical technologies. Biomed. Instrum. Technol. 44, 388. doi:10.2345/0899-8205-44.5.388
Herzog, C. R., Berzins, D. W., DenBesten, P., Gregory, R. L., Hargreaves, K. M., Messer, R. L. W., et al. (2018). Oral sciences PhD program enrollment, graduates, and placement: 1994 to 2016. J. Dent. Res. 97, 483–491. doi:10.1177/0022034517749506
Hickey, J. W., Neumann, E. K., Radtke, A. J., Camarillo, J. M., Beuschel, R. T., Albanese, A., et al. (2022). Spatial mapping of protein composition and tissue organization: a primer for multiplexed antibody-based imaging. Nat. Methods 19, 284–295. doi:10.1038/s41592-021-01316-y
Ho, T.-C., Chang, C.-C., Chan, H.-P., Chung, T.-W., Shu, C.-W., Chuang, K.-P., et al. (2022). Hydrogels: properties and applications in biomedicine. Molecules 27, 2902. doi:10.3390/molecules27092902
Huebsch, N., Arany, P. R., Mao, A. S., Shvartsman, D., Ali, O. A., Bencherif, S. A., et al. (2010). Harnessing traction-mediated manipulation of the cell/matrix interface to control stem-cell fate. Nat. Mater 9, 518–526. doi:10.1038/nmat2732
Huebsch, N., Lippens, E., Lee, K., Mehta, M., Koshy, S. T., Darnell, M. C., et al. (2015). Matrix elasticity of void-forming hydrogels controls transplanted stem cell-mediated bone formation. Nat. Mater 14, 1269–1277. doi:10.1038/nmat4407
Ingber, D. E. (2022). Human organs-on-chips for disease modelling, drug development and personalized medicine. Nat. Rev. Genet. 23, 467–491. doi:10.1038/s41576-022-00466-9
Ingber, D. E., Dike, L., Hansen, L., Karp, S., Liley, H., Maniotis, A., et al. (1994). “Cellular tensegrity: exploring how mechanical changes in the cytoskeleton regulate cell growth, migration, and tissue pattern during morphogenesis,” in International review of cytology, mechanical engineering of the cytoskeleton in developmental biology. Editor R. Gordon (Academic Press), 173–224. doi:10.1016/S0074-7696(08)61542-9
Ito, Y. (1999). Surface micropatterning to regulate cell functions. Biomaterials 20, 2333–2342. doi:10.1016/s0142-9612(99)00162-3
Joshi, A., Choudhury, S., Gugulothu, S. B., Visweswariah, S. S., and Chatterjee, K. (2022). Strategies to promote vascularization in 3D printed tissue scaffolds: trends and challenges. Biomacromolecules 23, 2730–2751. doi:10.1021/acs.biomac.2c00423
Kang, Y., Yeo, M., Derman, I. D., Ravnic, D. J., Singh, Y. P., Alioglu, M. A., et al. (2023). Intraoperative bioprinting of human adipose-derived stem cells and extra-cellular matrix induces hair follicle-like downgrowths and adipose tissue formation during full-thickness craniomaxillofacial skin reconstruction. Bioact. Mater., 560695. doi:10.1101/2023.10.03.560695
Kim, M. H., Banerjee, D., Celik, N., and Ozbolat, I. T. (2022). Aspiration-assisted freeform bioprinting of mesenchymal stem cell spheroids within alginate microgels. Biofabrication 14, 024103. doi:10.1088/1758-5090/ac4dd8
Langer, R., and Vacanti, J. P. (1993). Tissue engineering. Science 260, 920–926. doi:10.1126/science.8493529
Leung, C. M., de Haan, P., Ronaldson-Bouchard, K., Kim, G.-A., Ko, J., Rho, H. S., et al. (2022). A guide to the organ-on-a-chip. Nat. Rev. Methods Prim. 2, 33–29. doi:10.1038/s43586-022-00118-6
Li, H., Iyer, K. S., Bao, L., Zhai, J., and Li, J. J. (2023). Advances in the development of granular microporous injectable hydrogels with non-spherical microgels and their applications in tissue regeneration. Adv. Healthc. Mater. 2301597, e2301597. doi:10.1002/adhm.202301597
Li, J., and Mooney, D. J. (2016). Designing hydrogels for controlled drug delivery. Nat. Rev. Mater 1, 16071. doi:10.1038/natrevmats.2016.71
Ligorio, C., and Mata, A. (2023). Synthetic extracellular matrices with function-encoding peptides. Nat. Rev. Bioeng. 1, 518–536. doi:10.1038/s44222-023-00055-3
Liu, A. P., Appel, E. A., Ashby, P. D., Baker, B. M., Franco, E., Gu, L., et al. (2022). The living interface between synthetic biology and biomaterial design. Nat. Mat. 21, 390–397. doi:10.1038/s41563-022-01231-3
Liu, X., Smith, L. A., Hu, J., and Ma, P. X. (2009). Biomimetic nanofibrous gelatin/apatite composite scaffolds for bone tissue engineering. Biomaterials 30, 2252–2258. doi:10.1016/j.biomaterials.2008.12.068
Loh, Q. L., and Choong, C. (2013). Three-dimensional scaffolds for tissue engineering applications: role of porosity and pore size. Tissue Eng. Part B Rev. 19, 485–502. doi:10.1089/ten.teb.2012.0437
Ma, P. X., and Zhang, R. (1999). Synthetic nano-scale fibrous extracellular matrix. J. Biomed. Mat. Res. 46, 60–72. doi:10.1002/(SICI)1097-4636(199907)46:1<60::AID-JBM7>3.0.CO;2-H
Mantha, S., Pillai, S., Khayambashi, P., Upadhyay, A., Zhang, Y., Tao, O., et al. (2019). Smart hydrogels in tissue engineering and regenerative medicine. Materials 12, 3323. doi:10.3390/ma12203323
Martins, I. M., Reis, R. L., and Azevedo, H. S. (2016). Phage display technology in biomaterials engineering: progress and opportunities for applications in regenerative medicine. ACS Chem. Biol. 11, 2962–2980. doi:10.1021/acschembio.5b00717
McCormack, A., Highley, C. B., Leslie, N. R., and Melchels, F. P. W. (2020). 3D printing in suspension baths: keeping the promises of bioprinting afloat. Trends Biotechnol. 38, 584–593. doi:10.1016/j.tibtech.2019.12.020
Mills, C. (2012). M1 and M2 macrophages: oracles of health and disease. Crit. Rev. Immunol. 32, 463–488. doi:10.1615/CritRevImmunol.v32.i6.10
Moncal, K. K., Gudapati, H., Godzik, K. P., Heo, D. N., Kang, Y., Rizk, E., et al. (2021). Intra-operative bioprinting of hard, soft, and hard/soft composite tissues for craniomaxillofacial reconstruction. Adv. Funct. Mater. 31, 2010858. doi:10.1002/adfm.202010858
Muir, V. G., Qazi, T. H., Shan, J., Groll, J., and Burdick, J. A. (2021). Influence of microgel fabrication technique on granular hydrogel properties. ACS Biomater. Sci. Eng. 7, 4269–4281. doi:10.1021/acsbiomaterials.0c01612
Muschler, G. F., Nakamoto, C., and Griffith, L. G. (2004). Engineering principles of clinical cell-based tissue engineering. J. Bone Jt. Surgery-American 86, 1541–1558. doi:10.2106/00004623-200407000-00029
Nam, S. Y., Ricles, L. M., Suggs, L. J., and Emelianov, S. Y. (2015). Imaging strategies for tissue engineering applications. Tissue Eng. Part B Rev. 21, 88–102. doi:10.1089/ten.teb.2014.0180
Nichol, J. W., and Khademhosseini, A. (2009). Modular tissue engineering: engineering biological tissues from the bottom up. Soft Matter 5, 1312. doi:10.1039/b814285h
Nunes, C. R., Simske, S. J., Sachdeva, R., and Wolford, L. M. (1997). Long-term ingrowth and apposition of porous hydroxylapatite implants. J. Biomed. Mat. Res. 36, 560–563. doi:10.1002/(SICI)1097-4636(19970915)36:4<560::AID-JBM15>3.0.CO;2-E
Qazi, T. H., and Burdick, J. A. (2021). Granular hydrogels for endogenous tissue repair. Biomaterials Biosyst. 1, 100008. doi:10.1016/j.bbiosy.2021.100008
Riley, L., Schirmer, L., and Segura, T. (2019). Granular hydrogels: emergent properties of jammed hydrogel microparticles and their applications in tissue repair and regeneration. Curr. Opin. Biotechnol. 60, 1–8. doi:10.1016/j.copbio.2018.11.001
Roberts, S. F., Fischhoff, M. A., Sakowski, S. A., and Feldman, E. L. (2012). Perspective: transforming science into medicine how clinician–scientists can build bridges across research’s “valley of death.”. Acad. Med. 87, 266–270. doi:10.1097/ACM.0b013e3182446fa3
Rowley, J. A., Madlambayan, G., and Mooney, D. J. (1999). Alginate hydrogels as synthetic extracellular matrix materials. Biomaterials 20, 45–53. doi:10.1016/s0142-9612(98)00107-0
Rowley, J. A., and Mooney, D. J. (2002). Alginate type and RGD density control myoblast phenotype. J. Biomed. Mat. Res. 60, 217–223. doi:10.1002/jbm.1287
Ryan, G., Pandit, A., and Apatsidis, D. (2006). Fabrication methods of porous metals for use in orthopaedic applications. Biomaterials 27, 2651–2670. doi:10.1016/j.biomaterials.2005.12.002
Salzlechner, C., Haghighi, T., Huebscher, I., Walther, A. R., Schell, S., Gardner, A., et al. (2020). Adhesive hydrogels for maxillofacial tissue regeneration using minimally invasive procedures. Adv. Healthc. Mater. 9, 1901134. doi:10.1002/adhm.201901134
Sari, B., Isik, M., Eylem, C. C., Bektas, C., Okesola, B. O., Karakaya, E., et al. (2022). Omics technologies for high-throughput-screening of cell–biomaterial interactions. Mol. Omics 18, 591–615. doi:10.1039/D2MO00060A
Schwartz, J. G., Kumar, U. N., Azagury, D. E., Brinton, D. J., and Yock, P. G. (2016). Needs-based innovation in cardiovascular medicine. JACC: Basic Transl. Sci. 1, 541–547. doi:10.1016/j.jacbts.2016.06.011
Shen, M., Wang, L., Gao, Y., Feng, L., Xu, C., Li, S., et al. (2022). 3D bioprinting of in situ vascularized tissue engineered bone for repairing large segmental bone defects. Mater. Today Bio 16, 100382. doi:10.1016/j.mtbio.2022.100382
Shi, H., Shi, Q., Grodner, B., Lenz, J. S., Zipfel, W. R., Brito, I. L., et al. (2020). Highly multiplexed spatial mapping of microbial communities. Nature 588, 676–681. doi:10.1038/s41586-020-2983-4
Sittinger, M., Hutmacher, D. W., and Risbud, M. V. (2004). Current strategies for cell delivery in cartilage and bone regeneration. Curr. Opin. Biotechnol. 15, 411–418. doi:10.1016/j.copbio.2004.08.010
Skylar-Scott, M. A., Uzel, S. G. M., Nam, L. L., Ahrens, J. H., Truby, R. L., Damaraju, S., et al. (2019). Biomanufacturing of organ-specific tissues with high cellular density and embedded vascular channels. Sci. Adv. 5, eaaw2459. doi:10.1126/sciadv.aaw2459
Smith, L. A., Liu, X., Hu, J., and Ma, P. X. (2010). The Enhancement of human embryonic stem cell osteogenic differentiation with nano-fibrous scaffolding. Biomaterials 31, 5526–5535. doi:10.1016/j.biomaterials.2010.03.065
Smith, L. A., Liu, X., Hu, J., Wang, P., and Ma, P. X. (2009). Enhancing osteogenic differentiation of mouse embryonic stem cells by nanofibers. Tissue Eng. Part A 15, 1855–1864. doi:10.1089/ten.tea.2008.0227
Swanson, W. B., and Ma, P. X. (2020). “1.4.7 - textured and porous biomaterials,” in Biomaterials science. Editors W. R. Wagner, S. E. Sakiyama-Elbert, G. Zhang, and M. J. Yaszemski Fourth Edition (Academic Press), 601–622. doi:10.1016/B978-0-12-816137-1.00039-8
Swanson, W. B., Omi, M., Woodbury, S. M., Douglas, L. M., Eberle, M., Ma, P. X., et al. (2022). Scaffold pore curvature influences μsc fate through differential cellular organization and YAP/TAZ activity. IJMS 23, 4499. doi:10.3390/ijms23094499
Swanson, W. B., Omi, M., Zhang, Z., Nam, H. K., Jung, Y., Wang, G., et al. (2021). Macropore design of tissue engineering scaffolds regulates mesenchymal stem cell differentiation fate. Biomaterials 272, 120769. doi:10.1016/j.biomaterials.2021.120769
Swanson, W. B., Zhang, Z., Xiu, K., Gong, T., Eberle, M., Wang, Z., et al. (2020). Scaffolds with controlled release of pro-mineralization exosomes to promote craniofacial bone healing without cell transplantation. Acta Biomater. 118, 215–232. doi:10.1016/j.actbio.2020.09.052
Taylor, D. P., Yoshida, M., Fuller, K., Giannobile, W. V., Sfeir, C. S., Wagner, W. R., et al. (2021). Translating dental, oral, and craniofacial regenerative medicine innovations to the clinic through interdisciplinary commercial translation architecture. J. Dent. Res. 100, 1039–1046. doi:10.1177/00220345211009502
Tuftee, C., Alsberg, E., Ozbolat, I. T., and Rizwan, M. (2023). Emerging granular hydrogel bioinks to improve biological function in bioprinted constructs. Trends Biotechnol. doi:10.1016/j.tibtech.2023.09.007
Uman, S., Dhand, A., and Burdick, J. A. (2020). Recent advances in shear-thinning and self-healing hydrogels for biomedical applications. J Appl. Polym. Sci 137, 48668. doi:10.1002/app.48668
Vining, K. H., and Mooney, D. J. (2017). Mechanical forces direct stem cell behaviour in development and regeneration. Nat. Rev. Mol. Cell Biol. 18, 728–742. doi:10.1038/nrm.2017.108
Wang, S., and Larina, I. V. (2017). “8 - high-resolution imaging techniques in tissue engineering,” in Monitoring and evaluation of biomaterials and their performance in vivo. Editor R. J. Narayan (Woodhead Publishing. Sawston, United Kingdom), 151–180. doi:10.1016/B978-0-08-100603-0.00008-0
Whitehead, J., Griffin, K. H., Gionet-Gonzales, M., Vorwald, C. E., Cinque, S. E., and Leach, J. K. (2021). Hydrogel mechanics are a key driver of bone formation by mesenchymal stromal cell spheroids. Biomaterials 269, 120607. doi:10.1016/j.biomaterials.2020.120607
Woo, K. M., Chen, V. J., and Ma, P. X. (2003). Nano-fibrous scaffolding architecture selectively enhances protein adsorption contributing to cell attachment. J. Biomed. Mater. Res. 67A, 531–537. doi:10.1002/jbm.a.10098
Wu, D. T., Diba, M., Yang, S., Freedman, B. R., Elosegui-Artola, A., and Mooney, D. J. (2022a). Hydrogel viscoelasticity modulates migration and fusion of mesenchymal stem cell spheroids. Bioeng. Transl. Med. 8, e10464. doi:10.1002/btm2.10464
Wu, D. T., Jeffreys, N., Diba, M., and Mooney, D. J. (2022b). Viscoelastic biomaterials for tissue regeneration. Tissue Eng. Part C Methods 28, 289–300. doi:10.1089/ten.TEC.2022.0040
Wu, M., and Briant, P. (2012). “The use of finite element analysis in design, Life prediction, and failure analysis of biomaterials and medical devices,” in Degradation of implant materials. Editor N. Eliaz (New York, NY: Springer), 449–461. doi:10.1007/978-1-4614-3942-4_16
Yamada, K. M., and Sixt, M. (2019). Mechanisms of 3D cell migration. Nat. Rev. Mol. Cell Biol. 20, 738–752. doi:10.1038/s41580-019-0172-9
You, S., Xiang, Y., Hwang, H. H., Berry, D. B., Kiratitanaporn, W., Guan, J., et al. (2023). High cell density and high-resolution 3D bioprinting for fabricating vascularized tissues. Sci. Adv. 9, eade7923. doi:10.1126/sciadv.ade7923
Keywords: biomaterials, tissue engineering, mechanobiology, mechanoregeneration, scaffold architecture, 3D bioprinting, hydrogels, spatial biology
Citation: Rangel R, Swanson WB and Wu DT (2024) The future of cell-instructive biomaterials for tissue regeneration–a perspective from early career clinician-scientists. Front. Mater. 10:1328904. doi: 10.3389/fmats.2023.1328904
Received: 27 October 2023; Accepted: 29 December 2023;
Published: 16 January 2024.
Edited by:
Babatunde Okesola, University of Nottingham, United KingdomReviewed by:
Cosimo Ligorio, University of Nottingham, United KingdomCopyright © 2024 Rangel, Swanson and Wu. This is an open-access article distributed under the terms of the Creative Commons Attribution License (CC BY). The use, distribution or reproduction in other forums is permitted, provided the original author(s) and the copyright owner(s) are credited and that the original publication in this journal is cited, in accordance with accepted academic practice. No use, distribution or reproduction is permitted which does not comply with these terms.
*Correspondence: David T. Wu, RGF2aWRUX1d1QGhzZG0uaGFydmFyZC5lZHU=