- 1Ningbo Cigarette Factory, China Tobacco Zhejiang Industrial Co., Ltd., Ningbo, China
- 2Department of Civil Engineering, Zhejiang University, Hangzhou, China
- 3Faculty of Mechanical Engineering and Mechanics, Ningbo University, Ningbo, China
- 4Technology Center, China Tobacco Zhejiang Industrial Co., Ltd., Hangzhou, China
A wide variety of abundant plant leaves exist in nature, and the wettability of their surfaces is formed to adapt to diverse external environments. In this paper we will focus on the factors influencing the wettability of various plant leaves prevalent in nature. And we hope to investigate the interfacial problems of plants from a mechanical point of view. It is found that there are many factors affecting the surface wettability of leaves, such as chemical composition, surface microstructures, hierarchical structures, and growth age. Different influencing factors have different contributions to the change of surface wettability. The surface wax composition influences the surface wettability from a chemical point of view while the hierarchical structure consisting of nanostructures and micron structures also influences the wettability from a structural point of view. Also as the growth age of the plant increases, there is a combined effect on the chemical composition and microstructure of the leaves. Then we discuss the surface/interface mechanics of droplets on various plant leaves and analyze the wetting properties of droplets on different substrates. Finally, we hope that the surface/interface mechanics of plant leaves may be systematically utilized in the future for the preparation of multifunctional biomimetic materials, realizing the crossover of chemistry, biology, mechanics, and other materials science fields.
1 Introduction
Nature is full of diverse hydrophilic and hydrophobic surfaces, which are determined by measuring the contact angle. The contact angle is defined by the geometry of water on a flat surface-specifically, which is the angle between a droplet and the surface near contact line. Plant leaves exhibit varying hydrophilic and hydrophobic characteristics by improving their own structures to adapt to diverse living environments, and over time a specific structural system of superiority and inferiority has developed.
In our daily life, a variety of wetting behavior exist on the surface of plants, as shown in Figure 1. For example, the surface of the lotus leaf is a superhydrophobic material (Yu et al., 2007; Bai et al., 2018; Yun et al., 2020; Ghasemlou et al., 2021). Droplets can roll freely on its surface and effectively remove pollutants. The rose, a more common plant around us, utilizes its microstructure to allow droplets penetrate into it, thereby increasing the contact area and improving the contact angle hysteresis effect (Bhushan, 2018; Chen et al., 2019; Dai et al., 2019; Zong et al., 2019; Kang et al., 2021). Other hydrophobic leaf surfaces are taro leaves (Huang et al., 2016), miscanthus (Chen et al., 2022), gypsum (Kayabaş and Yildirim, 2022), banana leaves (Kayabaş and Yildirim, 2022), and pitcher plant peristome (Miguel et al., 2018; Li et al., 2020). Also, in nature there are many plants that exhibit hydrophilic properties. For example, in some arid environments, some plants have to keep their leaves wet in order to minimize water loss and reduce gas exchange, such as bromeliads, mosses, lichens, chrysanthemum leaves, and abutilon (Koch and Barthlott, 2009).
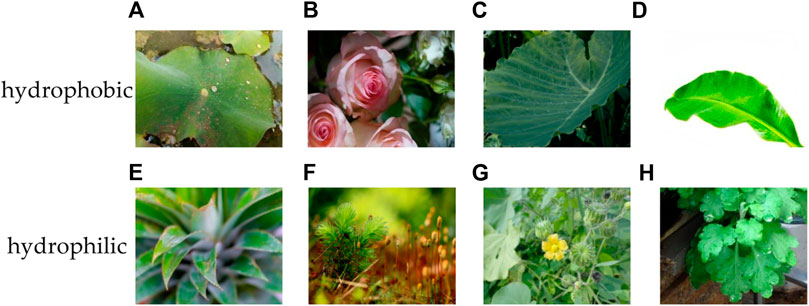
FIGURE 1. Plant leaves commonly found in nature: (A) Lotus leaf; (B) Rose; (C) Taro leaf; (D) Banana leaf; (E) Bromeliads leaf; (F) Moss; (G) Abutilon leaf; (H) Chrysanthemum leaf.
Nowadays, more and more researchers are dedicating themselves to exploring the applications of the wettability of plant surfaces in various engineering fields, such as engineering, biology, and vehicle (Maharbiz et al., 2004; Patel et al., 2010; Flores-Vivian et al., 2013; Moradi et al., 2016; Zhao et al., 2018; Han et al., 2019; Wang et al., 2021a; Ge-Zhang et al., 2022). For instance, plant surface coatings can be used in civil engineering for waterproofing structures which reduces the erosion of the structure and achieves stability in Figure 2A (Flores-Vivian et al., 2013; Zhao et al., 2018; Wang et al., 2021a). These coatings also have important implications for biomedical engineering such as the prevention of blood coagulation in catheters therapy enabled by their hydrophobic property in Figure 2B (Moradi et al., 2016; Han et al., 2019). Additionally, in the field of vehicle engineering, as shown in Figure 2C, anti-fogging surfaces have been designed to increase visibility in fogging weather by taking advantage of the hydrophobic nature of plants in (Maharbiz et al., 2004; Patel et al., 2010; Ge-Zhang et al., 2022).
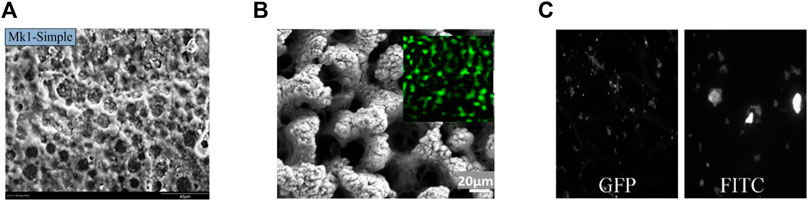
FIGURE 2. Surface imaging in some fields: (A) Surface structure of concrete superhydrophobic coatings. Adapted with permission (Flores-Vivian et al., 2013). Copyright 2013, American Chemical Society. (B) Surface imaging of the adhesion of platelet. Adapted with permission (Moradi et al., 2016). Copyright 2016, American Chemical Society. (C) Structure of superhydrophobic surface after treatment in green fluorescent protein (GFP) and fluorescein isothiocyanate (FITC) solutions. Adapted with permission (Patel et al., 2010). Copyright 2010, Elsevier.
The investigation and application of the wetting properties of plant surfaces have been a prominent area of research in recent decades. To present a comprehensive investigation on the wetting properties of plant surfaces, this review is structured in the following order: Session 2 focuses on the influencing factors affecting the wettability of plant surfaces, including the surface chemical composition, surface microstructure, hierarchical structure, and growth age; Session 3 introduces the surface/interface mechanics of droplets on rigid substrates, fluid substrates and soft solid substrates, and reviews the related studies for wettability on different substrates; Session 4 discusses the relationship between the wettability of plant leaves and the property of anti-soil; Finally, it summarizes the main conclusions and outlooks of this paper.
2 Factors effecting the wetting properties of plant surfaces
The wetting behavior of droplets on different surfaces varies due to many factors. Some droplets will roll on the leaf surface, while others will adhere to the leaf surface. Here, we will review the factors affecting the wetting properties of the leaf surface in three aspects, namely, chemical composition, surface microstructure, hierarchical structure, and growth age.
2.1 Chemical composition
Leaves are composed of many rich chemical components. These chemical components play a crucial role in the wettability of the plant surface. The selection and arrangement of chemical groups exert a great influence on plant properties that cannot be ignored.
2.1.1 Surface wax composition
The cuticle, which contains surface wax, typically forms the outermost layer of plant leaves. The hydrophobic properties of surface wax indirectly determine the wetting characteristics of plant leaves. The compositions of chemical components directly affect the hydrophobicity of the surface wax, such as alkanes, alkyl esters, secondary alcohols, primary alcohols, fatty acids, alkyl ketones, diketones, and alky aldehydes (Holloway, 1969). From Figure 3, we can see the various wax components contained on the different surfaces. Combination of different chemical groups can significantly change the hydrophobic properties of leaf surface (York et al., 2019; Saubade et al., 2021). Among the different chemical components, alkanes exhibit the best hydrophobic effects (Holloway, 1969; Holloway, 1970). An experimental investigation was conducted to assess the effect of adding different natural wax components to the surface of plant leaves on surface wettability (Cheng et al., 2023), such as beeswax, candelilla wax, and carnauba wax. The contact angle of water droplets decreased sharply when the surface vegetable wax was removed (Yamamoto et al., 2015). Therefore, a comprehensive wetting model was developed to predict the wetting behavior of different leaf surfaces (Nairn et al., 2011; Nairn et al., 2022). Then, an approach was proposed for fine-tuning of surface properties to achieve specific wetting objectives.
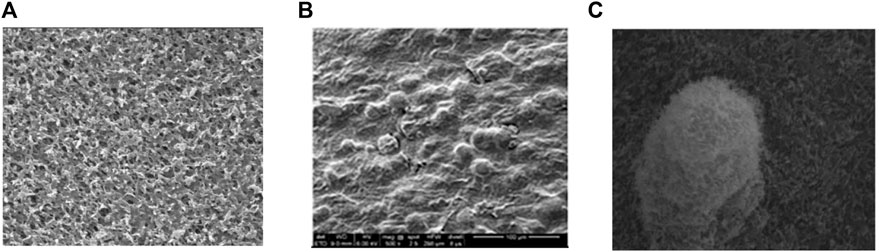
FIGURE 3. Various wax components contained on different surfaces: (A) Single dip carnauba wax. Adapted with permission (York et al., 2019). Copyright 2019, Elsevier. (B) Surface morphology of Starch/gelatin films. Adapted with permission (Cheng et al., 2023). Copyright 2022, Elsevier. (C) Natural lotus leaf surface wax composition. Adapted with permission (Yamamoto et al., 2015). Copyright 2015, American Chemical Society.
By modifying the composition of wax, it is possible to enhance wetting properties and make them suitable for various practical applications. The addition of specific chemical compositions, such as natural wax and Tween-80 (Muscat et al., 2013), hydrocarbon compounds (Liao et al., 2013), hexadecyltrimethoxysilane and octadecyltrichlorosilane (Tan et al., 2021), lipid polymers (Cheng et al., 2021), and fluorine-based polymers (Lee et al., 2021a), can effectively modify the properties of surface morphology and topology. As shown in Figure 4, the contact angle significantly changed over time with the addition of different surface wax compositions. In Figure 4A, as silver nanoparticles increased, the contact angle increased for both integrally modified poly (vinylidene fluoride) (I-PVDF) membranes and surface-modified poly (vinylidene fluoride) (S-PVDF) membranes. In Figure 4B, we find that with the addition of different physical state lipids, the hydrophobicity of the film was improved. Similarly, the contact angle decreases with time in Figure 4C. The increasing hydrophobicity of the structural surface was attributed to the addition of different wax compositions. The anti-pollution and lubrication properties of TiO2 electrospinning film were improved by adding fluorosilanes in the process of preparation (Guo et al., 2019). Furthermore, the stability of films could be achieved by adding 8 vinyl-grafted polyhedral oligosilicone (Ju et al., 2020). To verify the effectiveness of adding chemical components in improving surface properties, molecular dynamics simulations were employed to study the adsorption on the surface and analyzed the effects of surface active molecules on the hydrophobicity of surface (Staniscia et al., 2022).
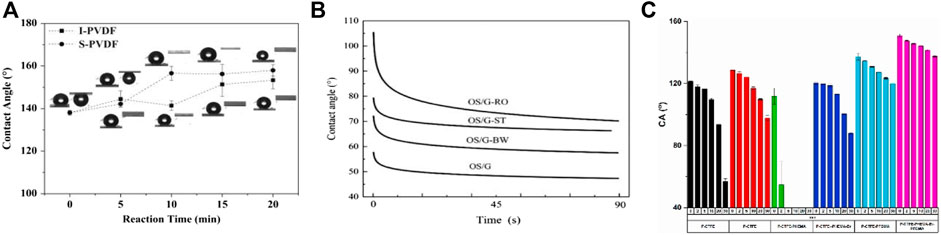
FIGURE 4. Change of contact angles with the addition of different surface wax compositions over time: (A) Variation of contact angles of the modified film with reaction time. Surface and integral properties were modified by silver nanoparticles. Adapted with permission (Liao et al., 2013). Copyright 2013, Elsevier. (B) Changes in contact angles due to the addition of different physical state lipids [rapeseed oil (RO), shortening (ST), beeswax (BW)] in hydroxypropyl starch (OS)/gelatin (G) blown films. Adapted with permission (Cheng et al., 2021). Copyright 2021, Elsevier. (C) Variation of contact angles of a poly (vinylidene fluoride-co-chlorotrifluoroethylene) (PVDF-CTFE) membrane with time when exposed to deionized water. Adapted with permission (Lee et al., 2021a). Copyright 2021, Elsevier.
2.1.2 Arrangement of chemical groups
The reorientation of chemical groups is also responsible for the change in contact angles between droplets and surfaces. Reorientation of the polar chemical groups could lead to a dramatic decrease in the contact angle of the surface, resulting in a surface that exhibits higher hydrophilicity (Wiącek, 2015; Amirabadi et al., 2020; Huth et al., 2022). This effect was particularly evident in the contact angle and surface tension. The changes in the orientation of polar and nonpolar groups can be achieved by cold pkasma treatment (Hashemi Gahruie et al., 2022). Additionally, the prolonged exposure of chemical groups can improve wettability (Liu et al., 2015; Mao et al., 2021; Kalaivendan et al., 2023; Qu et al., 2023), leading to a sudden change in contact angles. In practical applications, adding tea polyphenols to active edible films could effectively reduce the hydrophilicity of the surface, primarily due to the film being covered by hydrophilic groups (Dou et al., 2018). Argon (Ledari et al., 2020) and fluorine (Oberlintner et al., 2022) were also introduced to modify the surface microstructural characteristics, resulting in the appearances and arrangements of relevant chemical groups. The introduction of argon achieved a decrease in contact angle and an increase in hydrophilicity. However, the increase of fluorine achieved a rapid transition from hydrophilic to hydrophobic.
Under the effect of external environments, the presence of dust affects the structure of the wax layer and chemical composition, which in turn has a non-negligible effect on the wettability of the blade (Neinhuis and Barthlott, 1998; Wang et al., 2013; Barima et al., 2016). Over time, dust accumulates further on the surface, changing the roughness of the blade surface (Zhang et al., 2020; Andrade et al., 2022).
2.2 Surface microstructure
The image of microscope reveals the presence of a rich surface morphology on the leaf surface. Then microscopic textures can play a significant role in hydrophobicity. NEINHUIS and BARTHLOTT (1997) studied the micromorphological characteristics of two hundred hydrophobic plant surfaces and analyzed their functions in terms of anti-adhesion and self-cleaning. Under the impact of drizzle, the dust on the surface was gradually dislodged (Hassan et al., 2020) for the purpose of cleaning the surface, which also depends on the adhesion between the dust and the blades. It is evident that the surface morphology plays an important role in the wetting state of a structure. This section reviews the influence of the shape and arrangement of leaf surface papillae on the properties of surfaces.
2.2.1 Shape of papillae
Through numerous simulations, it has been observed that different shapes of papillae significantly affect the wetting properties and states of the structure. Various types of rough surfaces are analyzed to study the wettability of surfaces, including a cylinder, a truncated cone, a paraboloid, a hemisphere (Bittoun and Marmur, 2009), and columnar microstructures (Xu et al., 2022). Among them, parabolic protrusions have been found to achieve the best superhydrophobic property (Bittoun and Marmur, 2009). Besides, the effects of different papillae shapes on surface wettability were analyzed using both two-dimensional and three-dimensional models as shown in Figure 5. In a 2-D model, the transition of surface microstructures from upright cone to inverted cone surfaces was analyzed by using a free-energy approach to study the wetting state of droplets on different surfaces (Figure 5A) (Ou et al., 2019). In 3-D models, regular and irregular microstructures (He et al., 2016; He et al., 2018; Jiang et al., 2020; Sui et al., 2022) were investigated (Figures 5B–D). With different initial conditions, such as higher apparent contact angle or higher critical pressure, different microstructures could achieve different wetting results (Xu et al., 2022). The substrate was also modeled as a conical surface, establishing a relationship between the microstructure of the conical surface and the contact angle of the three-phase contact line (Sui et al., 2020). Changes in the substrate angle of conical surface corresponded to the changes in the wetting state. To improve the stability of the wetting state, the radius of the protrusion (Sui et al., 2022) or the radius of curvature of the top (Wang et al., 2021b) can be increased appropriately. In a word, both contact angle and wetting area are heavily dependent on the morphology of the substrate. By adjusting the shape of papillary microcolumns, wettability can be achieved and controlled for interactions of droplets and substrates.
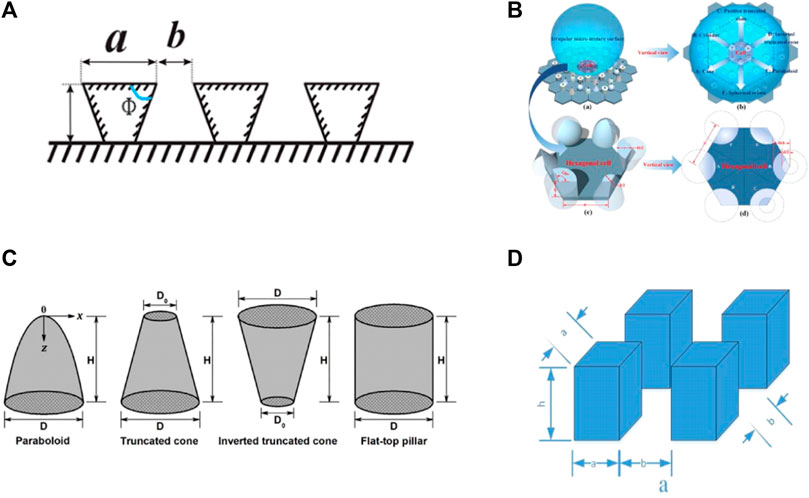
FIGURE 5. Different papillae shapes: (A) The inverted trapezoidal surface. Adapted with permission (Ou et al., 2019). Copyright 2019, American Chemical Society. Copyright 2020, Elsevier. (B) Irregular three-dimensional rough microstructure. Adapted with permission (Sui et al., 2022). Copyright 2022, Elsevier. (C) Four different papillae shapes (paraboloid, truncated cone, inverted truncated cone, and flat-top pillar shapes). Adapted with permission (Jiang et al., 2020). (D) Rectangular surface microstructures. Adapted with permission (He et al., 2016). Copyright 2016, Elsevier.
2.2.2 Arrangement of papillae
The regular or irregular distributions of papillae greatly affect the wettability of the surface. Numerical simulations and experiments have been conducted to analyze the effects of the morphology and distribution of the microstructures on hydrophobic surfaces (Haj Ibrahim et al., 2022). Randomly textured surfaces (Khandoker and Golovin, 2020) and regular square lattice arrangement (Marmur, 2004) were analyzed to study the effects of different arrangements in Figure 6. It was found that as the slope and protrusion distance increased, the substrate surface exhibited higher contact angle and meanwhile became more hydrophobic. The relationship between the spacing distance of cylindrical shapes and the transition of the wetting state was also analyzed (Liang et al., 2019). To characterize contact angles and wetting states, contact ratios of surface structures were used (Onda et al., 1996; Shibuichi et al., 1996). By altering the root-mean-square slope of the surface structure, transitions between different wetting states could be achieved (Schiavon et al., 2022). The various arrangements significantly influence the contact behavior and contact area of droplets with the substrate, resulting in distinct wetting effects.
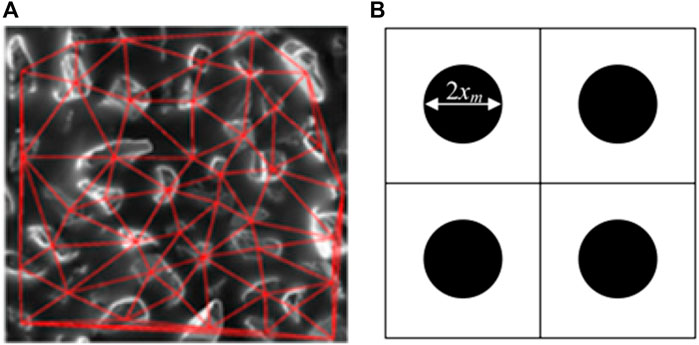
FIGURE 6. Irregular and regular arrangements: (A) Randomly textured surfaces, in which red lines show the triangulation of centroids of grits obtained from SEM image. Adapted with permission (Khandoker and Golovin, 2020). Copyright 2020, American Chemical Society. (B) Regular square lattice arrangement with rotating paraboloidal microstructure form. Adapted with permission (Marmur, 2004). Copyright 2004, American Chemical Society.
2.3 Hierarchical structure
The lotus leave is an exemplary example of hydrophobic plants with a dual-scale structure consisting of 10 µm papillae and a surface wax layer measuring 100 nm. Such micro/nano-scale structure plays an important role in achieving superhydrophobicity on surfaces. Within this hierarchical structure, the microstructure and the nanostructure play different roles. The nanostructure effectively reduces the contact area between the droplet and the substrate surface, while the microstructure increases the pressure difference required for the transitions between different wetting states. Through the combined influence of these two structures, the energy difference is further amplified, ultimately leading to hydrophobicity. The microstructures of the superhydrophobic surface are observed by means of microscopy, field-emission scanning electronic microscopy (Li et al., 2022) and white light interference (Xu et al., 2023). Many irregular-shaped nanoparticles covered the bottom and top of the microgroove area and a lot of submicron-particles adhered to the edges of the platform region (Li et al., 2022). Lotus leaves in Figure 7A also exhibit micron-scale mastoid accumulation on the submillimeter structure (Li et al., 2021). This layered structure ensures the hydrophobicity of the leaf edges and reduces the damage caused by external loads. In Figures 7A, B superhydrophobic surface was created from the petal surface (Abd Aziz et al., 2022) to effectively preventing adhesion of substances such as palm oil and ethylene glycol. Additionally, Figure 7C demonstrates a miro-nano hierarchical structure formed by the nano-scale particles adhering to the surface of papillae structures. The combination of multiple levels and scales of structures has also been found to improve the resistance to contamination (Zhan et al., 2022).
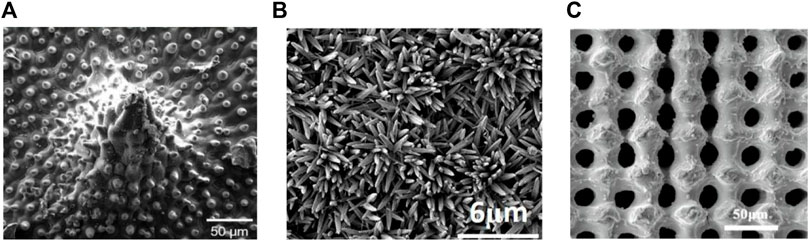
FIGURE 7. Hierarchical structure: (A) Micropapillae of 5–10 µm radius on submillimeter surfaces. Adapted with permission (Li et al., 2021). Copyright 2020, Wiley. (B) SEM imaging of the composite interface on the petal surface. Adapted with permission (Abd Aziz et al., 2022). Copyright 2022, Elsevier. (C) SEM imaging of surface microstructures of micro-nano structured selective laser melting (SLM-NiTi) surface. Adapted with permission (Xu et al., 2023). Copyright 2022, Elsevier.
The wettability and stability of the substrate surface are strongly influenced by the different hierarchical structures, leading to changes in the contact angles of the three-phase contact line (Bhushan et al., 2009) and decrease of contact angle hysteresis (Kim et al., 2013). The hierarchical structure from nanometer to micron plays a crucial role in the hydrophobic properties of the surface, thereby altering the energy difference between the different wetting states (Su et al., 2010; Ebert and Bhushan, 2012). The amplitude of energy difference controls the transition. Secondary nanoscale structure exerts a significant influence on the wetting behavior of the surface (Ahammed et al., 2020; Zheng et al., 2021). By optimizing the design, a smaller solid-liquid base area can be achieved. This resulting layered structure contributes to larger contact angles, thereby increasing the hydrophobicity of surface.
Based on the observed hierarchical structure of superhydrophobic surfaces, an increasing number of manufacturing processes are being implemented in production. As depicted in Figure 8, a hydrophobic film with a dual-scale structure has been developed, significantly improving the resistance of films to contamination (Xiao et al., 2020) and the chemical stability of the surface (Tang et al., 2021). The relationship between microstructure and hydrophobicity has been utilized to effectively repel conidia (Alon et al., 2022). Besides, carbon nanotubes have been implanted on the nickel surface to maintain the superhydrophobicity of the structure (Jung et al., 2022). This approach not only preserves superhydrophobic properties but also enhances the overall stiffness of the structure.
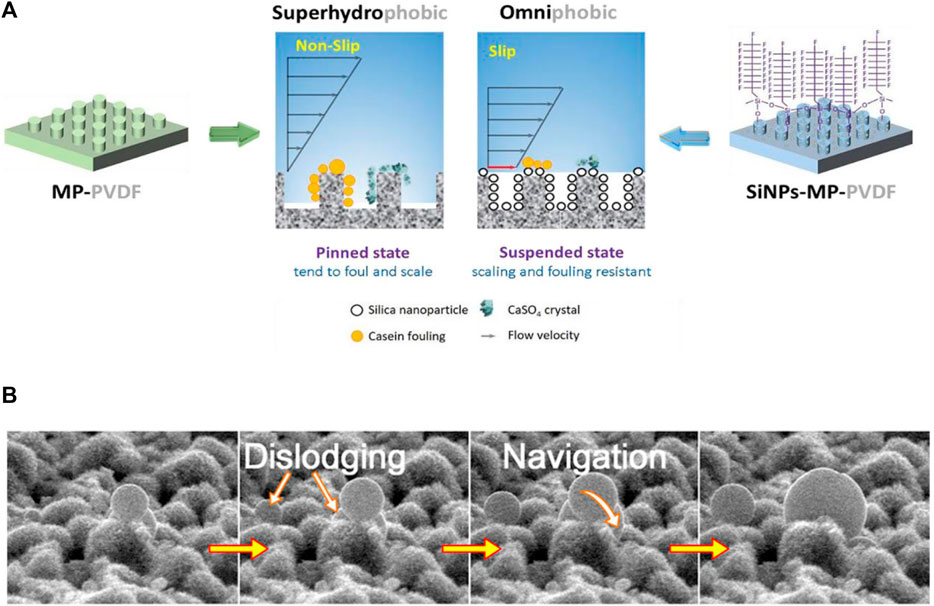
FIGURE 8. Manufacturing process in practice: (A) Bi-scale hydrophobic micro-pillared polyvinylidene (MP-PVDF) and silica nanoparticles polyvinylidene (SiNPs-MP-PVDF) membranes. Adapted with permission (Xiao et al., 2020). Copyright 2020, Elsevier. (B) Layered micromorphology formed by nanograss-covered micropapillae. Adapted with permission (Tang et al., 2021). Copyright 2021, American Chemical Society.
2.4 Growth age
The wettability of the surface of plant leaves changes throughout their growth age. The process of leaf growth and aging is accompanied by changes in surface wax composition and microstructure at different growth stages (Neinhuis and Barthlott, 1998; Fernández et al., 2014; Zhu et al., 2014; Dawson and Goldsmith, 2018).
Taking the lotus leaf as an example, when the lotus leaf is a newly grown young leaf, the droplets on the surface are approximately spherical, in which the mature lotus leaf shows stronger hydrophobicity. However, when the lotus leaves have aged, the droplets on the surface show a hemispherical shape. It could be seen that the contact angle of the senescent leaves was significantly reduced (Sun et al., 2014). Looking closely at the surface microstructure, the hierarchical structure on the surface of senescent leaves was no longer evident and the distributed wax tubes almost disappeared (Gou and Guo, 2019; Losso et al., 2023). Similarly over time, the green rape leaves shrunk and curled, the contact angle gradually decreased, and finally the wettability gradually transitioned from hydrophobic to hydrophilic (Zhu and Guo, 2016).
Top-down contact angle measurements were used to measure Cattleya warneri orchids and Dracaena draco at different ages and it was observed that the older leaves showed hydrophilicity while the younger leaves showed hydrophobicity (Janeczko et al., 2019; Jura-Morawiec and Marcinkiewicz, 2020). Unique leaf structures on the surface of young leaves during their developmental stages, such as various papillae, play an important role in the increase of wettability. For Tea tree leaves, wettability then showed two trends (Gao et al., 2022; Pan et al., 2023). For Maolu leaves, the contact angle decreased and then increased slightly with leaf age. For Zhongcha 108 and Anji Baicha leaves, the contact angle remained basically constant.
For different growing environments, the contact angles of new leaves were higher than those of old leaves in arid areas (Xiong et al., 2018), and decayed leaves were more hydrophilic in urban areas (Wang et al., 2013). In different climatic zones, pear leaves in the early growth period were more hydrophobic than those in the late growth period, attributed to significant changes in surface wax composition (Gao et al., 2018). In addition, reduced surface wax composition allowed summer laurel leaves to also exhibit greater hydrophobicity than fall leaves due to fall climate (Kang et al., 2018). Changes in surface microstructure also influence the alternating changes in wettability of leaves throughout the year. Among them, the change of wettability of deciduous plants was more obvious (Wang et al., 2013; Wang et al., 2023a). It can be seen that plant leaves exhibit different surface wettability at different growth stages, which can help to study the wetting and diffusion behaviors of droplets on the leaf surface according to the different growth stages.
3 Surface/interface mechanics
To investigate the hydrophobic properties of plant surfaces in different states, it is especially critical to study the mechanics of the contact interface. The transfer mechanism at the interface has a significant effect on the properties of material (Wang et al., 2020; Gao et al., 2021). A more comprehensive understanding of the contact wetting problem can be achieved by considering the mechanical aspects. Substrates are generally categorized into rigid, fluid, and soft solid based on their stiffness. The influence of different substrates on the wetting state is also different. In the following section, we will review the wetting behavior of droplets and the deformation of substrates for these three types.
3.1 Contact on a rigid surface
The wettability of rigid solid surfaces has important applications in many fields, such as engineering, life, pharmaceuticals, and energy. The static contact angle is usually used to characterize the wettability of a rigid surface, which is the angle between the solid-liquid interface and the liquid-vapor interface near the three-phase contact line.
For the contact between a droplet and an ideal smooth surface in Figure 9A, the contact angle is usually described by Young’s equation (Young, 1805), and the corresponding equation is as follows:
where

FIGURE 9. Physical model: (A) Young’s model; (B) Wenzel model: the droplet completely fills the microcolumns of the surface and there are no air cavities at the droplet-substrate contact. (C) Cassie model: the droplet does not completely penetrate into the microcolumns of the surface during the wetting process and that air cavities exist with the rough substrate.
In reality, genuinely smooth substrates are rarely encountered. Therefore, two models, namely, the Wenzel model and the Cassie model, have been subsequently developed to describe the interaction between droplets and rough surfaces. Both two models use microcolumns arranged on a smooth substrate to simulate the roughness of the surface. The wetting behavior of droplets on rough surfaces is directly affected by the properties of substrate and array topology.
The first is the Wenzel model (Wenzel, 1936), which assumes that the droplet completely fills the microcolumns on the surface and there are no air cavities at the droplet-substrate contact, as shown in Figure 9B:
where
There is also the Cassie model in Eq. (3) (Cassie and Baxter, 1944), which differs from the Wenzel model by assuming that the droplet does not completely penetrate into microcolumns of the surface during the wetting process and that air cavities exist with the rough substrate in Figure 9C:
where
The study of wettability of droplets on rigid substrates has also become the focus of many researchers in recent years, leading to in-depth discussions from different perspectives. The first is the analysis of the contact angle of droplets on rigid substrates. The variation of contact angle on the three-phase contact line was investigated under the effect of gravitational fields (Ward and Sasges, 1998). In the case of substrates with variable stiffness, the contact angle between a droplet and a substrate has been studied based on the principle of minimum potential energy (Bormashenko, 2018). From Figure 10A, we can find that the cosine of the apparent contact angle increases with the radius of the contact angle under different gradients of the interfacial tensions, in which green line had the largest differences of gradients. Furthermore, the influence of surface tension on contact behavior with randomly rough substrates has been examined to determine the contact area between solids and liquids (Yuan et al., 2018). Under the action of surface tension, smaller droplets tend to be spherical under surface tension because the droplet size is smaller than the capillary length (Meng et al., 2021; Style et al., 2021).
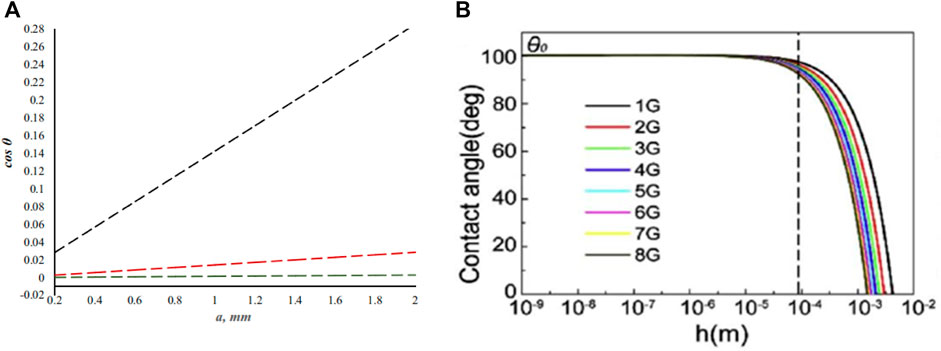
FIGURE 10. Dependence of contact angles: (A) Law of variation of cosine of contact angle with contact radius on variable stiffness substrate. The black dashed line represents the value of difference of gradients of the interfacial tensions is equal to 0.1 N/m2. The red dashed line represents 1 N/m2. The green dashed line represents 10 N/m2. Adapted with permission (Bormashenko, 2018). Copyright 2018, Elsevier. (B) Contact angle at different heights under different gravitational forces. Adapted with permission (Liu et al., 2020). Copyright 2019, Elsevier.
At the same time, there are some studies that derive the droplet profile from the most basic Young–Laplace equation. An analytical expression for the two-dimensional droplet profile on a rigid substrate under gravity has been established (Lv and Shi, 2018). This expression could be extended to an inclined substrate to study the contact angle hysteresis. In this way, solving the Young–Laplace equation can obtain the functional expression of the droplet profile, in order to get the surface tension at the solid-liquid interface (Tang and Cheng, 2022). Moreover, the interface of a droplet on a rough substrate has been derived by using Gibbsian composite-system thermodynamics (Shardt and Elliott, 2019). It is note that the contact angle at the solid-liquid interface is influenced by the contact line.
Indeed, the aforementioned discussions were primarily based on theoretical derivations. However, there are also relevant studies that focus on the experimental aspects. For instance, the contact angles of droplets on smooth and rough substrates were measured using a long-arm hanging basket centrifuge (Liu et al., 2020). Figure 10B shows the contact angles at different heights under different gravitational forces. It has been shown that the acceleration of gravity has a non-negligible effect on the contact angle.
Some relevant studies also focus on the morphology of droplets in various external environments, such as electric (Cheng and Chaddock, 1986; Chen et al., 2007), gravitational (Zeng et al., 2022), noise (Borcia et al., 2019), and thermal fields (Adera et al., 2013; Lee et al., 2021b; Dai et al., 2022). Therefore, we can apply an appropriate external field to regulate the wetting behavior of the droplet, by adjusting the contact angle on a rigid substrate.
As previously mentioned, the existence of droplets on rough substrates is divided into two states, but the transformation and coexistence of droplet states on rough substrates is an urgent problem requiring resolution. From a theoretical point of view, a cross-scale predictive model was developed from the nanoscale to analyze the transition between Cassie and Wenzel states of liquid droplets on a superhydrophobic surface (Wu et al., 2022). From a simulation point of view, the coexistence and transition between Cassie and Wenzel state on a superhydrophobic substrate were analyzed by molecular dynamics simulations (Koishi et al., 2009; Rauter et al., 2021) and a two-dimensional standard Monte Carlo protocol (Lopes et al., 2013). These simulations have investigated the effects of column spacing, height, and contact angle on the state transitions. From an experimental standpoint, the transition between different states on a rough substrate has been studied by compressing droplets between superhydrophobic surface planes (Garcia-Gonzalez et al., 2022) as illustrated in Figure 11A. Besides, in the case of different Wenzel and Cassie droplets depicted in Figure 11B, smaller Wenzel droplets on rough superhydrophobic surfaces typically were merged with larger Cassie droplets, resulting in the formation of larger Cassie droplets (Zhang et al., 2023).
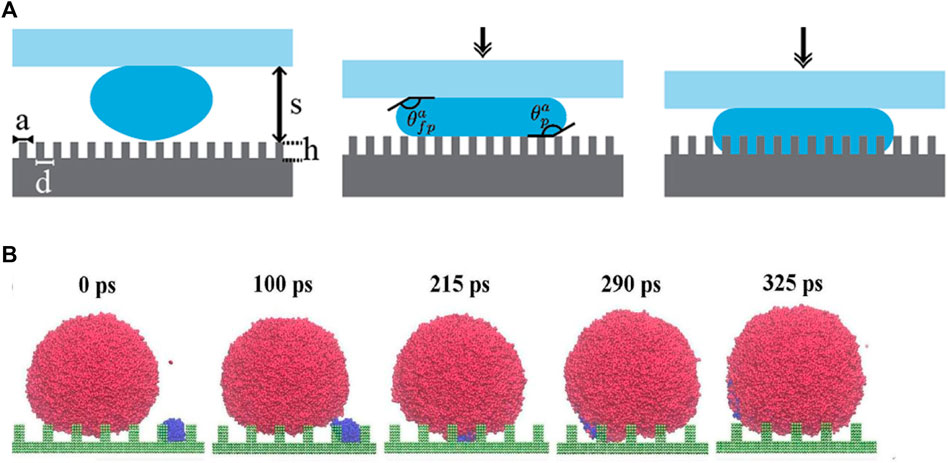
FIGURE 11. The transformation and coexistence of different states: (A) Transition of droplet wetting states on superhydrophobic micropillar arrays. Adapted with permission (Garcia-Gonzalez et al., 2022). Copyright 2022, American Chemical Society. (B) Engulfment of small Wenzel droplets by large Cassie droplets on nanopillars. Adapted with permission (Zhang et al., 2023). Copyright 2022, Elsevier.
Various approaches have been investigated to regulate the transition between the Cassie and Wenzel states. In particular, the transition between different wetting states of nanodroplets on the surface with the columnar array was investigated in the presence of an external electric field (He et al., 2021; He et al., 2022). Figures 12A, B demonstrates that the droplets gradually penetrated into the pores of the substrate over time under the influence of the external electric field. Additionally, the transition between different states could effectively be regulated by applying a vibration field (Wang et al., 2022a). The control of the wetting state could be effectively achieved by adjusting the vibration amplitudes as depicted in Figure 12C.
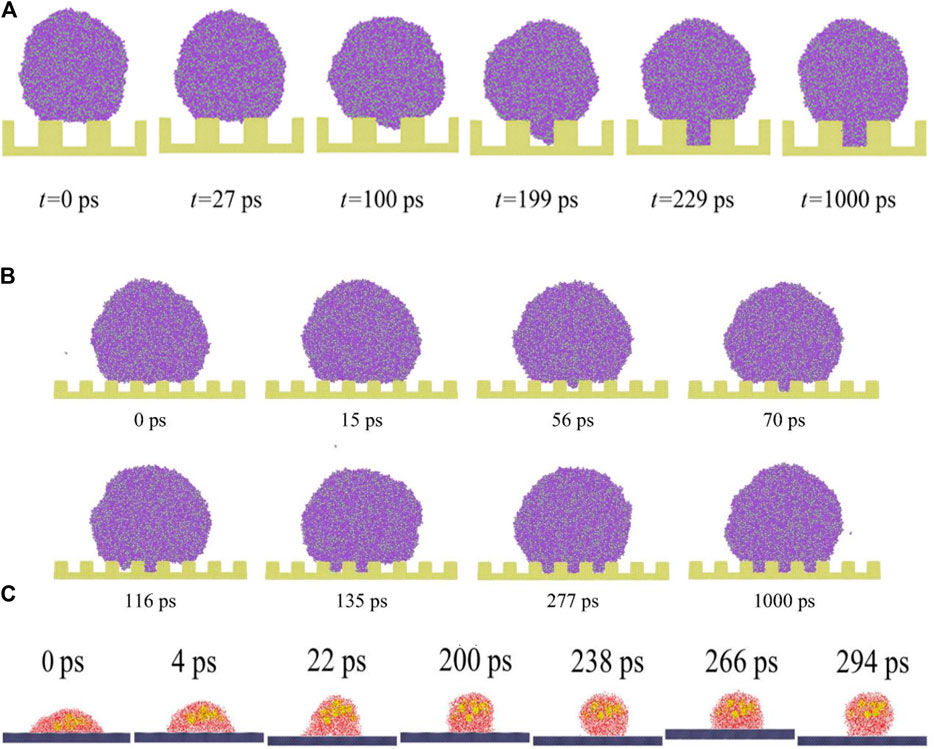
FIGURE 12. Transformation of different states under external fields: (A) Transition of different wetting states under the action of external electric field. Adapted with permission (He et al., 2021). Copyright 2021, American Chemical Society. (B) Morphological evolution of nanodroplets on columnarly arranged surfaces under the action of electric fields. Adapted with permission (He et al., 2022). Copyright 2021, Elsevier. (C) Wetting states of nanofluid droplets on the surface during vibration of substrate. Adapted with permission (Wang et al., 2022a). Copyright 2021, Elsevier.
3.2 Contact on a fluid surface
When a droplet is dropped on another immiscible fluid, a three-phase contact line similar to that of a rigid substrate is also formed. Unlike rigid substrates, fluid substrates are also deformed, forming a fluid lens. The final shape depends on the balance of the surface tensions around the three-phase contact line in Eqs. (4) and (5), also known as Neumann construction (Neumann and Wangerin, 1894):
where
Many studies related to this liquid-liquid floating system have been done from experimental (Burton et al., 2010; Pototsky et al., 2021; Zhao et al., 2023) and theoretical (Phan et al., 2012; Wong et al., 2017; Hack et al., 2020; Ravazzoli et al., 2020; Liu et al., 2021) perspectives. The equilibrium shape of the liquid lens was achieved from experiments, which was attributed to the competition between inertial forces, adhesion forces, and surface tensions. However, the above equilibrium shapes existed simultaneously only when the droplet volume was small (Hack et al., 2020). On the other, the stability profile of water floating on oil with different contact angles was solved, which was mainly controlled by the surface tension, droplet volume, and fluid density. Consider the effect of gravity, two different sets of equilibrium lens shapes were derived by setting different reference pressures (Phan et al., 2012). However, one of them was the easiest to exist in nature due to its minimum energy.
In our daily lives, the most intuitive idea is that heavier fluids sink to the bottom of lighter fluids. This phenomenon can be changed by applying external regulation so that the heavier fluid floats on top of the lighter fluid. The primary factor influencing this behavior is surface tension (Singh et al., 2023). As a result of surface tension, heavier fluids can form a liquid lens that floats on lighter fluids. One approach to achieving this is by applying surfactant on the surface (Phan, 2014). By this way the floating behavior of droplets can be realized with acute contact angle in Figure 13A. Additionally, in Figure 13B, the heavier fluid was enabled to float stably on top of the lighter fluid by applying vertical vibrations (Pototsky et al., 2019). This process involves bidirectional motion of droplets with varying volumes on the oil meniscus, driven by capillary action (Sun and Weisensee, 2023). This novel concept presents an innovative way to control the movement of the liquid-liquid floating system.
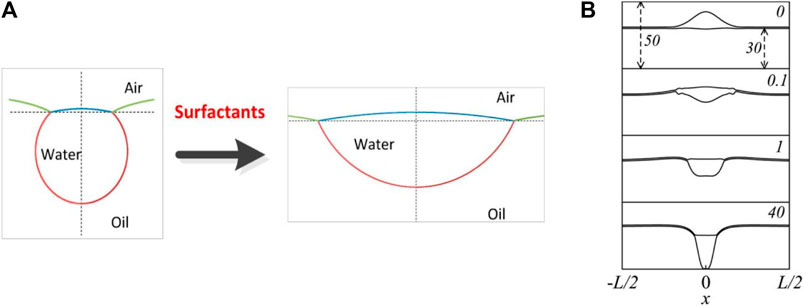
FIGURE 13. Stability of a floating lens under external factors: (A) Liquid-liquid floating system under the action of surfactants. Adapted with permission (Phan, 2014). Copyright 2014, American Chemical Society. (B) Stabilization of vibration-induced floating systems. Adapted with permission (Pototsky et al., 2019). Copyright 2019, AIP Publishing.
All of the above are two kinds of droplets acting on each other, but when the two kinds of droplets come into contact with a rigid substrate, a different phenomenon occurs. Compound droplets are formed mainly by the contact of two or more immiscible droplets. Unlike the liquid-liquid floating system, the volume of both droplets in compound droplets is not significantly different, but rather limited. Due to surface tension, volume, and other factors, compound droplets also exist in a variety of forms. The transition process between different configurations of compound droplets on rigid substrates was analyzed (Neeson et al., 2012), revealing stable and unstable configurations. Experimental measurements of the equilibrium shape of compound sessile droplets were conducted in Figures 14A, B (Zhang et al., 2016; Zhang et al., 2021; Rostami and Auernhammer, 2022). The transition between different equilibrium configurations was mainly influenced by properties of the substrate, contact radius, height, and the volume ratio of the droplets. In addition, a phase field model (Bhopalam et al., 2022) was employed to simulate the elastic capillary phenomenon of compound droplets on a thin elastic solid in Figure 14C.
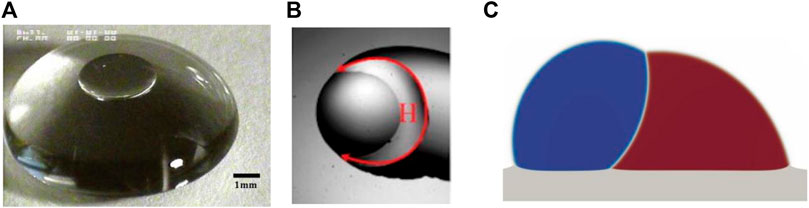
FIGURE 14. Different configurations of compound droplets: (A) Stable configurations of compound droplets from a phase separated mixture of aqueous 22% CsCl solution with benzyl alcohol, density ratio 0.859. Adapted with permission (Zhang et al., 2016). Copyright 2015, Elsevier. (B) Top view of a composite droplet formed by water and bromocycloheptane in contact with each other. Adapted with permission (Rostami and Auernhammer, 2022). Copyright 2022, AIP Publishing. (C) Deformation of a compound droplet on a soft substrate in stable condition. Adapted with permission (Bhopalam et al., 2022). Copyright 2022, Elsevier.
3.3 Contact on a soft surface
The surface/interface mechanics of droplets on rigid and fluid substrates have been previously discussed. It is evident that the elastic modulus of rigid substrates is at the GPa level, while that of fluid substrates is at the Pa level. But when the droplet falls on the substrate at kPa level, what kind of morphology will the droplet and the substrate show?
When a droplet is dropped on a deformable soft solid, the droplet causes deformation of the substrate due to the elasticity of the substrate. Therefore, we need to consider the additional elastic potential energy when calculating the total energy. To simulate the deformation of the elastic substrate, a Winkler foundation model was introduced combining the Derjaguin (disjoining) pressure (Henkel et al., 2021). In Figure 15A the deformation of the elastic substrate under the action of droplets caused by capillary forces was calculated (Liu et al., 2009). Likewise, the wetting behavior of polymer droplets on deformable solids was simulated by molecular dynamics in Figure 15B (Léonforte and Müller, 2011). In addition, many studies have investigated the equilibrium shape of liquid droplets on soft solid substrates (Style and Dufresne, 2012; Bostwick and Daniels, 2013; Style et al., 2013; Schulman and Dalnoki-Veress, 2015; Dervaux et al., 2020; Zhao et al., 2021). The deformation of the elastic substrate was mainly related to the radius of the droplet, surface tension, elastic modulus of the substrates, and viscoelasticity. Another effect of surface strain, i.e., the Shuttleworth effect, was analyzed to study the wetting process of droplets on a viscoelastic substrate (van Gorcum et al., 2020), which may have a significant effect.
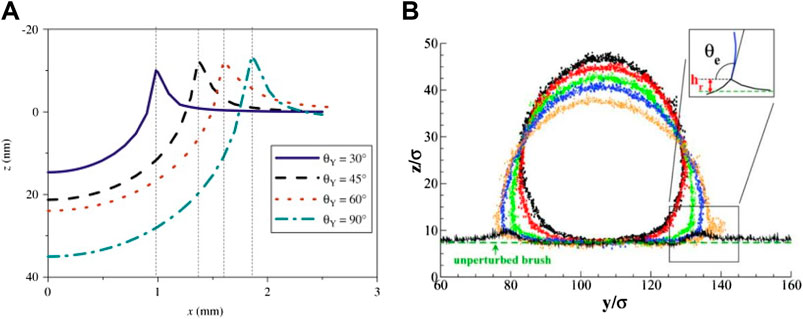
FIGURE 15. Deformation of the elastic substrate under the action of droplets: (A) Total deformation of the substrate at different contact angles. Adapted with permission (Liu et al., 2009). Copyright 2008, Elsevier. (B) Variation of contact ridges of droplets with soft substrates simulated by molecular dynamics under different various compatibility parameters. The black is equal to 0.3 and the orange is equal to 0.8. Adapted with permission (Léonforte and Müller, 2011). Copyright 2011, AIP Publishing.
The wetting behavior of the droplet and the deformation of the substrate can be significantly altered by adjusting the stiffness of the substrate. When the stiffness is increased, the droplet tends to move in the direction of greater stiffness, which is known as Durotaxis. This deformation near the three-phase contact line was independent of the elastic modulus of the substrate (Jerison et al., 2011). Therefore, the main concern is the deformation that occurs away from the three-phase contact line. Numerous studies (Marchand et al., 2012; Charitatos and Kumar, 2021; Khattak et al., 2022; Lai and Li, 2022) have shown that changes in substrate stiffness can result in modifications to the geometry of liquid droplets on soft elastic solids. The relation between the variation of contact angle and substrate stiffness was analyzed on a three-phase contact line. When applying pre-stretch to the substrate (Liang and Li, 2020; Song et al., 2020), the deformation of the elastomer can be calculated by surface Green functions considering effects of gravity and surface tension. As a result, we can control the shape of the wetting ridges formed by the droplets on the soft substrate by regulating the stiffness of the substrate.
The soft substrate not only causes substrate deformation due to liquid droplets, but also generates a corresponding surface strain. Therefore, incorporating surface strain in the correction of surface tension provides a more accurate determination of the substrate morphology. To investigate this process from multiple scales, researchers have explored the variation of contact angle and its connection in the multi-scale model (Lubbers et al., 2014; Henkel et al., 2022; Xie et al., 2022). Multi-scale analysis plays an important role in gaining insights into the linkages between different scales such as macroscopic, mesoscopic, and microscopic scales (Wang et al., 2017; Wang and Pindera, 2017; Chen and Wang, 2019; Wu et al., 2023). The transition of contact angles on soft substrates was analyzed separately using macroscopic and microscopic theories, which consider capillary interaction at different levels (Lubbers et al., 2014). Furthermore, the wetting of droplets on soft substrates was studied by considering the effect of surface strain at macroscopic and mesoscopic scales (Henkel et al., 2022) (see Figure 16). At the macroscopic scale, the neo-Hookean law was employed, while the simpler Winkler foundation model was utilized at the mesoscopic scale, respectively, to describe the behavior of the substrate.
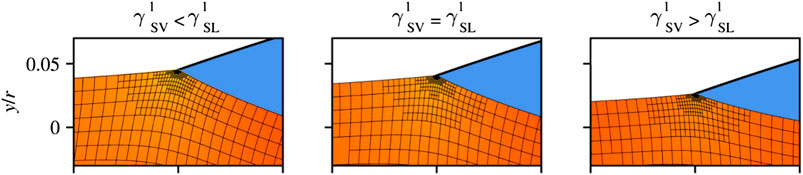
FIGURE 16. The substrate and droplet deformation obtained by considering the surface strain under the macroscopic model under different surface tensions. Adapted with permission (Henkel et al., 2022). Copyright 2011, the Royal Society.
4 Discussion
In this paper, we mainly synthesize the wettability of plant leaves from the chemical and mechanical point of view. Besides there is a relationship between the wettability of plant leaves and the property of anti-soil. This is a complex connection between the wettability of plant leaves and the property of anti-soil, which promote or inhibit each other and jointly affect plant growth and development. Soil resistance of plant leaves refers to the resistance of plants to adverse factors in the soil, which is a comprehensive ability, including drought resistance, cold resistance, salinity resistance and other abilities. Changes in the wettability of plant leaves affect the exchange of air and water between the plant and the outside world, thereby affecting photosynthesis and respiration (Shi et al., 2011; Holder, 2012; Cavallaro et al., 2023). Wettability of the leaf surface can effectively improve water distribution within the plant (Gotsch et al., 2014; Ali et al., 2022), and also inhibit the exchange of gases at the interface, which promotes the growth of pathogens (Sase et al., 2008).
Prolonged exposure to a wide variety of climatic environments leads to an increase in the waxes on the surface of the cuticle, which affects the transport of water at the interface (Chin et al., 2023). Maize leaves in the Sahara Desert, due to their high wettability, favored the accumulation of rainwater and safeguard the transport of water under arid conditions (Revilla et al., 2016). Under drought conditions, plant leaf surface microstructures, such as trichomes, influenced the wettability state of the leaf, thereby controlling water adhesion to the surface (Wang et al., 2023b; Liu et al., 2023). In foggy days, wheat leaves adjusted morphological features to increase the wettability of the leaf surface, which in turn maintains the moisture condition on the surface (Merrium et al., 2022a). In addition adjustment of leaf surface wettability is achieved by optimizing leaf surface structure and promoted droplet pooling and water transport (Merrium et al., 2022b; Hakeem et al., 2023). The modification of plant leaf wettability can be utilized to effectively adjust the water utilization capacity of desert plants and achieve the purpose of drought resistance.
The wettability of plant leaf surfaces can have different effects on the surface icing state, which in turn affects the cold resistance of the leaf. The formation of ice crystals on the leaf surface depends largely on the wax composition and microstructure. It has been found that superhydrophobic surfaces could effectively inhibit the formation of ice nuclei (Gorb and Gorb, 2022; Kumar et al., 2022). The hierarchical structure of the Phyllostachys viridis leaf surface greatly prolonged the freezing time of the droplets (Feng et al., 2023). Utilization of the waxy protrusions of plant leaves reduced the contact area of ice crystals with the substrate and eased the freezing of the leaf surface. Thus plants maintain normal physiological functions by maintaining higher wettability in cold environments, thus enhancing cold tolerance.
In saline and alkaline environments, water evaporates faster and the wettability of leaves can help plants better absorb and utilize the limited water, thus maintaining normal growth and development. The salinity resistance of plants mainly depended on the morphological characteristics and waxy composition of the leaf surface (Du and Hesp, 2020; Wang et al., 2022b; Li et al., 2023). However, excessive uptake could inhibit plant growth and cause damage such as leaf senescence (Mereu et al., 2011). Studies of ornamental plants in coastal areas have found that surface wettability affected salt accumulation (Piccolo et al., 2023). Leaf surfaces that were more wettable (smaller contact angle) were able to take up large amounts of Na+ and Cl−. Magnesium salt uptake was much higher in highly wettable lettuce leaves than in leaves that were extremely non-wettable (Barlas et al., 2023). Leaf wettability also promotes gas exchange and increases the efficiency of photosynthesis and respiration, thereby enhancing the salinity resistance of plants.
The study of the relationship between the wettability of plant leaves and the property of anti-soil is complex and multifaceted and needs further exploration. This paper only starts from a small point and more work is needed in the future.
5 Conclusion
For various plant leaves commonly found in nature, this paper mainly reviews the wettability of their surfaces. The factors that influence the hydrophobicity of plant surfaces are discussed, focusing on their chemical composition, surface microstructure, hierarchical structure, and growth age.
The chemical compositions of leaf surfaces are analyzed for a wide variety of plant surface wax components. It is found that alkanes exhibit the highest hydrophobic effect. The wettability of the surface can be effectively improved by regulating the type and content of wax components of leaf surface, and has numerous applications in food chemistry and other fields. Meanwhile, the orientation of surface chemical groups significantly influences the wettability, primarily by altering the contact angle between the droplet and substrate.
Moreover, the influence of surface microstructures is presented. The papillae on the surface significantly impact the surface properties. The shapes of papillae, such as cylindrical, hemispherical, square, inverted cone, and mushroom shapes, affect the contact area of the droplet with the substrate. The wettability of the surface can be improved by altering the shape, height, and protrusion radius of the papillae. Furthermore, the arrangement of the papillae, whether in a regular array or irregular distribution, also have a great influence.
Furthermore, the hierarchical structure of plant surfaces, consisting of micron structures and nanostructures, enhances the wetting characteristics of the surface while also affecting its stability. These structures have gradually found applications in the manufacturing field.
Also the growth age of the leaves has a non-negligible effect on wettability. The chemical composition and microstructure of the surface undergoes significant changes as the leaf grows. Decayed leaves tend to exhibit stronger wettability than young leaves. The wettability of leaves changes during leaf growth and aging.
Then, the surface/interface properties of the plant leaf surface are analyzed from the mechanical point of view, considering rigid substrates, soft solid substrates and fluid substrates, respectively.
For rigid substrates, the wetting behavior is influenced by surface roughness and forms different wetting models. The transformation and coexistence of these models effectively impact the substrate’s performance. Various external fields, such as gravitational, magnetic, thermal, and vibrational fields, can significantly influence the wetting behavior of droplets on substrates. Thus, the wettability of the surface can be macroscopically regulated under these applied external fields. We then provided an overview of recent advancements through both experimental and theoretical calculations for the floating system formed on a fluid substrate. The study and application of compound droplets generated on fluid substrates are further illustrated. We also reviewed the wetting performance of a soft solid substrate. The effects of the elasticity of the substrate and surface strain on the morphological change of this system are considered.
This paper mainly reviews the current research progress in related areas. The hydrophobicity of plants can find broad applications in fields such as civil engineering and aerospace, particularly in developing high-performance corrosion-resistant devices. Further studies can explore the wetting behavior of droplets on various substrates, including those with anisotropy, variable cross-sections, and variable stiffness, to broaden the range of available substrates.
Author contributions
JT: Conceptualization, Funding acquisition, Investigation, Methodology, Visualization, Writing–original draft. MG: Conceptualization, Investigation, Methodology, Visualization, Writing–original draft. YH: Conceptualization, Investigation, Methodology, Visualization, Writing–original draft, Writing–review and editing. KL: Conceptualization, Supervision, Writing–review and editing. HW: Conceptualization, Funding acquisition, Supervision, Writing–review and editing.
Funding
The author(s) declare that no financial support was received for the research, authorship, and/or publication of this article.
Conflict of interest
Author JT was employed by China Tobacco Zhejiang Industrial Co., Ltd. Author HW was employed by China Tobacco Zhejiang Industrial Co., Ltd.
The remaining authors declare that the research was conducted in the absence of any commercial or financial relationships that could be construed as a potential conflict of interest.
Publisher’s note
All claims expressed in this article are solely those of the authors and do not necessarily represent those of their affiliated organizations, or those of the publisher, the editors and the reviewers. Any product that may be evaluated in this article, or claim that may be made by its manufacturer, is not guaranteed or endorsed by the publisher.
References
Abd Aziz, M. H., Othman, M. H. D., Tavares, J. R., Pauzan, M. A. B., Tenjimbayashi, M., Lun, A. W., et al. (2022). Self-cleaning and anti-fouling superhydrophobic hierarchical ceramic surface synthesized from hydrothermal and fluorination methods. Appl. Surf. Sci. 598, 153702. doi:10.1016/j.apsusc.2022.153702
Adera, S., Raj, R., Enright, R., and Wang, E. N. (2013). Non-wetting droplets on hot superhydrophilic surfaces. Nat. Commun. 4 (1), 2518. doi:10.1038/ncomms3518
Ahammed, S., Liu, F., Khin, M. N., Yokoyama, W. H., and Zhong, F. (2020). Improvement of the water resistance and ductility of gelatin film by zein. Food Hydrocoll. 105, 105804. doi:10.1016/j.foodhyd.2020.105804
Ali, Z., Merrium, S., Habib-ur-Rahman, M., Hakeem, S., Saddique, M. A. B., and Sher, M. A. (2022). Wetting mechanism and morphological adaptation; leaf rolling enhancing atmospheric water acquisition in wheat crop—a review. Environ. Sci. Pollut. Res. 29 (21), 30967–30985. doi:10.1007/s11356-022-18846-3
Alon, H., Vitoshkin, H., Ziv, C., Gunamalai, L., Sinitsa, S., and Kleiman, M. (2022). Self-cleaning biomimetic surfaces—the effect of microstructure and hydrophobicity on conidia repellence. Materials 15 (7), 2526. doi:10.3390/ma15072526
Amirabadi, S., Milani, J. M., and Sohbatzadeh, F. (2020). Application of dielectric barrier discharge plasma to hydrophobically modification of gum Arabic with enhanced surface properties. Food Hydrocoll. 104, 105724. doi:10.1016/j.foodhyd.2020.105724
Andrade, G. C., Santana, B. V. N., Rinaldi, M. C. S., Ferreira, S. O., da Silva, R. C., and da Silva, L. C. (2022). Leaf surface traits related to differential particle adsorption–A case study of two tropical legumes. Sci. Total Environ. 823, 153681. doi:10.1016/j.scitotenv.2022.153681
Bai, H., Zhang, L., and Gu, D. (2018). Micrometer-sized spherulites as building blocks for lotus leaf-like superhydrophobic coatings. Appl. Surf. Sci. 459, 54–62. doi:10.1016/j.apsusc.2018.07.183
Barima, Y. S. S., Angaman, D. M., N’gouran, K. P., Bi, F. Z. T., and Samson, R. (2016). Involvement of leaf characteristics and wettability in retaining air particulate matter from tropical plant species. Environ. Eng. Res. 21 (2), 121–131. doi:10.4491/eer.2015.120
Barlas, N. T., Bahamonde, H. A., Pimentel, C., Domínguez-Huidobro, P., Pina, C. M., and Fernández, V. (2023). Evaluating leaf wettability and salt hygroscopicity as drivers for foliar absorption. Plants 12 (12), 2357. doi:10.3390/plants12122357
Bhopalam, S. R., Bueno, J., and Gomez, H. (2022). Elasto-capillary fluid–structure interaction with compound droplets. Comput. Methods Appl. Mech. Eng. 400, 115507. doi:10.1016/j.cma.2022.115507
Bhushan, B. (2018). Characterization of rose petals and fabrication and characterization of superhydrophobic surfaces with high and low adhesion. Biomimetics, 259–287. doi:10.1007/978-3-319-71676-3_9
Bhushan, B., Jung, Y. C., and Koch, K. (2009). Micro-nano-and hierarchical structures for superhydrophobicity, self-cleaning and low adhesion. Philos. T R. Soc. A 367 (1894), 1631–1672. doi:10.1098/rsta.2009.0014
Bittoun, E., and Marmur, A. (2009). Optimizing super-hydrophobic surfaces: criteria for comparison of surface topographies. J. Adhes. Sci. Technol. 23 (3), 401–411. doi:10.1163/156856108X369958
Borcia, R., Borcia, I. D., Bestehorn, M., Varlamova, O., Hoefner, K., and Reif, J. (2019). Drop behavior influenced by the correlation length on noisy surfaces. Langmuir 35 (4), 928–934. doi:10.1021/acs.langmuir.8b03878
Bormashenko, E. (2018). Wetting of flat gradient surfaces. J. Colloid Interface Sci. 515, 264–267. doi:10.1016/j.jcis.2018.01.043
Bormashenko, E. (2020). Variational framework for defining contact angles: a general thermodynamic approach. J. Adhes. Sci. Technol. 34 (2), 219–230. doi:10.1080/01694243.2019.1663030
Bostwick, J. B., and Daniels, K. E. (2013). Capillary fracture of soft gels. Phys. Rev. E 88 (4), 042410. doi:10.1103/PhysRevE.88.042410
Burton, J., Huisman, F., Alison, P., Rogerson, D., and Taborek, P. (2010). Experimental and numerical investigation of the equilibrium geometry of liquid lenses. Langmuir 26 (19), 15316–15324. doi:10.1021/la102268n
Cassie, A., and Baxter, S. (1944). Wettability of porous surfaces. Trans. Faraday Soc. 40, 546–551. doi:10.1039/TF9444000546
Cavallaro, A., Carbonell-Silletta, L., Askenazi, J. O., Goldstein, G., Bucci, S. J., and Scholz, F. G. (2023). Phenotypic plasticity in leaf traits in response to experimental precipitation increase: wettability, foliar water uptake and gas exchange. Ecohydrology 16, e2573. doi:10.1002/eco.2573
Charitatos, V., and Kumar, S. (2021). Droplet evaporation on soft solid substrates. Soft Matter 17 (41), 9339–9352. doi:10.1039/D1SM00828E
Chen, C., Liu, M., Zhang, L., Hou, Y., Yu, M., and Fu, S. (2019). Mimicking from rose petal to lotus leaf: biomimetic multiscale hierarchical particles with tunable water adhesion. ACS Appl. Mater Interfaces 11 (7), 7431–7440. doi:10.1021/acsami.8b21494
Chen, F., Peng, Y., Song, Y., and Chen, M. (2007). EHD behavior of nitrogen bubbles in DC electric fields. Exp. Therm. Fluid Sci. 32 (1), 174–181. doi:10.1016/j.expthermflusci.2007.03.006
Chen, J., Yu, S., Fu, T., Xu, L., Tang, Y., and Li, Z. (2022). The kapok petal: superhydrophobic surface induced by microscale trichomes. Bioinspir Biomim. 17 (2), 026007. doi:10.1088/1748-3190/ac392e
Chen, Q., and Wang, G. (2019). PSO-driven micromechanical identification of in-situ properties of fiber-reinforced composites. Compos Struct. 220, 608–621. doi:10.1016/j.compstruct.2019.04.005
Cheng, K., and Chaddock, J. (1986). Maximum size of bubbles during nucleate boiling in an electric field. Int. J. Heat. Fluid Flow. 7 (4), 278–282. doi:10.1016/0142-727X(86)90005-6
Cheng, Y., Sun, C., Zhai, X., Zhang, R., Zhang, S., Sun, C., et al. (2021). Effect of lipids with different physical state on the physicochemical properties of starch/gelatin edible films prepared by extrusion blowing. Int. J. Biol. Macromol. 185, 1005–1014. doi:10.1016/j.ijbiomac.2021.06.203
Cheng, Y., Zhai, X., Wu, Y., Li, C., Zhang, R., Sun, C., et al. (2023). Effects of natural wax types on the physicochemical properties of starch/gelatin edible films fabricated by extrusion blowing. Food Chem. 401, 134081. doi:10.1016/j.foodchem.2022.134081
Chin, A. R., Guzmán-Delgado, P., Kerhoulas, L. P., and Zwieniecki, M. A. (2023). Acclimation of interacting leaf surface traits affects foliar water uptake. Tree Physiol. 43 (3), 418–429. doi:10.1093/treephys/tpac120
Dai, Q., Chen, S., Huang, W., Wang, X., and Hardt, S. (2022). On the thermocapillary migration between parallel plates. Int. J. Heat. Mass Transf. 182, 121962. doi:10.1016/j.ijheatmasstransfer.2021.121962
Dai, S., Zhu, Y., Gu, Y., and Du, Z. (2019). Biomimetic fabrication and photoelectric properties of superhydrophobic ZnO nanostructures on flexible PDMS substrates replicated from rose petal. Appl. Phys. A 125, 138–211. doi:10.1007/s00339-019-2438-7
Dawson, T. E., and Goldsmith, G. R. (2018). The value of wet leaves. New Phytol. 219 (4), 1156–1169. doi:10.1111/nph.15307
Dervaux, J., Roché, M., and Limat, L. (2020). Nonlinear theory of wetting on deformable substrates. Soft Matter 16 (22), 5157–5176. doi:10.1039/D0SM00395F
Dou, L., Li, B., Zhang, K., Chu, X., and Hou, H. (2018). Physical properties and antioxidant activity of gelatin-sodium alginate edible films with tea polyphenols. Int. J. Biol. Macromol. 118, 1377–1383. doi:10.1016/j.ijbiomac.2018.06.121
Du, J., and Hesp, P. A. (2020). Salt spray distribution and its impact on vegetation zonation on coastal dunes: a review. Estuaries Coasts 43 (8), 1885–1907. doi:10.1007/s12237-020-00820-2
Ebert, D., and Bhushan, B. (2012). Durable Lotus-effect surfaces with hierarchical structure using micro-and nanosized hydrophobic silica particles. J. Colloid Interface Sci. 368 (1), 584–591. doi:10.1016/j.jcis.2011.09.049
Feng, X., Chu, J., Tian, G., Wang, Z., Zhou, W., Zhang, X., et al. (2023). Phyllostachys viridis-leaf-like MLMN surfaces constructed by nanosecond laser hybridization for superhydrophobic antifogging and anti-icing. ACS Appl. Mater Interfaces. doi:10.1021/acsami.3c14083
Fernández, V., Sancho-Knapik, D., Guzmán, P., Peguero-Pina, J. J., Gil, L., Karabourniotis, G., et al. (2014). Wettability, polarity, and water absorption of holm oak leaves: effect of leaf side and age. Plant Physiol. 166 (1), 168–180. doi:10.1104/pp.114.242040
Flores-Vivian, I., Hejazi, V., Kozhukhova, M. I., Nosonovsky, M., and Sobolev, K. (2013). Self-assembling particle-siloxane coatings for superhydrophobic concrete. ACS Appl. Mater Interfaces 5 (24), 13284–13294. doi:10.1021/am404272v
Gao, M., Yang, B., Huang, Y., and Wang, G. (2021). Effects of general imperfect interface/interphase on the in-plane conductivity of thermal composites. Int. J. Heat. Mass Transf. 172, 121213. doi:10.1016/j.ijheatmasstransfer.2021.121213
Gao, X., Wang, D., Jiang, Z., Li, X., and Chen, G. (2022). Effect of adjuvants on the wetting behaviors of bifenthrin droplets on tea leaves. Appl. Sci. 12 (9), 4217. doi:10.3390/app12094217
Gao, Y., Guo, R., Fan, R., Liu, Z., Kong, W., Zhang, P., et al. (2018). Wettability of pear leaves from three regions characterized at different stages after flowering using the OWRK method. Pest Manag. Sci. 74 (8), 1804–1809. doi:10.1002/ps.4878
Garcia-Gonzalez, D., Corrales, T. P., Dacunzi, M., and Kappl, M. (2022). Squeezing drops: force measurements of the cassie-to-wenzel transition. Langmuir 38 (48), 14666–14672. doi:10.1021/acs.langmuir.2c02095
Ge-Zhang, S., Cai, T., Yang, H., Ding, Y., and Song, M. (2022). Biology and nature: bionic superhydrophobic surface and principle. Front. Bioeng. Biotechnol. 10, 1033514. doi:10.3389/fbioe.2022.1033514
Ghasemlou, M., Le, P. H., Daver, F., Murdoch, B. J., Ivanova, E. P., and Adhikari, B. (2021). Robust and eco-friendly superhydrophobic starch nanohybrid materials with engineered lotus leaf mimetic multiscale hierarchical structures. ACS Appl. Mater Interfaces 13 (30), 36558–36573. doi:10.1021/acsami.1c09959
Gorb, S. N., and Gorb, E. V. (2022). Anti-icing strategies of plant surfaces: the ice formation on leaves visualized by Cryo-SEM experiments. Sci. Nat. 109 (2), 24. doi:10.1007/s00114-022-01789-7
Gotsch, S. G., Asbjornsen, H., Holwerda, F., Goldsmith, G. R., Weintraub, A. E., and Dawson, T. E. (2014). Foggy days and dry nights determine crown-level water balance in a seasonal tropical montane cloud forest. Plant, Cell Environ. 37 (1), 261–272. doi:10.1111/pce.12151
Gou, X., and Guo, Z. (2019). Superhydrophobic plant leaves: the variation in surface morphologies and wettability during the vegetation period. Langmuir 35 (4), 1047–1053. doi:10.1021/acs.langmuir.8b03996
Guo, J., Deka, B. J., Kim, K.-J., and An, A. K. (2019). Regeneration of superhydrophobic TiO2 electrospun membranes in seawater desalination by water flushing in membrane distillation. Desalination 468, 114054. doi:10.1016/j.desal.2019.06.020
Hack, M. A., Tewes, W., Xie, Q., Datt, C., Harth, K., Harting, J., et al. (2020). Self-similar liquid lens coalescence. Phys. Rev. Lett. 124 (19), 194502. doi:10.1103/PhysRevLett.124.194502
Haj Ibrahim, S., Wejrzanowski, T., Przybyszewski, B., Kozera, R., García-Casas, X., and Barranco, A. (2022). Role of surface topography in the superhydrophobic effect—experimental and numerical studies. Materials 15 (9), 3112. doi:10.3390/ma15093112
Hakeem, S., Ali, Z., Saddique, M. A. B., Merrium, S., Arslan, M., and Habib-ur-Rahman, M. (2023). Leaf wettability and leaf angle affect air-moisture deposition in wheat for self-irrigation. BMC Plant Biol. 23 (1), 115–212. doi:10.1186/s12870-023-04123-z
Han, K., Park, T. Y., Yong, K., and Cha, H. J. (2019). Combinational biomimicking of lotus leaf, mussel, and sandcastle worm for robust superhydrophobic surfaces with biomedical multifunctionality: antithrombotic, antibiofouling, and tissue closure capabilities. ACS Appl. Mater Interfaces 11 (10), 9777–9785. doi:10.1021/acsami.8b21122
Hashemi Gahruie, H., Eskandari, M. H., Sadeghi, R., and Hosseini, S. M. H. (2022). Atmospheric pressure cold plasma modification of basil seed gum for fabrication of edible film incorporated with nanophytosomes of vitamin D3 and tannic acid. Foods 12 (1), 71. doi:10.3390/foods12010071
Hassan, G., Yilbas, B. S., Bahatab, S., Al-Sharafi, A., and Al-Qahtani, H. (2020). A water droplet-cleaning of a dusty hydrophobic surface: influence of dust layer thickness on droplet dynamics. Sci. Rep. 10 (1), 14746. doi:10.1038/s41598-020-71743-y
He, L., Liang, W., and Akbarzadeh, A. (2018). Thermodynamic analysis on wetting properties of a droplet on a solid surface with engineered trapezoidal microarchitecture. Int. J. Heat. Mass Transf. 119, 733–741. doi:10.1016/j.ijheatmasstransfer.2017.11.153
He, L., Liang, W., Wang, Z., Yang, B., Duan, Z., and Chen, Y. (2016). 3-D thermodynamic analysis on wetting behavior of superhydrophobic surfaces. Colloids Surf. A 504, 201–209. doi:10.1016/j.colsurfa.2016.05.070
He, X., Wang, Y.-F., Zhang, B.-X., Wang, S.-L., Yang, Y.-R., Wang, X.-D., et al. (2021). Effects of nanodroplet sizes on wettability, electrowetting transition, and spontaneous dewetting transition on nanopillar-arrayed surfaces. Langmuir 37 (50), 14571–14581. doi:10.1021/acs.langmuir.1c01807
He, X., Zhang, B.-X., Wang, S.-L., Wang, Y.-F., Yang, Y.-R., Wang, X.-D., et al. (2022). Electrowetting-based control of wetting transition of a nanodroplet on pillar-arrayed surfaces. J. Mol. Liq. 345, 117049. doi:10.1016/j.molliq.2021.117049
Henkel, C., Essink, M., Hoang, T., Van Zwieten, G., Van Brummelen, E., Thiele, U., et al. (2022). Soft wetting with (a) symmetric Shuttleworth effect. P Roy. Soc. A 478 (2264), 20220132. doi:10.1098/rspa.2022.0132
Henkel, C., Snoeijer, J. H., and Thiele, U. (2021). Gradient-dynamics model for liquid drops on elastic substrates. Soft Matter 17 (45), 10359–10375. doi:10.1039/D1SM01032H
Holder, C. D. (2012). The relationship between leaf hydrophobicity, water droplet retention, and leaf angle of common species in a semi-arid region of the western United States. Agric For Meteorol 152, 11–16. doi:10.1016/j.agrformet.2011.08.005
Holloway, P. (1969). Chemistry of leaf waxes in relation to wetting. J. Sci. Food Agric. 20 (2), 124–128. doi:10.1002/jsfa.2740200214
Holloway, P. (1970). Surface factors affecting the wetting of leaves. Pestic. Sci. 1 (4), 156–163. doi:10.1002/ps.2780010411
Huang, J.-A., Zhang, Y.-L., Zhao, Y., Zhang, X.-L., Sun, M.-L., and Zhang, W. (2016). Superhydrophobic SERS chip based on a Ag coated natural taro-leaf. Nanoscale 8 (22), 11487–11493. doi:10.1039/C6NR03285K
Huth, M. A., Huth, A., Schreiber, L., and Koch, K. (2022). Design of a biomimetic, small-scale artificial leaf surface for the study of environmental interactions. Beilstein J. Nanotechnol. 13 (1), 944–957. doi:10.3762/bjnano.13.83
Janeczko, C., Martelli, C., Canning, J., and Dutra, G. (2019). Assessment of orchid surfaces using top-down contact angle mapping. IEEE Access 7, 31364–31375. doi:10.1109/ACCESS.2019.2902730
Jerison, E. R., Xu, Y., Wilen, L. A., and Dufresne, E. R. (2011). Deformation of an elastic substrate by a three-phase contact line. Phys. Rev. Lett. 106 (18), 186103. doi:10.1103/PhysRevLett.106.186103
Jiang, Y., Lian, J., Jiang, Z., Li, Y., and Wen, C. (2020). Thermodynamic analysis on wetting states and wetting state transitions of rough surfaces. Adv. Colloid Interface Sci. 278, 102136. doi:10.1016/j.cis.2020.102136
Ju, J., Fejjari, K., Cheng, Y., Liu, M., Li, Z., Kang, W., et al. (2020). Engineering hierarchically structured superhydrophobic PTFE/POSS nanofibrous membranes for membrane distillation. Desalination 486, 114481. doi:10.1016/j.desal.2020.114481
Jung, K. K., Jung, Y., Park, B.-G., Choi, C. J., and Ko, J. S. (2022). Super wear resistant nanostructured superhydrophobic surface. Int. J. P. R. Eng. Man-G T 9 (4), 1177–1189. doi:10.1007/s40684-021-00325-8
Jura-Morawiec, J., and Marcinkiewicz, J. (2020). Wettability, water absorption and water storage in rosette leaves of the dragon tree (Dracaena draco L.). Planta 252, 30–38. doi:10.1007/s00425-020-03433-y
Kalaivendan, R. G. T., Eazhumalai, G., and Annapure, U. S. (2023). Impact of pin-to-plate cold plasma depolymerization on the gelation and functional attributes of guar galactomannan. J. Food Process Eng. 46, e14340. doi:10.1111/jfpe.14340
Kang, F., Yi, Z., Zhao, B., and Qin, Z. (2021). Surface physical structure and durability of superhydrophobic wood surface with epoxy resin. BioResources 16 (2), 3235–3254. doi:10.15376/biores.16.2.3235-3254
Kang, H., Graybill, P. M., Fleetwood, S., Boreyko, J. B., and Jung, S. (2018). Seasonal changes in morphology govern wettability of Katsura leaves. PLoS ONE 13 (9), e0202900. doi:10.1371/journal.pone.0202900
Kayabaş, A., and Yildirim, E. (2022). New approaches with ATR-FTIR, SEM, and contact angle measurements in the adaptation to extreme conditions of some endemic Gypsophila L. taxa growing in gypsum habitats. Spectrochim. Acta, Part A 270, 120843. doi:10.1016/j.saa.2021.120843
Khandoker, M. A. R., and Golovin, K. (2020). Statistical heuristic wettability analysis of randomly textured surfaces. Langmuir 36 (47), 14361–14371. doi:10.1021/acs.langmuir.0c02703
Khattak, H. K., Karpitschka, S., Snoeijer, J. H., and Dalnoki-Veress, K. (2022). Direct force measurement of microscopic droplets pulled along soft surfaces. Nat. Commun. 13 (1), 4436. doi:10.1038/s41467-022-31910-3
Kim, P., Kreder, M. J., Alvarenga, J., and Aizenberg, J. (2013). Hierarchical or not? Effect of the length scale and hierarchy of the surface roughness on omniphobicity of lubricant-infused substrates. Nano Lett. 13 (4), 1793–1799. doi:10.1021/nl4003969
Koch, K., and Barthlott, W. (2009). Superhydrophobic and superhydrophilic plant surfaces: an inspiration for biomimetic materials. Philos. T R. Soc. A 367 (1893), 1487–1509. doi:10.1098/rsta.2009.0022
Koishi, T., Yasuoka, K., Fujikawa, S., Ebisuzaki, T., and Zeng, X. C. (2009). Coexistence and transition between Cassie and Wenzel state on pillared hydrophobic surface. P Nat. Acad. Sci. 106 (21), 8435–8440. doi:10.1073/pnas.0902027106
Kumar, V., Verma, R., and Bairwa, H. K. (2022). Fabrication of superhydrophobic surfaces by laser surface texturing and autoxidation. J. Electrochem Sci. Eng. 12 (4), 639–649. doi:10.5599/jese.1260
Lai, X., and Li, S. (2022). Substrate elasticity and surface tension mediate the spontaneous rotation of active chiral droplet on soft substrates. J. Mech. Phys. Solids 161, 104788. doi:10.1016/j.jmps.2022.104788
Ledari, S. A., Milani, J. M., and Lanbar, F. S. (2020). Improving gelatin-based emulsion films with cold plasma using different gases. Food Sci. Nutr. 8 (12), 6487–6496. doi:10.1002/fsn3.1939
Lee, H. G., Yang, J., Kim, S., and Kim, J. (2021b). Modeling and simulation of droplet evaporation using a modified Cahn–Hilliard equation. Appl. Math. Comput. 390, 125591. doi:10.1016/j.amc.2020.125591
Lee, H. K., Ray, S. S., Huyen, D. T. T., Kang, W., and Kwon, Y.-N. (2021a). Chemical and surface engineered superhydrophobic patterned membrane with enhanced wetting and fouling resistance for improved membrane distillation performance. J. Membr. Sci. 629, 119280. doi:10.1016/j.memsci.2021.119280
Léonforte, F., and Müller, M. (2011). Statics of polymer droplets on deformable surfaces. J. Chem. Phys. 135 (21), 214703. doi:10.1063/1.3663381
Li, C., Yu, C., Zhou, S., Dong, Z., and Jiang, L. (2020). Liquid harvesting and transport on multiscaled curvatures. Proc. Nati Acad. Sci. 117 (38), 23436–23442. doi:10.1073/pnas.2011935117
Li, H.-J., Bai, W.-P., Liu, L.-B., Liu, H.-S., Wei, L., Garant, T. M., et al. (2023). Massive increases in C31 alkane on Zygophyllum xanthoxylum leaves contribute to its excellent abiotic stress tolerance. Ann. Bot. 131 (4), 723–736. doi:10.1093/aob/mcad038
Li, P., Wang, L., Zhao, F., Feng, S., Zhang, Q., Zhao, H., et al. (2021). Design of flexible multi-level topography for enhancing mechanical property. Nano Sel. 2 (3), 541–548. doi:10.1002/nano.202000203
Li, X., Jiang, Y., Tan, X., Zhang, Z., Jiang, Z., Lian, J., et al. (2022). Superhydrophobic brass surfaces with tunable water adhesion fabricated by laser texturing followed by heat treatment and their anti-corrosion ability. Appl. Surf. Sci. 575, 151596. doi:10.1016/j.apsusc.2021.151596
Liang, W., Sui, X., Zhang, X., He, L., and Sun, Y. (2019). 3D thermodynamic analysis of superhydrophobicity of cylinder microtextured surfaces. Surf. Innov. 8 (1–2), 28–37. doi:10.1680/jsuin.19.00033
Liang, X., and Li, K. (2020). Adhesion of a cell on a prestretched elastomer incorporating gravity effect. Eur. J. Mech-A/Solid 84, 104077. doi:10.1016/j.euromechsol.2020.104077
Liao, Y., Wang, R., and Fane, A. G. (2013). Engineering superhydrophobic surface on poly (vinylidene fluoride) nanofiber membranes for direct contact membrane distillation. J. Membr. Sci. 440, 77–87. doi:10.1016/j.memsci.2013.04.006
Liu, F., Antoniou, J., Li, Y., Ma, J., and Zhong, F. (2015). Effect of sodium acetate and drying temperature on physicochemical and thermomechanical properties of gelatin films. Food Hydrocoll. 45, 140–149. doi:10.1016/j.foodhyd.2014.10.009
Liu, J., Nie, Z., and Jiang, W. (2009). Deformation field of the soft substrate induced by capillary force. Phys. B 404 (8-11), 1195–1199. doi:10.1016/j.physb.2008.11.196
Liu, L., Jiang, J., Zhang, S., Zhu, M., Dong, X., and Mi, M. (2021). Morphology evolution of a volatile liquid lens on another immiscible liquid surface induced by evaporation. Langmuir 37 (48), 14081–14088. doi:10.1021/acs.langmuir.1c02157
Liu, Y., Hanati, A., and Lan, H. (2023). Characterization of leaf trichomes and their influence on surface wettability of Salsola ferganica, an annual halophyte in the desert. Physiol. Plant 175 (3), e13905. doi:10.1111/ppl.13905
Liu, Y.-M., Wu, Z.-Q., and Yin, D.-C. (2020). Measurement of contact angle under different gravity generated by a long-arm centrifuge. Colloids Surf. A 588, 124381. doi:10.1016/j.colsurfa.2019.124381
Lopes, D. M., Ramos, S. M., de Oliveira, L. R., and Mombach, J. C. (2013). Cassie–Baxter to Wenzel state wetting transition: a 2D numerical simulation. RSC Adv. 3 (46), 24530–24534. doi:10.1039/C3RA45258A
Losso, A., Dämon, B., Hacke, U., and Mayr, S. (2023). High potential for foliar water uptake in early stages of leaf development of three woody angiosperms. Physiol. Plant 175, e13961. doi:10.1111/ppl.13961
Lubbers, L. A., Weijs, J. H., Botto, L., Das, S., Andreotti, B., and Snoeijer, J. H. (2014). Drops on soft solids: free energy and double transition of contact angles. J. Fluid Mech. 747, R1. doi:10.1017/jfm.2014.152
Lv, C., and Shi, S. (2018). Wetting states of two-dimensional drops under gravity. Phys. Rev. E 98 (4), 042802. doi:10.1103/PhysRevE.98.042802
Maharbiz, M. M., Holtz, W. J., Howe, R. T., and Keasling, J. D. (2004). Microbioreactor arrays with parametric control for high-throughput experimentation. Biotechnol. Bioeng. 85 (4), 376–381. doi:10.1002/bit.10835
Mao, D., Wang, X., Wu, Y., Gu, Z., Wang, C., and Tu, Y. (2021). Unexpected hydrophobicity on self-assembled monolayers terminated with two hydrophilic hydroxyl groups. Nanoscale 13 (46), 19604–19609. doi:10.1039/D1NR05048F
Marchand, A., Das, S., Snoeijer, J. H., and Andreotti, B. (2012). Contact angles on a soft solid: from Young’s law to Neumann’s law. Phys. Rev. Lett. 109 (23), 236101. doi:10.1103/PhysRevLett.109.236101
Marmur, A. (2004). The lotus effect: superhydrophobicity and metastability. Langmuir 20 (9), 3517–3519. doi:10.1021/la036369u
Meng, C., Fehler, M., and Hager, B. (2021). Elastic medium containing interacting fluid inclusions. Compos Struct. 256, 113018. doi:10.1016/j.compstruct.2020.113018
Mereu, S., Gerosa, G., Marzuoli, R., Fusaro, L., Salvatori, E., Finco, A., et al. (2011). Gas exchange and JIP-test parameters of two Mediterranean maquis species are affected by sea spray and ozone interaction. Environ. Exp. Bot. 73, 80–88. doi:10.1016/j.envexpbot.2011.02.004
Merrium, S., Ali, Z., Habib-ur-Rahman, M., Hakeem, S., and Khalid, M. A. (2022a). Leaf rolling and leaf angle improve fog capturing and transport in wheat; adaptation for drought stress in an arid climate. Bot. Stud. 63 (1), 13. doi:10.1186/s40529-022-00343-y
Merrium, S., Ali, Z., Tahir, M. H. N., Habib-ur-Rahman, M., and Hakeem, S. (2022b). Leaf rolling dynamics for atmospheric moisture harvesting in wheat plant as an adaptation to arid environments. Environ. Sci. Pollut. Res. 29 (32), 48995–49006. doi:10.1007/s11356-022-18936-2
Miguel, S., Hehn, A., and Bourgaud, F. (2018). Nepenthes: state of the art of an inspiring plant for biotechnologists. J. Biotechnol. 265, 109–115. doi:10.1016/j.jbiotec.2017.11.014
Moradi, S., Hadjesfandiari, N., Toosi, S. F., Kizhakkedathu, J. N., and Hatzikiriakos, S. G. (2016). Effect of extreme wettability on platelet adhesion on metallic implants: from superhydrophilicity to superhydrophobicity. ACS Appl. Mater Interfaces 8 (27), 17631–17641. doi:10.1021/acsami.6b03644
Muscat, D., Adhikari, R., McKnight, S., Guo, Q., and Adhikari, B. (2013). The physicochemical characteristics and hydrophobicity of high amylose starch–glycerol films in the presence of three natural waxes. J. Food Eng. 119 (2), 205–219. doi:10.1016/j.jfoodeng.2013.05.033
Nairn, J. J., Forster, W. A., and van Leeuwen, R. M. (2011). Quantification of physical (roughness) and chemical (dielectric constant) leaf surface properties relevant to wettability and adhesion. Pest Manag. Sci. 67 (12), 1562–1570. doi:10.1002/ps.2213
Nairn, J. J., Forster, W. A., and van Leeuwen, R. M. (2022). Quantification of physical (roughness) and chemical (dielectric constant) leaf surface properties relevant to wettability and adhesion. Pest Manag. Sci. 67, 1562–1570. doi:10.1002/ps.2213
Neeson, M. J., Tabor, R. F., Grieser, F., Dagastine, R. R., and Chan, D. Y. (2012). Compound sessile drops. Soft Matter 8 (43), 11042–11050. doi:10.1039/C2SM26637G
Neinhuis, C., and Barthlott, W. (1998). Seasonal changes of leaf surface contamination in beech, oak, and ginkgo in relation to leaf micromorphology and wettability. New Phytol. 138 (1), 91–98. doi:10.1046/j.1469-8137.1998.00882.x
Neinhuis, C., and Barthlott, W. (1997). Characterization and distribution of water-repellent, self-cleaning plant surfaces. Ann. Bot. 79 (6), 667–677. doi:10.1006/anbo.1997.0400
Neumann, F. E., and Wangerin, A. (1894). Vorlesung über die Theorie der Capillarität. Leipzig, Germany: B. G. Teubner Verlag.
Oberlintner, A., Shvalya, V., Vasudevan, A., Vengust, D., Likozar, B., Cvelbar, U., et al. (2022). Hydrophilic to hydrophobic: ultrafast conversion of cellulose nanofibrils by cold plasma fluorination. Appl. Surf. Sci. 581, 152276. doi:10.1016/j.apsusc.2021.152276
Onda, T., Shibuichi, S., Satoh, N., and Tsujii, K. (1996). Super-water-repellent fractal surfaces. Langmuir 12 (9), 2125–2127. doi:10.1021/la950418o
Ou, J., Fang, G., Li, W., and Amirfazli, A. (2019). Wetting transition on textured surfaces: a thermodynamic approach. J. Phys. Chem. C 123 (39), 23976–23986. doi:10.1021/acs.jpcc.9b05477
Pan, Q., Lu, Y., Xue, L., and Hu, Y. (2023). Leaf wettability difference among tea leaf ages and analysis based on microscopic surface features. Phyton 92 (2), 411–421. doi:10.32604/phyton.2022.023437
Patel, P., Choi, C. K., and Meng, D. D. (2010). Superhydrophilic surfaces for antifoqging and antifouling microfluidic devices. JALA J. Lab. Autom. 15 (2), 114–119. doi:10.1016/j.jala.2009.10.012
Phan, C. M. (2014). Stability of a floating water droplet on an oil surface. Langmuir 30 (3), 768–773. doi:10.1021/la403830k
Phan, C. M., Allen, B., Peters, L. B., Le, T. N., and Tade, M. O. (2012). Can water float on oil? Langmuir 28 (10), 4609–4613. doi:10.1021/la204820a
Piccolo, E. L., Lauria, G., Pellegrini, E., Cotrozzi, L., Guidi, L., Skoet, M., et al. (2023). Testing the suitability for coastal green areas of three ornamental shrub species through physiological responses to the saline nebulization. Urban For Urban Green 84, 127920. doi:10.1016/j.ufug.2023.127920
Pototsky, A., Oron, A., and Bestehorn, M. (2019). Vibration-induced floatation of a heavy liquid drop on a lighter liquid film. Phys. Fluids 31 (8), 31. doi:10.1063/1.5099661
Pototsky, A., Oron, A., and Bestehorn, M. (2021). Equilibrium shapes and floatability of static and vertically vibrated heavy liquid drops on the surface of a lighter fluid. J. Fluid Mech. 922, A31. doi:10.1017/jfm.2021.546
Qu, Z., Chen, G., Wang, J., Xie, X., and Chen, Y. (2023). Preparation, structure evaluation, and improvement in foaming characteristics of fibrotic pea protein isolate by cold plasma synergistic organic acid treatment. Food Hydrocoll. 134, 108057. doi:10.1016/j.foodhyd.2022.108057
Rauter, M. T., Schnell, S. K., and Kjelstrup, S. (2021). Cassie–baxter and wenzel states and the effect of interfaces on transport properties across membranes. J. Phys. Chem. B 125 (46), 12730–12740. doi:10.1021/acs.jpcb.1c07931
Ravazzoli, P. D., Gonzalez, A. G., Diez, J. A., and Stone, H. (2020). Buoyancy and capillary effects on floating liquid lenses. Phys. Rev. Fluids 5 (7), 073604. doi:10.1103/PhysRevFluids.5.073604
Revilla, P., Fernández, V., Álvarez-Iglesias, L., Medina, E. T., and Cavero, J. (2016). Leaf physico-chemical and physiological properties of maize (Zea mays L.) populations from different origins. Plant Physiol. Biochem. 107, 319–325. doi:10.1016/j.plaphy.2016.06.017
Rostami, P., and Auernhammer, G. K. (2022). Capillary filling in drop merging: dynamics of the four-phase contact point. Phys. Fluids 34 (1), 012107. doi:10.1063/5.0073057
Sase, H., Takahashi, A., Sato, M., Kobayashi, H., Nakata, M., and Totsuka, T. (2008). Seasonal variation in the atmospheric deposition of inorganic constituents and canopy interactions in a Japanese cedar forest. Environ. Pollut. 152 (1), 1–10. doi:10.1016/j.envpol.2007.06.023
Saubade, F., Pilkington, L. I., Liauw, C. M., Gomes, L. C., McClements, J., Peeters, M., et al. (2021). Principal component analysis to determine the surface properties that influence the self-cleaning action of hydrophobic plant leaves. Langmuir 37 (27), 8177–8189. doi:10.1021/acs.langmuir.1c00853
Schiavon, C. S., Moreira, M. L., Cava, S. S., Raubach, C. W., and Jardim, P. L. (2022). Wetting-state transition of random surfaces. Thin Solid Films 745, 139102. doi:10.1016/j.tsf.2022.139102
Schulman, R. D., and Dalnoki-Veress, K. (2015). Liquid droplets on a highly deformable membrane. Phys. Rev. Lett. 115 (20), 206101. doi:10.1103/PhysRevLett.115.206101
Shardt, N., and Elliott, J. A. (2019). Gibbsian thermodynamics of wenzel wetting (was wenzel wrong? revisited). Langmuir 36 (1), 435–446. doi:10.1021/acs.langmuir.9b02984
Shi, H., Wang, H.-X., and Li, Y.-Y. (2011). Wettability on plant leaf surfaces and its ecological significance. Shengtai Xuebao/Acta Ecol. Sin. 31 (15), 4287–4298. doi:10.1093/mp/ssq070
Shibuichi, S., Onda, T., Satoh, N., and Tsujii, K. (1996). Super water-repellent surfaces resulting from fractal structure. J. Phys. Chem. 100 (50), 19512–19517. doi:10.1021/jp9616728
Singh, P., Singh, N., and Pal, A. (2023). Hanging droplets from liquid interfaces. J. Fluid Mech. 958, A48. doi:10.1017/jfm.2023.137
Song, Z., Liang, X., Li, K., and Cai, S. (2020). Surface mechanics of a stretched elastomer layer bonded on a rigid substrate. Int. J. Solids Struct. 200, 1–12. doi:10.1016/j.ijsolstr.2020.04.015
Staniscia, F., Guzman, H. V., and Kanduc, M. (2022). Tuning contact angles of aqueous droplets on hydrophilic and hydrophobic surfaces by surfactants. J. Phys. Chem. B 126 (17), 3374–3384. doi:10.1021/acs.jpcb.2c01599
Style, R. W., Boltyanskiy, R., Che, Y., Wettlaufer, J., Wilen, L. A., and Dufresne, E. R. (2013). Universal deformation of soft substrates near a contact line and the direct measurement of solid surface stresses. Phys. Rev. Lett. 110 (6), 066103. doi:10.1103/PhysRevLett.110.066103
Style, R. W., and Dufresne, E. R. (2012). Static wetting on deformable substrates, from liquids to soft solids. Soft Matter 8 (27), 7177–7184. doi:10.1039/c2sm25540e
Style, R. W., Tutika, R., Kim, J. Y., and Bartlett, M. D. (2021). Solid–liquid composites for soft multifunctional materials. Adv. Funct. Mater 31 (1), 2005804. doi:10.1002/adfm.202005804
Su, Y., Ji, B., Zhang, K., Gao, H., Huang, Y., and Hwang, K. (2010). Nano to micro structural hierarchy is crucial for stable superhydrophobic and water-repellent surfaces. Langmuir 26 (7), 4984–4989. doi:10.1021/la9036452
Sui, X., Sun, Y., Liang, W., and Wang, Y. (2020). Thermodynamic analysis of hydrophobic property of a circular truncated cone microtexture. Surf. Innov. 9 (4), 231–242. doi:10.1680/jsuin.20.00067
Sui, X., Tam, J., Erb, U., and Liang, W. (2022). Thermodynamic analysis on wetting state transitions of rough surfaces with 3D irregular microstructure. Surf. Interfaces 34, 102378. doi:10.1016/j.surfin.2022.102378
Sun, J., and Weisensee, P. B. (2023). Marangoni-induced reversal of meniscus-climbing microdroplets. Soft Matter 19 (4), 625–633. doi:10.1039/D2SM00979J
Sun, Y., Yang, X., Yang, Z., Wang, Q., and Men, Y. (2014). Difference in wettability of lotus leaves in typical states and its mechanism analysis. Trans. Chin. Soc. Agric. Eng. 30 (13), 263–267. doi:10.3969/j.issn.1002-6819.2014.13.032
Tan, H.-F., Tan, W.-L., Ooi, B., and Leo, C. (2021). Superhydrophobic PVDF/micro fibrillated cellulose membrane for membrane distillation crystallization of struvite. Chem. Eng. Res. Des. 170, 54–68. doi:10.1016/j.cherd.2021.03.027
Tang, H., and Cheng, X. (2022). Measurement of liquid surface tension by fitting the lying droplet profile. Measurement 188, 110379. doi:10.1016/j.measurement.2021.110379
Tang, Y., Yang, X., Li, Y., Lu, Y., and Zhu, D. (2021). Robust micro-nanostructured superhydrophobic surfaces for long-term dropwise condensation. Nano Lett. 21 (22), 9824–9833. doi:10.1021/acs.nanolett.1c01584
van Gorcum, M., Karpitschka, S., Andreotti, B., and Snoeijer, J. H. (2020). Spreading on viscoelastic solids: are contact angles selected by Neumann's law? Soft Matter 16 (5), 1306–1322. doi:10.1039/C9SM01453E
Wang, G., Gao, M., Yang, B., and Chen, Q. (2020). The morphological effect of carbon fibers on the thermal conductive composites. Int. J. Heat. Mass Transf. 152, 119477. doi:10.1016/j.ijheatmasstransfer.2020.119477
Wang, G., and Pindera, M.-J. (2017). Elasticity-based microstructural optimization: an integrated multiscale framework. Mater Des. 132, 337–348. doi:10.1016/j.matdes.2017.07.003
Wang, G., Tu, W., and Pindera, M.-J. (2017). Tailoring the moduli of composites using hollow reinforcement. Compos Struct. 160, 838–853. doi:10.1016/j.compstruct.2016.10.060
Wang, H., Li, Z., and Yang, J. (2023b). Effects of leaf hydrophilicity and stomatal regulation on foliar water uptake capacity of desert plants. Forests 14 (3), 551. doi:10.3390/f14030551
Wang, H., Shi, H., Li, Y., Yu, Y., and Zhang, J. (2013). Seasonal variations in leaf capturing of particulate matter, surface wettability and micromorphology in urban tree species. Front. Environ. Sci. Eng. 7, 579–588. doi:10.1007/s11783-013-0524-1
Wang, J., Liu, Y., Hu, S., Xu, J., Nian, J., Cao, X., et al. (2022b). LEAF TIP RUMPLED 1 regulates leaf morphology and salt tolerance in rice. Int. J. Mol. Sci. 23 (15), 8818. doi:10.3390/ijms23158818
Wang, L., Zhao, F., Tang, S., Zhao, H., and Liu, J. (2021b). Optimal design of micro-topography on natural leaf surface. AIP Adv. 11 (9), 11. doi:10.1063/5.0061602
Wang, N., Wang, Q., Xu, S., and Lei, L. (2021a). Fabrication of hierarchical structures on concrete surfaces with superhydrophobicity using replicated micro-nano dendritic structures. J. Ind. Eng. Chem. 103, 314–321. doi:10.1016/j.jiec.2021.07.047
Wang, Y., Li, B., Bao, P., Wang, R., Min, A., and Xiong, P. (2023a). A case study of leaf wettability variability and the relations with leaf traits and surface water storage for urban landscape plants. Water 15 (12), 2152. doi:10.3390/w15122152
Wang, Z., Wang, P., Song, H., and Chen, Z. (2022a). Dynamic wetting behavior of nanofluid droplet on a vertically vibrating surface: a molecular dynamics study. J. Mol. Liq. 347, 118360. doi:10.1016/j.molliq.2021.118360
Ward, C., and Sasges, M. (1998). Effect of gravity on contact angle: a theoretical investigation. J. Chem Phys 109 (9), 3651–3660. doi:10.1063/1.476962
Wenzel, R. N. (1936). Resistance of solid surfaces to wetting by water. Ind. Eng. Chem. 28 (8), 988–994. doi:10.1021/ie50320a024
Wiącek, A. E. (2015). Effect of surface modification on starch biopolymer wettability. Food Hydrocoll. 48, 228–237. doi:10.1016/j.foodhyd.2015.02.005
Wong, C. Y., Adda-Bedia, M., and Vella, D. (2017). Non-wetting drops at liquid interfaces: from liquid marbles to Leidenfrost drops. Soft Matter 13 (31), 5250–5260. doi:10.1039/C7SM00990A
Wu, R., Xu, R., and Wang, G. (2023). Multiscale viscoelastic analysis of FRP-strengthened concrete beams. Int. J. Mech. Sci. 253, 108396. doi:10.1016/j.ijmecsci.2023.108396
Wu, Z., Li, Y., Cui, S., Li, X., Zhou, Z., and Tian, X. (2022). A study of the critical velocity of the droplet transition from the Cassie to wenzel state on the symmetric pillared surface. Symmetry 14 (9), 1891. doi:10.3390/sym14091891
Xiao, Z., Guo, H., He, H., Liu, Y., Li, X., Zhang, Y., et al. (2020). Unprecedented scaling/fouling resistance of omniphobic polyvinylidene fluoride membrane with silica nanoparticle coated micropillars in direct contact membrane distillation. J. Membr. Sci. 599, 117819. doi:10.1016/j.memsci.2020.117819
Xie, Y., Li, S., Hu, X., and Bishara, D. (2022). An adhesive Gurtin-Murdoch surface hydrodynamics theory of moving contact line and modeling of droplet wettability on soft substrates. J. Comput. Phys. 456, 111074. doi:10.1016/j.jcp.2022.111074
Xiong, P., Chen, Z., Jia, Z., Wang, Z., Palta, J. A., and Xu, B. (2018). Variability in leaf wettability and surface water retention of main species in semiarid Loess Plateau of China. Ecohydrology 11 (8), e2021. doi:10.1002/eco.2021
Xu, P., Sui, X., Ge, A., Wang, S., Coyle, T. W., and Mostaghimi, J. (2022). Hydrocarbon-induced reversible wetting behaviors of hierarchically-structured yttrium oxide coatings. Surf. Coat. Technol. 449, 128996. doi:10.1016/j.surfcoat.2022.128996
Xu, Z., Guo, Y., Liu, Y., Jia, B., Sha, P., Li, L., et al. (2023). An extremely efficiency method to achieve stable superhydrophobicity on the surface of additive manufactured NiTi Alloys:“Ultrasonic Fluorination”. Appl. Surf. Sci. 612, 155947. doi:10.1016/j.apsusc.2022.155947
Yamamoto, M., Nishikawa, N., Mayama, H., Nonomura, Y., Yokojima, S., Nakamura, S., et al. (2015). Theoretical explanation of the lotus effect: superhydrophobic property changes by removal of nanostructures from the surface of a lotus leaf. Langmuir 31 (26), 7355–7363. doi:10.1021/acs.langmuir.5b00670
York, D. W., Collins, S., and Rantape, M. (2019). Measuring the permeability of thin solid layers of natural waxes. J. Colloid Interface Sci. 551, 270–282. doi:10.1016/j.jcis.2019.03.104
Young, T. (1805). An essay on the cohesion of fluids. Philos. T R. Soc. (95), 65–87. doi:10.1098/rstl.1805.0005
Yu, Y., Zhao, Z.-H., and Zheng, Q.-S. (2007). Mechanical and superhydrophobic stabilities of two-scale surfacial structure of lotus leaves. Langmuir 23 (15), 8212–8216. doi:10.1021/la7003485
Yuan, W., Long, J., Ding, Y., and Wang, G. (2018). Statistical contact model of rough surfaces: the role of surface tension. Int. J. Solids Struct. 138, 217–223. doi:10.1016/j.ijsolstr.2018.01.014
Yun, X., Xiong, Z., He, Y., and Wang, X. (2020). Superhydrophobic lotus-leaf-like surface made from reduced graphene oxide through soft-lithographic duplication. RSC Adv. 10 (9), 5478–5486. doi:10.1039/C9RA10373B
Zeng, H., Lyu, S., Legendre, D., and Sun, C. (2022). Influence of gravity on the freezing dynamics of drops on a solid surface. Phys. Rev. Fluids 7 (10), 103605. doi:10.1103/PhysRevFluids.7.103605
Zhan, F., Gao, W., Zhao, F., Qin, P., Sun, X., Sun, C., et al. (2022). Design of a flexible surface/interlayer for packaging. Soft Matter 18 (11), 2123–2128. doi:10.1039/D1SM01799C
Zhang, C.-Y., Gao, P., Li, E.-Q., and Ding, H. (2021). On the compound sessile drops: configuration boundaries and transitions. J. Fluid Mech. 917, A37. doi:10.1017/jfm.2021.314
Zhang, K., Li, H., Xin, L., Li, P., and Sun, W. (2023). Engulfed small Wenzel droplets on hierarchically structured superhydrophobic surface by large Cassie droplets: experiments and molecular dynamics simulations. Appl. Surf. Sci. 608, 155000. doi:10.1016/j.apsusc.2022.155000
Zhang, X., Lyu, J., Han, Y., Sun, N., Sun, W., Li, J., et al. (2020). Effects of the leaf functional traits of coniferous and broadleaved trees in subtropical monsoon regions on PM2. 5 dry deposition velocities. Environ. Pollut. 265, 114845. doi:10.1016/j.envpol.2020.114845
Zhang, Y., Chatain, D., Anna, S. L., and Garoff, S. (2016). Stability of a compound sessile drop at the axisymmetric configuration. J. Colloid Interface Sci. 462, 88–99. doi:10.1016/j.jcis.2015.09.043
Zhao, B., Bonaccurso, E., Auernhammer, G. K., and Chen, L. (2021). Elasticity-to-Capillarity transition in soft substrate deformation. Nano Lett. 21 (24), 10361–10367. doi:10.1021/acs.nanolett.1c03643
Zhao, C., Kern, V. R., Carlson, A., and Lee, T. (2023). Engulfment of a drop on solids coated by thin and thick fluid films. J. Fluid Mech. 958, A41. doi:10.1017/jfm.2023.110
Zhao, Y., Liu, Y., Liu, Q., Guo, W., Yang, L., and Ge, D. (2018). Icephobicity studies of superhydrophobic coatings on concrete via spray method. Mater Lett. 233, 263–266. doi:10.1016/j.matlet.2018.09.008
Zheng, G., Yao, L., You, X., Liao, Y., Wang, R., and Huang, J. J. (2021). Effects of different secondary nano-scaled roughness on the properties of omniphobic membranes for brine treatment using membrane distillation. J. Membr. Sci. 620, 118918. doi:10.1016/j.memsci.2020.118918
Zhu, H., and Guo, Z. (2016). Wetting characterizations of oilseed rapes. J. Bionic Eng. 13 (2), 213–219. doi:10.1016/S1672-6529(16)60295-0
Zhu, Y. Q., Yu, C. X., Li, Y., Zhu, Q. Q., Zhou, L., Cao, C., et al. (2014). Research on the changes in wettability of rice (Oryza sativa.) leaf surfaces at different development stages using the OWRK method. Pest Manag. Sci. 70 (3), 462–469. doi:10.1002/ps.3594
Zong, C., Hu, M., Azhar, U., Chen, X., Zhang, Y., Zhang, S., et al. (2019). Smart copolymer-functionalized flexible surfaces with photoswitchable wettability: from superhydrophobicity with “rose petal” effect to superhydrophilicity. ACS Appl. Mater Interfaces 11 (28), 25436–25444. doi:10.1021/acsami.9b07767
Keywords: wettability, plant surfaces, effect factors, surface/interface mechanics, substrates
Citation: Tie J, Gao M, Huang Y, Li K and Wang H (2023) Factors influencing wettability and surface/interface mechanics of plant surfaces: a review. Front. Mater. 10:1311735. doi: 10.3389/fmats.2023.1311735
Received: 10 October 2023; Accepted: 06 December 2023;
Published: 21 December 2023.
Edited by:
Vipul Sharma, University of Turku, FinlandReviewed by:
Kyriacos Yiannacou, Tampere University of Technology, FinlandJie Feng, Zhejiang University of Technology, China
Copyright © 2023 Tie, Gao, Huang, Li and Wang. This is an open-access article distributed under the terms of the Creative Commons Attribution License (CC BY). The use, distribution or reproduction in other forums is permitted, provided the original author(s) and the copyright owner(s) are credited and that the original publication in this journal is cited, in accordance with accepted academic practice. No use, distribution or reproduction is permitted which does not comply with these terms.
*Correspondence: Yulin Huang, eXVsaW5odWFuZ0B6anUuZWR1LmNu; Kecheng Li, bGlrZWNoZW5nQG5idS5lZHUuY24=; Hui Wang, d2FuZ2h1aUB6anRvYmFjY28uY29t