- 1Department of Chemical Engineering, Hanyang University, Seoul, Republic of Korea
- 2Advanced Photovoltaics Research Center, Korea Institute of Science and Technology (KIST), Seoul, Republic of Korea
The commercialization of organo-inorganic hybrid perovskite materials for optoelectronic applications is limited owing to the restriction of lead (Pb) usage in consumer electronics and the instability of organic cations in the perovskite structure. To address these challenges, we synthesize TlSnX3 (X = Cl, Br, and I) perovskite nanoparticles (NPs) with high crystallinity and uniformity using the hot-injection method. The optical properties of TlSnX3 NPs are fine-tuned by substituting the halide ions of TlSnX3. In addition, the oxidation of Sn in TlSnX3 NPs is effectively prevented by the strong reducing ligands such as dioleamide (DOA) and trioctylphosphine (TOP). Furthermore, TlSnX3 NPs-based perovskite solar cells (PSCs) are fabricated by a spin-coating method; they exhibited a high open-circuit voltage (∼1.4 V). These results demonstrate that TlSnX3 NPs can be an attractive candidate for solution-processable optoelectronic devices.
Introduction
Organo-inorganic hybrid perovskite materials are emerging as the most promising candidates for optoelectronic applications such as photovoltaics, light-emitting diodes, photodetectors, and lasers (Xing et al., 2013; Xing et al., 2014; Cho et al., 2015; Zhu et al., 2015; Kwon et al., 2023; Zhang et al., 2023). Organo-inorganic hybrid perovskites are chemical compounds with the structural formula ABX3, where the A site is occupied by an organic ammonium ion (CH3NH3+ and HC(NH2)2+), B is a p-block metal cation (Sn2+ and Pb2+), and X is a halide anion (Cl−, Br−, and I−). Recently, organo-inorganic hybrid perovskites based on lead (Pb) ion at the B site have attracted significant attention because perovskite solar cells (PSCs) based on them have shown a certified photoconversion efficiency (PCE) of 26.1% (National Renewable Energy Laboratory, 2023). Furthermore, light-emitting diodes based on CH3NH3PbX3 (X = halide ion) exhibit a high external quantum efficiency because they have high color purity (Cho et al., 2015). Their unique optoelectronic properties demonstrated that perovskites based on Pb are one of the strongest candidates for next-generation optoelectronic devices (Xing et al., 2013; Xing et al., 2014; Cho et al., 2015; Zhu et al., 2015).
However, the main challenge in the commercialization of Pb-based perovskite materials is the European Union restriction on Pb-containing materials in consumer electronics (Restriction of Hazardous Substances, RoHS) (Luo et al., 2022; Pancini et al., 2023). Thus, tin (Sn) has been suggested as an alternative p-block metal cation for Pb-free perovskite materials (Takahashi et al., 2011; Cao and Yan, 2021). The electrical properties of an Sn-based perovskite system can be easily controlled by chemical stoichiometry. Mitzi et al. reported that organic-based layered halide perovskites such as (C4H9NH3)2(CH3NH3)n-1Snnl3n+1 showed transition from semiconducting to metallic properties with increasing n (Mitzi et al., 1994). Further, Chung et al. demonstrated that an Sn-based perovskite exhibited high electrical conductivity and strong near-infrared photoluminescence (Chung et al., 2012).
Another key challenge with organo-inorganic hybrid perovskite materials is the instability of the organic cation in the A site. Organic cations such as CH3NH3+ and HC(NH2)2+ can suffer from compositional degradation at high temperatures and/or in humid atmospheres. To improve the stability of the perovskite, the organic cation in the A site is substituted with an alkali metal ion such as cesium (Cs) or rubidium (Rb) (Gou et al., 2017). However, inorganic perovskite materials based on a Cs ion in the A site exhibit temperature-sensitive phase transitions. Møller et al. demonstrated that the crystal structures of CsPbCl3 and CsPbBr3 could be distorted even at room temperature (Møller, 1959).
Therefore, to overcome the disadvantage of inorganic perovskite materials based on Cs or Rb, it is necessary to consider alternatives to occupy the A site of perovskite materials. Thallium (Tl) is the heaviest nonradioactive element among p-block metals, which can exist as a monovalent or trivalent cation. Its chemical behavior closely resembles that of alkali metals (Salmon, 1963). Shand et al. reported that monovalent Tl compounds are more stable than trivalent thallium compounds (Shand et al., 1998). Tl could be the most promising candidate for perovskite materials because perovskite structures based on Tl show no phase transition induced by temperature (Stoeger, 1997). In addition, Tl-based perovskite structure such as TlPbI3 has lower total energy than CsPbI3 because a strong bond occurs between Tl and I (Liu et al., 2017). Khyzhun et al. demonstrated that TlPbI3 single crystals could be grown in bulk form by using the Bridgman–Stockbarger technique (Khyzhun et al., 2016). However, this approach requires a high temperature and pressure to fabricate the single-crystal structure, presenting a limitation for diverse applications.
To solve the aforementioned limitations, we synthesized TlSnX3 (X = Cl, Br, and I) perovskite structures as nanoparticles (NPs) by using ligands, dioleamide (DOA) and trioctylphosphine (TOP) ligands, which are strong reducing agents. By using the hot-injection method, TlSnX3 NPs with high uniformity and crystallinity were obtained and applied to solution-processable optoelectronic devices. Furthermore, we demonstrate unique optoelectronic properties of Tl-based perovskite nanostructures, which have not been reported by downsizing perovskite nanostructures.
Materials and methods
Materials
Thallium (I) acetate (CH3CO2Tl, 99%), oleic acid (CH3(CH2)7CH=CH(CH2)7COOH, 90%, OAc), oleylamine (CH3(CH2)7CH=CH(CH2)7CH2NH2, 70%, OAm), tin (II) iodide (SnI2, 99.99%), tin (II) bromide (SnBr2, 99.99%), tin (II) chloride (SnCl2, 99.99%), TOP (97%), anhydrous 1-butanol (99.8%), n-octane (extra pure, ≥99%), chlorobenzene (CB, anhydrous, 99.8%), acetonitrile (ACN, anhydrous, 99.8%), and Li-bis(trifluoromethanesulfonyl) imide (Li-TFSI) were purchased from Sigma Aldrich. Tin (IV) oxide (SnO2 NPs, 15% in H2O Colloidal dispersion) was purchased from Alfa Aesar. 2,20,7,70-Tetrakis(N,N-di-p-methoxyphenylamine)-9,90-spirobifluorene (Spiro-OMeTAD) and tris(2-(1Hpyrazol-1-yl)-4-tert-butylpyridine)-cobalt(III) tris(bis(trifluoromethylsulfonyl)imide) (FK209) were purchased from Lumtec. 2-Amylpyridine (≥98.0%) was purchased from TCI.
Synthesis of dioleamide (DOA)
DOA was prepared by adding a 1:1 molar mixture of OAc and OAm in a three-neck flask under an argon atmosphere. The mixture was then heated to 120 °C for 12 h with consistent, vigorous stirring. The reaction occurs through the dehydration condensation of the carboxylic group of OAc and the amine group of OAm (Cara et al., 2015). After the reaction was completed, the product was degassed under vacuum at 100 °C for 1 h to remove moisture generated during reaction. Finally, the resulting yellow liquid was cooled to room temperature and stored in the refrigerator.
Synthesis of TlSnX3 NPs
DOA-capped TlSnX3 nanoparticles (DOA-TlSnX3 NPs) were synthesized using a modified hot-injection method (Protesescu et al., 2015). CH3CO2Tl (0.32 g), DOA (2 ml), and 1-octdecene (50 ml) were loaded into a three-neck flask and degassed under vacuum at 80 °C for 1 h. After the flask was filled with argon, the mixed solution was heated to 120 °C and vigorously stirred for 12 h until all the CH3CO2Tl was dissolved. Subsequently, the Tl precursor mixture was heated to 175 °C. After further stirring for 2 h, 5 ml of 1M solution of SnX2 (X = Cl, Br, I) dissolved in TOP was injected into the prepared Tl precursor solution. Then, the mixed solution was heated at 170 °C for 10 s and then ice-cold water bath was placed under the three-neck flask to quench further TlSnX3 NPs growth. Purification of the as-synthesized TlSnX3 NPs were performed in an argon-filled glovebox. The resulting NPs in solution were washed by centrifugation (8,000 rpm, 5 min) three times with excess 1-butanol to remove surfactant residuals. The precipitated final products were dispersed in hexane. The supernatant was collected and stored in the dark at 4 °C for 48 h, after which the precipitated products were removed. The remaining n-hexane was dried under vacuum, and the dried TlSnX3 NP pellets were redispersed in n-octane at a concentration of ∼75 mg/mL.
Fabrication of TlSnX3 NP-based solar cell device
Patterned indium tin oxide (ITO, 8 Ω/sq) substrates were sequentially cleaned by sonicating in detergent water, deionized water, acetone, and isopropyl alcohol for 10 min and then dried in an oven. Next, ultraviolet ozone (UVO) treatment was performed to remove the organic residues on the substrates. For the preparation of the electron transporting layer (ETL), SnO2 NPs were diluted with deionized water at a weight ratio of 1:6. This solution was then deposited on UVO-treated ITO substrates by spin-coating at 3,000 rpm for 30 s, followed by annealing at 150 °C for 30 min. After transfer to the glovebox, to fabricate TlSnX3 NP-based light-absorbing layers, a solution of TlSnX3 NPs in n-octane (75 mg/mL) was spin-coated onto the SnO2/ITO substrates at 1,000 rpm for 20 s, followed by 2000 rpm for 5 s. For the preparation of the hole-transporting layer, Spiro-OMeTAD was dissolved in CB with 39-µL 2-amylpyridine, 23-µL Li-TFSI (520 mg/mL in ACN), and 5-µL FK209 (180 mg/mL in ACN). This solution was spin-coated at 4,000 rpm for 30 s. Finally, an 80-nm-thick Au electrode was deposited by using a thermal evaporator.
Characterization of TlSnX3 NPs and devices
Attenuated total reflectance-Fourier transform infrared spectroscopy (ATR-FTIR) (Nicolet iS50, Thermo Fisher Scientific) was used to characterize the synthesis of DOA. High-resolution transmission electron microscopy (HR-TEM) (FEI, TECNAI G2 F30 ST, at 100 kV) was performed to investigate as-synthesized TlSnX3 NPs. The crystal structure of the TlSnX3 NPs was examined by an X-ray diffractometer (XRD) (Rigaku, DMAX-RB) operating at 20 kV and 200 mA with a rotating anode and Cu-Kα radiation of 0.15418 nm. X-ray photoelectron spectroscopy (XPS) was used to analyze the binding state of TlSnX3 (X = Cl, Br, and I) by using an AXIS-HIS spectrometer with monochromatic Al Kα radiation (1,486.3 eV). XPS data were acquired in the range of 0–1,400 eV with a constant pass energy of 117.4 eV at a nominal photoelectron takeoff angle of 45°. The steady-state photoluminescence (PL) spectra of the TlSnX3 NPs were obtained by excitation at 550 nm using a Fluorolog3-22 photoluminescence spectrometer system with a monochromator (iHR320, HORIBA Scientific). Commission Internationale de l'Eclairage (CIE) coordinates of TlSnX3 NPs were measured using a visible-light spectrometer (DARSA PRO-5200, PSI) equipped with an integrating sphere at an applied current of 20 mA. The ultraviolet-visible (UV–Vis) absorption spectra of TlSnX3 NPs were examined using a Lambda 35 spectrometer (PerkinElmer). Ultraviolet photoelectron spectroscopy (UPS) measurements were performed with a scanning XPS microprobe (PHI 5000 Versa Probe, Ulvac-PHI) using HeI (21.2 eV). The morphology of a TlSnX3 NP film was analyzed by field-emission scanning electron microscopy (FE-SEM) (Verios G4 UC, FEI). Under an N2 atmosphere, the current density–voltage (J−V) curves of the devices were measured using a Keithley 2,400 source under a xenon-lamp-based solar simulator (Newport 91160s, AAA class). A Si solar cell calibrated by the National Renewable Energy Laboratory was employed to adjust the light intensity to the AM 1.5G 1-sun condition (100 mW/cm2). The voltage sweep rate was 20 mV with a delay of 20 ms for the reverse (forward) sweeping direction from 1.5 V (−0.1 V) to −0.1 V (1.5 V). The devices were evaluated using an SUS304 aperture with an area of 0.09 cm2.
Results
Synthesis of TlSnX3 NPs
Figure 1A illustrates the overall synthesis process of TlSnX3 (X = Cl, Br, and I) perovskite NPs. Initially, Tl-oleate was prepared by vigorously agitating Tl+ ions together with the synthesized DOA (step 1). For the preparation of Tl-oleate, we investigated various ligands to achieve the synthesis of highly dispersed TlSnX3 nanoparticles in nonpolar solvents. However, TlSnX3 NPs were not successfully synthesized when OAm or OAc was used as the ligand for the Tl ion. Moreover, the size and shape control of TlSnX3 NPs by using these ligands is considerably challenging. To address these limitations, we synthesized DOA with two functional groups and employed it as an alternative ligand for the preparation of Tl precursors. DOA was synthesized by combining OAc and OAm in a 1:1 ratio. An angle of 120° between the carboxyl group of OAc and amine group of OAm leads to the formation of amide bonds between the acid and amine groups. As shown in Figure 1B, FTIR spectra indicate that the carboxyl acid peak of OAc and the amine peak of OAm have disappeared, which confirms the amide bonding. Thus, we demonstrate the presence of DOA with two functional groups. Subsequently, SnX2 was dissolved in TOP, a coordinating ligand, under an inert atmosphere (step 2). TOP is a strong reducing agent that can prevent the oxidization to Sn4+ during the synthesis. Finally, this mixture was injected into a solution containing Tl-oleate at 170 °C, yielding colloidally stable TlSnX3 NPs (for details, see the Experimental section). Figures 1C–E show TlSnI3 NPs synthesized by using various ligands. When TlSnI3 NPs were synthesized by using an OAm-Tl precursor, they exhibited aggregation (Figure 1C). Furthermore, the combination of OAc and OAm (the molar ratio of OAc/OAm = 80%) resulted in nonuniform and rectangular cube-shaped NPs (Figure 1D). By contrast, DOA-capped TlSnI3 NPs showed high size uniformity without aggregation (Figure 1E). According to a previous study, as the ratio of OAc increases, the shape of Au NPs becomes anisotropic, and larger particles can be obtained because the excess OAc can adsorb on the specific facet (111) and hinder further growth of the (111) facet (Mohamed et al., 2010). Further, Calatayud et al. reported that the shape and size can be controlled by the ratio of OAc and OAm. An excess of OAc selectively binds to the {001} facets of TiO2 NPs, which leads to a truncated octahedron or nanorod shape of TiO2 NPs because of the higher electronegativity of the carboxylic group of OAc (Calatayud et al., 2013). Unlike the case of the combination of OAc and OAm (molar ratio of OAc/OAm = 80%), a high size uniformity of DOA-capped TlSnI3 NPs is attributed to the pre-synthesized DOA ligand. Water molecules, which can degrade the perovskite structure, are the byproduct of the condensation between OAc and OAm (Cara et al., 2015). Thus, when the combination of OAc and OAm (the molar ratio of OAc/OAm = 80%) is used as the ligand, water molecules can be generated and degrade the perovskite structure during the synthesis process. On the contrary, as the water molecules are pre-eliminated during the synthesis of the DOA ligand, the perovskite structure can be preserved, which results in the high size uniformity of TlSnI3 NPs.
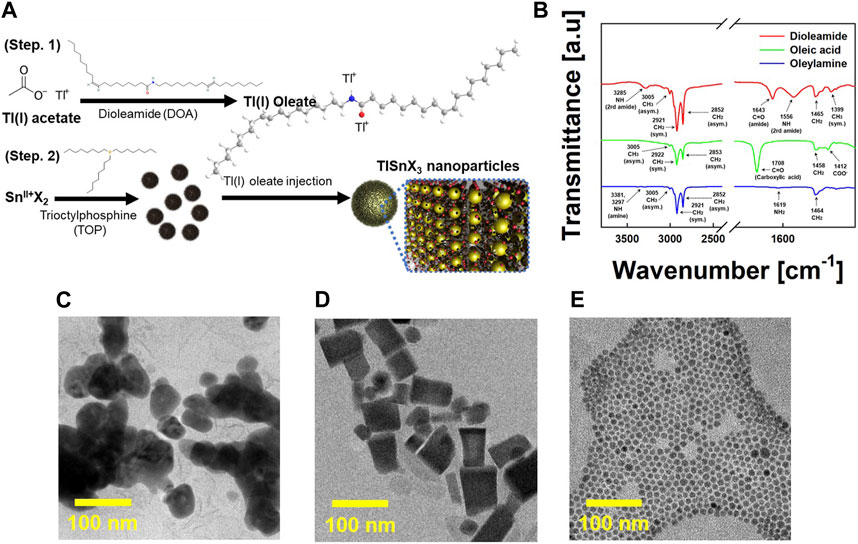
FIGURE 1. (A) Schematic of the synthesis process of TlSnX3 nanoparticles (NPs) (X = Cl, Br, and I). (B) Fourier-transform infrared spectroscopy (FTIR) spectra of dioleamide (DOA, red), oleic acid (OAc, green), and oleylamine (OAm, blue). High-resolution (HR) transmission electron microscopy (HR-TEM) of TlSnI3 perovskite NPs, synthesized by using (C) OAm, (D) the combination of OAc and OAm (the molar ratio of OAc/OAm = 80%) and (E) DOA.
Structural and morphological characterization of TlSnX3 NPs
The HR-TEM images in Figure 2A depict the DOA-capped TlSnX3 NPs with various halogen ions at the X site (X = Cl, Br, and I) exhibiting high size uniformity (diameter: 15 nm) without aggregation. As shown in Figure 2B, all peaks in the XRD pattern indicate that DOA-capped TlSnX3 perovskite NPs have a cubic crystal structure similar to that of CsSnX3 NPs (Jellicoe et al., 2016). The broadening of the peaks observed in the XRD patterns, known as Scherrer broadening, can be attributed to the nano-sized formation of TlSnX3 NPs. Interestingly, with halide ion substitution from I− to Br−to Cl−, the 2θ values decreased. Notably, these trends have been frequently observed in cases of other metal halide perovskite analogues (Akkerman et al., 2015; Jang et al., 2015; Nedelcu et al., 2015). Supplementary Figure S1A depicts that Tl ions and halogen (X) ions occupy the (100) plane of the TlSnX3 perovskite structure. The (100) plane is exposed to the outer surface of TlSnX3, which affords a higher adsorption capacity of excess DOA ligands on the surface of TlSnX3 NPs. These results demonstrate that TlSnX3 NPs satisfy the Goldschmidt tolerance factor (Green et al., 2014). Thus, they can form stable cubic crystal structures (Supplementary Figure S1B).
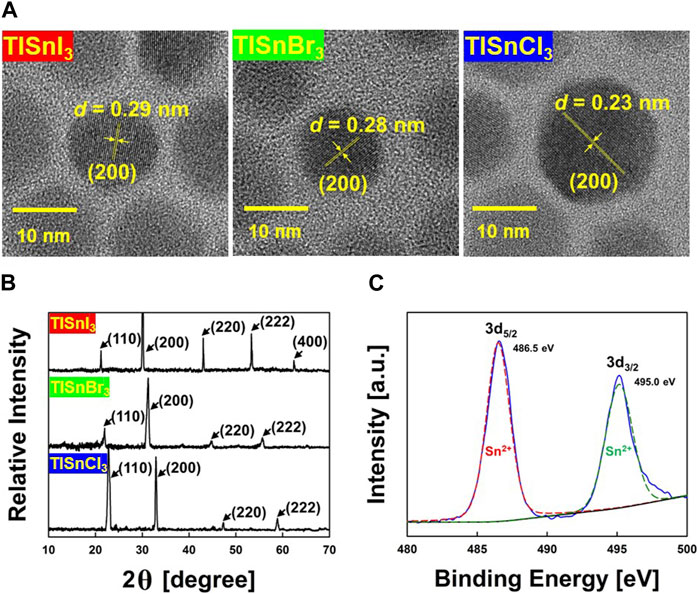
FIGURE 2. (A) HR-TEM Images of TlSnI3 (left), TlSnBr3 (middle), and TlSnCl3 (right) perovskite NPs. (B) XRD patterns of TlSnI3, TlSnBr3, and TlSnCl3 perovskite NPs. (C) X-ray photoelectron spectroscopy (XPS) spectra of as-synthesized TlSnI3 perovskite NPs. The binding energy core levels of the Sn 3d3/2 and Sn 3d5/2 were measured at 494.5 and 486.9 eV, respectively.
The major challenge associated with Sn-based perovskite structures lies in their chemical instability when exposed to an ambient atmosphere because the presence of Sn2+ in the perovskite crystal structure makes them vulnerable to oxidation, which could lead to the formation of Sn4+. Therefore, the TOP ligand was used as a reducing agent, which efficiently prevented the oxidation of Sn2+ within the perovskite structure. To assess the chemical stability of TlSnX3 NPs, we performed XPS measurements. As observed in the XPS spectra in Figure 2C, there are two distinct peaks at 494.5 and 486.9 eV corresponding to the Sn 3d5/2 and Sn 3d3/2 transitions, respectively. For the passivation approach, two electrons are provided to the d-orbital of Sn by L-type ligands such as TOP and DOA, which effectively prevents the oxidation of Sn in the TlSnX3 NCs (Shukla et al., 2003; Mourdikoudis and Liz-Marzán, 2013; Stranks et al., 2014). Thus, these results demonstrate that both TOP and DOA ligands contribute to the prevention of Sn oxidation, which thereby leads to the successful synthesis of a chemically stable cubic phase of TlSnX3 NPs with high size uniformity.
Optical characterization of TlSnX3 NPs
To investigate the optical properties of the TlSnX3 NPs, absorbance and PL measurement were performed. In Figure 3A, the absorption onsets of TlSnI3, TlSnBr3, and TlSnCl3 NPs are 601 nm (2.00 eV), 552 nm (2.42 eV), and 438 nm (2.85 eV), respectively. Further, the PL peaks of TlSnX3 NPs are blue-shifted by substituting halide ions from I− to Br− to Cl−. Specifically, TlSnI3 NPs exhibited a red emission peak at 601 nm with a full width at half maximum (FWHM) of 30 nm, TlSnBr3 NPs displayed a green emission peak at 472 nm with an FWHM of 50 nm, and TlSnCl3 NCs showed a blue emission peak at 418 nm with an FWHM of 65 nm. Notably, TlSnI3 NPs exhibited no Stokes shift, whereas TlSnCl3 NPs exhibited a Stokes shift because of a stronger structural distortion in the excited state by a shorter Sn–Cl bond (Zhou et al., 2018). These results indicate that RGB color variation is achieved by modulating the halogen composition of TlSnX3 NPs.
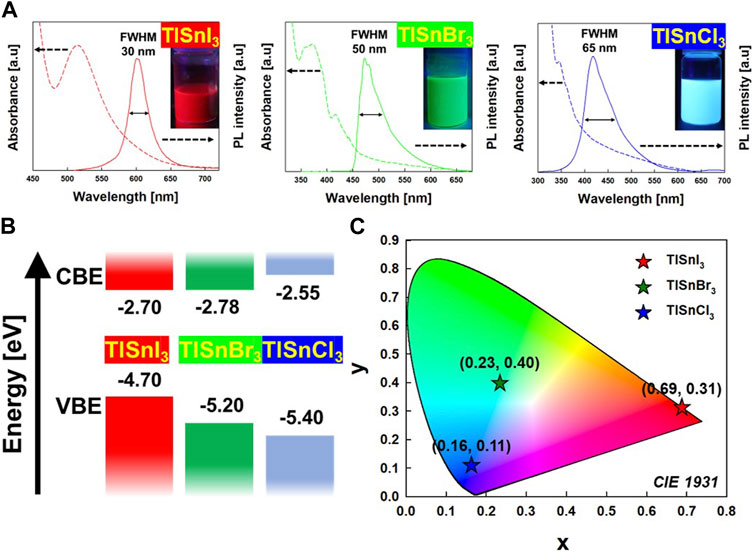
FIGURE 3. (A) Absorbance (dotted line) and photoluminescence (PL) (solid line) spectra of TlSnI3 (left), TlSnBr3 (middle), and TlSnCl3 (right) perovskite NPs. (B) Band diagram of TlSnI3 (red color), TlSnBr3 (green color), and TlSnCl3 (blue color) perovskite NPs. (C) The Commission Internationale del’Eclairage (CIE 1931) diagram of TlSnI3 (red color), TlSnBr3 (green color), and TlSnCl3 (blue color) perovskite NPs.
Moreover, we performed UPS measurements to investigate the precise position of the valence band and comprehend the electronic structure of the material. Figure 3B shows the energy band diagram of TlSnX3. The electronic band structure is predominantly determined by the orbitals of [SnX6]4− octahedra, with a minor contribution by electrons originating from the A site (Tl). The band gap of TlSnX3 becomes larger by substituting halide ions (X) from I− to Br− and Cl−. This trend is attributed to the increasing electronegativity of the halide ions (electronegativity: Cl > Br > I) (Matheu et al., 2022).
To investigate the emission colors of TlSnX3 NPs, the CIE 1931 chromaticity diagram was used. This diagram displays the dominant emission color of a sample by using chromaticity coordinates (x, y), which represent the blue, green, and red constituents of the emission. These coordinates indicate the dominant emission colors displayed by the NPs. As shown in Figure 3C, the CIE coordinates of the red (TlSnI3), green (TlSnBr3), and blue (TlSnCl3) emissions are (0.69, 0.31), (0.23, 0.40), and (0.16, 0.11), respectively. This CIE diagram is consistent with the PL spectra and photographs from solution emission color data (Figure 3A). These results confirm that TlSnX3 NPs are attractive materials for optoelectronic applications.
Application of TlSnX3 NPs to optoelectronic devices
As TlSnI3 has a suitable electronic band structure for solar cells, we applied it as a light-absorption layer for PSCs. Cross-section SEM image shows that the TlSnI3 was spin-coated on a SnO2 ETL to fabricate an 88-nm-thick dense and crack-free film (Figure 4A). Figure 4B shows the photocurrent density–voltage (J–V) curves of the TlSnI3-based PSCs (device structure of ITO/SnO2/TlSnI3/Spiro-OMeTAD/Au) under forward/reverse bias. The TlSnI3-based PSC exhibited a short-circuit current density (JSC) of 0.67 mA/cm2, open-circuit voltage (VOC) of 1.41 V, fill factor (FF) of 49.86%, and a PCE of 0.44% under forward bias. Despite the relatively low PCE of the TlSnI3-based PSC, the high value of VOC (1.41 V) demonstrates the feasibility of TlSnI3 NPs for solar cell application. We can expect the improvement of photovoltaic performance by substituting the short-chain ligands for DOA ligands during the fabrication process of devices, which could mitigate the charge recombination and enhance the charge transport within the solar cell (Kim et al., 2019). Furthermore, we can use more precise coating method, such as the layer-by-layer method, to control the thickness of the TlSnI3 layer, which could increase the light absorption capabilities and JSC (Sanehira et al., 2017). Moreover, TlSnX3 (X = I, Br, and Cl) films emitted vivid RGB colors, namely, red (TlSnI3), green (TlSnBr3), and blue (TlSnCl3), which strongly support their feasibility for light-emitting devices (Figure 4C). These results demonstrate that TlSnX3 (X = I, Br, and Cl) NPs are promising candidates for optoelectronic applications.
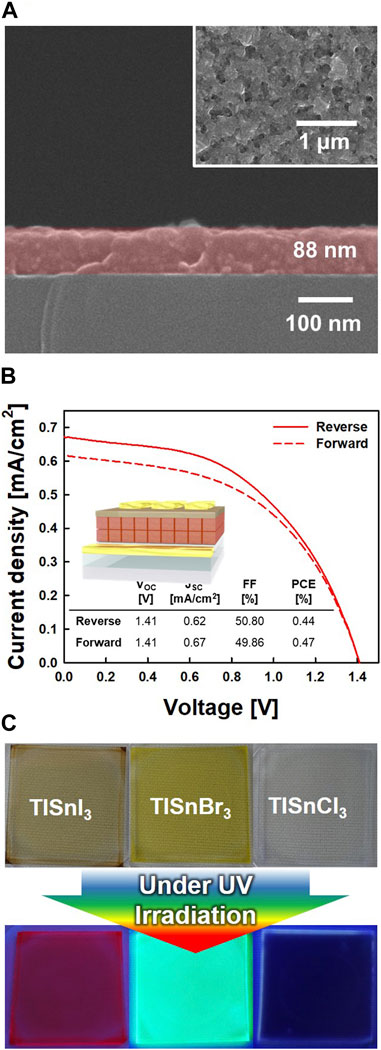
FIGURE 4. (A) Cross-section scanning electron microscopy (SEM) image of TlSnI3 film coated on SnO2 electron transporting layer (ETL) (Inset: surface SEM image of TlSnI3 film). (B) Photocurrent density-voltage (J-V) curves of TlSnI3 based perovskite solar cells (PSCs) measured at reverse (solid line) and forward (dotted line) scans. (C) The photographs of TlSnI3 (red emission color), TlSnBr3 (green emission color), and TlSnCl3 (blue emission color) films under UV irradiation.
Discussion
In conclusion, we successfully synthesized TlSnX3 (X = I, Br, Cl) NPs using DOA and TOP ligands, which are strong reducing agents. Both DOA and TOP ligands effectively prevent the oxidation of Sn2+, which leads to a chemically stable cubic phase of TlSnX3 NPs. Furthermore, we successfully tuned the optical bandgap of TlSnX3 NPs by substituting different halides in the perovskite structure. The PL peaks of TlSnX3 NPs were blue-shifted under halide ion substitution from I− to Br− to Cl−. Additionally, according to the CIE 1931 diagram, the dominant emission colors by TlSnI3, TlSnBr3, and TlSnCl3 were red, green, and blue, respectively. Moreover, the electronic structure determined by UPS and UV–Vis spectroscopy also demonstrated that with halide ion substitution from I−to Br− to Cl−, the band gap of TlSnX3 became larger, which facilitated the control of RGB color. On the basis of these results, TlSnI3 NPs were used as light absorbers, and the fabricated device exhibited a JSC of 0.67 mA/cm2, VOC of 1.41 V, FF of 49.86%, and PCE of 0.44% under forward bias. Furthermore, the TlSnX3 (X = I, Br, and Cl) films emitted vivid RGB colors, which strongly support their feasibility for light-emitting devices. These results demonstrate that TlSnX3 (X = I, Br, and Cl) NPs are promising candidates for optoelectronic applications. In the future, to enhance the performance of optoelectronic devices based on TlSnX3 NPs, it is necessary to investigate the incorporation of additives or deposition processes, with the goal of preventing Sn2+ oxidation and controlling defects of TlSnX3 NPs. Furthermore, it is vital to study the application of encapsulation layers or the insertion of interlayers to protect TlSnX3 NPs from external stimuli. These studies will not only improve the long-term stability of TlSnX3 NPs-based devices but also reduce the risk of metal ion leakage into the environment by the degradation of TlSnX3 NPs (Babayigit et al., 2016; Vashishtha et al., 2018). Our research provides valuable insights into the utilization of TlSnX3 NPs in optoelectronic devices, and further investigations hold the promise of enhancing its performance and stability.
Data availability statement
The original contributions presented in the study are included in the article/Supplementary Material, further inquiries can be directed to the corresponding authors.
Author contributions
WK: Data curation, Formal Analysis, Methodology, Writing–original draft. BK: Conceptualization, Formal Analysis, Methodology, Writing–original draft. MJK: Conceptualization, Methodology, Supervision, Writing–original draft, Writing–review and editing. HJ: Funding acquisition, Investigation, Methodology, Resources, Supervision, Writing–original draft, Writing–review and editing.
Funding
The authors declare financial support was received for the research, authorship, and/or publication of this article. This work was supported by the Korea Institute of Science and Technology (KIST) institutional programs (HJ). This work was supported by the Korea Institute of Energy Technology Evaluation and Planning (KETEP) grant funded by the Korea government (MOTIE) (20221B1010003B, Integrated High-Quality Technology Development of Remanufacturing Spent Cathode for Low Carbon Resource Recirculation) (MJK).
Conflict of interest
The authors declare that the research was conducted in the absence of any commercial or financial relationships that could be construed as a potential conflict of interest.
Publisher’s note
All claims expressed in this article are solely those of the authors and do not necessarily represent those of their affiliated organizations, or those of the publisher, the editors and the reviewers. Any product that may be evaluated in this article, or claim that may be made by its manufacturer, is not guaranteed or endorsed by the publisher.
Supplementary material
The Supplementary Material for this article can be found online at: https://www.frontiersin.org/articles/10.3389/fmats.2023.1298188/full#supplementary-material
References
Akkerman, Q. A., D’Innocenzo, V., Accornero, S., Scarpellini, A., Petrozza, A., Prato, M., et al. (2015). Tuning the optical properties of cesium lead halide perovskite nanocrystals by anion exchange reactions. J. Am. Chem. Soc. 137, 10276–10281. doi:10.1021/jacs.5b05602
Babayigit, A., Thanh, D. D., Ethirajan, A., Manca, J., Muller, M., Boyen, H.-G., et al. (2016). Assessing the toxicity of Pb- and Sn-based perovskite solar cells in model organism Danio rerio. Sci. Rep. 6, 18721. doi:10.1038/srep18721
Calatayud, D. G., Jardiel, T., Rodriguez, M., Peiteado, M., Fernandez-Hevia, D., and Caballero, A. C. (2013). Soft solution fluorine-free synthesis of anatase nanoparticles with tailored morphology. Ceram. Int. 39, 1195–1202. doi:10.1016/j.ceramint.2012.07.044
Cao, J., and Yan, F. (2021). Recent progress in tin-based perovskite solar cells. Energy enivron. Sci. 14, 1286–1325. doi:10.1039/D0EE04007J
Cara, C., Musinu, A., Mameli, V., Ardu, A., Niznansky, D., Bursik, J., et al. (2015). Dialkylamide as both capping agent and surfactant in a direct solvothermal synthesis of magnetite and titania nanoparticles. Cryst. Growth Des. 15, 2364–2372. doi:10.1021/acs.cgd.5b00160
Cho, H., Jeong, S.-H., Park, M.-H., Kim, Y.-H., Wolf, C., Lee, C.-L., et al. (2015). Overcoming the electroluminescence efficiency limitations of perovskite light-emitting diodes. Science 350, 1222–1225. doi:10.1126/science.aad1818
Chung, I., Song, J.-H., Im, J., Androulakis, J., Malliakas, C. D., Li, H., et al. (2012). CsSnI3: semiconductor or metal? High electrical conductivity and strong near-infrared photoluminescence from a single material. High hole mobility and phase-transitions. J. Am. Chem. Soc. 134, 8579–8587. doi:10.1021/ja301539s
Gou, G., Young, J., Liu, X., and Rondinelli, J. M. (2017). Interplay of cation ordering and ferroelectricity in perovskite tin iodides: designing a polar halide perovskite for photovoltaic applications. Inorg. Chem. 56, 26–32. doi:10.1021/acs.inorgchem.6b01701
Green, M. A., Ho-Baillie, A., and Snaith, H. J. (2014). The emergence of perovskite solar cells. Nat. Photonics 8, 506–514. doi:10.1038/nphoton.2014.134
Jang, D. M., Park, K., Kim, D. H., Park, J., Shojaei, F., Kang, H. S., et al. (2015). Reversible halide exchange reaction of organometal trihalide perovskite colloidal nanocrystals for full-range band gap tuning. Nano Lett. 15, 5191–5199. doi:10.1021/acs.nanolett.5b01430
Jellicoe, T. C., Richter, J. M., Glass, H. F. J., Tabachnyk, M., Brady, R., Dutton, S. E., et al. (2016). Synthesis and optical properties of lead-free cesium tin halide perovskite nanocrystals. J. Am. Chem. Soc. 138, 2941–2944. doi:10.1021/jacs.5b13470
Khyzhun, O. Y., Fochuk, P. M., Kityk, I. V., Piasecki, M., Levkovets, S. I., Fedorchuk, A. O., et al. (2016). Single crystal growth and electronic structure of TlPbI3. Mat. Chem. Phys. 172, 165–172. doi:10.1016/j.matchemphys.2016.01.058
Kim, J., Koo, B., Kim, W.-H., Choi, J., Choi, C., Lim, S. J., et al. (2019). Alkali acetate-assisted enhanced electronic coupling in CsPbI3 perovskite quantum dot solids for improved photovoltaics. Nano Energy 66, 104130. doi:10.1016/j.nanoen.2019.104130
Kwon, N., Lee, J., Ko, M. J., Kim, Y. Y., and Seo, J. (2023). Recent progress of eco-friendly manufacturing process of efficient perovskite solar cells. Nano Converg. 10, 28. doi:10.1186/s40580-023-00375-5
Liu, Z., Zhang, T., Wang, Y., Wang, C., Zhang, P., Sarvari, H., et al. (2017). Electronic properties of a new all-inorganic perovskite TlPbI3 simulated by the first principles. Nanoscale Res. Lett. 12, 232. doi:10.1186/s11671-017-2015-y
Luo, H., Li, P., Ma, J., Han, L., Zhang, Y., and Song, Y. (2022). Sustainable Pb management in perovskite solar cells toward eco-friendly development. Adv. Energy Mat. 12, 2201242. doi:10.1002/aenm.202201242
Matheu, R., Vigil, J. A., Crace, E. J., and Karunadasa, H. I. (2022). The halogen chemistry of halide perovskites. Trends Chem. 4, 206–219. doi:10.1016/j.trechm.2021.12.002
Mitzi, D. B., Feild, C. A., Harrison, W. T. A., and Guloy, A. M. (1994). Conducting tin halides with a layered organic-based perovskite structure. Nature 369, 467–469. doi:10.1038/369467a0
Mohamed, M. B., AbouZeid, K. M., Abdelsayed, V., Aljarash, A. A., and El-Shall, S. (2010). Growth mechanism of anisotropic gold nanocrystals via microwave synthesis: formation of dioleamide by gold nanocatalysis. ACS Nano 4, 2766–2772. doi:10.1021/nn9016179
Møller, C. K. (1959). The structure of caesium plumbo iodide CsPbI3. Mat. Fys. Medd. Dan. Vid. Selsk. 32, 2.
Mourdikoudis, S., and Liz-Marzán, L. M. (2013). Oleylamine in nanoparticle synthesis. Chem. Mat. 25, 1465–1476. doi:10.1021/cm4000476
National Renewable Energy Laboratory (NREL) (2023). Best solar cell efficiencies chart. Available at https://www.nrel.gov/pv/assets/pdfs/best-research-cell-efficiencies.pdf (Accessed on July 26, 2023).
Nedelcu, G., Protesescu, L., Yakunin, S., Bodnarchuk, M. I., Grotevent, M. J., and Kovalenko, M. V. (2015). Fast anion-exchange in highly luminescent nanocrystals of cesium lead halide perovskites (CsPbX3, X = Cl, Br, I). Nano Lett. 15, 5635–5640. doi:10.1021/acs.nanolett.5b02404
Pancini, L., Montecucco, R., Larini, V., Benassi, A., Mirani, D., Pica, G., et al. (2023). A fluorescent sensor to detect lead leakage from perovskite solar cells. Mat. Adv. 4, 2410–2417. doi:10.1039/D3MA00068K
Protesescu, L., Yakunin, S., Bodnarchuk, M. I., Krieg, F., Caputo, R., Hendon, C. H., et al. (2015). Nanocrystals of cesium lead halide perovskites (CsPbX3, X = Cl, Br, and I): novel optoelectronic materials showing bright emission with wide color gamut. Nano Lett. 15, 3692–3696. doi:10.1021/nl5048779
Sanehira, E. M., Marshall, A. R., Christians, J. A., Harvey, S. P., Ciesielski, P. N., Wheeler, L. M., et al. (2017). Enhanced mobility CsPbI3 quantum dot arrays for record-efficiency, high-voltage photovoltaic cells. Sci. Adv. 3, eaao4204. doi:10.1126/sciadv.aao4204
Shand, P., Edmunds, W. M., and Ellis, J. (1998). The hydrogeochemistry of thallium in natural waters” in Water–rock interaction: proceedings of the 9th International symposium on water–rock interaction. 1st ed. Taupo, FL: CRC Press, 75–78.
Shukla, N., Liu, C., Jones, P. M., and Weller, D. (2003). FTIR study of surfactant bonding to FePt nanoparticles. J. Magn. Magn. Mat. 266, 178–184. doi:10.1016/s0304-8853(03)00469-4
Stranks, S. D., Burlakov, V. M., Leijtens, T., Ball, J. M., Goriely, A., and Snaith, H. J. (2014). Recombination kinetics in organic-inorganic perovskites: excitons, free charge, and subgap states. Phys. Rev. Appl. 2, 034007. doi:10.1103/physrevapplied.2.034007
Takahashi, Y., Obara, R., Lin, Z.-Z., Takahashi, Y., Naito, T., Inabe, T., et al. (2011). Charge-transport tin-iodide perovskite CH3NH3SnI3: origin of high conductivity. Dalton Trans. 40, 5563–5568. doi:10.1039/C0DT01601B
Vashishtha, P., Metin, D. Z., Cryer, M. E., Chen, K., Hodgkiss, J. M., Gaston, N., et al. (2018). Shape-size-and composition-controlled thallium lead halide perovskite nanowires and nanocrystals with tunable band gaps. Chem. Mat. 30, 2973–2982. doi:10.1021/acs.chemmater.8b00421
Xing, G., Mathews, N., Lim, S. S., Yantara, N., Liu, X., Sabba, D., et al. (2014). Low-temperature solution-processed wavelength-tunable perovskites for lasing. Nat. Mat. 13, 476–480. doi:10.1038/nmat3911
Xing, G., Mathews, N., Sun, S., Lim, S. S., Lam, Y. M., Grätzel, M., et al. (2013). Long-range balanced electron- and hole-transport lengths in organic-inorganic CH3NH3PbI3. Science 342, 344–347. doi:10.1126/science.1243167
Zhang, Z., Kim, W., Ko, M. J., and Li, Y. (2023). Perovskite single-crystal thin films: preparation, surface engineering, and application. Nano Converg. 10, 23. doi:10.1186/s40580-023-00373-7
Zhou, C., Worku, M., Neu, J., Lin, H., Tian, Y., Lee, S., et al. (2018). Facile preparation of light emitting organic metal halide crystals with near-unity quantum efficiency. Chem. Mat. 30, 2374–2378. doi:10.1021/acs.chemmater.8b00129
Keywords: lead-free pseudo-alkali metal halide perovskite, nanocrystals, dioleamide ligands, perovskite solar cells, optoelectronics
Citation: Kim W, Koo B, Ko MJ and Jung H (2023) Hot-injection synthesis of lead-free pseudo-alkali metal-based perovskite (TlSnX3) nanoparticles with tunable optical properties. Front. Mater. 10:1298188. doi: 10.3389/fmats.2023.1298188
Received: 21 September 2023; Accepted: 26 October 2023;
Published: 09 November 2023.
Edited by:
Gabseok Seo, Frontier Energy Solution, Republic of KoreaReviewed by:
Seunghwan Bae, Korea Institute of Industrial Technology, Republic of KoreaJae-Yup Kim, Dankook University, Republic of Korea
Ahmed Mourtada Elseman, Central Metallurgical Research and Development Institute (CMRDI), Egypt
Copyright © 2023 Kim, Koo, Ko and Jung. This is an open-access article distributed under the terms of the Creative Commons Attribution License (CC BY). The use, distribution or reproduction in other forums is permitted, provided the original author(s) and the copyright owner(s) are credited and that the original publication in this journal is cited, in accordance with accepted academic practice. No use, distribution or reproduction is permitted which does not comply with these terms.
*Correspondence: Bonkee Koo, Ym9ua2Vla29vQGhhbnlhbmcuYWMua3I=; Min Jae Ko, bWprb0BoYW55YW5nLmFjLmty; Heesuk Jung, c3Blc0BraXN0LnJlLmty