- 1InnoRenew CoE, Izola, Slovenia
- 2Faculty of Mathematics, Natural Sciences and Information Technologies, University of Primorska, Koper, Slovenia
- 3Andrej Marušič Institute, University of Primorska, Koper, Slovenia
Systems found in nature are a valuable source of inspiration for several applications. Scientists and researchers from different fields (structural engineering, robotics, medicine, and materials science) use the concepts of biomimicking, biomimetics, and bioinspiration. More recently the possibility to benefit from solutions developed by nature has become of interest for sustainable architecture. Living organisms use smart, optimised, and elegant solutions to survive, thanks to continuous selection and mutation processes. For over 460 million years plants have been evolving in a constantly changing environment and have become well-adapted to different climatic conditions. Faced with several challenges (water loss, extreme temperatures, UV radiation, etc.) plants, for example, developed tissues with barrier properties. Furthermore, due to their immobility, plants are excellent biological materials for detecting climate phenomena. While animals, being mobile, developed other creative survival strategies through a long evolutionary process. Being exposed to various environments, they not only developed multifunctional surfaces, but also movements and a broad portfolio of sensing methods that increased their survival efficiency. Comprehensive analysis and evaluation of the adaptation strategies of plants (both static strategies and dynamic mechanisms) and animals to their environment in different climate zones are indispensable for transferring concepts from biology to architecture. Consequently, specific adaptation solutions might be implemented in new materials that will be used for building envelopes erected in the same climatic zones. Integrating length scales and mixing biological, chemical, and physical concepts for tailoring the properties of materials during preparation should allow for better designing of future smart materials. The process should lead to the development of active biomaterials that perform as interfaces between outdoor conditions and internal comfort. In that they should be able to regulate humidity, temperature, CO2, and light as well as capture and filter pollutants; in addition, they should have self-assembling, self-cleaning, grafting, and self-healing properties. This contribution provides an analysis of several examples that represent the adaptation of organisms to various environments and are presented with the aim to inspire future researchers in the development of new building materials.
1 Introduction
It is estimated that approximately 10 million species live on Earth, even though only 1.8 million have been scientifically documented (Bhushan, 2009). Each living organism made an advance in its structure, shape, and function under particular environmental conditions. Consequently, nature, during its 3.8 billion years of development, has created efficient, elegant, and simple solutions, allowing full adaptation to the environment. The results of such adaptations are available to scientists, who can observe and be inspired by nature’s approach of using the minimum materials for a maximum effect. In fact, bioinspired designing, the process of developing concepts, approaches and technologies that build and control the way nature does, is not a new approach (NSF Report, 2023). It is based on observing a particular task performed by a natural system, gain inspiration for a synthetic system to affect the same task (National Research Council, 2008). It started with mimicking an animal’s motion and has now shifted to the development of new materials and devices (Ke et al., 2014). Biomimicry, defined by Cambridge dictionary as “the practice of making technological and industrial design copy natural processes,” aims to understand the fundamental principles of a biological process and adapt these concepts for bio-inspired product applications and/or to solve specific technical challenges (Lurie-Luke, 2014). In other words, biomimicry is the process of learning the mechanistic principle of a natural function and then trying to achieve that function in a synthetic material (National Research Council, 2008). By mimicking the structural, behavioural, functional, and morphological aspects of natural organisms, new materials and methods for structural design, thermal insulation, and waterproofing can be developed (Imani et al., 2018).
Biological materials are often organised hierarchically from the molecular toward nano to micro to macroscale. Consequently, the properties might vary at different levels, but all of them contribute to their multifunctionality. Biomimicry is an elegant shortcut towards the optimisation of design in many recent technological advances. Not looking at nature limits the possibility of product improvement. Biomimetics, defined as an interdisciplinary field of science that deals with the analysis and systematic transfer of biological insights into technical applications (Speck and Speck, 2021) can be used as a complementary method to improve the design process. Biomimicry is a new strategy that has become one of the major drivers behind sustainable design. The Fermenian Economic Institute predicts that by 2030, bioinspiration will generate $425 billion of U.S. GDP and $1.6 trillion of global GDP (GMIS, 2019). Similar numbers were forecasted by Ataide and Gallagher (2013), who calculated that bioinspiration could generate $1.6 trillion of total output (research, products, investments, start-up companies, etc.) while saving another $500 billion via resource and pollution mitigation by 2030.
In recent years, nature has been studied with the intention to not only develop biomimetic materials, but also mimic natural processes for the creation of new materials (Preti et al., 2019). However, as stated by Müller et al. (2018), only a small number of biological systems are currently being used in bioinspiration. Since the success of bioinspiration depends on a good match between an engineering problem and a biological solution, the narrow focus limits the innovation potential of bioinspiration as a whole. The analysis of bioinspired materials requires knowledge of both biological and engineering principles, disciplines that traditionally do not overlap (Chen et al., 2012). However, the interdisciplinary approach promoted in research and development simplifies the interaction between materials scientists, engineers, biologists, designers, and architects. It certainly expands on the fascination that human beings have with nature, by providing scientific knowledge, and the humility that is required to understand the solutions before implementing them. The convergence of scientific developments in materials characterisation and digitalisation, computational analysis of biological functions, and data science enable harnessing bioinspiration for engineering knowledge (Bhushan, 2018; Müller et al., 2018).
An analysis of bioinspired innovations can be approached from different perspectives: how things are created in nature (materials), how organisms sense their environment (sensors), how they move in their environment (biomechanics and kinetics), and how they behave and function (processes) (Lurie-Luke, 2014). This manuscript focuses on biological strategies that are or might be an inspiration for designing new materials. In addition to presenting aspects and levels of biomimicry, it provides an overview of the different strategies that organisms use for adaptation and explains how those might be useful for innovative materials’ design and/or new approaches for their manufacturing.
2 Bioinspiration aspects
Promising results of fundamental biological research might serve as inspiration for new solutions/materials for a bottom-up approach. In this case, analysis of morphology, anatomy, biomechanics, and/or physiology leads to a deeper understanding of biological structures, their shapes and functions. The concept then should be separated from the biological model to be understood by scientists working in other fields. Industrial implementation is usually done at two levels: the laboratory and then at engineering scale. The product is usually optimised for production sequence and costs, advertised and sold (Speck and Speck, 2008).
The top-down approach is driven by existing engineering problems and is related to the improvement of existing products. The process starts by defining boundary conditions, followed by screening the most promising biological solutions, which are then investigated experimentally and technically implemented. The remaining steps are similar to those in the bottom-up approach (Speck and Speck, 2008). Both processes lead to materials innovation and improvement. The bottom-up approach is usually longer (a matter of years) but leads to more ground-breaking developments. Conversely, in the top-down approach, expected results are usually less innovative but the implementation process is faster (a matter of months).
Biology offers examples of structures and processes at every scale (Whitesides, 2015). The most relevant aspects that drive bioinspiration are function, simplicity, and dissipation. Nature always generates structures that have specific functions. Certain characteristics give an advantage to organisms that enable their survival and reproduction. When the environment is not favourable, the cell/organism will select dormancy and if the surroundings are conducive, reproduction is preferred. Biological solutions are often simple. Extraordinary complexity is costly in nature, but often complex subsystems are integrated into simple, functional, and reliable solutions. As a result, nature creates elegant designs for complex mechanisms. Biological systems are dissipative, which means that biological systems function when there is a flow of free energy. During their growth, living organisms need a constant energy supply for reproduction and survival under changing conditions. Consequently, they increase in size by developing organised structures and complexity. The energy supply is covered in an efficient manner allowing functioning, reproduction, and survival (Demirel, 2014).
3 Manufacturing mode
The way that nature creates materials often differs from current manufacturing methods. Nearly 96% of natural materials are made from four elements: carbon, oxygen, hydrogen, and nitrogen. The remaining 4% is made up of seven elements, with minimal traces of a few more (Pawlyn, 2016). Nature hierarchically organises materials, mainly to achieve a given objective (for example, stiffness and fracture control) with minimal material quantity (Pawlyn, 2016). Efficient structures, such as plant stems, human bones, and feather quills, are built to provide the necessary mechanical resistance in critical places. A good example is bamboo, where nodes provide resistance to structural failure, and in trees growing in a steep terrain, the reaction wood (compression in conifers and tension in hardwood) is formed to keep the stem in a normal (vertical) position. The way nature builds is contrary to the conventional methods used by humans (mining, crushing, moulding, refining, and forming). Nature uses additive manufacturing methods (similar to 3D printing) and self-assembling methods (similar to 4D printing) that use less material while maximising the shape. It starts from molecular self-assembly that leads to macromolecular structures.
A major challenge in using biomimicry is the replication of the biological fabrication process. Most of the structures achieved by nature result from a growth process that might be challenging to reproduce. To create complex geometries, shapes and high degree of detailed novel techniques such as additive manufacturing (AM) are implemented in the building and construction sector (Ortega Del Rosario et al., 2023). Additive manufacturing is defined as a process that uses the information from a computer-aided-design (CAD) file to build three dimensional objects or to print the materials in a layer-by-layer approach. It can use three main types of materials—powder, solid, or liquid (Sidor and Meyer, 2022). A broad portfolio of fabrication techniques such as 3D printing, chemical modification, self-assembly, electrospinning, and templating allow to obtain various morphologies and material properties (Wang et al., 2020). Novel 3D printing technologies are under development to handle multiscale fabrication and to realise continuous fabrication from nanoscale to macroscale. Future developments will focus on multi-scale, high-efficiency, and low-cost fabrication of multi-materials with numerous functional properties such as reinforced mechanical, electrical, electromagnetic, optical, and chemical properties (Wang et al., 2020). Besides precision, such technologies have the potential to reduce the waste of materials, and the time associated with the manufacturing process, thus improving its sustainability (Delgado Camacho et al., 2018). Such an approach is crucial, since nature uses resources in closed-loop cycles, contrary to linear use that is often implemented by humans, which generates a large amount of waste. Long-term release of toxic emissions is very rare in nature, and, almost immediately, these emissions are biodegraded soon after they serve their purpose. Biomimetics, therefore, incorporates principles that promote sustainability much like nature does from “cradle to grave”, from raw material usage to its recyclability (Eadie and Ghosh, 2011).
4 Biomimicry levels
The three main levels of biomimicry are related to organisms, behaviour, and ecosystems that are applicable to any field (Benyus, 1997). The organism level includes simple characteristics that might be transferred from nature (e.g., its form and appearance). The behavioural stage is related to functional aspects, and the ecosystem level is related to the broader impact of the organisms that are transferred from nature, investigated, and transmitted to the ecosystem. Within each of these levels, five dimensions can be distinguished. The design may be biomimetic in terms of what it looks like (form), what it is made from (material), how it is made (construction), how it works (process), or what it can do (function) (Zari, 2007). There is an overlap between different components. The form, even if related to morphological attributes, often defines how the organism functions and vice versa. The material often defines how the structure is created and outlines its purpose. A close interconnection between all levels and dimensions allows efficient functionality and adaptation. Beynus (1997) proposed the consideration of nature as a source of inspiration in three aspects. Nature can act as a model to help solve human problems. It can act as a mentor; in this case, we can evaluate possible options and adopt the most successful solutions. Finally, nature can be considered as a measure; it can provide solutions that work in sustainable ways since it has already preserved those innovations that are ecologically sound and have proved their potential through evolution. On the other hand, evolutionary changes can be driven by extreme events (both endo and exogenous). Survival in this case requires flexibility and ingenuity, and in the long term also reproduction under new conditions. The hybridization between species allows adaptation to stressful environments and it usually takes a million years to recover species diversity and to stabilize entire ecosystems (Grant et al., 2017).
The Biomimicry Institute developed alternative taxonomy for biomimicry, which categorises the different ways that organisms and natural systems meet functional challenges into groups of related functions. The top level, “Group,” represents a broad function performed in nature, the second level is a “Sub-Group” of functions, and the third level is a specific “Function.” In total, the taxonomy features eight groups which are comprised of 30 sub-groups that contain more than 160 functions. Such a classification intends to be used as a critical thinking tool and might help to solve future innovation challenges (Ask Nature).
5 Examples of plant adaptation
Plants, due to their immobility, can serve as valuable sources of inspiration for designing materials that can be implemented in building structures. During their 460 million years of evolution, plants have adapted extremely well to various climatic conditions such as droughts and floods, extreme temperatures, and solar radiation. As a result of exposure to certain conditions, plants developed biological adaptations enabling them to survive and efficiently reproduce in these specific climates (López et al., 2017). Plants also possess phenotypical and morphological plasticity that developed in response to the variability in their environment (Mazzolai et al., 2014). Such solutions can serve as inspiration for designing new functional materials as well as effective façade systems (Sandak et al., 2019). Brief descriptions of ecosystems specific to different climatic zones that inspire transferring biological adaptation to building façade systems are presented in Figure 1 and are discussed below.
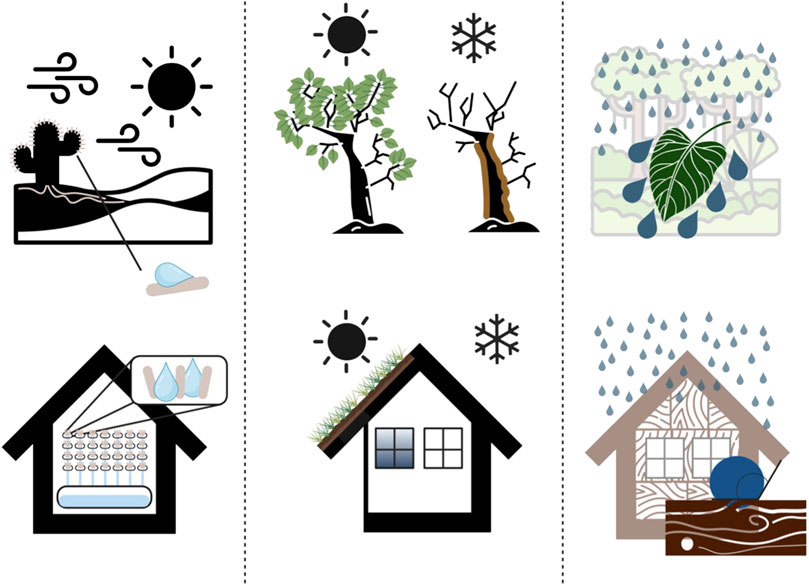
FIGURE 1. Examples of ecosystems (desert, temperate forest, and rain forest) typical for various climatic zones. Every ecosystem offers biological adaptations that can be transferred to building façade systems. Created wihh BioRender.
5.1 Desert
The desert climate is characterised by direct Sun, extreme daily temperatures, strong wind, and drought conditions. Plants adapted to this environment by developing systems that collected and stored water, thereby reducing water loss: thick, small leaves that often possess hairs and pins, covered by a thick layer of wax for reducing transpiration; long rooting systems facilitated water uptake from deeper parts of the ground (Bargel et al., 2006). Such adaptations might be useful especially while designing building materials for the arid climate. UV protection and reduction of evaporation might be achieved by efficient shading and reflective systems implemented in materials and entire building façades. The problem with water accumulation and supply might be solved by designing water-capturing and filtering systems integrated into building façades (Sandak et al., 2019).
5.2 Prairie
Less severe, but also demanding, is the prairie (also called grassland, savannah, steppe) where drought is very common since rainfall is uncertain. Seasonal temperatures are extreme with hot summers and cold winters in addition to strong winds, all requiring strategies for survival. The development of a specific shape and narrow leaves enabled plants to lower their transpiration. Thick bark and efficient reproduction systems (seed dispersing, rhizome) were developed as protection against animals. An efficient water storage system was developed due to uncertain rainfall. Frequent fires meant that plants created quick regeneration processes. All these solutions might inspire the development of materials capable of self-healing and regeneration. Seed realizing systems, sensitive to high temperatures, might serve as an inspiration for dynamic façade elements capable of opening and closing, assuring efficient ventilation. Studying the shape of plants might help in designing structures that are resistant to strong wind.
5.3 Temperate forests
Temperate climates have warm and hot summers and cool winters (temperatures below 0°C). Temperate forests are characterised by deciduous trees, which lose their leaves during the winter. Such forests usually do not suffer due to limited water availability, but plants face four seasons and, consequently, temperature changes. Even if plants developed efficient photosynthesis, for some (e.g., angiosperms) the process is possible only during the vegetative season. During winter, trees enter dormancy, saving energy to survive the winter period. The thick bark serves as high thermal insulation. Lower temperatures and shorter days initiate an abscission process allowing leaves to fall without an open wound. This phenomenon might be used in materials that are capable of self-maintenance and self-healing. Certain triggers (e.g., temperature, UV) might stimulate materials to produce an additional layer and remove the old one.
At the end of the vegetative season, chlorophyll production stops, revealing the red and yellow pigments that were masked by green. This phenomenon (e.g., observed in beech leaves) might be used for communicating and signalling actions when, again, certain triggers deactivate/activate a pigmentation system. Plants in this climate are usually sensitive to different stimuli, often exhibiting animal-like movement behaviour (Simons, 1992). Thigmonasty is a nondirectional (nastic) movement of plants and fungi in response to touch (or vibration), as seen in the mimosa (Jaffe et al., 2002; Almeida and Brand, 2017). Nyctinasty (circadian rhythm-based nastic movement) occurs in response to temperature and light intensity (such as in Oxalis). Other examples of self-movement might be Heliantus (exhibiting phototropism) or pinecones (opening due to moisture changes). The fundamental mechanisms of these fast moving plants might be an inspiration for several engineering applications, such as artificial muscles, multi-stable structures, and bioinspired robots (Mazzolai et al., 2014; Guo et al., 2015). At the macro scale, such movements might be implemented in developing façade shading, dynamic energy storage systems, or inspire the architecture of an entire building (e.g., Villa Girasole, designed by Angelo Invernizzi in 1935, that moves according to sunlight).
5.4 Tundra
Temperate forests are substituted by tundra growing in the polar zone, where summers are cold and short and winters are severe and long; rainfall is low, water uptake is often limited by permafrost, and solar radiation varies. Plants developed wax and hair as protection against freezing. Moreover, specific colours, capable of efficiently absorbing solar radiation developed in addition to shiny surfaces that can modulate light reflection. These solutions can be implemented for modulating light transmission in building façades, designing anti-freezing surfaces, for developing dynamic shading, and self-actuating energy storage systems (Sandak et al., 2020).
5.5 Rain forest
In tropical climates, plants do not suffer due to lack of water. Conversely, they are exposed to heavy rains, uneven solar radiation, high temperatures, and high relative humidity. Tropical plant morphology allows water runoff (e.g., drip tip of leaf shape) or water storage. A thick wax layer assures protection and hydrophobicity. Aerial roots allow CO2 uptake. Such mechanisms can be used for the development of highly hydrophobic and self-cleaning surfaces as well as filtering and phytoremediation systems dealing with the accumulation and cleaning of rainwater. Another example is the open mechanism of the flower, Bird-of-Paradise (Strelitzia reginae), that inspired new façade-shading systems. In this case, an external mechanical force (by pollinating birds) initiates a complex deformation of multiple structural members (Mazzolai et al., 2014). This mechanism has been implemented through a glass fibre-reinforced composite, allowing large elastic deformation, driven by variations of temperature that can passively control the façade-shading systems (Lienhard et al., 2011).
5.6 Water ecosystem
Plants growing in an aquatic environment have unlimited access to water. Water temperatures are relatively stable and plants immersed in water are not directly exposed to Sun irradiation. However, water currents and sporadic floods might be an issue. Features such as flexible stems help keep the plant relatively stable while floating seeds allow for efficient reproduction. Surfaces are hydrophobic (e.g., lotus leaf) and a thin cuticula layer allows high CO2 uptake. Such developments are best practice examples for designing materials where the stiffness/flexibility levels can be adjusted, and with hydrophobic and self-cleaning surfaces (Barthlott et al., 2017).
6 Biomimicry for materials development
According to Lurie-Luke (2014), the largest area of biomimicry research is related to materials, accounting for around 50% of published research. The research is mainly related to smart materials reacting in response to external stimuli, surface modifications, and novel shapes and structural arrangement of materials. Nature organises materials into sophisticated hierarchical structures providing exceptional mechanical, thermal, or optical properties (Yaragi and Kisailus, 2018). The hierarchical structure assures outstanding properties and achieve higher performance per unit mass. An example is spider silk, which possesses tensile strength comparable to steel, and considering its density, is more than four times stronger per unit mass. Similarly, the shell nacre possesses a high Young modulus, tensile strength, and fracture toughness even though it is composed mostly of brittle materials (Wang et al., 2020b). The relationships between the morphological features and physicochemical properties of surfaces of living organisms inspired researchers to design multi-function surfaces. Most of the outstanding properties, such as superhydrophobicity, directional wetting, anti-reflexivity, self-cleaning, and controllable attachment are due to specific macro- and nanostructures (Irzmańska et al., 2022). Figure 2 presents a portfolio of properties exhibited by leaves due to their structure. However, simple duplication of natural materials and structures might not be sufficient to benefit from its properties. Therefore, an understanding of the design principles and physical/chemical mechanisms that determine the optimised structural organisation in biological systems and its relationship to function is essential (Yang et al., 2018).
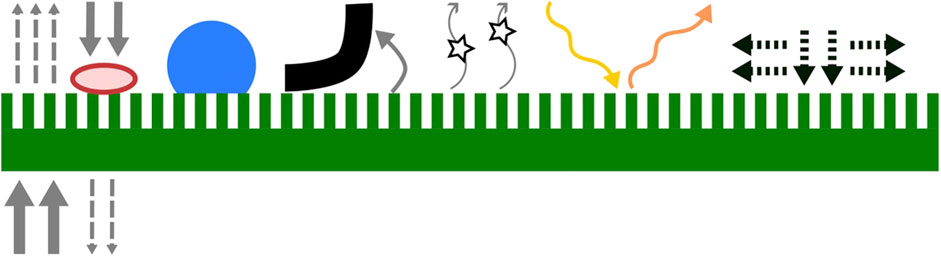
FIGURE 2. Examples of leaf surface functions resulting from the microstructure. From left: transport barrier, wettability, anti-adhesion, signaling, UV-reflection, mechanical resistance (based on Bargel et al., 2006).
6.1 Smart materials
Smart materials have dynamic parameters capable of responding to changes within their surrounding environment (Lurie-Luke, 2014). Such changes can be triggered by chemical or physical stimuli. The typical chemical trigger is a change in pH. An example might be a new type of adhesive inspired by mussels. At low pH, the material is liquid; at high pH, it transforms into a gel. The changes are reversible; this technology might be useful for underwater or medical adhesives (Holten-Anderson et al., 2011).
The range of physical stimuli is wide: light, temperature, pressure, vibration, sound, gravity, water content, volatile chemicals. A well-known example is the pinecone, which opens or closes in response to changes in humidity. The motion of pinecone scales is caused by morphological changes. Different orientations of sclereids and fibres in pinecone scales affect the movement of the scales. Their tissues expand anisotropically in a direction perpendicular to the direction of the fibrils when the scales absorb water (Song and Lee, 2017). This mechanism was an inspiration for several researchers using this phenomenon for smart biomimetic actuators in architecture (Mazzucchelli and Doniacova, 2017; Poppinga et al., 2018).
The bioinspired sensors exhibiting visible colour changes inspired by chameleons were developed after an in-depth investigation of their ability to adjust their colours between a concealed (camouflaged) state and a highly visible (excited) state. The sensor exhibits a colour shift from red to blue when stretching, and a colour change from red to green when compressing (Wang et al., 2020b).
An interesting group of smart materials are shape-memory materials owing to their ability to generate programmable movements (bending, twisting, or contraction) when subjected to an external stimulus, such as an environmental change (Lendlein and Gould, 2019). The shape-memory actuation can be one-way or reversible (bidirectional). Such effects can be obtained at the macroscale and at the micro- and nanoscale. Advanced manufacturing has increased the morphological diversity of shape-memory devices, and materials with programable shape-memory capabilities have been manufactured in ceramics, alloys, and soft materials (Lendlein and Gould, 2019). However, to achieve their widespread application, further development in terms of their performance and multi-sensitivity, as well as a decrease in manufacturing costs, is necessary.
6.2 Surface modifications
Materials’ surface modifications include a wide range of modifications related to surface energy, appearance, adhesion, and unique properties such as self-regeneration and self-healing. Modifications can be related to surface topography, patterning, activation, or functionalisation.
6.2.1 Modification of surface energy
The wettability of a surface depends on the surface tension of the liquid on it. Water has a very high surface tension (72.8 mN·m−1); therefore, it tends to wet only surfaces bearing highly polar groups. Conversely, apolar liquids of lower surface tension produce drops flatter than those of water. The low-energy surfaces (hydrophobic or oleophobic) are difficult to wet with water or apolar liquids, respectively. The wettability is usually determined by measuring the contact angle (CA) of a water droplet on a solid surface. In the case of a solid surface, when a CA of water drop is larger than 150°, it is called superhydrophobic (Guo et al., 2011). Various behaviour of water on the plant surfaces is presented on Figure 3.
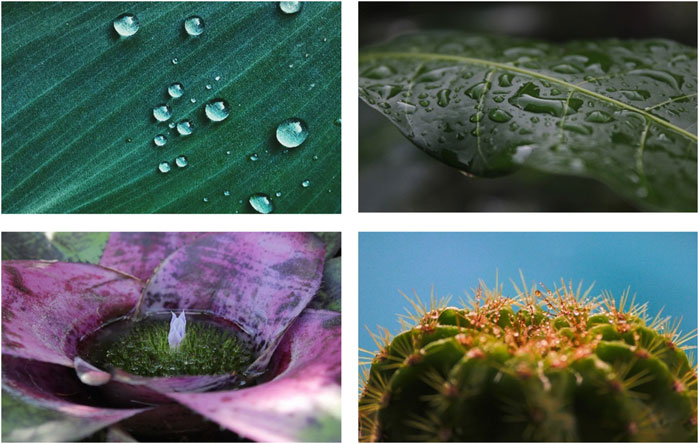
FIGURE 3. Different behaviour of water on plant surfaces. Source: Public domain images—pixabay, shared under creative commons CC0 dedication.
Controlling wettability is important from a fundamental and practical perspective, as superhydrophobic surfaces exhibit self-cleaning properties (Guo et al., 2011). The typical examples are lotus, rice, and taro leaf, which exhibit a microscopic roughness on different length scales together with the presence of hydrophobic epicuticular wax crystalloids. The lotus leaf is covered with very small bumps that are 5 microns in size, which is about a 10th of the size of human hair. The entire surface is additionally covered by 3D epicuticular wax, which self assembles in the form of nanotubes (with the dimension of hundred nanometres) (Yamamoto et al., 2015). The hierarchical structure of the lotus leaf was the inspiration for the development of superhydrophobic surfaces by nanoscale casting (Si and Guo, 2015) or UV-nanoimprint lithography (Lee and Kwon, 2007). Other methods for obtaining superhydrophobic surfaces include wet chemical reactions, electrochemical deposition, self-assembly, layer-by-layer, and chemical vapour deposition (Guo et al., 2011). Superhydrophobic surfaces can be used to obtain anti-icing, super oil-repellent, anti-fogging, self-cleaning, or electrowetting effects, among others.
Hydrophilicity, or water attraction, is the opposite phenomenon of hydrophobicity. Superhydrophilic surfaces are divided into those that are permanently wet, can absorb water, or where water easily spreads. The first group is comprised of plants submerged in water; they possess smooth cell surfaces and have no three-dimensional wax or hair. The second group has a porous surface and multicellular hair. Peat moss is a typical example of such a plant which has a water uptake that is up to 20 times its dry weight (Koch and Barthlott, 2009). An alternative to pores might be thorns (spines), allowing for collection of water by desert plants, dead cells of epiphytic orchids building aerial roots, or organs responsible for guttation (hydathodes). The third category of plant surface allows water to quickly spread to a flat film. This can be obtained by different cell types that form channel-like structures, leading to slippery surfaces. The presence of water defends against drought-induced stress, but also reduces gas exchange and leads to the formation of biofilms on the surface. The superhydrophilic phenomena can be implemented when drying out and anti-fogging of the surface is desired. It can be also implemented when easy cleaning, anti-condensation, and anti-fouling are required. Future developments related to multi-functionality and the incorporation of additional properties into self-cleaning structures might increase their utility and application ranges (Xu et al., 2016).
6.2.2 Modification of surface appearance
Structural colouring is a visible consequence of the particular pattern of a reflecting surface with regular structures at submicrometre-length scales (Dumanli and Savin, 2016). This phenomenon is a result of diffraction, interference, or scattering of light and often appears as bright, shiny, metallic, or iridescent (Figure 4). Contrary to pigment colours, structural colours do not fade as they are not sensitive to chemical and environmental alterations. Understanding the material structure at the nanometre level, together with the development of new fabrication processes (patterning, etching, moulding, deposition), allows for highly reproducible and high throughput microfabrication. The structural colour can be generated by diffraction gratings (e.g., butterfly scales), film interference (e.g., insects wings), photonic crystals (e.g., marine animals, chameleons), and scattering (e.g., bird feathers). However, in nature, very often structural colour is assured by merging different physical phenomena. Morpho butterfly wings combine multilayer interference, diffraction, scattering, and pigment-induced absorption to produce its singular, angle-independent, brilliant blue colour (Dumanli and Savin, 2016). Moreover, living organisms very often implement more than one functionality in their body parts. Butterfly wings, besides being an example of structural colour, present self-cleaning, directional adhesion, superhydrophobicity, chemical sensing capability, and fluorescence emission functions (Niu et al., 2015).
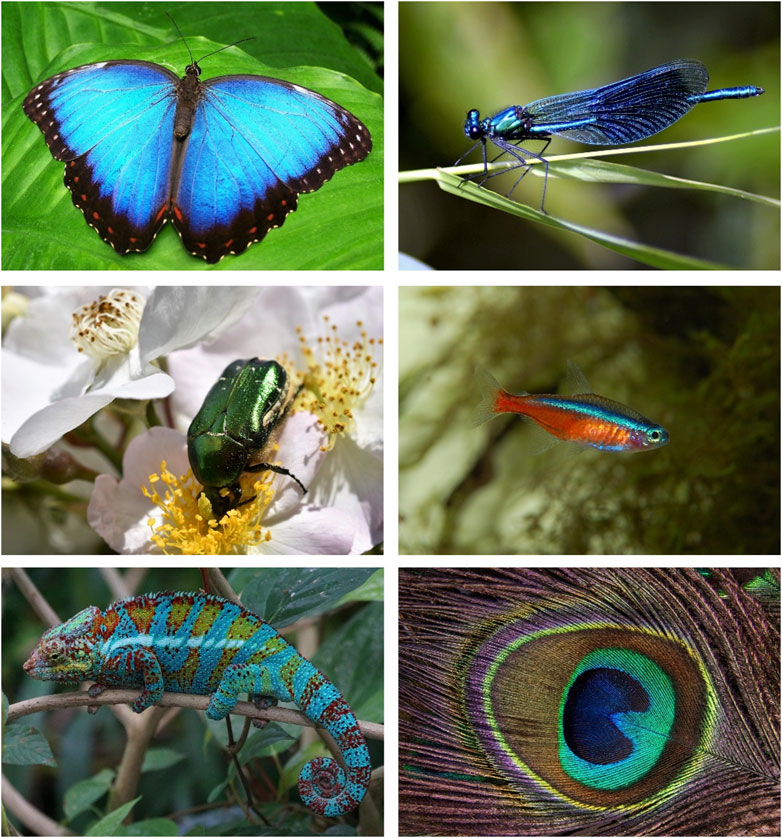
FIGURE 4. The structural colour observed in various animals. Source: public domain images—pixabay, shared under creative commons CC0 dedication.
Structural colours can be produced by previously described strategies: top-down and bottom-up. Top-down includes various lithographic techniques that might use templates (photolithography, holographic lithography, nanoimprinting lithography, and soft lithography), or not (electron beam lithography, focused ion beam lithography, and scanning probe lithography), layer-by-layer deposition techniques (liquid or vapour based), interactive size reduction, or a combination of the above (Dumanli and Savin, 2016). The bottom-up strategies include self-assembly (colloidal, anisotropic particle, block copolymer) and biomimetic templates (direct or inverse).
Structural colours, in addition to being more durable, might be also more sustainable since they are not produced through chemical processes. The most typical building blocks used for development of structural colours are block-copolymers, inorganic platelets, and colloidal particles (Goerlitzer, et al., 2018). Structural colour can be also manufactured from synthetic melanin nanoparticles as an alternative to polystyrene colloidal particles. This provides a broader application range and assures biocompatibility (Xiao et al., 2015). The interesting area for structural colour is their response to environmental stimuli. This phenomenon has great potential for application in advanced materials (paints, textiles), sensors (MEM-based technology), actuators, cosmetics, security, art, or even food products (Saito, 2011).
6.2.3 Modification of surface adhesion
Biological evolution has led to the optimisation of different attachment systems. The dynamic attachment ability is called reversible adhesion or smart adhesion (Bhushan, 2009). There are two types of nature-inspired adhesion processes, differing in physical phenomena and allowing certain actions. The dry adhesion force comes mainly from van der Waals force or hook-loop adhesion, whereas the wet adhesion force comes mainly from surface tension or capillary force (Li et al., 2011).
Under dry conditions, reversible adhesion can be obtained through external stimuli such as pH, light, temperature, humidity, and magnetic force or modulation of bulk material properties (Zhao et al., 2017). A gecko is the largest animal capable of generating dry adhesion due to its complex hierarchical structure of the skin, which is comprised of lamellae, setae, branches, and spatula. Such a multi-level hierarchical structure allows safe movement on surfaces with different roughness. The gecko-inspired adhesive can be used for reusable, dry, clean, and reversible adhesive materials (Wang et al., 2021). The hook-loop adhesion mechanism was an inspiration for development of Velcro adhesive (Postiglione, 1993). The hook-loop adhesion can be divided into two stages. In the loop hooked up stage, hooks penetrate the fibrous cluster to hook up the fibrous loops. In the pull-out stage, hooks are pulled out accompanying the loops that gradually straighten and slide from the hooks (Ouyang et al., 2022). Moreover, the hook-loop adhesion has been extended and designed so that the gripper can switch in the gecko-like and Velcro-like state for griping the smooth materials and fabrics (Zhang et al., 2019).
Even more challenging to design is adhesion technology that performs well in wet environments. Wet adhesion refers to the ability to achieve or maintain adhesion on wet surfaces or in wet environments (Cai et al., 2021; Cui and Liu, 2021; Wei et al., 2023). Insects with wet adhesion systems have soft, smooth pads that can adjust to the shape of a surface and stick to different kinds of smooth and rough surfaces (Li et al., 2011). Amphibians are another group of animals that can attach to wet surfaces. This is due to their specific toe morphology that facilitates squeezing while avoiding trapping the liquid. In this case, the adhesion force is mainly attributed to the combined effect of capillary and Steffen adhesion (Xie et al., 2018).
Several aquatic organisms have evolved to achieve rapid and robust attachment to various materials underwater through synthesising, secreting, and curing biological adhesives (Gan et al., 2022). Naturally produced adhesives are common in many biological systems: bacteria, spiders, marine tubeworms, sea cucumbers, barnacles, mussels, and diatoms (Silverman and Roberto, 2007; Guan et al., 2021; Sun et al., 2023). Mussels are capable of strong underwater adhesion to a variety of surfaces through the synergistic effect of lysine, which displaces the water molecules at the interface, and 3,4-dihydroxyphenylalanine, which interacts with the surface (Shin et al., 2020). The production of adhesive inspired by mussel adhesive proteins will allow for the implementation of an ecologically safe formulation as an alternative to petroleum-based ones, in demanding underwater environments (Siebert and Wilker, 2019). Examples of organisms utilising dry, wet and underwater adhesion are presented in Figure 5.
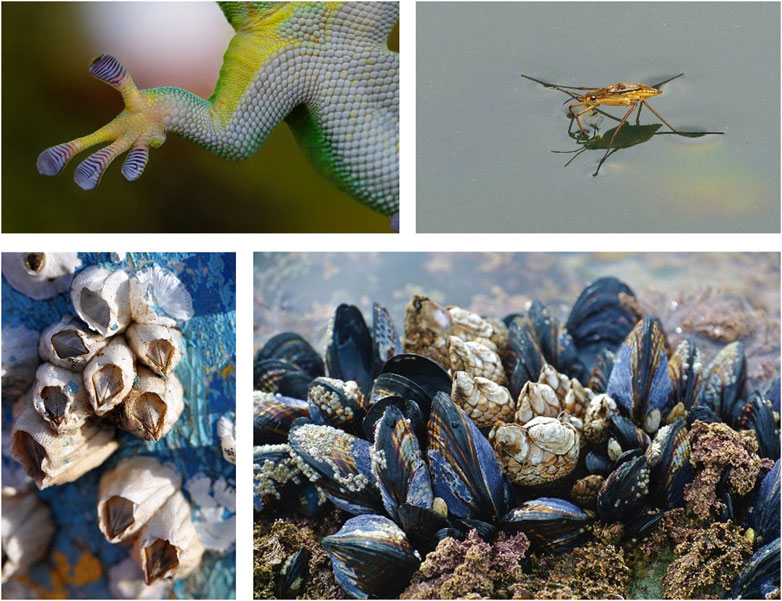
FIGURE 5. Examples of dry and wet adhesion (top) and organisms capable of attaching underwater: barnacles and mussels (bottom). Source: Public domain images—pixabay, shared under creative commons CC0 dedication.
6.2.4 Self-repairing
Most surfaces are exposed to damage, which reduces their functionality and requires repair or replacement. Plants and animals approach healing in similar ways but with unique pathways, such as damage containment in plants or clotting in animals (Cremaldi and Bhushan, 2018). Biological tissues are capable of self-repair by forming intermediate tissue. The fracture releases chemical signals that initiate a series of responses leading to regeneration and performance recovery. The repairing mechanisms are used in polymer composites and can be achieved twofold: by molecular diffusion and thermally reversible solid-state reactions or by the release of healing agents stored in the material (Bhushan, 2009). Speck and Speck, (2019) divide self-repair mechanisms in higher animals and plants into an initial self-sealing phase and a subsequent self-healing phase. Self-sealing is limited to the restoration of functionality, but the mechanical properties are not completely restored. The self-healing phase leads to structural reparation and restoration of mechanical properties. Self-sealing can occur within a few minutes (by filling wounds with latex, resin, mucilage), or hours (by overlapping of wound sites, close contact of wound surfaces, rolling-in of wound edges). Self-healing in certain plants is related to the formation of a ligno-suberized boundary layer and might take several days to weeks (Speck and Speck, 2019). An example of a self-repairing mechanism is clearly visible on succulent plants (e.g., Delosperma cooperi). The sealing phase takes approximately 60 min during which time the entire leaf bends until the edges of the wound meet. Subsequently the wound heals over days or weeks. Clotting is a mechanism used to prevent material from flowing out of animals (both vertebrates and invertebrates). The mechanism relies on material (e.g., cells, proteins) aggregation at the wound site that plugs the wound and stops the material from flowing out of the body while preventing microorganisms from entering the body (Cremaldi and Bhushan, 2018).
The self-repairing phenomena observed in biology are a challenge for building materials since they are not alive in terms of living organisms. However, the development of bio-concrete (where limestone-producing bacteria are activated when a crack occurs) and bio-coatings (where living Aureobasidium pullulans moulds re-grow on a wooden surface after damage) provide the first proof of concept solutions demonstrating novel capacities of materials (Sailer et al., 2010; Naidu et al., 2018; Poohphajai et al., 2021). The commercial potential for self-healing polymers is significant. The estimated costs associated with corrosion reach up to $500 billion worldwide every year (Lee, 2014). Self-healing paint, in which small scratches repair themselves, will have an obvious advantage for many industrial products (Lenau et al., 2018).
Another interesting mechanism that evolved in plants due to their inability to move is abscission, a process where a weakening part of the stem causes it to break. A protective layer is created first to prevent the entry of harmful microbes, and then the stem or branch detaches from the plant (Cremaldi and Bhushan, 2018). This common phenomenon observed when trees lose their leaves, might inspire the design of materials that, after a certain period, eliminate unneeded parts or layers (e.g., self-maintaining facades).
6.2.5 Anti-fouling (AF)
Biofouling is the accumulation of microorganisms, plants, algae, or small animals on wetted surfaces. Biofilm-forming microorganisms often produce slime that protects them against environmental fluctuations. Slime, known as exopolymeric substances (EPS), contain proteins, carbohydrates, and uronic acids, among others (Chapman et al., 2014). The EPS matrix regulates the ability of the biofilm to adhere to surfaces, provides mechanical stability to the three-dimensional biofilm structure, and determines the ability of the biofilm to adsorb gasses, solutes, and foreign cells (Sandak, 2023). Biofilm formation is influenced by numerous factors, including matrix production, genetic regulation, cell differentiation, and metabolic changes. Microorganisms can alter their behaviour or the density of the population. This process is commonly referred to as quorum sensing (QS). Interrupting the quorum-sensing pathway poses a new opportunity for developing an effective AF material (Chapman et al., 2014).
The biofouling process is very common in underwater construction, ships, and desalination plants, and in paper manufacturing, food processing, medical devices, and membranes. Effective antifouling protection will save the global maritime industry an estimated 150 billion US dollars per year (Yang et al. 2014) Moreover, fouling causes the relocation of marine organisms that may, due to global warming, invade non-native environments (Holland et al., 2021).
Sharks are model animals for biofilm prevention as they are continuously exposed to bacteria, algae, and other marine organisms. Their dermal denticles are grooved parallel to the local flow of water. Their rough surface reduces the amount of surface area for bacterial organisms to attach and creates an unstable surface as it is responding constantly to variations in internal and external pressure (Lee, 2014). Dermal denticles accelerate water flow and allow air pockets to form between scales that repel biofouling. The micro-texture in this case might be achieved by laser ablation, photolithography, nanoparticles, and casting. The first micropatterned textured design to prevent bacteria colonisation was Sharklet AFTM designed by Brennan (Reddy et al., 2011). Chapman et al. (2014) demonstrated that specific material topography enhanced by chemistry can reduce biofouling by up to 40%. In the optimal case, chemical substances should be effective but also environmentally friendly. Promising results have been obtained by merging surface topography with furanone compounds from brown algae (de Araujo et al., 2010), or by applying substances that ensure strong surface hydration in an aqueous environment, such as poly (ethylene glycol) (PEG) containing polymers and zwitterionic polymers (Del Grosso et al., 2020).
6.3 Structural materials
Several cellular materials, such as bone, wood, bamboo, cuttlebone, or honeycomb are lightweight with favourable strength. Natural materials show an optimal strength-toughness balance, this being a key requirement for structural materials. Bone tissue combines inorganic (hydroxyapatite) and organic (protein) constituents as building blocks to build up a complex hierarchical structure. Such a combination enables achieving remarkable mechanical properties and a large amplification in toughness that is not observed in synthetic counterparts (Libonati and Vergani, 2016). Bone is one of the biomimetic model materials based on which various reinforced materials such as fibre-reinforced composites are designed.
Wood is an anisotropic material and consists of three main chemical components which are cellulose, hemicellulose, and lignin. The performance, lightweight, and strength of wood used in structural applications are influenced by its physical properties, such as density, mechanical resistance, sorption and permeability, dimensional stability, thermal conductivity, acoustic and electric properties, natural durability and chemical resistance. Burget et al. (2015) proposed three approaches aimed at developing novel and advanced bioinspired and bio-based functional materials based on wood. The functionalisation of wood might utilise the hierarchical structure of wood at different length scales. It might retain its integrity or be used as a template to engineer wood-derived inorganic, non-metallic materials. In the third approach the wood cellulose nanofibres (CNF) allows for designing structures with different porosities and new combinations of anisotropic properties.
The structure of lightweight panels is often inspired by the honeycomb. Gross et al. (2020) investigated the influence of the radius at the corner of each cell, the coping at the top of the cell walls, and the interface between cell arrays on the out-of-plane compression and bending. The combination of modelling and experimental approach allowed to improve the design and enhance bending performance.
A systematic investigation of how the bamboo’s hierarchical structural features (e.g., gradient fibre distribution, periodic nodes, and others) contribute to its compression capacity was recently performed (Cui et al., 2020). Identification of the morphological features that contribute toward the mechanics of bamboo could provide a guide for engineers to predict the material mechanics according to its structure, design bamboo-inspired composite materials, and construct high-performance architectures (Cui et al., 2020).
6.4 Nature design
The principle governing architecture in nature is less material, more design (Pawlyn, 2016). Consequently, maximum performance can be obtained with minimum materials. Natural structures consist of a small number of mostly light elements and only a few polymeric substance groups (proteins, polysaccharides, lipids, nucleic acids) (Dunlop and Fratzl, 2010). Individual cells form tissues that are combined to create “organs” with different functions. Majority of the natural structures are not isotropic. They consist of fibres such as cellulose or collagen with direction-dependent properties. Moreover, the basic building components serve not only as mechanical support but play an active role in the transport of substances and in recognising molecular signals (Knippers and Speck, 2012). Designs in nature always provide functional optimisation which may inspire the design of new material architectures with applications across a range of industries. For example, the skeletons of birds are lightweight, many of their bones are pneumatised and feature crisscrossing internal structures that increase their strength (Lurie-Luke, 2014). Understanding the evolution of bone shapes and material properties contributed to the development of new lightweight structures for airplanes (Dumont, 2010). Another example is bamboo; to maintain strength over their length (some species can reach 40 m), bamboo stems have regular nodes that prevent buckling (Youssefian and Rahbar, 2015). At the macroscale, trees demonstrate optimised junction shapes that avoid stress concentration. In places potentially exposed to stress, the material is built up until it can evenly distribute force (Pawlyn, 2016). A structure built from wood is the best possible choice for building high columns that do not buckle. Even though wood is a polymeric material, its mechanical performance for this task is better than typical polymeric materials used in engineering (Fratzl and Weinkamer, 2007).
Living organisms are also able to build hybrid organic-inorganic materials that serve different purposes (Mann, 2001). Approximately 80% of biogenic minerals are crystalline and only 20% are amorphous (Lowenstam and Weiner, 1989). The mineral component (typically calcium carbonate, calcium phosphate, and amorphous silica with a concentration on the order of 95%) provides strength, and the organic components (typically keratin, collagen, and chitin in the range of 1%–5%) provide ductility (Bhushan, 2009). Biominerals, due to their specific composition, structure, size, and morphology, possess unique properties that are often superior to engineered ceramics. Natural biomineralisation is being used as inspiration for the development of implants, wear-resistant, and self-sharpening structures (Bushan, 2009).
Biological structures and materials represent systems that actively respond to the biophysical stimuli of their environment. They can adapt their architecture to improve their functionality during growth, re-establish it according to external constraints thus adapting to changes in the environment. Such strategies are based on adding new material, possibly with specific mechanical properties, or maintaining the existing material using repair mechanisms (Fratzl and Weinkamer, 2007).
6.5 Engineered Living Materials
Characteristics of conventional building materials are limited compared to living organisms. Properties of materials created by nature include programmability, multifunctionality, or “self” properties such as self-growth, self-adaptivity, self-assembly, or self-healing. The biomimetic approach allows for the derivation of optimal designs, benefitting from improvements made during the evolution of living organisms and the efficient use of natural resources in a more sustainable and environmentally friendly manner. However, bioinspired or biomimetic materials despite their advantages, also have limitations compared to materials in nature, in the degree to which they are environmentally friendly and energy-efficient, and in the range of their properties. These limitations are caused by the fact that all these materials are not living. Engineered Living Materials (ELM) are the most relevant contemporary revolution in materials science and engineering and aim to outperform current examples of “smart”, active, or multifunctional materials, enabling countless industrial and societal applications. ELMs are defined as materials composed, either entirely or partially, of living cells. Various microorganisms might be incorporated as living and bioactive components in the form of a matrix or be a part of the living scaffold (Rodrigo-Navarro et al., 2021). ELMs are classified into two categories: biological ELMs (bio-ELMs), which are solely made of cells and hybrid living materials (HLMs), consisting of abiotic scaffolds and living cells (Srubar and Wil, 2021). ELMs represent a fundamental change in materials’ production and performance since they can replicate the function and capabilities of living organisms. Their development is expected to push the boundaries and frontiers of synthetic biology, materials engineering, nanotechnology, biomaterials, and artificial intelligence. They also possess a broad portfolio of functionalities relevant to architecture and have enormous potential for the building sector (Sandak, 2023) (Figure 6). ELMs can be implemented as basic building blocks (Heveran et al., 2020), insulation (McBee et al., 2022), active elements (Liu and Xu, 2020; Rivera-Tarazona et al., 2020), or protective surfaces (Poohphajai et al., 2021). ELMs might be considered as an interesting alternative for the future of resilient architecture since they outperform conventional human-made materials with decreased costs and environmental impact (Sandak, 2023). However, in addition to overcoming recent constraints related to the design and upscaling of the manufacturing process ethical, legal, and social aspects are as important for their successful implementation (Srubar, 2022).
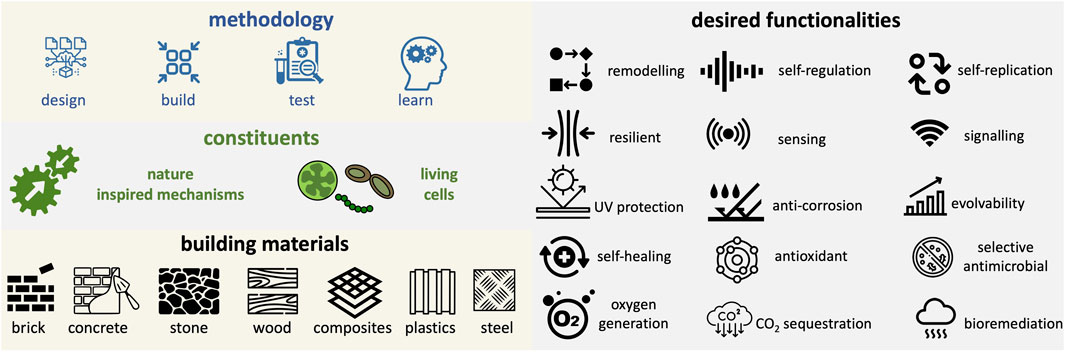
FIGURE 6. ELMs possessing unique functionalities, compared to traditional building materials represent a fundamental change in the production and performance of materials. The Design–Build–Test–Learn (DBTL) approach is often used to manage computational and experimental workflows. The concept of ELMs benefits from the synergistic strength of living cells (often multi-species consortia) and bioinspired concepts for materials design. This shifts the traditional materials design and allows for the development of living materials that are capable of interacting, adapting, and responding to environmental changes.
7 Conclusion
The convergence of solutions developed by animals, plants, microorganisms, and potentially viruses indicates that nature has developed the most optimal solutions to solve specific problems. Such solutions should be the first candidates for biomimetic implementation within the engineering context. Like many great ideas, biomimicry started from a simple imitation of natural organisms. With the progress in science and the development of modern and powerful analytical techniques like scanning tunnelling microscopy (STM), atomic force microscopy (AFM), and selective plane illumination microscopy (SPIM) the functions created by nature become an inspiration for the discovery of new materials and alternative ways of production. This is especially important since rapid industrialisation has resulted in environmental pollution and a shortage of natural resources.
Biomimetic materials’ science has great potential for finding new solutions to engineering problems. To successfully implement the biological system and understand the structure–function relation of the biological material, its physical and biological constraints should be deeply investigated. Bioinspiration, biomimicry, and biomimetics can inspire not only materials development but also help understand the way nature is organised from a broader environmental perspective. New bioinspired materials might help to mitigate the extent of climate change by preventing carbon dioxide emissions or sequestering carbon. They also might help us to adapt to the effects of climate change by reducing harm and damage from extreme events such as flooding, rise in sea levels and extreme heat events (Mabon, 2020). Nature provides an inspiration for designing and creating products more efficiently, assuring their biodegradability and compatibility with nature rather than being destructive.
Recently bioinspired design has moved a step further. Living building blocks and biosynthetic pathways of natural biopolymers have been captured for making biobased materials with unique renewable, recyclable, and biodegradable potential. The convergence of engineering, biology, and materials science allows the integration of unicellular and multicellular organisms in the next-generation engineered systems. ELMs outperform current examples of “smart materials” and are the future of sustainable and resilient architecture. Biomimetics, therefore, might contribute to the development of new materials, processes, and technologies that will lead to significant scientific, societal, and economic impacts in the near future.
Author contributions
AS: Conceptualization, Funding acquisition, Resources, Supervision, Writing–original draft, Writing–review and editing. KB: Conceptualization, Writing–review and editing.
Funding
The authors declare financial support was received for the research, authorship, and/or publication of this article. The author gratefully acknowledges the European Commission for funding the InnoRenew project [Grant Agreement # 739574] under the Horizon 2020 Widespread-Teaming program and the Republic of Slovenia (investment funding of the Republic of Slovenia and the European Regional Development Fund). Part of this work was conducted during the WoodLCC project, which is supported under the umbrella of ERA-NET Cofund ForestValue by the Ministry of Education, Science and Sport (MIZS)—Slovenia. Additional funding by the European Union was provided under the ERC Grant Number #101044468 ARCHI-SKIN.
Conflict of interest
The authors declare that the research was conducted in the absence of any commercial or financial relationships that could be construed as a potential conflict of interest.
Publisher’s note
All claims expressed in this article are solely those of the authors and do not necessarily represent those of their affiliated organizations, or those of the publisher, the editors and the reviewers. Any product that may be evaluated in this article, or claim that may be made by its manufacturer, is not guaranteed or endorsed by the publisher.
Author disclaimer
Views and opinions expressed are, however, those of the author(s) only and do not necessarily reflect those of the European Union or the European Research Council Executive Agency. Neither the European Union nor the granting authority can be held responsible for them.
References
Almeida, M. C., and Brand, A. C. (2017). Thigmo responses: the fungal sense of touch. Microbiol. Spectr. 5, 2. doi:10.1128/microbiolspec.FUNK-0040-2016
Ask Nature. Browse the biomimicry taxonomy. Ask Nature, The Biomimicry Institute, Available at: http://toolbox.biomimicry.org/wp-content/uploads/2015/01/AN_Biomimicry_Taxonomy.pdf (Accessed October 4, 2023).
Ataide, R. M., and Gallagher, C. L. (2013). Bioinspiration: an economic progress report. USA: Fermanian Business and Economic Institute. Technical Report.
Bargel, H., Koch, K., Cerman, Z., and Neinhuis, C. (2006). Structure–function relationships of the plant cuticle and cuticular waxes—a smart material? Funct. Plant Biol. 33, 893. doi:10.1071/FP06139
Barthlott, W., Mail, M., Bhushan, B., and Koch, K. (2017). Plant surfaces: structures and functions for biomimetic innovations. Nano-Micro Lett. 9, 23. doi:10.1007/s40820-016-0125-1
Bhushan, B. (2009). Biomimetics: lessons from nature – an overview. Phil. Trans. R. Soc. A 367, 1445–1486. doi:10.1098/rsta.2009.0011
Bhushan, B. (2018). Biomimetics bioinspired hierarchical-structured surfaces for green science and technology. 3rd edition. Germany: Springer Nature Switzerland.
Burgert, I., Cabane, E., Zollfrank, C., and Berglund, L. (2015). Bio-inspired functional wood-based materials – hybrids and replicates. Int. Mater. Rev. 60, 431–450. doi:10.1179/1743280415Y.0000000009
Cai, C., Chen, Z., Chen, Y., Li, H., Yang, Z., and Liu, H. (2021). Mechanisms and applications of bioinspired underwater/wet adhesives. J. Polym. Sci. 59, 2911–2945. doi:10.1002/pol.20210521
Chapman, J., Hellio, C., Sullivan, T., Brown, R., Russell, S., Kiterringham, E., et al. (2014). Bioinspired synthetic macroalgae: examples from nature for antifouling applications. Int. Biodeterior. Biodegrad. 86, 6–13. doi:10.1016/j.ibiod.2013.03.036
Chen, P. Y., McKittrick, J., and Meyers, M. A. (2012). Biological materials: functional adaptations and bioinspired designs. Prog. Mater. Sci. 57, 1492–1704. doi:10.1016/j.pmatsci.2012.03.001
Cremaldi, J. C., and Bhushan, B. (2018). Bioinspired self-healing materials: lessons from nature. J. Nanotechnol. 9, 907–935. doi:10.3762/bjnano.9.85
Cui, C., and Liu, W. (2021). Recent advances in wet adhesives: adhesion mechanism, design principle and applications. Prog. Polym. Sci. 116, 101388. doi:10.1016/j.progpolymsci.2021.101388
Cui, J., Qin, Z., Masic, A., and Buehler, M. J. (2020). Multiscale structural insights of load bearing bamboo: a computational modeling approach. J. Mech. Behav. Biomed. Mater 107, 103743. doi:10.1016/j.jmbbm.2020.103743
de Araujo, C., Balestrino, D., Roth, L., Charbonnel, N., and Forestier, C. (2010). Quorum sensing affects biofilm formation through lipopolysaccharide synthesis in Klebsiella pneumoniae. Res. Microbiol. 161, 595–603. doi:10.1016/j.resmic.2010.05.014
Delgado Camacho, D., Clayton, P., O’Brien, W. J., Seepersad, C., Juenger, M., Ferron, R., et al. (2018). Applications of additive manufacturing in the construction industry – a forward-looking review. Autom. Constr. 89, 110–119. doi:10.1016/j.autcon.2017.12.031
Del Grosso, C. A., Leng, C., Zhang, K., Hung, H.-C., Jiang, S., Chen, Z., et al. (2020). Surface hydration for antifouling and bio-adhesion. Chem. Sci. 11, 10367–10377. doi:10.1039/D0SC03690K
Demirel, Y. (2014). Thermodynamics and biological systems. Thermodyn. Biol. Syst., 485–562. doi:10.1016/B978-0-444-59557-7.00011-4
Dumanli, A. G., and Savin, T. (2016). Recent advances in the biomimicry of structural colours. Chem. Soc. Rev. 45, 6698–6724. doi:10.1039/c6cs00129g
Dumont, E. R., (2010). Bone density and the lightweight skeletons of birds, Proc. R. Soc. B Biol. Sci. 277, 2193–2198. doi:10.1098/rspb.2010.0117
Dunlop, J. W. C., and Fratzl, P. (2010). Biological composites. Annu. Rev. Mat. Res. 40, 1–24. doi:10.1146/annurev-matsci-070909-104421
Eadie, L., and Ghosh, T. K. (2011). Biomimicry in textiles: past, present and potential. An overview. J. R. Soc. Interface 8, 761–775. doi:10.1098/rsif.2010.0487
Fratzl, P., and Weinkamer, R. (2007). Nature’s hierarchical materials. Prog. Mater. Sci. 52, 1263–1334. doi:10.1016/j.pmatsci.2007.06.001
Gan, K., Liang, C., Bi, X., Wu, J., Ye, Z., Wu, W., et al. (2022). Adhesive materials inspired by barnacle underwater adhesion: biological principles and biomimetic designs. Front. Bioeng. Biotechnol. 10, 870445. doi:10.3389/fbioe.2022.870445
Goerlitzer, E. S. A., Klupp Taylor, R. N., and Vogel, N. (2018). Bioinspired photonic pigments from colloidal self-assembly. Adv. Mat. 30, e1706654–e1706654. doi:10.1002/adma.201706654
Goss, D., Mistry, Y., Niverty, S., Noe, C., Santhanam, B., Ozturk, C., et al. (2020). Bioinspired honeycomb core design: an experimental study of the role of corner radius, coping and interface. Biomimetics 4, (4):59. doi:10.3390/biomimetics5040059
Grant, P. R., Grant, B. R., Huey, R. B., Johnson, M. T. J., Knoll, A. H., and Schmitt, J. (2017). Evolution caused by extreme events. Philos. Trans. R. Soc. Lond B Biol. Sci. 372, 20160146, 20160146. doi:10.1098/rstb.2016.0146
Guan, Y., Chen, R., Sun, G., Liu, Q., Liu, J., Yu, J., et al. (2021). Secretion mechanism and adhesive mechanism of diatoms: direct evidence from the quantitative analysis. Micron 140, 102951. doi:10.1016/j.micron.2020.102951
Guo, Q., Dai, E., Han, X., Xie, S., Chao, E., and Chen, Z. (2015). Fast nastic motion of plants and bioinspired structures. J. R. Soc. Interface 12 (110), 20150598. doi:10.1098/rsif.2015.0598
Guo, Z., Liu, W., and Su, B. L. (2011). Superhydrophobic surfaces: from natural to biomimetic to functional. J. Colloid Interface Sci. 353, 335–355. doi:10.1016/j.jcis.2010.08.047
Heveran, C. M., Williams, S. L., Qiu, J., Artier, J., Hubler, M. H., Cook, S. M., et al. (2020). Biomineralization and successive regeneration of engineered living building materials. Matter 2 (2), 481–494. doi:10.1016/j.matt.2019.11.016
Holland, O., Shaw, J., Stark, J. S., and Wilson, K. A. (2021). Hull fouling marine invasive species pose a very low, but plausible, risk of introduction to East Antarctica in climate change scenarios. Divers. Distrib. 27, 973–988. doi:10.1111/ddi.13246
Holten-Anderson, N., Harrington, M. J., Birkedal, H., Lee, B. P., Messersmith, P. B., Lee, K. Y. C., et al. (2011). pH-induced metal–ligand crosslinks inspired by mussel yield self-healing polymer networks with near-covalent elastic moduli. Proc. Natl. Acad. Sci. U. S. A. 108, 2651–2655. doi:10.1073/pnas.1015862108
Imani, M., Donn, M., and Balador, Z. (2018). “Bio-inspired materials: contribution of biology to energy efficiency of buildings,” in Handbook of ecomaterials (Germany: Springer International Publishing AG).
Irzmańska, E., Jastrzębska, A., and Michalski, M. (2022). A biomimetic approach to protective glove design: inspirations from nature and the structural limitations of living organisms. AUTEX Res. J. 23, 1. doi:10.2478/aut-2022-0004
Jaffe, M. J., Leopold, A. C., and Staples, R. C. (2002). Thigmo responses in plants and fungi. Am. J. Bot. 89, 375–382. doi:10.3732/ajb.89.3.375
Ke, P., Jiao, X. N., Ge, X. H., Xiao, W. M., and Yu, B. (2014). From macro to micro: structural biomimetic materials by electrospinning. RSC Adv. 75 (4), 39704–39724. doi:10.1039/C4RA05098C
Knippers, J., and Speck, T. (2012). Design and construction principles in nature and architecture. Bioinspir Biomim. 7, 015002. doi:10.1088/1748-3182/7/1/015002
Koch, E., and Barthlott, W. (2009). Superhydrophobic and superhydrophilic plant surfaces: an inspiration for biomimetic materials. Phil. Trans. R. Soc. A 367, 1487–1509. doi:10.1098/rsta.2009.0022
Lee, M. (2014). Remarkable natural material surfaces and their engineering potential. Germany: Springer International Publishing Switzerland.
Lee, S. M., and Kwon, T. H. (2007). Effects of intrinsic hydrophobicity on wettability of polymer replicas of a superhydrophobic lotus leaf. J. Micromech. Microeng. 17 (4), 687–692. doi:10.1088/0960-1317/17/4/003
Lenau, T. A., Orrù, A. M., and Linkola, L., (2018). Biomimicry in the nordic countries, doi:10.6027/10.6027/NA2018-906
Lendlein, A., and Gould, O. E. C. (2019). Reprogrammable recovery and actuation behaviour of shape-memory polymers. Nat. Rev. Mater 4, 116–133. doi:10.1038/s41578-018-0078-8
Li, M. H., He, B., Qin, H. Y., Zhou, Y. M., Lu, H. X., and Yue, J. G. (2011). A wet adhesion inspired biomimetic pad with direction dependence and adaptability. Chin. Sci. Bull. 56, 1935–1941. doi:10.1007/s11434-011-4375-5
Libonati, F., and Vergani, L. (2016). Cortical bone as a biomimetic model for the design of new composites. Procedia Struct. Integr. 2, 1319–1326. doi:10.1016/j.prostr.2016.06.168
Lienhard, J., Schleicher, S., Poppinga, S., Masselter, T., Milwich, M., Speck, T., et al. (2011). Flectofin: a hingeless flapping mechanism inspired by nature. Bioinspir. Biomim. 6, 045001. doi:10.1088/1748-3182/6/4/045001
Liu, S., and Xu, W. (2020). Engineered living materials-based sensing and actuation. Front. Sens. 1, 586300. doi:10.3389/fsens.2020.586300
López, M., Rubio, R., Martín, S., and Croxford, B. (2017). How plants inspire façades. From plants to architecture: biomimetic principles for the development of adaptive architectural envelopes. Renew. Sustain. Energy Rev. 67, 692–703. doi:10.1016/j.rser.2016.09.018
Lurie-Luke, E. (2014). Product and technology innovation: what can biomimicry inspire? Biotechnol. Adv. 3, 1494–1505. doi:10.1016/j.biotechadv.2014.10.002
Mabon, L. (2020). Environmental justice in urban greening for subtropical Asian cities: the view from Taipei. Singap. J. Trop. Geogr. 41 (3), 432–449. doi:10.1111/sjtg.12341
Mazzolai, B., Beccai, L., and Mattoli, V. (2014). Plants as model in biomimetics and biorobotics: new perspectives. Front. Bioeng. Biotechnol. 2, 2. doi:10.3389/fbioe.2014.00002
Mazzucchelli, E. S., and Doniacovo, L. (2017).The integration of BIPV Adaptive Flakes in the building envelope, Proceedings of the 12th Conference on Advanced Building Skins, 2-3 October 2017, Switzerland. Bern, 1331–1340.
McBee, R. M., Lucht, M., Mukhitov, N., Richardson, M., Srinivasan, T., Meng, D., et al. (2022). Engineering living and regenerative fungal–bacterial biocomposite structures. Nat. Mat. 21, 471–478. doi:10.1038/s41563-021-01123-y
Müller, R., Abaid, N., Boreyko, J. B., Fowlkes, C., Goel, A. K., Grimm, C., et al. (2018). Biodiversifying bioinspiration. Biodiversifying Bioinspiration. Bioinspir. Biomim. 13, 053001. doi:10.1088/1748-3190/aac96a
Naidu, K. S. S. T., Rao, M. S., and Vempada, R. R. (2018). Analytical model for predicting stress-strain behaviour of bacterial concrete. Int. J. Civ. Eng. Technol. 9 (11), 2383–2393.
National Research Council (2008). Inspired by biology: from molecules to materials to machines. Washington, DC, USA: National Academies Press.
Nature Browse the biomimicry taxonomy, ask nature, the biomimicry Institute. Available at: http://toolbox.biomimicry.org/wp-content/uploads/2015/01/AN_Biomimicry_Taxonomy.pdf.
Niu, S., Li, B., Mu, Z., Yang, M., Zhang, J., Han, Z., et al. (2015). Excellent structure-based multifunction of morpho butterfly wings: a review. A Rev. J. Bionic Eng. 12, 170–189. doi:10.1016/S1672-6529(14)60111-6
NSF Report (2023). NSF Report bio-inspired design. Available at: https://nsf-gov-resources.nsf.gov/2023-03/Bio-inspired%20Design%20Workshop%20Report_2232327_October%202022_Final.508.pdf.
Ortega Del Rosario, M. D. L. Á., Beermann, K., and Chen Austin, M. (2023). Environmentally responsive materials for building envelopes: a review on manufacturing and biomimicry-based approaches. Biomimetics 8, 52. doi:10.3390/biomimetics8010052
Ouyang, Z., Chen, Y., Yan, Y., Qin, H., and Liu, Y. (2022). Mechanical model of hook-loop adhesion. Int. J. Solids Struct. 243, 111589. doi:10.1016/j.ijsolstr.2022.111589
Poohphajai, F., Sandak, J., Sailer, M., Rautkari, L., Belt, T., and Sandak, A. (2021). Bioinspired living coating system in service: evaluation of the wood protected with biofinish during one-year natural weathering. Coatings 11 (6), 701. doi:10.3390/coatings11060701
Poppinga, S., Zollfrank, C., Prucker, O., Rühe, J., Menges, A., Cheng, T., et al. (2018). Toward a new generation of smart biomimetic actuators for architecture. Adv. Mater. 30 (19), 1703653. doi:10.1002/adma.201703653
Postiglione, R. A. (1993). Velcro and seed dispersal. Am. Biol. Teach. 55 (1), 44–45. doi:10.2307/4449577
Preti, L., Lambiase, B., Campodoni, E., Sandri, M., Ruffini, A., Pugno, N., et al. (2019). Nature-inspired processes and structures: new paradigms to develop highly bioactive devices for hard tissue regeneration, bio-inspired technology. IntechOpen: Ruby Srivastava. doi:10.5772/intechopen.82740
Reddy, S. T., Chung, K. K., McDaniel, C. J., Darouiche, R. O., Landman, J., and Brennan, A. B. (2011). Micropatterned surfaces for reducing the risk of catheter-associated urinary tract infection: an in vitro study on the effect of Sharklet micropatterned surfaces to inhibit bacterial colonization and migration of uropathogenic Escherichia coli. J. Endourol. 25 (9), 1547–1552. doi:10.1089/end.2010.0611
Rivera-Tarazona, L. K., Campbell, Z. T., and Ware, T. H., (2020). Stimuli-responsive engineered living materials. Soft Matter, 17, 785–809. doi:10.1039/D0SM01905D
Rodrigo-Navarro, A., Sankaran, S., Dalby, M. J., del Campo, A., and Salmeron-Sanchez, M. (2021). Engineered living biomaterials. Nat. Rev. Mater 6, 1175–1190. doi:10.1038/s41578-021-00350-8
Sailer, M., van Nieuwenhuijzen, E. J., and Knol, W. (2010). Forming of a functional biofilm on wood surfaces. Ecol. Eng. 36 (2), 163–167. doi:10.1016/j.ecoleng.2009.02.004
Saito, A. (2011). Material design and structural color inspired by biomimetic approach. Sci. Technol. Adv. Mater. 12 (6), 064709. doi:10.1088/1468-6996/12/6/064709
Sandak, A. (2023). Engineered living materials for sustainable and resilient architecture. Nat. Rev. Mater. 8, 357–359. doi:10.1038/s41578-023-00554-0
Sandak, A., Brzezicki, M., and Sandak, J. (2020). “Trends and perspectives in the use of timber and derived products in building façades,” in New materials in civil engineering. (USA: Butterworth-Heinemann).
Sandak, A., Sandak, J., Brzezicki, M., and Kutnar, A. (2019). Bio-based building skin. Singapore: Springer.
Shin, M., Shin, J. Y., Kim, K., Yang, B., Han, J. W., Kim, N. K., et al. (2020). The position of lysine controls the catechol-mediated surface adhesion and cohesion in underwater mussel adhesion. J. Colloid Interface Sci. 563, 168–176. doi:10.1016/j.jcis.2019.12.082
Si, Y., and Guo, Z. (2015). Superhydrophobic nanocoatings: from materials to fabrications and to applications. Nanoscale 7, 5922–5946. doi:10.1039/C4NR07554D
Sidor, L. M., and Meyer, A. S. (2022). “Additive manufacturing of engineered living materials,” in Engineered living materials. Editor W. V. SrubarIII (Cham: Springer). doi:10.1007/978-3-030-92949-7_6
Siebert, H., and Wilker, J. J. (2019). Deriving commercial level adhesive performance from a bio-based mussel mimetic polymer. ACS Sustain. Chem. Eng. 7 (15), 13315–13323. doi:10.1021/acssuschemeng.9b02547
Silverman, H. G., and Roberto, F. F. (2007). Understanding marine mussel adhesion. Mar. Biotechnol. 9, 661–681. doi:10.1007/s10126-007-9053-x
Simons, P. (1992). The action plant: movement and nervous behaviour in plants. China: Blackwell Publishers.
Song, K., and Lee, S. J. (2017). Pine cone scale-inspired motile origami. NPG Asia Mater. 9, e389. doi:10.1038/am.2017.79
Speck, O., and Speck, T. (2021). Biomimetics and education in europe: challenges, opportunities, and variety. Biomimetics 6, 49. doi:10.3390/biomimetics6030049
Speck, T., and Speck, O. (2008). Process sequences in biomimetic research. WIT Trans. Ecol. Environ. 114 (10), 3–11. doi:10.2495/DN080011
Speck, T., and Speck, O. (2019). An overview of bioinspired and biomimetic self-repairing materials. Biomimetics 4, 26. doi:10.3390/biomimetics4010026
Srubar, , and Wil, V. (2021). Engineered living materials: taxonomies and emerging trends. Trends Biotechnol. 39 (6), 574–583. doi:10.1016/j.tibtech.2020.10.009
W. V. SrubarIII (Editor) (2022). Engineered living materials (Germany: Springer Nature). doi:10.1007/978-3-030-92949-7
Sun, W., Liu, T., Zhang, X., Zhang, X., Yan, Q., Yin, J., et al. (2023). Aquatic diatoms-inspired universal adhesive coacervates triggered by water. Adv. Healthc. Mater 12, e2300669. doi:10.1002/adhm.202300669
Wang, D., Chen, D., and Chen, Z. (2020). Recent progress in 3D printing of bioinspired structures. Front. Mater. 7. doi:10.3389/fmats.2020.00286
Wang, W., Liu, Y., and Xie, Z. (2021). Gecko-like dry adhesive surfaces and their applications: a review. J. Bionic Eng. 18, 1011–1044. doi:10.1007/s42235-021-00088-7
Wang, Y., Naleway, S. E., and Wang, B. (2020b). Biological and bioinspired materials: structure leading to functional and mechanical performance. Bioact. Mater 5, 745–757. doi:10.1016/j.bioactmat.2020.06.003
Wei, X., Wang, J., Yang, X., Wu, J., Hong, R., Liu, Y., et al. (2023). Wet adhesion enhancement through citric-acid-regulated supramolecular network. Compos. Part B Eng. 265, 110964. doi:10.1016/j.compositesb.2023.110964
Whitesides, G. M. (2015). Bioinspiration: something for everyone. Interface Focus 5, 20150031. doi:10.1098/rsfs.2015.0031
Xiao, M., Li, Y., Allen, M. C., Deheyn, D. D., Yue, X., Zhao, J., et al. (2015). Bio-inspired structural colors produced via self-assembly of synthetic melanin nanoparticles. ACS Nano 9, 5454–5460. doi:10.1021/acsnano.5b01298
Xie, J., Li, M., Dai, Q., Huang, W., and Wang, X. (2018). Key parameters of biomimetic patterned surface for wet adhesion. Int. J. Adhesion Adhesives 82, 72–78. doi:10.1016/j.ijadhadh.2018.01.004
Xu, Q., Zhang, W., Dong, C., Sreeprasad, T. S., and Xia, Z. (2016). Biomimetic self-cleaning surfaces: synthesis, mechanism and applications. J. R. Soc. Interface 13, 20160300. doi:10.1098/rsif.2016.0300
Yamamoto, M., Nishikawa, N., Mayama, H., Nonomura, Y., Yokojima, S., Nakamura, S., et al. (2015). Theoretical explanation of the Lotus effect: superhydrophobic property changes by removal of nanostructures from the surface of a Lotus leaf. Langmuir 31, 7355–7363. doi:10.1021/acs.langmuir.5b00670
Yang, W. J., Neoh, K. G., Kang, E. T., Teo, S. L. M., and Rittschof, D. (2014). Polymer brush coatings for combating marine biofouling. Prog. Polym. Sci. 39 (5), 1017–1042. doi:10.1016/j.progpolymsci.2014.02.002
Yang, Y., Song, X., Li, X., Chen, Z., Zhou, C., Zhou, Q., et al. (2018). Recent progress in biomimetic additive manufacturing technology: from materials to functional structures. Adv. Mater. 1706539, e1706539. doi:10.1002/adma.201706539
Yaraghi, N. A., and Kisailus, D. (2018). Biomimetic structural materials: inspiration from design and assembly. Annu. Rev. Phys. Chem. 69, 1. doi:10.1146/annurev-physchem-040215-112621
Youssefian, S., and Rahbar, N. (2015). Molecular origin of strength and stiffness in bamboo fibrils. Sci. Rep. 5, 11116. doi:10.1038/srep11116
Zari, M. P. (2007). Biomimetic approaches to architectural design for increased sustainability. Wellington: Victoria University. Paper number: 033, School of Architecture.
Zhang, T., Liang, T., Yue, X., and Sameoto, D. (2019). Integration of thermoresponsive velcro-like adhesive for soft robotic grasping of fabrics or smooth surfaces, 2nd IEEE international conference on soft robotics (RoboSoft). USA: IEEE, 120–125.
Keywords: biomimicking, bioinspiration, climate adaptations, building materials, sustainable architecture, engineered living materials
Citation: Sandak A and Butina Ogorelec K (2023) Bioinspired building materials—lessons from nature. Front. Mater. 10:1283163. doi: 10.3389/fmats.2023.1283163
Received: 25 August 2023; Accepted: 30 October 2023;
Published: 16 November 2023.
Edited by:
Daniel Garcia-Gonzalez, Universidad Carlos III de Madrid, SpainReviewed by:
Ille C. Gebeshuber, Vienna University of Technology, AustriaZhao Qin, Syracuse University, United States
Copyright © 2023 Sandak and Butina Ogorelec. This is an open-access article distributed under the terms of the Creative Commons Attribution License (CC BY). The use, distribution or reproduction in other forums is permitted, provided the original author(s) and the copyright owner(s) are credited and that the original publication in this journal is cited, in accordance with accepted academic practice. No use, distribution or reproduction is permitted which does not comply with these terms.
*Correspondence: Anna Sandak, YW5uYS5zYW5kYWtAaW5ub3JlbmV3LmV1