- 1China Construction Sixth Engineering Bureau Co., Ltd., Tianjin, China
- 2China Construction Sixth Engineering Bureau Civil Engineering Co., Ltd., Tianjin, China
- 3Department of Civil, Environmental and Natural Resources Engineering, Luleå University of Technology, Luleå, Sweden
- 4Department of Civil Engineering, School of Engineering, Monash University Malaysia, Bandar Sunway, Selangor, Malaysia
- 5Department of Civil Engineering, COMSATS University Islamabad, Abbottabad, Pakistan
- 6Department of Civil Engineering, University of Engineering and Technology, Taxila, Pakistan
Engineered cementitious composites (ECC) exhibits impressive tensile strength but has significant environmental drawbacks due to high cement consumption. Recently, engineered geopolymer composites (EGC) have gained attention as a potential ECC alternative. This comprehensive study reviews the latest EGC advancements, encompassing mix design, design theory, engineering properties, environmental benefits, and durability. It emphasizes how factors like activators, precursors, fibers, additives, and aggregates impact EGC properties, making it a cost-effective material for fire, chemical resistance, and dynamic loads. To address limitations in traditional literature reviews, innovative research methods, including scientometric analysis, were employed to provide a cohesive analysis. This review aims to facilitate knowledge dissemination and collaboration by summarizing EGC advances and highlighting remaining challenges in developing practical applications. It is revealed from the review that various manufacturing methods enhance geopolymers, especially in geopolymer concrete, where replacing 50% of ordinary Portland cement with fly ash boosts strength. Geopolymer concrete excels in pre-cast applications, offering durability and resistance to harsh conditions as an eco-friendly alternative to Portland cement. It suits highway pavement, walls, marine coatings, and tiles, reducing carbon emissions and promoting efficient waste management. EGCs find broad use in construction due to their strong, durable, and eco-friendly qualities, supporting sustainable infrastructure development.
1 Introduction
Cementitious concrete composite is an extensively used construction material that is strong in compression but has limited tensile properties (Khan et al., 2018; Farooqi and Ali, 2019; Li, 2019). To cater this issue, the interest of researchers has increased towards materials advancements in the form of fiber-reinforced concrete (Shakor and Pimplikar, 2011; Khan et al., 2022a; Farooqi and Ali, 2022). However, ordinary fiber-reinforced concrete (FRC) only slightly improves tensile strain capacity despite yielding advanced tensile properties compared to conventional concrete (Cao and Khan, 2021; Khan et al., 2022b). In the early 1990s, the development of engineered cementitious composites (ECC) ushered in a significant improvement in tensile ductility. ECC demonstrated remarkable attributes such as multiple cracking behavior and strain-hardening, resulting in significantly enhanced tensile properties compared to conventional concrete and fiber-reinforced composites. Moreover, ECC exhibited the ability to resist crack propagation under external loads, with cracks reaching a certain point and generating additional tensile distortion due to micro-crack emergence (Şahmaran and Li, 2009; Qaidi et al., 2022). These outstanding characteristics made ECC a preferred choice for extensive projects like bridges and high-rise buildings, ensuring prolonged service life and improved functionality (Maruta, 2005; Luković et al., 2019; Li et al., 2020).
Engineered cementitious composites (ECC) typically comprise cement, silica sand, fly ash, and fibers (Wang and Li, 2007; Shanmugasundaram and Praveenkumar, 2021). However, ECC’s heavy reliance on cement and its lack of coarse aggregates necessitates two to three times more cement usage than conventional concrete, contributing to approximately 8% of global CO2 emissions from cement production (Luukkonen et al., 2018; Riaz Ahmad et al., 2023). This unsustainable production pattern has driven the exploration of alternative binders for ECC, including geopolymer concrete. Geopolymers, comprised of alkaline activators and aluminosilicate materials, have emerged as a more sustainable alternative in developing engineered geopolymer composites (EGC) (Shakor et al., 2023). Geopolymer concrete’s ecological friendliness in construction materials is due to the abundant availability of raw materials, robust mechanical properties, and exceptional durability (Davidovits, 1991; Bakharev et al., 1999; Bakharev et al., 2003; Lemougna et al., 2016; Wang et al., 2017).
Compared to traditional concrete, EGC exhibits nearly identical or superior mechanical characteristics and durability while significantly reducing CO2 emissions (Yang et al., 2013; Provis, 2018; Lao et al., 2023a; Lao et al., 2023b). Furthermore, when compared to ECC, EGC boasts similar static loading properties but outperforms it in terms of dynamic loading properties (Shaikh, 2013; Nematollahi et al., 2017; Trindade et al., 2020; Cai et al., 2021; Zhang et al., 2022). EGC can be tailored to various material properties by employing diverse mix designs, fiber contents, mixing techniques, and curing methods (Ohno and Li, 2018; Zahid et al., 2018; Zhong and Zhang, 2022). Figure 1 illustrates how the utilization of such waste materials can positively impact both the economy and the environment, particularly given the abundance of these materials and the growing demand for cost-effective construction as the population continues to expand (Jindal, 2019; Ilcan et al., 2022; Lan et al., 2022; Sandanayake et al., 2022; Ahmad et al., 2023).
Geopolymer concrete obtains superior mechanical properties from the three-dimensional network structures of oxides generated through specialized inorganic polycondensation. This stable network structure grants remarkable durability and high strength to the geopolymer concrete during service. This protective effect on engineering structures ensures the concrete’s longevity (Davidovits, 1991; Wang et al., 2021; Rajak et al., 2022). Geopolymer concrete’s cementitious materials can be classified into three categories based on their calcium content: no calcium, low calcium, and high calcium (Chindaprasirt et al., 2007; Duxson et al., 2007; Adamiec et al., 2008; Provis and Van Deventer, 2009; Elchalakani et al., 2018; Luukkonen et al., 2018). The primary representatives of these categories are metakaolin, fly ash, and slag, respectively. Geopolymer concrete with metakaolin exhibits greater strength and durability than OPC-based materials (Mohmmad et al., 2023). However, the plate-shaped particles of metakaolin create rheological difficulties that complicate the manufacturing process (Adamiec et al., 2008; Li et al., 2010; Luukkonen et al., 2018). In alkali-activated reaction products of slag, the C-S-H and C-A-S-H gels with low Ca/Si ratios provide high strength and acid resistance to slag-based geopolymer materials (Provis and Van Deventer, 2009). Nonetheless, the high-temperature endurance of geopolymer materials based on slag reduces, while their dry shrinkage deformation and creep rise (Buchwald et al., 2005; Atiş et al., 2009; Khan and Ali, 2020).
Given the diverse mix compositions, intricate high-temperature mechanical properties, and varied testing methods, it is vital to comprehensively analyze existing research on the temperature-dependent material traits of Engineered Geopolymer Composites (EGC). This review primarily focuses on the influence of material composition and manufacturing procedures on EGC mechanical properties and durability. It aims to identify challenges in developing EGC with practical applicability. While prior reviews have touched on EGC characteristics and research prospects, this review offers a more extensive analysis of the interplay among critical material traits, addressing a research gap. This study systematically categorizes and consolidates research findings, providing a reliable reference for experts in this field. The scarcity of information in EGC research hinders innovation and collaboration. Therefore, establishing a plan to access crucial materials from dependable sources is crucial. A scientometric approach, facilitated by appropriate software tools, can address these limitations. This study conducts a scientometric analysis of bibliographic data published in 2023 regarding EGC utilization. The analysis reveals prolific publication sources, authors, related citations, co-occurring keywords, contributing countries, and highly cited articles in the EGC research domain. Data from relevant documents, including abstracts, bibliographies, keywords, funding information, and citations, are retrieved using the Scopus search engine. The VOS viewer tool is then employed for data analysis. Statistical and graphical representations of countries and academics can facilitate idea exchange and research collaboration. This study combines scientometric analysis with a comprehensive literature review to underscore the significance of EGC and its future potential.
2 Research significance
This article’s significance lies in addressing a critical issue in construction, i.e., the environmental impact of conventional cement-based materials. It aligns with global sustainability goals by highlighting Engineered Geopolymer Composites (EGCs) as a sustainable alternative. Given the extensive array of mix compositions available for EGCs, their intricate mechanical behavior under elevated temperatures, and the diverse heating methodologies employed in material testing, it becomes imperative to thoroughly examine the existing body of research concerning the temperature-dependent material attributes of EGCs. Additionally, it explores EGC advancements in mix design, engineering properties, and more, potentially leading to versatile and robust construction materials for various applications. While prior reviews have touched upon certain material traits and research prospects pertaining to EGC, this review delivers a more exhaustive examination of the interrelationships among pivotal material attributes to bridge the extant research gap. The article employs innovative research methods like scientometric analysis, setting a precedent for interdisciplinary approaches. It emphasizes cost-effective solutions by understanding factors influencing EGC properties enhancing accessibility for diverse projects. EGCs’ versatility, especially in geopolymer concrete, offers eco-friendly options for construction applications, supporting sustainable infrastructure development. Furthermore, the review facilitates knowledge dissemination and collaboration in the construction industry. In summary, this article has the potential to drive innovation, reduce environmental impact, and promote sustainable practices through EGC adoption, addressing crucial construction sector challenges.
3 Methodology
The present study employs a scientometric analysis (Afgan and Bing, 2021; Amin et al., 2022; Huang et al., 2022) of bibliographic data to quantify its various properties. The scientometric analysis utilizes scientific mapping, a method researchers devised to examine bibliometric data (Markoulli et al., 2017; Amin et al., 2022). In light of the extensive literature within the less-explored research field, employing a reliable search engine is crucial. Scholarly consensus suggests two databases, specifically Scopus and Web of Science, as dependable sources to fulfill the research objectives (Afgan and Bing, 2021; Huang et al., 2022). Scopus is selected to gather bibliographic data related to EGC applications research. The search on Scopus resulted in relevant findings, with several filters applied to scrutinize irrelevant data. Figure 2 illustrates a comprehensive flowchart that depicts the methodical steps, including data retrieval, data analysis, and the application of various filters in the analysis.
Previous studies have utilized the same approach (Oraee et al., 2017; Jin et al., 2018; Park and Nagy, 2018). The Scopus database is stored in CSV format for further analysis using appropriate software. The research team utilized the open-source VOS viewer tool (version 1.6.18) to perform a quantitative analysis and scientific visualization of the collected data, which is also commonly used in this type of research (Zuo and Zhao, 2014; Darko et al., 2017; Ahmad et al., 2021). Therefore, the study’s objectives are achieved by utilizing a VOS viewer. The VOS viewer software is used to analyze the data collected from Scopus, and the CSV file is imported into the VOS viewer for further evaluation. A scientometric analysis is then conducted to examine widely used keywords, publication sources, authors with the most citations and articles, the contributions of different countries, and highly cited papers. The analysis generates maps that illustrate the features, their co-occurrences, and interconnectivity; relevant statistics are presented in tables. Different colors are assigned to specific elements in the map to differentiate between groups. Density mapping is achieved using colors such as rainbow, plasma, rainbow, and Viridis.
4 Findings and evaluation
4.1 Subject areas and annual publication trends
In order to identify the principal research areas, an analysis using Scopus is performed. As illustrated in Figure 3, nearly 38% of documents are found within the “Engineering” discipline, and “Materials Science” also accounts for almost 38% of the documents. These two disciplines contribute to a total of 76% of the papers. Furthermore, a Scopus database analysis identifies the publication type within the researched field, as presented in Figure 4. Based on the analysis, journal articles comprise around 75% of publications, conference articles account for almost 10%, and conference review papers represent approximately 6%. Figure 5 illustrates the annual trend of publications related to EGC implications from 2014 to the start of 2023. It is important to note that the number of articles in this research area increased from 2014 to 2018. However, a minor decline in publication quantity was observed in 2018–2019. From 2019 onwards, a significant enhancement can be observed in the publications trend in this research area. It represents an encouraging trend of researchers’ growing attention towards using EGC.
4.2 Origins of publication
VOS Viewer analyses bibliographic data in this study to identify publication sources pertaining to Engineered geopolymer composites (EGC). Only sources with at least five relevant articles are taken into consideration. The findings are shown in Figure 6, which presents the number of publications from each source as of early 2023. Among the identified sources, the journal “Construction and Building Materials” is at the top to have the maximum number of publications, followed by “Cement and Concrete Composites,” which is subsequently followed by “Ceramics International.” The sources “Journal of Building Engineering” and “Composites Part B: Engineering” ranked fourth and fifth, respectively. These findings provide a fundamental basis for future scientometric analyses on research related to the practical implications of EGC.
4.3 Co-occurrence of keywords
Keywords play a crucial role in research by helping to distinguish and highlight the primary focus of a particular study (Song et al., 2021). In order to ensure adequate variation, a minimum keyword repetition threshold of 110 is employed. Table 1 provides an overview of the 20 keywords analyzed, with “Geopolymers,” “Geopolymer Composites,” “Compressive Strength,” “Strain Hardening,” and “Fly Ash” emerging as the top five most commonly used keywords in the field of EGC. Keyword analysis highlights that research in EGC primarily focuses on developing its mechanical properties like compressive strength and strain hardening. Figure 7 visually represents the frequency and connections of the analyzed keywords, facilitating a better understanding of their distribution and density within the research field. The circle size in Figure 7A indicates the frequency of each keyword, while their placement denotes co-occurrence in articles. The larger circles correspond to the most prominent keywords, emphasizing their importance in exploring the literature on EGC. Group formations represent co-occurrences of keywords in different publications, with distinct colors assigned to each group. Different colors allow easy differentiation of the four discrete groups shown in Figure 7A. Figure 7B illustrates the dense concentrations of keywords using various colors. The colors are arranged based on density, with red representing the highest density and green, blue, yellow, and purple indicating lower densities. From the particular note, the “Geopolymers,” “Fly Ash,” and “Strain Hardening” keywords are highlighted in red, indicating their high frequency of occurrence. This information can be helpful for new researchers in selecting appropriate keywords for their studies and facilitating their search for relevant publications in this research field.
4.4 Authors
One’s influence within a specific research field can be determined by examining citation records (Yu and Hayes, 2018). The study applied a minimum threshold of at least three articles from a specific author. VOS viewer is utilized to analyze bibliographic data and gain insights into the number of publications and citations of the authors within the research area. However, it is worth noting that evaluating an author’s impact based solely on the number of publications and citations may not always be the most accurate or comprehensive method. Other factors, such as the quality and significance of the work, the influence on the field, and the potential for future impact, may also be important considerations. Therefore, assessing the ranking of each parameter independently may be more effective. The interconnectivity between authors who have published at least three articles in this area is visualized in Figure 8. The analysis identified Nematollahi B. as the leading author, followed by Sanjayan J., Dai J.-G., Li V.C., and Shaikh F.U.A. Nematollahi B. and Sanjayan J. also had the highest number of citations, with 335 citations, followed by Dai J.-G. with 186 citations in the field of EGC research. In conclusion, the analysis suggests significant interconnectivity between authors, as far as their citations are concerned, who participated actively in research on EGC.
4.5 Documents
Citations received by a research paper are an important indicator of their significance in a particular research field. Typically, pioneering articles in a specific research domain have the highest citations. Figure 9 presents the density concentration and scientific visualization of citations for interrelated articles in the EGC research. The figure is divided into two parts: Figure 9A displays the connected articles based on citations extracted from VOS viewer, where the circle size for each article corresponds to its impact within the considered research domain. The visualization summarizes the interconnectivity between articles and the most influential articles in the field. Figure 9B, on the other hand, depicts the increasing trend of research articles by authors with an increase in years. The figure suggests that research on Engineered geopolymer composites (EGC) is a growing area of interest for researchers.
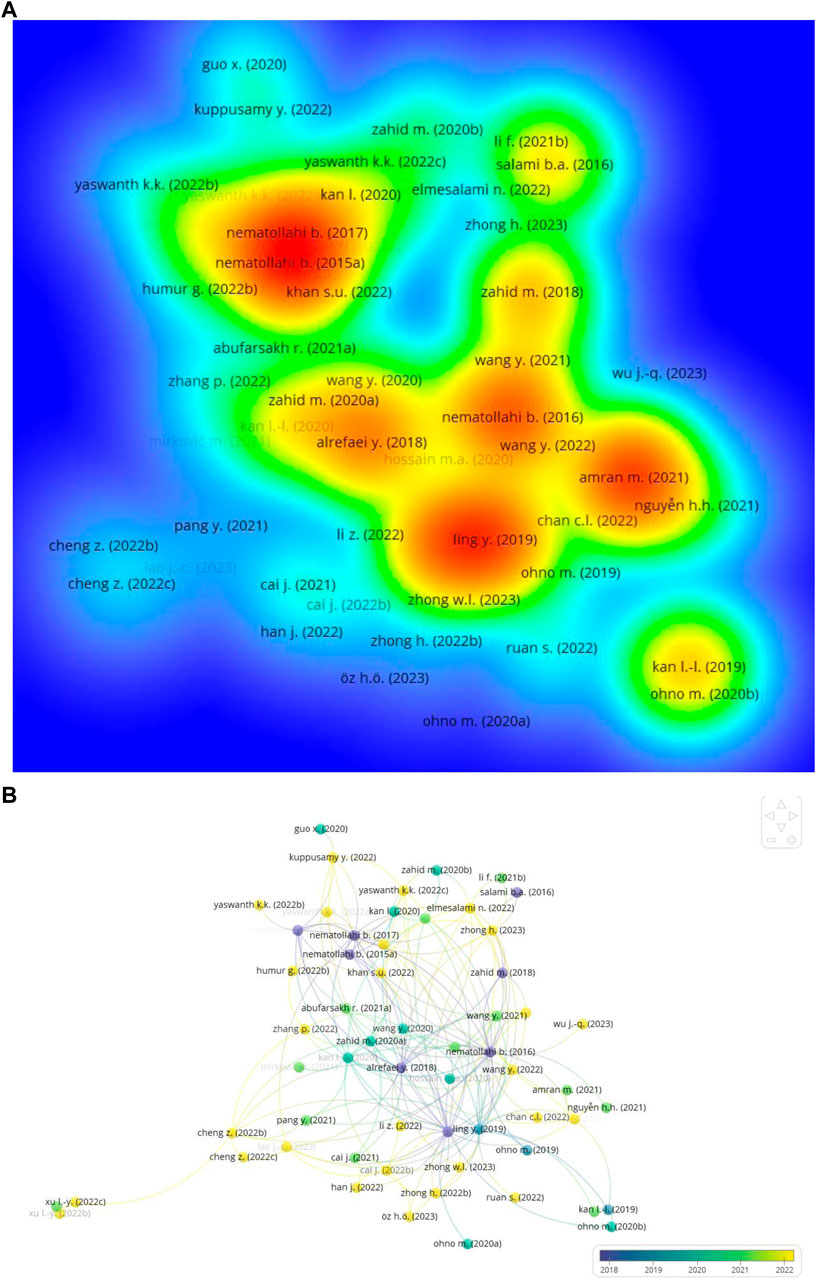
FIGURE 9. Scientific mapping of documents: (A) density of connected articles based on citation density, (B) increasing trend of research articles by authors.
4.6 Countries
Systematic bibliographic mapping is conducted to identify the countries with the most significant contributions to research on EGC to predict the characteristics of this research area. The United States, Australia, and Hong Kong are the leading countries. The United States of America received the maximum citations (i.e., 551), followed by Australia (i.e., 476) and Hong Kong (i.e., 244). Moreover, a visual representation of the interconnectivity between different countries in terms of citations and density concentration is also presented in Figure 10. Figure 10A shows the impact of each country in the research field, with the size of the circle representing the magnitude of the impact. Additionally, Figure 10B indicates that countries with more publications have a higher density. These statistical and graphical outputs benefit young researchers interested in collaborating and exchanging innovative concepts/techniques with professionals in related fields. This information also facilitates the formation of teams with diverse expertise from various countries interested in working on EGC.
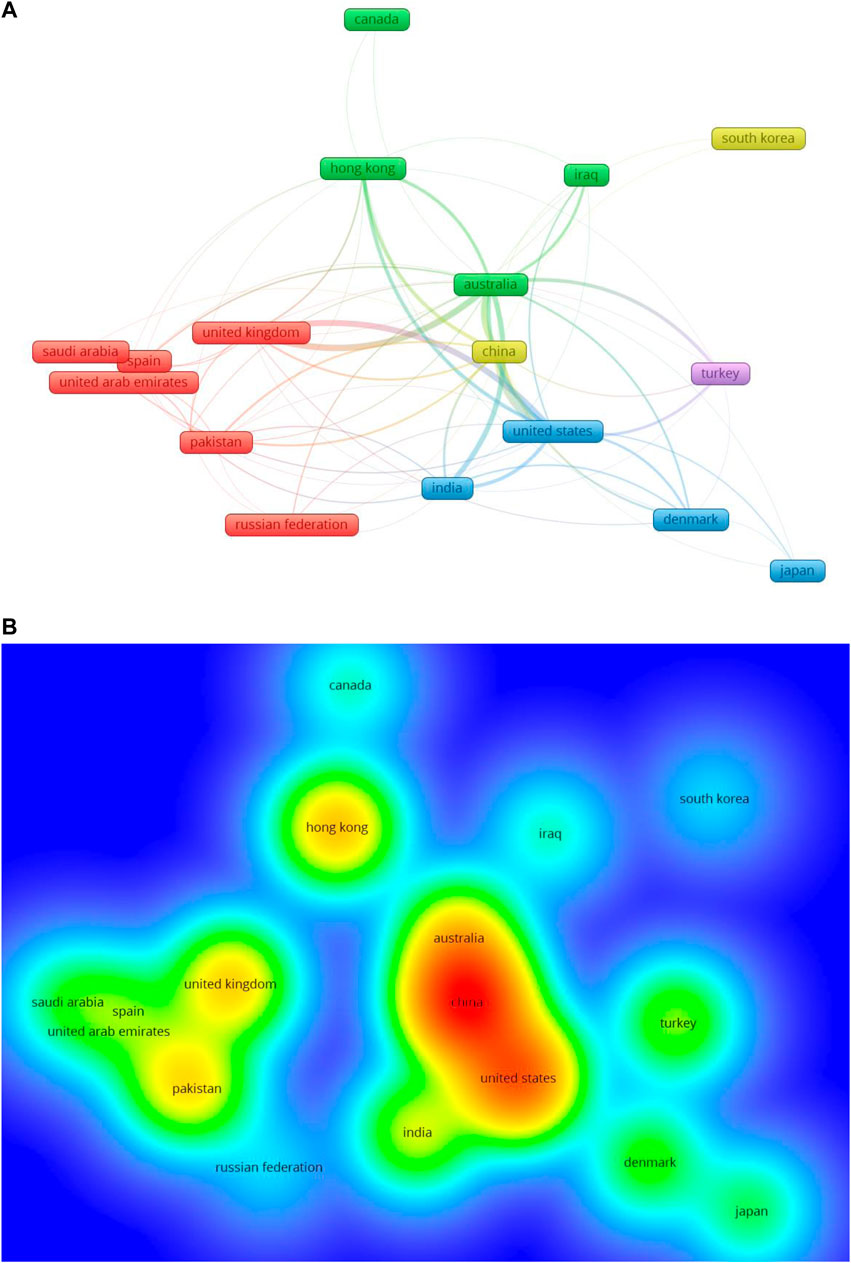
FIGURE 10. The scientific visualization of countries within the research domain (A) as a network visualization and (B) as a density visualization.
5 EGC manufacturing
Unlike conventional concrete that uses cement, geopolymer employs mineral admixture (alkali-activated) as a binder to create a compact mass with an inert aggregate. Geopolymer offers various advantages over regular concrete, such as high early strength, resistance to high temperatures, and good chemical resistance in harsh environments. Engineered geopolymer composites (EGC) is chosen for several reasons, such as using sustainable construction materials to preserve the environment. This is due to the global concern over the use of Portland cement-based composites. Over the last two decades, extensive research has been conducted on various EGC properties, resulting in significant experimental test data. Comprehending the mechanical properties of EGC is a crucial step toward manufacturing significant EGC amounts having predictable characteristics. According to reviewed literature, researchers have conducted extensive studies on the properties of geopolymers over the past 20 years and found several techniques available for producing geopolymers with varying properties. Various properties of geopolymer paste (Phoo-ngernkham et al., 2013; Adak et al., 2014), mortar (Adak et al., 2014), and concrete (Hardjito et al., 2005) have been studied experimentally. Based on concrete density, EGC has two types: light and normal weight. The lightweight geopolymer concrete can be in foamed concrete (Al Bakri Abdullah et al., 2012) or other variations utilizing lightweight aggregate (Posi et al., 2013). Various curing methods were explored by researchers for EGC, such as oven heating, membrane curing, steam curing, water curing, hydrothermal curing, and room temperature. Of these methods, the most effective one is oven curing (Sofi et al., 2007a). The schematic diagram for the manufacturing of EGC is shown in Figure 11, as illustrated by Wang et al. (2023).
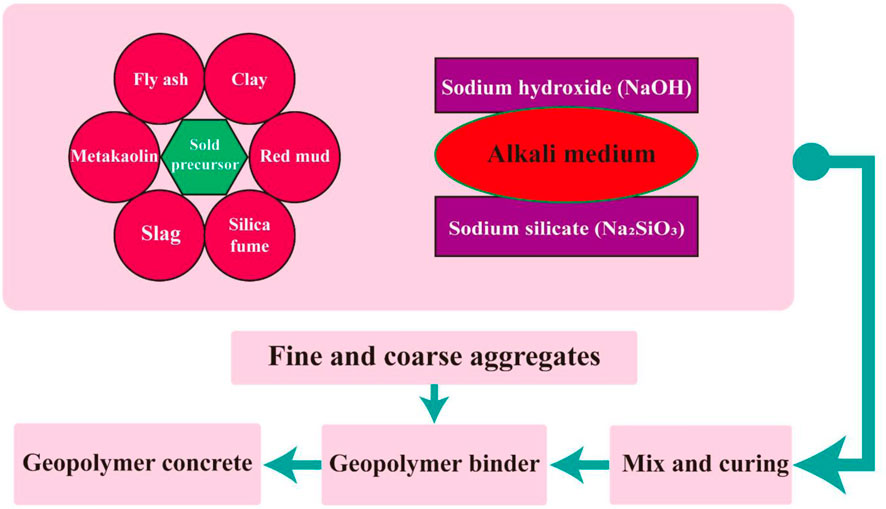
FIGURE 11. The manufacturing process of geopolymer concrete (Wang et al., 2023).
6 Microstructural analysis of EGC
The difference in the micro-structure of conventional concrete and EGC has been depicted by Alanazi (2022), as shown in Figure 12. The geopolymer mixture SEM image (Figure 12B) displays a complex matrix microstructure of multiple phases. Consequently, these phases’ unique properties and interactions are expected to influence geopolymer concrete’s overall physical and mechanical characteristics significantly. Further discussion on this matter will be provided later. Regarding the geometric characteristics of the interfacial transition zone (ITZ), the microstructures adjacent to the larger limestone aggregate demonstrate no discernible variations compared to the matrix phase microstructure, suggesting the absence of visible voids surrounding the limestone particles. In contrast, the SEM image for the microstructure of the OPC mixture reveals the presence of significant voids or pores surrounding the ITZ, as depicted in Figure 12A. Other researchers have also reported similar findings (Mondal et al., 2009; Zhang et al., 2009; Khedmati et al., 2018). The magnified images in Figure 13 provide evidence of the bonding phenomenon occurring at the ITZ between aggregates and the geopolymer matrix. Notably, the presence of microcracks visible in the images, though their confirmation is still pending, might be ascribed to additional stress encountered during the polishing (surface smoothing) operation or the cementitious shrinkage transpiring during the curing process. Incorporating a low-density interfacial transition zone would harm the overall properties of ordinary Portland cement mixtures, decreasing their strength. The weakness observed in the ITZ of OPC mixtures is often linked to calcium hydroxide crystals and the accumulation of pores at the ITZ, which occur due to excess water at the aggregate surface (Zhang et al., 2009). These factors contribute to a compromised ITZ structure, reducing the overall performance of the OPC mixture. However, in the case of geopolymer paste and its associated ITZ, the absence of such calcium hydroxide crystals indicates a more favorable microstructural condition, potentially resulting in improved properties compared to OPC mixtures.
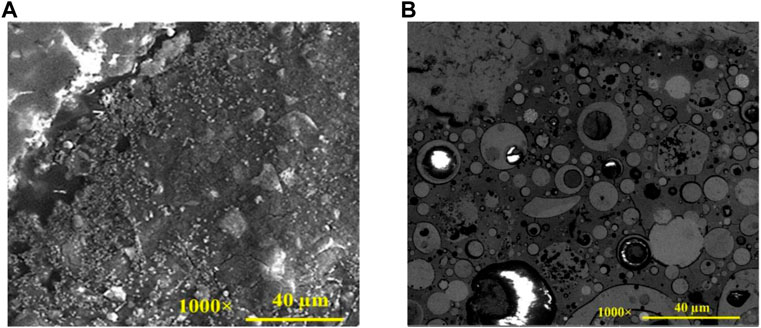
FIGURE 12. SEM images (A) conventional concrete (B) EGC (Alanazi, 2022).
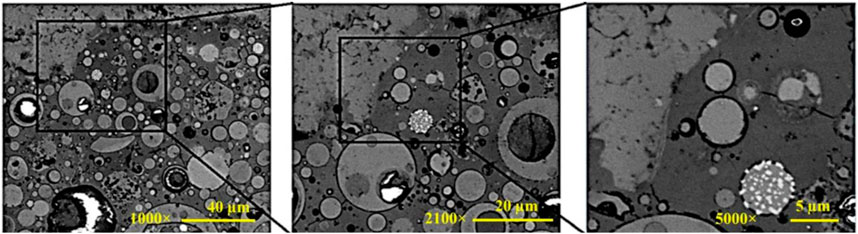
FIGURE 13. SEM magnified images of EGC mixture (Alanazi, 2022).
7 Mechanical properties of EGC
7.1 Compressive strength of EGC
The bond strength, compressive strength, flexural strength, and splitting tensile strength were reported to be uppermost using fly ash as a 50% replacement of OPC (Shehab et al., 2016). According to Lloyd and Rangan (2009), adding 24 h before curing can enhance the compressive strength of EGC. Concrete cured under ambient conditions may exhibit weak early strength; however, a considerable increase in strength can be achieved through high-temperature curing. One important factor that affects the geo-polymerization mechanism and strength of concrete is curing time. Studies have shown that longer curing times can improve strength, but excessively long durations at high temperatures can cause failure (Nurruddin et al., 2018). Researchers have also found that higher initial curing temperatures and longer durations generally lead to higher compressive strength (Hardjito et al., 2005; Jindal et al., 2017; Hassan et al., 2019). Joseph and Mathew (2012) identified 100°C as an appropriate temperature, and Chindaprasirt et al. (2007) observed an optimum curing time of 3 h at 60°C, with the ideal curing temperature being 75°C. These researchers concluded that the initial heat-curing process is crucial for strength development. Other researchers also reported similar observations (Abdullah et al., 2011; Vijai et al., 2011; Almuhsin et al., 2018). It should be emphasized that in each of these studies, maximum strength was attained, and no additional strength was observed beyond 7 days, indicating completion of the reaction. According to a study conducted by Dave et al. (2020), the strength of geopolymer concrete increases over time, a trend also observed in Portland cement, as shown in Figure 14. The authors attribute this compressive strength to the precursors’ persistent polymerization and condensation.
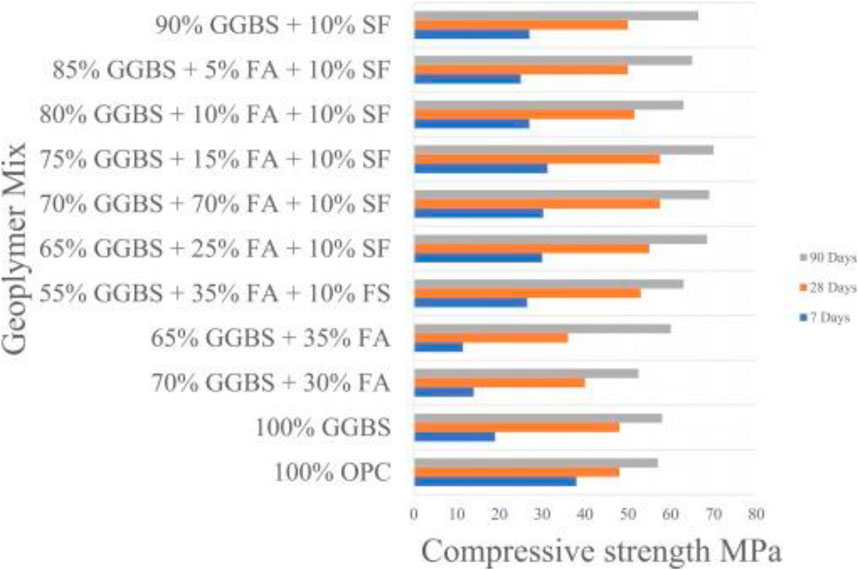
FIGURE 14. Compressive strength of EGC (Dave et al., 2020).
7.2 Elastic modulus of EGC
EGC elastic modulus generally exhibits a similar trend to its compressive strength. Hardjito et al. (2005) reported a correlation between the compressive strength of EGC and its modulus of elasticity; increasing the former can increase the latter. Mohammed et al. (2021) developed the relationship to show the variation between the compressive strength of EGC and its modulus of elasticity, as shown in Figure 15. In contrast, Nath and Sarker (2017) observed that the curing regime does not significantly impact the elastic modulus of EGC. In contrast, Saravanan and Elavenil (2018) reported a significant increase in the elastic modulus upon replacing fly ash with GGBS up to 50%, even though the compressive strength may not be correspondingly affected. Poisson’s EGC ratio has received relatively little attention compared to other properties, and limited test data is available. However, the limited data suggest that Poisson’s ratio of GPC typically falls within the range of 0.23–0.26 (Sofi et al., 2007b). In contrast, some researchers have reported lower values of Poisson’s ratio for different types of EGCs (Abdullah et al., 2012; Olivia and Nikraz, 2012; Albitar et al., 2015). The behavior of Poisson’s ratio in EGC appears to depend on the material’s compressive strength. For instance, some researchers have observed a reduction in Poisson’s ratio as the compressive strength decreases (Abdullah et al., 2012). Additionally, some examinations have shown that increasing fly ash replacement with GGBS can decrease Poisson’s ratio, in contrast to other properties of EGC (Sivakumar and Kishore, 2017). In summary, the correlation between the compressive strength and the modulus of elasticity of EGC has been reported by different researchers. The impact of the curing regime on the elastic modulus of EGC is debatable.
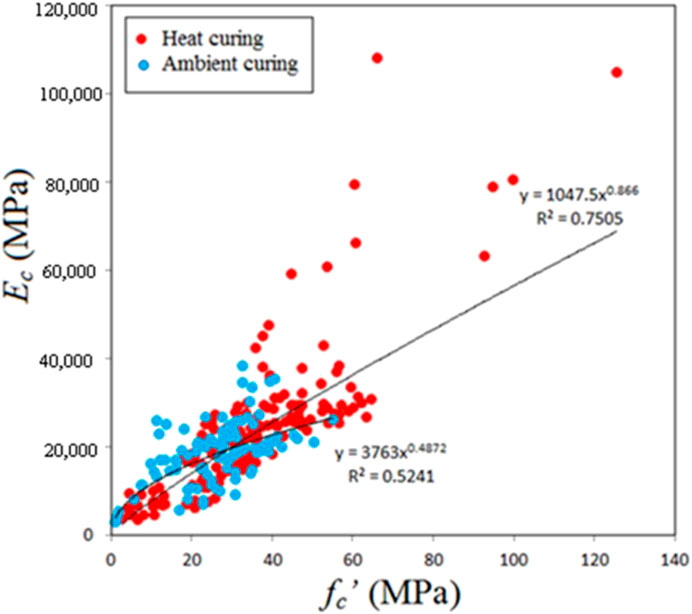
FIGURE 15. Elastic modulus variation in relation to compressive strength (Mohammed et al., 2021).
7.3 Flexural and tensile strength of EGC
The flexural strength of concrete refers to its capacity to withstand loads that cause bending or flexure. According to Wilkinson et al. (2016), concrete’s 28-day flexural strength results were generally around 4% of its corresponding 28-day compressive strengths. Studies indicate that the compressive strength of EGC has a similar impact on the indirect tensile strength and flexural strength (Raijiwala and Patil, 2010; Rashad, 2013). Typically, an increase in compressive strength leads to improvements in both splitting tensile and flexural strengths of the EGC. Wang et al. (2023) reported comparing mechanical properties (i.e., compressive, splitting-tensile, and flexural strengths) of conventional concrete and EGC, as shown in Figure 16. The splitting tensile strength of EGC is significantly lower than its compressive strength (Hardjito et al., 2005). However, researchers have reported certain deviations from this trend. Ryu et al. (2013) found that the rate of increase in tensile strength decreases as compressive strength increases. Moreover, the substitution of GGBS for fly ash has a comparatively lesser impact on splitting tensile and flexural strengths when compared to compressive strength (Abhilash et al., 2016). Furthermore, Yousefi Oderji et al. (2019) discovered that replacing fly ash with slag at 15%–20% reduced flexural strength despite increased compressive strength due to the modification. Partha et al. (2013) reported that utilizing a specialized heat curing technique increased the flexure/compression ratio and a minor rise in the tensile/compression ratio compared to curing at ambient temperature. Consequently, the studies that have been reported did not reveal any discernible relationship between the 28-day compressive strength and the 28-day flexural strength. However, like with compressive strength, research conducted by Dave et al. (2020) demonstrated that combining GGBS and SF with FA as precursors improved the flexural strength of geopolymer mixes (Figure 17). The geopolymer mix comprised 70% GGBS, 20% FA, and 10% SF as precursors demonstrated the highest level of flexural strength.
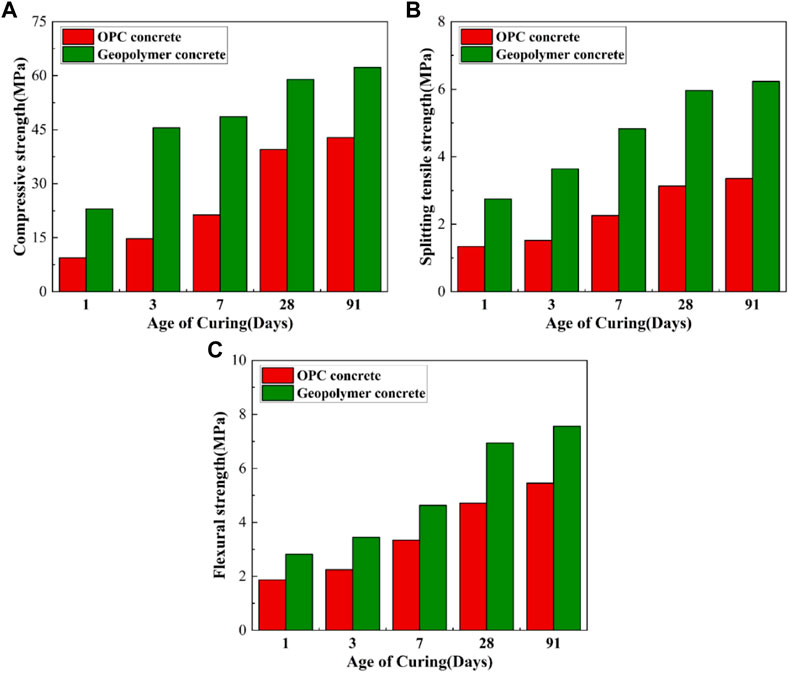
FIGURE 16. Comparison of (A) compressive strength, (B) splitting-tensile, and (C) flexural strength of conventional concrete and EGC (Wang et al., 2023).
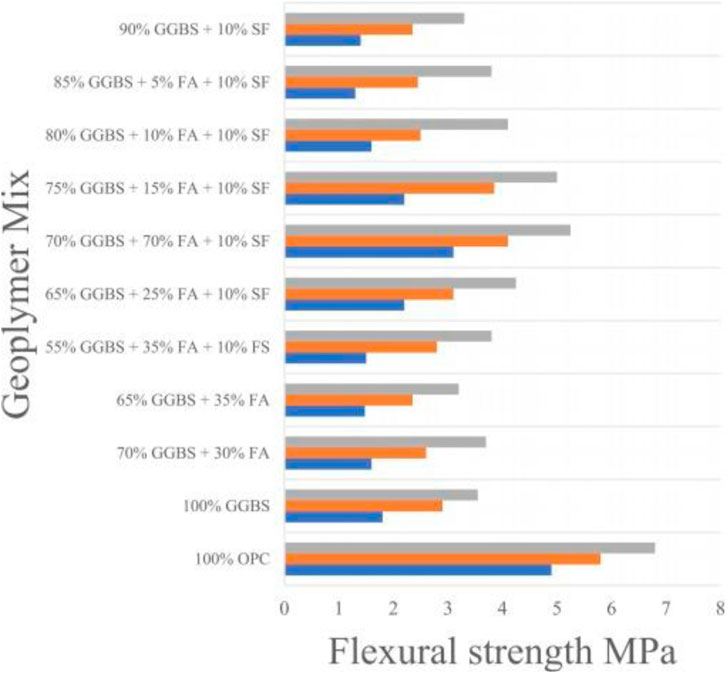
FIGURE 17. Flexural strength of EGC (Dave et al., 2020).
8 Durability of EGC
The durability of EGCs plays a vital in ensuring their long-term performance and reliability. However, specific standards for evaluating their durability are scarce due to the relatively recent emergence of geopolymer composites in cementitious materials. When assessing the durability of EGCs, similar to the standards used for conventional concrete evaluation (such as ASTM, AASHTO, and ACI), emphasis is placed on evaluating their transport properties, resistance to physical degradation, and chemical resistance (Li et al., 2021). Transport properties characterize the resistance of composites against the ingress of water, gas, and ions through cracks, pores, and air voids. These properties can be improved by incorporating appropriately dispersed nanomaterials in optimal quantities. Among these transport properties, water absorption holds particular significance as it directly influences the vulnerability of EGCs to various durability concerns. EGCs with lower water absorption demonstrate reduced susceptibility to multiple forms of degradation, given that water substantially contributes to deterioration mechanisms such as freeze/thaw damage, sulfate attack, alkali-aggregate reactions, carbonation, and rebar corrosion (Adak et al., 2015; Assaedi et al., 2016; Li and Shi, 2020). Water absorption in a material is affected by the transmission pathways and the characteristics of the contact surface. Hydrophilic materials generally demonstrate higher water absorption when evaluating materials with similar pore structures than hydrophobic materials (Li and Shi, 2020).
As far as the physical degradation is concerned, it typically includes abrasion (or erosion), weathering due to wet/dry cycling, and damage caused by freeze/thaw cycles. Understanding the fundamental mechanisms underlying physical damage in cementitious composites is an active area of ongoing research. However, it is widely agreed that the resistance to such cyclic degradations depends not only on the mechanical strength of the composite matrix but also on the characteristics of its pore structure. In the case of EGC, it is plausible to propose that the fluctuating temperatures may trigger the crystallization of temperature-sensitive phases found in fly ash, activators, and other additives. This crystallization process could generate pressure, potentially leading to the physical degradation of the EGC matrix. Additionally, it is conceivable that the alternating temperature conditions may cause the leaching of chemical ions, resulting in localized chemical attacks within the EGC (Xu and Shi, 2018; Xu and Shi, 2020). Moreover, EGCs in service conditions can encounter simultaneous deterioration from various sources, making them prone to physical and chemical assaults. Chemical degradation commonly observed in cementitious composites encompasses carbonation, sulfate attack, acid attack, and alkali-aggregate reactions. Conversely, failure mechanisms such as salt scaling and chloride-induced rebar corrosion entail combined chemical and physical deterioration processes (Ren et al., 2019; Xu and Shi, 2020). The formation process of geopolymers containing impurities, such as silico-aluminophosphate and alkali-aluminosilicate geopolymer, can be represented by considering the acidity and alkalinity activation conditions. These conditions are illustrated in Figure 18, as reported by Wang et al. (2019), showcasing the geopolymers’ step-by-step formation process. The presence of specific constituents in the precursor materials, referred to as “impurities,” which differ from aluminosilicates, can initiate reactions that deviate from the typical geo-polymeric processes. Consequently, when acidic or alkaline activators are utilized, distinct chemical compositions, levels of crystallinity, and phases are generated. Furthermore, beyond variations in pH conditions, significant disparities arise in the molecular structures of geopolymers, particularly in the chemical environment surrounding aluminum within the formed gels (Pacheco-Torgal et al., 2008; Yao et al., 2015; Wang et al., 2019).
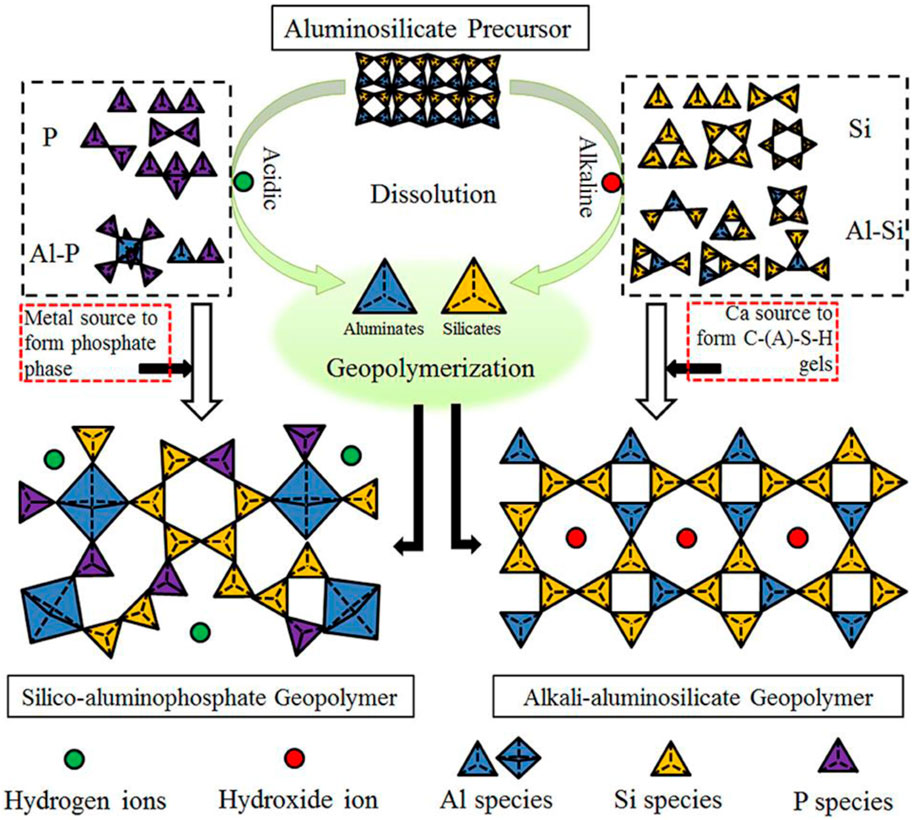
FIGURE 18. Process of activating aluminosilicate sources under acidic and alkaline conditions (Wang et al., 2019).
9 Applications of EGC
Geopolymer concrete, which contains 70%–80% aggregate by mass and plasticizers, is manufactured using standard concrete production methods (Almutairi et al., 2021). Since then, researchers have conducted comprehensive studies on geopolymer concrete’s strength and durability characteristics. The pre-cast application of geopolymer technology is considered highly advanced, primarily due to its ability to handle delicate materials (Aleem and Arumairaj, 2012; Almutairi et al., 2021). Historically, EGC has been mainly used for sewer pipes and railway sleepers (Sharma et al., 2017; Gourley and Johnson, 2019). Nevertheless, it is now feasible to produce structural components such as beams, columns, and tunnel segments using geopolymer concrete (Farhan et al., 2018; Hassan et al., 2020). According to Almutairi et al. (2021), geopolymer concrete represents an excellent alternative to conventional concrete, even for the scenarios in which steel rebars are embedded as reinforcement. Geopolymer concrete has been shown to meet specifications in extreme environments, such as sulfate soils. Therefore, it can serve as a sustainable substitute for producing durable structures. EGC exhibits significant chloride resistance, which translates to minimal mutilation during winter especially upon application of salt for dissolving ice. The outstanding capability of EGC to resist chloride corrosion makes it a viable option for utilization in concrete structures exposed to saltwater environments, such as coastal bridges, underwater concrete supports, and piers (Zhao et al., 2020; Churata et al., 2022; Tanu and Unnikrishnan, 2023). According to Yang et al. (2008), geopolymer concrete manufactured using slag can attain strength if cured at room temperature. Numerous types of research have demonstrated that EGC may serve as highway infrastructure repair material (Yun and Choi, 2014). Due to the exceptional performance observed in its initial applications, efforts have been made to integrate EGC into respective authority specifications (Dave et al., 2020). According to Tayeh et al. (2020), highway pavement applications represent a specialized area where geopolymer concrete could bring about a significant transformation. Geopolymer concrete has emerged as a sustainable alternative to Portland cement concrete for various applications due to its remarkable durability and resistance to harsh environments, such as sulfate soils and chloride corrosion. Originally used for sewer pipes and railway sleepers, geopolymer concrete has now been extended to include structural elements such as beams and columns and can also serve as a repair material for highway infrastructure. Thus, geopolymer concrete has the potential to transform highway pavement applications. Figure 19 illustrates the summary of EGC applications, as mentioned in the above discussion.
10 Sustainability aspect of EGC
The concrete industry is looking for sustainable alternatives to Portland cement, and one promising option is fly ash-based geopolymer concrete. Unlike traditional concrete, geopolymer concrete uses fly ash from coal-burning power stations as the binder, eliminating the need for Portland cement. Using fly ash-based geopolymer concrete has the potential to reduce global warming, as research has shown that the global warming potential of geopolymer concrete is between 26% and 45% lower compared to ordinary Portland cement concrete. Studies have shown that replacing traditional Portland cement concrete with geopolymer concrete, depending on the specific precursor and activator used, can lead to a reduction in embodied carbon of up to 80% (Tayeh et al., 2021). However, other ecological impact factors need to be considered as well. Life cycle assessment of geopolymers reveals that, compared to OPC concrete, geopolymer concrete has a reduced impact on global warming (Garces et al., 2021). Additional LCAs of geopolymers have also suggested that these materials can serve as sustainable substitutes. Further gains in sustainability could be realized by adopting alternative activators and precursors that are readily available locally (Salas et al., 2018; Dal Pozzo et al., 2019; Bumanis et al., 2020). Therefore, the concrete industry must explore alternative methods to make geopolymer concrete more environmentally friendly while maintaining its benefits as a sustainable alternative to traditional concrete. Geopolymer concrete is often produced using waste materials from industrial and agricultural processes as precursors. This makes it an eco-friendly option for managing bulk waste generated by several industries (Juenger et al., 2011; Palomo et al., 2015; Mehta and Siddique, 2016). Furthermore, the geopolymers’ sustainability can be enhanced using laterite soil as precursors. Therefore, using geopolymers as a replacement for Portland cement composites can substantially decrease greenhouse gas emissions, utilization of raw materials, and efficient waste management (Davidovits, 2008; Yang et al., 2013). Therefore, it can be concluded that geopolymer binders without using cement are a highly promising eco-friendly construction material. Figure 20 (Mishra et al., 2022) shows that these geopolymer composites can be produced using industrial wastes, promoting sustainable development and benefiting the environment.
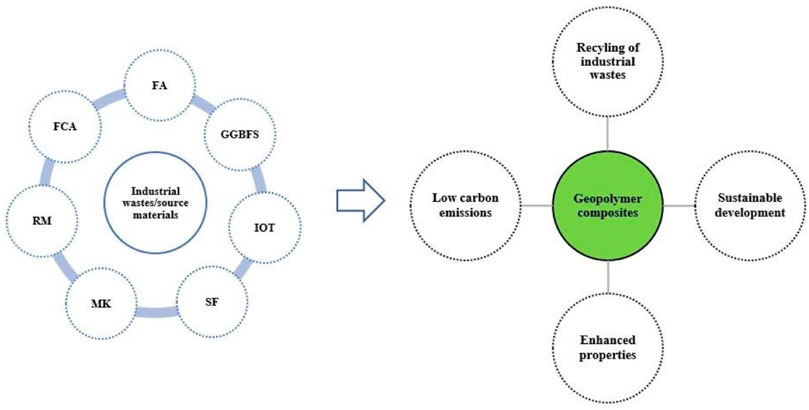
FIGURE 20. EGC—A way to sustainable development (Mishra et al., 2022).
11 Conclusion
This comprehensive bibliographic investigation thoroughly explores the dynamic and evolving domain of Engineered Geopolymer Composites (EGCs). By conducting an in-depth analysis of pertinent literature, numerous significant themes and trends have emerged, illuminating the present understanding of this specialized field. The review has yielded valuable insights into the manifold applications, properties, and challenges linked to EGCs, underscoring their capacity to bring about revolutionary changes across diverse industries. The scientific community has proposed bibliometric metrics that use statistical analysis techniques, such as scientometric analysis, to evaluate publications based on scientific conclusions. Such a review-based study can identify trends in the literature on EGC that benefit young scholars and researchers. The conclusions drawn are as follows:
• Many manufacturing methodologies have been devised to attain specific properties in geopolymers, with investigations encompassing geopolymer paste, mortar, and concrete. EGC can be classified into either lightweight or normal weight categories, including variants such as foamed concrete and the incorporation of lightweight aggregates. Extensive research has been dedicated to exploring various curing techniques, among which oven curing has emerged as the most efficient approach.
• The findings demonstrate that substituting 50% of OPC with fly ash results in improved bond strength, compressive strength, flexural strength, and splitting tensile strength in geopolymer concrete. The elastic modulus of EGC typically aligns with the pattern observed in compressive strength, with the latter influencing tensile and flexural strengths, thereby yielding enhancements in both aspects. Moreover, geopolymer concrete exhibits a continuous enhancement in strength over time, attributed to the precursors’ ongoing polymerization and condensation processes.
• Water absorption is pivotal in assessing the durability of EGCs, as it significantly influences their susceptibility to various deterioration mechanisms, including freeze/thaw damage, sulfate attack, alkali-aggregate reactions, carbonation, and rebar corrosion. Geopolymers that incorporate impurities undergo distinctive formation processes, resulting in variances in chemical compositions, crystallinity, phases, molecular structures, and the chemical milieu encompassing aluminum within the generated gel matrices.
• In pre-cast applications, such as structural components like beams and columns, geopolymer concrete demonstrates exceptional performance. It complies with requirements even in challenging environments, offers resistance to chloride exposure in saltwater conditions, and shows promise as a material for repairing highway infrastructure. Its durability and resilience in harsh conditions position it as an environmentally sustainable substitute for Portland cement concrete, with the potential to revolutionize various applications, including highway pavement, multi-layer wall construction, marine concrete coatings, and the production of geopolymer tiles.
• Geopolymer concrete presents a sustainable substitute for Portland cement, contributing to mitigating global warming potential and reducing embodied carbon. The production of waste materials in its manufacturing process facilitates effective waste management practices, and exploring alternative activators and precursors can further enhance its eco-friendliness. Geopolymer binders, devoid of traditional cement, hold significant promise as environmentally conscious construction materials, fostering sustainable development and yielding environmental benefits.
• EGCs have garnered widespread adoption in construction and infrastructure due to their impressive mechanical characteristics, exceptional durability, and heightened resilience in demanding environmental settings. Notably, their ability to reduce carbon emissions compared to traditional cement-based materials makes EGCs an attractive and sustainable choice for advancing infrastructure projects.
• A systematic examination of the existing literature reveals a limitation in the number of studies addressing the properties of EGC, highlighting the need for additional research efforts. To facilitate the broader adoption of EGC, several areas warrant further exploration, encompassing the assessment of how different alkali-activated mineral admixtures affect EGC, the utilization of diverse industrial byproducts, refinement of mix designs and curing techniques, the investigation of long-term performance and durability, exploration of EGC’s applicability in substantial infrastructure projects, and the evaluation of its environmental and economic ramifications.
12 Future research prospects
Engineered Geopolymer Composites (EGCs) represent a rapidly advancing and burgeoning area of research with substantial intellectual significance and far-reaching implications. However, there is a distinct need for additional foundational investigations to comprehensively explore and exploit the full potential of this environmentally sustainable cementitious composite. Furthermore, the current study highlights a lack of precise connections between different aspects of literature in conventional research studies on EGC. To address this issue, the authors conducted a systematic review that maps bibliographic data and provides statistical analysis. The review identifies frequently used keywords, the countries and sources contributing the most relevant articles, and the most credible authors in the EGC research domain. In the current study, an assessment of keywords shows that EGC has been studied as a sustainable and durable material. The analysis of highly contributing countries in the research domain is based on citation-based linkages in the literature. This analysis could help researchers to collaborate and advance research in this field. The study also employs scientometric analysis to evaluate keywords and interlinked literature, highlighting future perspectives in EGC research. Based on the current literature, it has been established that geopolymer concrete holds significant potential for a range of construction applications. However, additional research is required to address the following areas and facilitate greater utilization of geopolymers:
• A detailed investigation should be made to explore the impact of incorporating different alkali-activated mineral admixtures on geopolymer concrete properties, like compressive, splitting-tensile, and flexural strengths.
• The possibility of using different industrial byproducts, like rice husk ash, blast furnace slag, and red mud, as raw materials in the manufacturing of geopolymer concrete should also be explored.
• Developing novel mix designs and curing methods should also optimize EGC properties, like durability, strength development, and shrinkage behavior.
• Despite its longstanding use, further comprehensive and extensive studies are required to investigate the long-term performance, durability, and utilization of unconventional precursors in EGC. The emphasis must be placed on examining EGC’s long-term performance. Various accelerated tests could also be implemented to assess EGC’s long-term performance.
• Further exploration of using EGC in large-scale infrastructure projects, such as bridges, high-rise buildings, and tunnels, and comparing its cost-effectiveness with conventional concrete is also recommended.
• It is recommended to investigate the capacity of nanomaterials, like nanoparticles and nanofibers, to improve the characteristics of geopolymer concrete, encompassing attributes such as strength, longevity, and resilience to environmental influences.
• The studies should also be conducted to explore substitute activators for sodium or potassium hydroxide, such as alkali carbonates or silicates, to diminish the environmental footprint and cost associated with geopolymer manufacturing.
• Studies should also be conducted on integrating diverse waste materials like fly ash, slag, industrial by-products, and recycled aggregates into geopolymer concrete to enhance sustainability and reduce carbon footprint.
• To unlock future research potential, it is crucial to establish a robust link between MK-based geopolymer concretes and their impact on key properties like compressive, tensile, and flexural strength. A proposed connection between tensile and flexural strength with compressive strength would be valuable to the engineering community. This requires detailed investigation and experimentation to derive a dependable relationship. Such progress will promote wider adoption of MK-based geopolymer concrete, enhancing sustainability and concrete structure performance in construction projects.
• Assessing the environmental and economic implications of using EGC would enhance its awareness and promote its application. Such a comprehensive evaluation would provide valuable insights into numerous advanced approaches that may be employed to decrease adverse impacts on the environment and costs of EGC.
Author contributions
YZ: Conceptualization, Data curation, Funding acquisition, Methodology, Software, Writing–original draft, Visualization. HL: Conceptualization, Methodology, Visualization, Validation, Writing–review and editing. YG: Conceptualization, Methodology, Writing–review and editing, Data curation, Funding acquisition, Software, Writing–original draft. BI: Methodology, Supervision, Validation, Writing–review and editing. HM: Methodology, Supervision, Validation, Writing–review and editing.
Funding
The authors declare financial support was received for the research, authorship, and/or publication of this article. This work was sponsored in part by Technology research and development projects of China Construction Sixth Engineering Bureau Co., Ltd. (CSCEC6B-2022-Z-16). The funder was not involved in the study design, collection, analysis, interpretation of data, the writing of this article, or the decision to submit it for publication.
Acknowledgments
The authors would like to acknowledge Technology research and development projects of China Construction Sixth Engineering Bureau Co., Ltd. (CSCEC6B-2022-Z-16).
Conflict of interest
Author YZ was employed by China Construction Sixth Engineering Bureau Co., Ltd. Authors YZ and HL were employed by China Construction Sixth Engineering Bureau Co., Ltd.
The remaining authors declare that the research was conducted in the absence of any commercial or financial relationships that could be construed as a potential conflict of interest.
Publisher’s note
All claims expressed in this article are solely those of the authors and do not necessarily represent those of their affiliated organizations, or those of the publisher, the editors and the reviewers. Any product that may be evaluated in this article, or claim that may be made by its manufacturer, is not guaranteed or endorsed by the publisher.
References
Abdullah, M. M. A. B., Hussin, K., Bnhussain, M., Ismail, K. N., Yahya, Z., and Abdul Razak, R. (2012). Fly ash-based geopolymer lightweight concrete using foaming agent. Int. J. Mol. Sci. [Online] 13 (6), 7186–7198. doi:10.3390/ijms13067186
Abdullah, M. M. A. B., Kamarudin, H., Bnhussain, M., Ismail, K. N., Rafiza, A., and Zarina, Y. (2011). The relationship of NaOH molarity, Na<sub>2</sub>SiO<sub>3</sub>/NaOH ratio, fly ash/alkaline activator ratio, and curing temperature to the strength of fly ash-based geopolymer. Adv. Mater. Res. 328, 1475–1482. doi:10.4028/www.scientific.net/AMR.328-330.1475
Abhilash, P., Sashidhar, C., and Reddy, I. R. (2016). Strength properties of fly ash and GGBS based geo-polymer concrete. Int. J. ChemTech Res. 9 (3), 350–356. doi:10.34218/IJCIET.11.6.2020.012
Adak, D., Sarkar, M., Maiti, M., Tamang, A., Mandal, S., and Chattopadhyay, B. (2015). Anti-microbial efficiency of nano silver–silica modified geopolymer mortar for eco-friendly green construction technology. RSC Adv. 5 (79), 64037–64045. doi:10.1039/c5ra12776a
Adak, D., Sarkar, M., and Mandal, S. (2014). Effect of nano-silica on strength and durability of fly ash based geopolymer mortar. Constr. Build. Mater. 70, 453–459. doi:10.1016/j.conbuildmat.2014.07.093
Adamiec, P., Benezet, J.-C., and Benhassaine, A. (2008). Pozzolanic reactivity of silico-aluminous fly ash. Particuology 6 (2), 93–98. doi:10.1016/j.partic.2007.09.003
Afgan, S., and Bing, C. (2021). Scientometric review of international research trends on thermal energy storage cement based composites via integration of phase change materials from 1993 to 2020. Constr. Build. Mater. 278, 122344. doi:10.1016/j.conbuildmat.2021.122344
Ahmad, M. R., Das, C. S., Khan, M., and Dai, J.-G. (2023). Development of low-carbon alkali-activated materials solely activated by flue gas residues (FGR) waste from incineration plants. J. Clean. Prod. 397, 136597. doi:10.1016/j.jclepro.2023.136597
Ahmad, W., Khan, M., and Smarzewski, P. (2021). Effect of short fiber reinforcements on fracture performance of cement-based materials: A systematic review approach. Materials 14 (7), 1745. doi:10.3390/ma14071745
Al Bakri Abdullah, M. M., Hussin, K., Bnhussain, M., Ismail, K. N., Yahya, Z., and Razak, R. A. (2012). Fly ash-based geopolymer lightweight concrete using foaming agent. Int. J. Mol. Sci. 13 (6), 7186–7198. doi:10.3390/ijms13067186
Alanazi, H. (2022). Study of the interfacial transition zone characteristics of geopolymer and conventional concretes. Gels [Online] 8 (2), 105. doi:10.3390/gels8020105
Albitar, M., Visintin, P., Mohamed Ali, M. S., and Drechsler, M. (2015). Assessing behaviour of fresh and hardened geopolymer concrete mixed with class-F fly ash. KSCE J. Civ. Eng. 19 (5), 1445–1455. doi:10.1007/s12205-014-1254-z
Aleem, M. A., and Arumairaj, P. (2012). Geopolymer concrete–a review. Int. J. Eng. Sci. Emerg. Technol. 1 (2), 118–122. doi:10.7323/ijeset/v1_i2_14
Almuhsin, B., al-Attar, T., and Hasan, Q. (2018). Effect of discontinuous curing and ambient temperature on the compressive strength development of fly ash based Geopolymer concrete. Build. Mater. Eng. Constr. Manag. 162, 02026. doi:10.1051/matecconf/201816202026
Almutairi, A. L., Tayeh, B. A., Adesina, A., Isleem, H. F., and Zeyad, A. M. (2021). Potential applications of geopolymer concrete in construction: A review. Case Stud. Constr. Mater. 15, e00733. doi:10.1016/j.cscm.2021.e00733
Amin, M. N., Ahmad, W., Khan, K., and Sayed, M. M. (2022). Mapping research knowledge on rice husk ash application in concrete: A scientometric review. Materials 15 (10), 3431. doi:10.3390/ma15103431
Assaedi, H., Shaikh, F., and Low, I. M. (2016). Influence of mixing methods of nano silica on the microstructural and mechanical properties of flax fabric reinforced geopolymer composites. Constr. Build. Mater. 123, 541–552. doi:10.1016/j.conbuildmat.2016.07.049
Atiş, C. D., Bilim, C., Çelik, Ö., and Karahan, O. (2009). Influence of activator on the strength and drying shrinkage of alkali-activated slag mortar. Constr. Build. Mater. 23 (1), 548–555. doi:10.1016/j.conbuildmat.2007.10.011
Bakharev, T., Sanjayan, J. G., and Cheng, Y.-B. (1999). Alkali activation of Australian slag cements. Cem. Concr. Res. 29 (1), 113–120. doi:10.1016/S0008-8846(98)00170-7
Bakharev, T., Sanjayan, J. G., and Cheng, Y. B. (2003). Resistance of alkali-activated slag concrete to acid attack. Cem. Concr. Res. 33 (10), 1607–1611. doi:10.1016/S0008-8846(03)00125-X
Buchwald, A., Dombrowski, K., and Weil, M. (2005). The influence of calcium content on the performance of geopolymeric binder especially the resistance against acids. Proc. world geopolymer, 35–39.
Bumanis, G., Vitola, L., Pundiene, I., Sinka, M., and Bajare, D. (2020). Gypsum, geopolymers, and starch—alternative binders for bio-based building materials: A review and life-cycle assessment. Sustainability 12 (14), 5666. doi:10.3390/su12145666
Cai, J., Pan, J., Han, J., Lin, Y., and Sheng, Z. (2021). Impact behaviours of engineered geopolymer composite exposed to elevated temperatures. Constr. Build. Mater. 312, 125421. doi:10.1016/j.conbuildmat.2021.125421
Cao, M., and Khan, M. (2021). Effectiveness of multiscale hybrid fiber reinforced cementitious composites under single degree of freedom hydraulic shaking table. Struct. Concr. 22 (1), 535–549. doi:10.1002/suco.201900228
Chindaprasirt, P., Chareerat, T., and Sirivivatnanon, V. (2007). Workability and strength of coarse high calcium fly ash geopolymer. Cem. Concr. Compos. 29 (3), 224–229. doi:10.1016/j.cemconcomp.2006.11.002
Churata, R., Almirón, J., Vargas, M., Tupayachy-Quispe, D., Torres-Almirón, J., Ortiz-Valdivia, Y., et al. (2022). Study of geopolymer composites based on volcanic ash, fly ash, pozzolan, metakaolin and mining tailing. Buildings 12 (8), 1118. doi:10.3390/buildings12081118
Dal Pozzo, A., Carabba, L., Bignozzi, M. C., and Tugnoli, A. (2019). Life cycle assessment of a geopolymer mixture for fireproofing applications. Int. J. Life Cycle Assess. 24, 1743–1757. doi:10.1007/s11367-019-01603-z
Darko, A., Zhang, C., and Chan, A. P. (2017). Drivers for green building: A review of empirical studies. Habitat Int. 60, 34–49. doi:10.1016/j.habitatint.2016.12.007
Dave, N., Sahu, V., and Misra, A. K. (2020). Development of geopolymer cement concrete for highway infrastructure applications. J. Eng. Des. Technol. 18 (5), 1321–1333. doi:10.1108/jedt-10-2019-0263
Davidovits, J. (2008). Geopolymer chemistry and applications, Saint Quentin, France: Institute Geopolymer.
Davidovits, J. (1991). Geopolymers: inorganic polymeric new materials. J. Therm. Analysis Calorim. 37 (8), 1633–1656. doi:10.1007/bf01912193
Duxson, P., Fernández-Jiménez, A., Provis, J. L., Lukey, G. C., Palomo, A., and van Deventer, J. S. J. (2007). Geopolymer technology: the current state of the art. J. Mater. Sci. 42 (9), 2917–2933. doi:10.1007/s10853-006-0637-z
Elchalakani, M., Dong, M., Karrech, A., Li, G., Mohamed Ali, M., Xie, T., et al. (2018). Development of fly ash-and slag-based geopolymer concrete with calcium carbonate or microsilica. J. Mater. Civ. Eng. 30 (12), 04018325. doi:10.1061/(asce)mt.1943-5533.0002527
Farhan, N. A., Sheikh, M. N., and Hadi, M. N. (2018). Experimental investigation on the effect of corrosion on the bond between reinforcing steel bars and fibre reinforced geopolymer concrete. Structures 14, 251–261. doi:10.1016/j.istruc.2018.03.013
Farooqi, M. U., and Ali, M. (2022). A study on natural fibre reinforced concrete from materials to structural applications. Arabian J. Sci. Eng. 48, 4471–4491. doi:10.1007/s13369-022-06977-1
Farooqi, M. U., and Ali, M. (2019). Effect of pre-treatment and content of wheat straw on energy absorption capability of concrete. Constr. Build. Mater. 224, 572–583. doi:10.1016/j.conbuildmat.2019.07.086
Garces, J. I. T., Dollente, I. J., Beltran, A. B., Tan, R. R., and Promentilla, M. A. B. (2021). Life cycle assessment of self-healing geopolymer concrete. Clean. Eng. Technol. 4, 100147. doi:10.1016/j.clet.2021.100147
Gourley, J., and Johnson, G. (2019). “Developments in geopolymer precast concrete,” in World congress geopolymer (France: Geopolymer Institute Saint-Quentin), 139–143.
Hardjito, D., Wallah, S. E., Sumajouw, D. M., and Rangan, B. V. (2005). Fly ash-based geopolymer concrete. Aust. J. Struct. Eng. 6 (1), 77–86. doi:10.1080/13287982.2005.11464946
Hassan, A., Arif, M., and Shariq, M. (2020). A review of properties and behaviour of reinforced geopolymer concrete structural elements-A clean technology option for sustainable development. J. Clean. Prod. 245, 118762. doi:10.1016/j.jclepro.2019.118762
Hassan, A., Arif, M., and Shariq, M. (2019). Effect of curing condition on the mechanical properties of fly ash-based geopolymer concrete. SN Appl. Sci. 1, 1694–1699. doi:10.1007/s42452-019-1774-8
Huang, S., Wang, H., Ahmad, W., Ahmad, A., Ivanovich Vatin, N., Mohamed, A. M., et al. (2022). Plastic waste management strategies and their environmental aspects: A scientometric analysis and comprehensive review. Int. J. Environ. Res. Public Health 19 (8), 4556. doi:10.3390/ijerph19084556
Ilcan, H., Sahin, O., Kul, A., Yildirim, G., and Sahmaran, M. (2022). Rheological properties and compressive strength of construction and demolition waste-based geopolymer mortars for 3D-Printing. Constr. Build. Mater. 328, 127114. doi:10.1016/j.conbuildmat.2022.127114
Jin, R., Gao, S., Cheshmehzangi, A., and Aboagye-Nimo, E. (2018). A holistic review of off-site construction literature published between 2008 and 2018. J. Clean. Prod. 202, 1202–1219. doi:10.1016/j.jclepro.2018.08.195
Jindal, B. B. (2019). Investigations on the properties of geopolymer mortar and concrete with mineral admixtures: A review. Constr. Build. Mater. 227, 116644. doi:10.1016/j.conbuildmat.2019.08.025
Jindal, B. B., ParveenSinghal, D., and Goyal, A. (2017). Predicting relationship between mechanical properties of low calcium fly ash-based geopolymer concrete. Trans. Indian Ceram. Soc. 76 (4), 258–265. doi:10.1080/0371750x.2017.1412837
Joseph, B., and Mathew, G. (2012). Influence of aggregate content on the behavior of fly ash based geopolymer concrete. Sci. Iran. 19 (5), 1188–1194. doi:10.1016/j.scient.2012.07.006
Juenger, M., Winnefeld, F., Provis, J. L., and Ideker, J. (2011). Advances in alternative cementitious binders. Cem. Concr. Res. 41 (12), 1232–1243. doi:10.1016/j.cemconres.2010.11.012
Khan, M., and Ali, M. (2020). Optimization of concrete stiffeners for confined brick masonry structures. J. Build. Eng. 32, 101689. doi:10.1016/j.jobe.2020.101689
Khan, M., Cao, M., Ai, H., and Hussain, A. (2022a). Basalt fibers in modified whisker reinforced cementitious composites. Period. Polytech. Civ. Eng. 66 (2), 344–354. doi:10.3311/ppci.18965
Khan, M., Cao, M., Chu, S., and Ali, M. (2022b). Properties of hybrid steel-basalt fiber reinforced concrete exposed to different surrounding conditions. Constr. Build. Mater. 322, 126340. doi:10.1016/j.conbuildmat.2022.126340
Khan, U. A., Jahanzaib, H. M., Khan, M., and Ali, M. (2018). Improving the tensile energy absorption of high strength natural fiber reinforced concrete with fly-ash for bridge girders. Key Eng. Mater. 765, 335–342. doi:10.4028/www.scientific.net/KEM.765.335
Khedmati, M., Kim, Y.-R., Turner, J. A., Alanazi, H., and Nguyen, C. (2018). An integrated microstructural-nanomechanical-chemical approach to examine material-specific characteristics of cementitious interphase regions. Mater. Charact. 138, 154–164. doi:10.1016/j.matchar.2018.01.045
Lan, T., Meng, Y., Ju, T., Chen, Z., Du, Y., Deng, Y., et al. (2022). Synthesis and application of geopolymers from municipal waste incineration fly ash (MSWI FA) as raw ingredient-A review. Resour. Conservation Recycl. 182, 106308. doi:10.1016/j.resconrec.2022.106308
Lao, J.-C., Huang, B.-T., Xu, L.-Y., Khan, M., Fang, Y., and Dai, J.-G. (2023a). Seawater sea-sand Engineered Geopolymer Composites (EGC) with high strength and high ductility. Cem. Concr. Compos. 138, 104998. doi:10.1016/j.cemconcomp.2023.104998
Lao, J.-C., Xu, L.-Y., Huang, B.-T., Zhu, J.-X., Khan, M., and Dai, J.-G. (2023b). Utilization of sodium carbonate activator in strain-hardening ultra-high-performance geopolymer concrete (SH-UHPGC). Front. Mater. 10, 1–12. doi:10.3389/fmats.2023.1142237
Lemougna, P. N., Wang, K.-t., Tang, Q., Melo, U. C., and Cui, X.-m. (2016). Recent developments on inorganic polymers synthesis and applications. Ceram. Int. 42 (14), 15142–15159. doi:10.1016/j.ceramint.2016.07.027
Li, C., Sun, H., and Li, L. (2010). A review: the comparison between alkali-activated slag (Si+Ca) and metakaolin (Si+Al) cements. Cem. Concr. Res. 40 (9), 1341–1349. doi:10.1016/j.cemconres.2010.03.020
Li, V. C., Bos, F. P., Yu, K., McGee, W., Ng, T. Y., Figueiredo, S. C., et al. (2020). On the emergence of 3D printable engineered, strain hardening cementitious composites (ECC/SHCC). Cem. Concr. Res. 132, 106038. doi:10.1016/j.cemconres.2020.106038
Li, V. C. (2019). Engineered cementitious composites (ECC): Bendable concrete for sustainable and resilient infrastructure. Germany: Springer.
Li, Z., Fei, M.-E., Huyan, C., and Shi, X. (2021). Nano-engineered, fly ash-based geopolymer composites: an overview. Resour. Conservation Recycl. 168, 105334. doi:10.1016/j.resconrec.2020.105334
Li, Z., and Shi, X. (2020). Graphene oxide modified, clinker-free cementitious paste with principally alkali-activated fly ash. Fuel 269, 117418. doi:10.1016/j.fuel.2020.117418
Lloyd, N., and Rangan, V. (2009). Geopolymer concrete-sustainable cementless concrete. Proc. Tenth ACI Int. Conf. 261, 33–53. doi:10.14359/51663200
Luković, M., Hordijk, D., Huang, Z., and Schlangen, E. (2019). Strain Hardening Cementitious Composite (SHCC) for crack width control in reinforced concrete beams. Heron 64 (1/2), 181.
Luukkonen, T., Abdollahnejad, Z., Yliniemi, J., Kinnunen, P., and Illikainen, M. (2018). One-part alkali-activated materials: A review. Cem. Concr. Res. 103, 21–34. doi:10.1016/j.cemconres.2017.10.001
Markoulli, M. P., Lee, C. I., Byington, E., and Felps, W. A. (2017). Mapping human resource management: reviewing the field and charting future directions. Hum. Resour. Manag. Rev. 27 (3), 367–396. doi:10.1016/j.hrmr.2016.10.001
Maruta, M., Kanda, T., Nagai, S., and Yamamoto, Y. (2005). New high-rise RC structure using pre-cast ECC coupling beam. Concr. J. 43 (11), 18–26. doi:10.3151/coj1975.43.11_18
Mehta, A., and Siddique, R. (2016). An overview of geopolymers derived from industrial by-products. Constr. Build. Mater. 127, 183–198. doi:10.1016/j.conbuildmat.2016.09.136
Mishra, J., Nanda, B., Patro, S. K., and Krishna, R. S. (2022). Sustainable fly ash based geopolymer binders: A review on compressive strength and microstructure properties. Sustainability 14 (22), 15062. doi:10.3390/su142215062
Mohammed, A. A., Ahmed, H. U., and Mosavi, A. (2021). Survey of mechanical properties of geopolymer concrete: A comprehensive review and data analysis. Mater. [Online] 14 (16), 4690. doi:10.3390/ma14164690
Mohmmad, S. H., Shakor, P., Muhammad, J. H., Hasan, M. F., and Karakouzian, M. (2023). Sustainable alternatives to cement: synthesizing metakaolin-based geopolymer concrete using nano-silica. Constr. Mater. 3 (3), 276–286. doi:10.3390/constrmater3030018
Mondal, P., Shah, S., and Marks, L. (2009). Nanomechanical properties of interfacial transition zone in concrete. Nanotechnol. Constr. 3, 315–320. doi:10.1007/978-3-642-00980-8_42
Nath, P., and Sarker, P. K. (2017). Flexural strength and elastic modulus of ambient-cured blended low-calcium fly ash geopolymer concrete. Constr. Build. Mater. 130, 22–31. doi:10.1016/j.conbuildmat.2016.11.034
Nematollahi, B., Sanjayan, J., Qiu, J., and Yang, E.-H. (2017). Micromechanics-based investigation of a sustainable ambient temperature cured one-part strain hardening geopolymer composite. Constr. Build. Mater. 131, 552–563. doi:10.1016/j.conbuildmat.2016.11.117
Nurruddin, M. F., Sani, H., Mohammed, B. S., and Shaaban, I. (2018). Methods of curing geopolymer concrete: A review. Int. J. Adv. Appl. Sci. 5 (1), 31–36. doi:10.21833/ijaas.2018.01.005
Ohno, M., and Li, V. C. (2018). An integrated design method of Engineered Geopolymer Composite. Cem. Concr. Compos. 88, 73–85. doi:10.1016/j.cemconcomp.2018.02.001
Olivia, M., and Nikraz, H. (2012). Properties of fly ash geopolymer concrete designed by Taguchi method. Mater. Des. 36, 191–198. doi:10.1016/j.matdes.2011.10.036
Oraee, M., Hosseini, M. R., Papadonikolaki, E., Palliyaguru, R., and Arashpour, M. (2017). Collaboration in BIM-based construction networks: A bibliometric-qualitative literature review. Int. J. Proj. Manag. 35 (7), 1288–1301. doi:10.1016/j.ijproman.2017.07.001
Pacheco-Torgal, F., Castro-Gomes, J., and Jalali, S. (2008). Alkali-activated binders: A review. Constr. Build. Mater. 22 (7), 1305–1314. doi:10.1016/j.conbuildmat.2007.10.015
Palomo, Á., Kavalerova, E., Fernández-Jiménez, A., Krivenko, P., García-Lodeiro, I., and Maltseva, O. (2015). A review on alkaline activation: new analytical perspectives. Mater. construccion 64, e022. doi:10.3989/mc.2014.00314
Park, J. Y., and Nagy, Z. (2018). Comprehensive analysis of the relationship between thermal comfort and building control research-A data-driven literature review. Renew. Sustain. Energy Rev. 82, 2664–2679. doi:10.1016/j.rser.2017.09.102
Partha, S. D., Pradip, N., and Prabir, K. S. (2013). Strength and permeation properties of slag blended fly ash based geopolymer concrete. Adv. Mater. Res. 651, 168–173. doi:10.4028/www.scientific.net/AMR.651.168
Phoo-ngernkham, T., Chindaprasirt, P., Sata, V., Pangdaeng, S., and Sinsiri, T. (2013). Properties of high calcium fly ash geopolymer pastes with Portland cement as an additive. Int. J. Min. Metall. Mater 20 (2), 214–220. doi:10.1007/s12613-013-0715-6
Posi, P., Teerachanwit, C., Tanutong, C., Limkamoltip, S., Lertnimoolchai, S., Sata, V., et al. (2013). Lightweight geopolymer concrete containing aggregate from recycle lightweight block. Mater. Des. 52, 580–586. doi:10.1016/j.matdes.2013.06.001
Provis, J. L. (2018). Alkali-activated materials. Cem. Concr. Res. 114, 40–48. doi:10.1016/j.cemconres.2017.02.009
Provis, J. L., and Van Deventer, J. S. J. (2009). Geopolymers: Structures, processing, properties and industrial applications. Germany: Elsevier.
Qaidi, S. M., Atrushi, D. S., Mohammed, A. S., Ahmed, H. U., Faraj, R. H., Emad, W., et al. (2022). Ultra-high-performance geopolymer concrete: A review. Constr. Build. Mater. 346, 128495. doi:10.1016/j.conbuildmat.2022.128495
Raijiwala, D., and Patil, H. (2010). “Geopolymer concrete A green concrete,” in 2010 2nd international conference on Chemical, Biological and Environmental Engineering: IEEE), Cairo, Egypt, 2-4 November, 2010, 202–206.
Rajak, D. K., Wagh, P. H., and Linul, E. (2022). A review on synthetic fibers for polymer matrix composites: performance, failure modes and applications. Materials 15 (14), 4790. doi:10.3390/ma15144790
Rashad, A. M. (2013). Properties of alkali-activated fly ash concrete blended with slag. Iran. J. Mater. Sci. Eng. 10 (1), 57–64.
Ren, J., Guo, S.-Y., Su, J., Zhao, T.-J., Chen, J.-Z., and Zhang, S.-L. (2019). A novel TiO2/Epoxy resin composited geopolymer with great durability in wetting-drying and phosphoric acid solution. J. Clean. Prod. 227, 849–860. doi:10.1016/j.jclepro.2019.04.203
Riaz Ahmad, M., Khan, M., Wang, A., Zhang, Z., and Dai, J.-G. (2023). Alkali-activated materials partially activated using flue gas residues: an insight into reaction products. Constr. Build. Mater. 371, 130760. doi:10.1016/j.conbuildmat.2023.130760
Ryu, G. S., Lee, Y. B., Koh, K. T., and Chung, Y. S. (2013). The mechanical properties of fly ash-based geopolymer concrete with alkaline activators. Constr. Build. Mater. 47, 409–418. doi:10.1016/j.conbuildmat.2013.05.069
Şahmaran, M., and Li, V. C. (2009). Durability properties of micro-cracked ECC containing high volumes fly ash. Cem. Concr. Res. 39 (11), 1033–1043. doi:10.1016/j.cemconres.2009.07.009
Salas, D. A., Ramirez, A. D., Ulloa, N., Baykara, H., and Boero, A. J. (2018). Life cycle assessment of geopolymer concrete. Constr. Build. Mater. 190, 170–177. doi:10.1016/j.conbuildmat.2018.09.123
Sandanayake, M., Law, D., and Sargent, P. (2022). A new framework for assessing the environmental impacts of circular economy friendly soil waste-based geopolymer cements. Build. Environ. 210, 108702. doi:10.1016/j.buildenv.2021.108702
Saravanan, S., and Elavenil, S. (2018). Strength properties of geopolymer concrete using M sand by assessing their mechanical characteristics. ARPN J. Eng. Appl. Sci. 13 (13), 4028–4041.
Shaikh, F. (2013). Deflection hardening behaviour of short fibre reinforced fly ash based geopolymer composites. Mater. Des. 50, 674–682. doi:10.1016/j.matdes.2013.03.063
Shakor, P., Hasan, S., Awuzie, B. O., Singh, A. K., Rauniyar, A., Karakouzian, M., et al. (2023). Evaluating the potential of geopolymer concrete as a sustainable alternative for thin white-topping pavement. Front. Mater. 10, 1181474. doi:10.3389/fmats.2023.1181474
Shakor, P. N., and Pimplikar, S. (2011). Glass fibre reinforced concrete use in construction. Int. J. Technol. Eng. Syst. 2 (2).
Shanmugasundaram, N., and Praveenkumar, S. (2021). Influence of supplementary cementitious materials, curing conditions and mixing ratios on fresh and mechanical properties of engineered cementitious composites–A review. Constr. Build. Mater. 309, 125038. doi:10.1016/j.conbuildmat.2021.125038
Sharma, R. C., Palli, S., Sharma, S. K., and Roy, M. (2017). Modernization of railway track with composite sleepers. Int. J. Veh. Struct. Syst. 9 (5), 321–329. doi:10.4273/ijvss.9.5.10
Shehab, H. K., Eisa, A. S., and Wahba, A. M. (2016). Mechanical properties of fly ash based geopolymer concrete with full and partial cement replacement. Constr. Build. Mater. 126, 560–565. doi:10.1016/j.conbuildmat.2016.09.059
Sivakumar, A., and Kishore, R. (2017). Evaluation of mechanical properties of fly ash and GGBS based geopolymer concrete. J. Emerg. Technol. Innov. Res. 4, 1028–1033.
Sofi, M., Van Deventer, J., Mendis, P., and Lukey, G. (2007a). Engineering properties of inorganic polymer concretes (IPCs). Cem. Concr. Res. 37 (2), 251–257. doi:10.1016/j.cemconres.2006.10.008
Sofi, M., van Deventer, J. S. J., Mendis, P. A., and Lukey, G. C. (2007b). Engineering properties of inorganic polymer concretes (IPCs). Cem. Concr. Res. 37 (2), 251–257. doi:10.1016/j.cemconres.2006.10.008
Song, H., Liu, J., He, K., and Ahmad, W. (2021). A comprehensive overview of jute fiber reinforced cementitious composites. Case Stud. Constr. Mater. 15, e00724. doi:10.1016/j.cscm.2021.e00724
Tanu, H., and Unnikrishnan, S. (2023). Review on durability of geopolymer concrete developed with industrial and agricultural byproducts. Mater. Today Proc. 2023. doi:10.1016/j.matpr.2023.03.335
Tayeh, B. A., Al Saffar, D. M., and Alyousef, R. (2020). The utilization of recycled aggregate in high performance concrete: a review. J. Mater. Res. Technol. 9 (4), 8469–8481. doi:10.1016/j.jmrt.2020.05.126
Tayeh, B. A., Zeyad, A. M., Agwa, I. S., and Amin, M. (2021). Effect of elevated temperatures on mechanical properties of lightweight geopolymer concrete. Case Stud. Constr. Mater. 15, e00673. doi:10.1016/j.cscm.2021.e00673
Trindade, A. C. C., Heravi, A. A., Curosu, I., Liebscher, M., de Andrade Silva, F., and Mechtcherine, V. (2020). Tensile behavior of strain-hardening geopolymer composites (SHGC) under impact loading. Cem. Concr. Compos. 113, 103703. doi:10.1016/j.cemconcomp.2020.103703
Vijai, K., Kumutha, R., and Vishnuram, B. (2011). Experimental investigations on mechanical properties of geopolymer concrete composites. Mater. Proceeding 60, 40. doi:10.1016/j.matpr.2021.11.325
Wang, K., Lemougna, P. N., Tang, Q., Li, W., He, Y., and Cui, X. (2017). Low temperature depolymerization and polycondensation of a slag-based inorganic polymer. Ceram. Int. 43 (12), 9067–9076. doi:10.1016/j.ceramint.2017.04.052
Wang, S., and Li, V. C. (2007). Engineered cementitious composites with high-volume fly ash. ACI Mater. J. 104 (3), 233. doi:10.1201/b15883-8
Wang, S., Xue, Q., Ma, W., Zhao, K., and Wu, Z. (2021). Experimental study on mechanical properties of fiber-reinforced and geopolymer-stabilized clay soil. Constr. Build. Mater. 272, 121914. doi:10.1016/j.conbuildmat.2020.121914
Wang, T., Fan, X., Gao, C., Qu, C., Liu, J., and Yu, G. (2023). The influence of fiber on the mechanical properties of geopolymer concrete: A review. Polymers 15 (4), 827. doi:10.3390/polym15040827
Wang, Y.-S., Alrefaei, Y., and Dai, J.-G. (2019). Silico-aluminophosphate and alkali-aluminosilicate geopolymers: A comparative review. Front. Mater. 6, 106. doi:10.3389/fmats.2019.00106
Wilkinson, A., Magee, B., Woodward, D., and Tretsiakova-McNally, S. (2016). “Development of resilient and environmentally responsible highway infrastructure solutions using geopolymer cement concrete,” in Civil Engineering Research in Ireland 2016 (CERI2016) Conference: Civil Engineering Research Association of Ireland, Galway, Ireland, August 29, 2016.
Xu, G., and Shi, X. (2018). Characteristics and applications of fly ash as a sustainable construction material: A state-of-the-art review. Resour. Conservation Recycl. 136, 95–109. doi:10.1016/j.resconrec.2018.04.010
Yang, K.-H., Song, J.-K., Ashour, A. F., and Lee, E.-T. (2008). Properties of cementless mortars activated by sodium silicate. Constr. Build. Mater. 22 (9), 1981–1989. doi:10.1016/j.conbuildmat.2007.07.003
Yang, K.-H., Song, J.-K., and Song, K.-I. (2013). Assessment of CO2 reduction of alkali-activated concrete. J. Clean. Prod. 39, 265–272. doi:10.1016/j.jclepro.2012.08.001
Yao, Z. T., Ji, X., Sarker, P., Tang, J., Ge, L., Xia, M., et al. (2015). A comprehensive review on the applications of coal fly ash. Earth-science Rev. 141, 105–121. doi:10.1016/j.earscirev.2014.11.016
Yousefi Oderji, S., Chen, B., Ahmad, M. R., and Shah, S. F. A. (2019). Fresh and hardened properties of one-part fly ash-based geopolymer binders cured at room temperature: effect of slag and alkali activators. J. Clean. Prod. 225, 1–10. doi:10.1016/j.jclepro.2019.03.290
Yu, F., and Hayes, B. E. (2018). Applying data analytics and visualization to assessing the research impact of the cancer cell biology (CCB) program at the university of North Carolina at chapel hill. J. eScience Librariansh. 7 (1), e1123. doi:10.7191/jeslib.2018.1123
Yun, K.-K., and Choi, P. (2014). Causes and controls of cracking at bridge deck overlay with very-early strength latex-modified concrete. Constr. Build. Mater. 56, 53–62. doi:10.1016/j.conbuildmat.2014.01.055
Zahid, M., Shafiq, N., Isa, M. H., and Gil, L. (2018). Statistical modeling and mix design optimization of fly ash based engineered geopolymer composite using response surface methodology. J. Clean. Prod. 194, 483–498. doi:10.1016/j.jclepro.2018.05.158
Zhang, J., Sun, H., Wan, J., and Yi, Z. (2009). Study on microstructure and mechanical property of interfacial transition zone between limestone aggregate and Sialite paste. Constr. Build. Mater. 23 (11), 3393–3397. doi:10.1016/j.conbuildmat.2009.06.037
Zhang, N., Yan, C., Li, L., and Khan, M. (2022). Assessment of fiber factor for the fracture toughness of polyethylene fiber reinforced geopolymer. Constr. Build. Mater. 319, 126130. doi:10.1016/j.conbuildmat.2021.126130
Zhao, R., Yuan, Y., Wei, X., Shen, R., Zheng, K., Qian, Y., et al. (2020). Review of annual progress of bridge engineering in 2019. Adv. Bridge Eng. 1, 11–57. doi:10.1186/s43251-020-00011-w
Zhong, H., and Zhang, M. (2022). Engineered geopolymer composites: A state-of-the-art review. Cem. Concr. Compos. 135, 104850. doi:10.1016/j.cemconcomp.2022.104850
Keywords: engineered geopolymer composites (EGC), geopolymer, tensile ductility, 3D printing concrete, fiber, cementitious matrix
Citation: Zhang Y, Li H, Gamil Y, Iftikhar B and Murtaza H (2023) Towards modern sustainable construction materials: a bibliographic analysis of engineered geopolymer composites. Front. Mater. 10:1277567. doi: 10.3389/fmats.2023.1277567
Received: 14 August 2023; Accepted: 12 September 2023;
Published: 05 October 2023.
Edited by:
Sahar A. Mostafa, Beni-Suef University, EgyptReviewed by:
Pshtiwan Shakor, Sulaimani Polytechnic University, IraqChaopeng Xie, North China University of Water Conservancy and Electric Power, China
Copyright © 2023 Zhang, Li, Gamil, Iftikhar and Murtaza. This is an open-access article distributed under the terms of the Creative Commons Attribution License (CC BY). The use, distribution or reproduction in other forums is permitted, provided the original author(s) and the copyright owner(s) are credited and that the original publication in this journal is cited, in accordance with accepted academic practice. No use, distribution or reproduction is permitted which does not comply with these terms.
*Correspondence: Yin Zhang, cXkxMTAzNzI5QDE2My5jb20=; Yaser Gamil, eWFzZXIuZ2FtaWxAbHR1LnNl