- 1Key Laboratory of Fluid and Power Machinery (Xihua University), Ministry of Education, Chengdu, China
- 2Zigong Zhaoqiang Sealing Products Industrial Co., Ltd., Zigong, China
A series of ferrite nanoparticles were synthesized via ion doping and then were coated by surfactant and dispersed in perfluorinated polyether oil (PFPE-oil), and the various ferrite ferrofluids were obtained. The scanning electron microscope was used to characterize the morphology of particles and the dispersed state of ferrofluid, energy-dispersive spectroscopy was used to study the chemical composition of particles, fourier transform infrared spectroscopy (FTIR) and thermogravimetric analysis were used to study the coated effect of PFPE-acids on particles, vibrating sample magnetometer was used to research the magnetization curves of ferrite particles, and the rheological property of the ferrite ferrofluids was studied by a rheometer. The results show that Zn2+, Mn2+/Zn2+, and Dy3+ ions were doped in the ferrite nanoparticles with a size less than 50 nm. The four kinds of ferrite nanoparticles have the characteristics of super-paramagnetic materials, and the M-T curves decrease with increasing temperature, while their decline rates are notably different. The ferrite particles are coated with PFPE acids chemically, and the ferrofluids have well dispersion stability. The rheological properties of the ferrite ferrofluids change with the variation of ion doping, magnetic field strength, temperature, etc. The magnetism and viscosity of ferrite ferrofluids are regularly affected by ion doping, and the results will have a great significance on basic research and related applications.
1 Introduction
Ferrofluid is a kind of colloidal solution, which is synthesized through dispersing magnetic nanoparticles coated by surfactant in base liquid (Chen et al., 2018). Under a magnetic field, ferrofluids have both magnetism and mobility and thus are widely used in sealing, biomedicine, aerospace, military, etc. (Cunha et al., 2018; Chen et al., 2019; Nilankush et al., 2019; Chen et al., 2021a; Taghizadeh et al., 2021; Li et al., 2022). The rheological properties of ferrofluid, such as viscosity-temperature, magneto-viscous, and viscosity-shear rate, greatly affect the related application effects, such as sealing, biomedicine, and damping (Hong et al., 1997; Hosseini et al., 2012; Wang et al., 2012; Chand et al., 2013; Chen et al., 2021b). The relationship between the rheological property and magnetic field strength was researched by Odenbach, and the experimental law was confirmed to be consistent with the simulated law (Odenbach and Raj, 2000). Stork revealed that the viscosity of ferrofluid under a magnetic field was larger and bigger than that of the fluids under no magnetic field (Odenbach and Stork, 1998). It was reported by Ruoyu Hong that the viscosity of water-based ferrofluids was related to nanoparticle concentration and shear rate (Hong et al., 2007). The relationship between the rheological property of the ferrofluid, shear rate, and magnetic field strength was revealed by Cuifeng Jiang (Jiang et al., 2008). Ly H. V. found that when the nanoparticle concentration of paraffin-based ferrofluids was too high, the peak value of the viscosity curve appeared, which was caused by the phase separation (Ly et al., 1999). Saeid Atashrouz revealed that the relationship between the rheological property of alcohol-water-based ferrofluid and temperature was consistent with the theoretical prediction (Atashrouz et al., 2016). It follows that the rheological properties of ferrofluids are related to particle nanoparticles, base liquid type, shear rate, magnetic field strength, and temperature.
What is more? Hongchao Cui revealed that the viscosity-temperature of PFPE oil-based ferrofluid was affected by base liquid type (Cui and Li, 2019). Bai Le showed that the silicon oil-based ferrofluid had superior stability of viscosity-temperature (Bai et al., 2017). J. R. Cantow (Cantow et al., 1987) and Z. K. Li (Li et al., 2017) reported that the viscosity of PFPE oil-based ferrofluid decreased gradually with increasing temperature, attributing to the viscosity-temperature characteristics of base liquid. In addition, the rheological property of ferrofluids was related to the magnetism temperature of magnetic nanoparticles, which was reported by S. Odenbach (Odenbach and Stork, 1998).
Ion doping has a great effect on the magnetism temperature of ferrite nanoparticles and their ferrofluids. The Mn1-xZnxFe2O4 ferrofluids had temperature-sensitive characteristics, and their saturation magnetization was reduced with rising temperature (Zhuang et al., 2012). The Mn1-xZnxFe2O4 ferrofluid with temperature-sensitive characteristics was obtained by Z. H. Liao, and its curie temperature was reduced with increasing ion doping (Liao et al., 2011). Yibiao Chen revealed that the saturation magnetization of ferrofluids reduced with increasing temperature (Chen, 2019).
Nevertheless, the effect of ion doping on the rheological properties of ferrite ferrofluids has not been reported. Therefore, in the paper, a series of ferrite nanoparticles are synthesized by ion doping, the micro-structures and magnetism of the particles are characterized, and the influence of magnetism temperature of the ferrite particles on the rheological properties is revealed.
2 Experimental
2.1 Preparation of ferrite nanoparticles and ferrofluids
Briefly, ferric chloride (FeCl3·6H2O), ferrous sulfate (FeSO4·7H2O), zinc chloride (ZnCl2), manganese chloride (MnCl2·4H2O), dysprosium chloride (DyCl3), sodium hydroxide (NaOH), and hydrochloric acid (HCl) were purchased from Alfa Aesar Chemical Reagent Co., Ltd. (Shanghai, China). PFPE oil (F-(C3F6O)n-C2F5, Mw = 4600g/moL) and PFPE acids (C3F7O-(C3F6O)n-C3F4O2H, Mw = 3800g/moL) were purchased from Shanghai ICAN Chemical S&T Co., Ltd. (Shanghai, China). All chemicals were of analytical grade and were used without any further purification.
In a typical procedure, the ZnxFe3-xO4 (x = 0.2) ferrite nanoparticles were synthesized via a chemical co-precipitation method by the following steps: firstly, FeCl3·6H2O (0.032 M, 8.66 g, 99%), FeSO4·7H2O (0.013 M, 3.56 g, 99%), and ZnCl2 (0.003 M, 0.43 g, 99.99%) were dissolved in ultrapure water (600 mL) and stirred at 300r/min and 70°C. Secondly, NaOH (0.200 M, 8.00 g, and 99.99%) was added to the solution until the pH reached 12.0; meanwhile, mechanical agitation was carried out. After 30 min, the pH of the solution was adjusted to be ∼6.5 by adding HCl solution, and subsequently, PFPE acids (0.001 M, 3.00 g, 99%) were added to coat particles. One hour later, the particles were cleaned with ultra-pure water until the pH of the solution reached ∼7.0, and the particles were separated by a magnet. Next, particles were dried in an oven for 10 h and were ground to a powder-like state using a mortar. Finally, the particles were dispersed by mechanical agitation at 500 r/min for 10 h, and the ferrofluids were synthesized, respectively. Fe3O4, Mn1-xZnxFe2O4, and DyxFe3-xO4 ferrite nanoparticles and their ferrofluids could be prepared in the same way as described above, and the doping ratio x is shown in Table 1. A similar method has been reported by the author (Chen et al., 2018).
2.2 Characterization methods
The morphology of particles and the dispersed state of ferrofluids were characterized by scanning electron microscopy (SEM, Phenom Pharos G2); the energy-dispersive spectroscopy (EDS) was united to analyze the chemical composition of particles. Fourier Transform Infrared Spectrometer (FTIR, Nicolet iS10) spectra were used over the wavenumber range of 400-4000 cm-1 to investigate the interactions between surfactant and particles. The coated mass of PFPE acid on the particles was performed using a thermogravimetric analysis analyzer (TGA, TG209F1) from RT to 600°C with a heating rate of 10 C/min in an argon atmosphere. A vibrating sample magnetometer (VSM, Lake Shore 7410) was used to test the magnetic characterization of nanoparticles at an external magnetic field ranging from −×2.5104 Oe to +2.5×104 Oe at 25°C. The magnetism temperature (M-T) of ferrite of nanoparticles was measured in the temperature range of 5–400 K, 3.0×104 Oe. The rheological properties of ferrite ferrofluids were investigated using a rotational rheometer (MCR 301, Anton-Paar), which generates an external magnetic field in a direction perpendicular to shear flow. A cone-plate system of non-magnetic metal with a diameter of 20 mm was used, and the gap was set to 0.5 mm.
3 Results and discussion
3.1 The micro-structure and the chemical composition of particles
Figure 1 shows the SEM images and EDS images of particles. In Figure 1, the morphology of the four kinds of particles is nearly spherical, and the size of the particles is less than 50 nm. From the EDS results, it can be seen that Zn2+, Mn2+/Zn2+, and Dy3+ have been doped in the ferrite particles. Fe3O4 was partly oxidized and the atom ratio between Fe and O was deviated at 3/4. The atom ratio between Zn and Fe in the ZnxFe3-xO4, the atom ratio between Mn and Zn in the Mn1-xZnxFe2O4, and the atom ration between Dy and Fe in the DyxFe3-xO4, respectively, are all close to the raw material ratio, indicating the successful ion doping in particles.
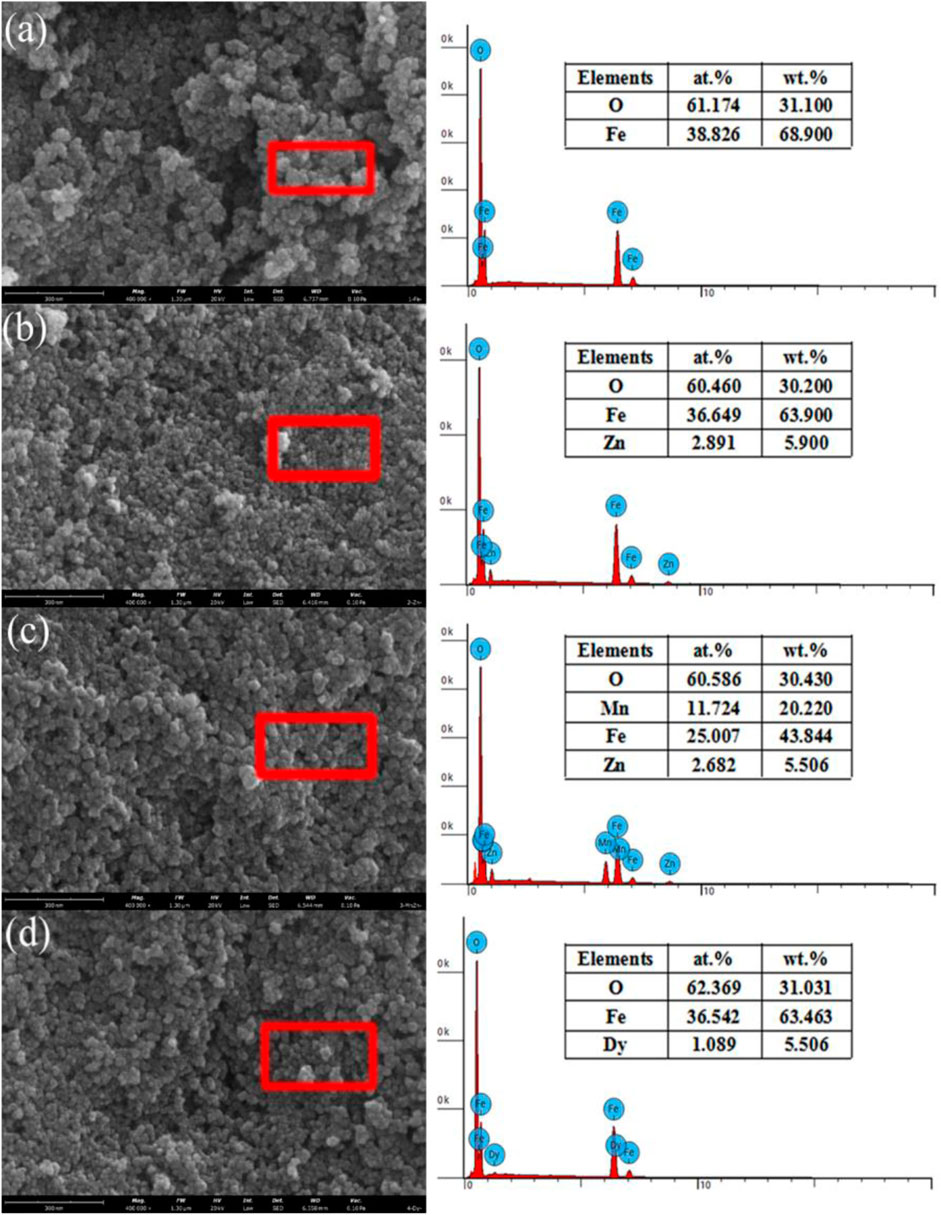
FIGURE 1. The SEM and EDS images of the particles: (A) Fe3O4, (B) ZnxFe3-xO4, (C) Mn1-xZnxFe2O4, and (D) DyxFe3-xO4.
Figure 2 shows the magnetization curves of the four kinds of ferrite nanoparticles. From the VSM curves tested at RT (Figure 2A), the magnetization of particles becomes saturated when the applied magnetic field magnitude reaches 30,000 Oe. Moreover, the four kinds of particles have the characteristics of super-paramagnetic material; the magnetization curves are “S” shape and their coercivity is very little. The distribution of ions in ferrite particles is generally represented by the formula (Fe3+)A [Fe2+Fe3+]BO4, where the parentheses and square brackets refer to tetrahedral and octahedral voids, respectively (Srivastava et al., 2018). The four kinds of particles are ordered by the size of their saturation magnetization as ZnxFe3-xO4, DyxFe3-xO4, Fe3O4, and Mn1-xZnxFe2O4, which is because the condition of ions occupied in A and B was changed by doped ions, and the net magnetic moment was also changed. What is more? The magnetism value is also affected by the type of doping ions, doping mass, particle size, and particle size distribution (Chen et al., 2021a).
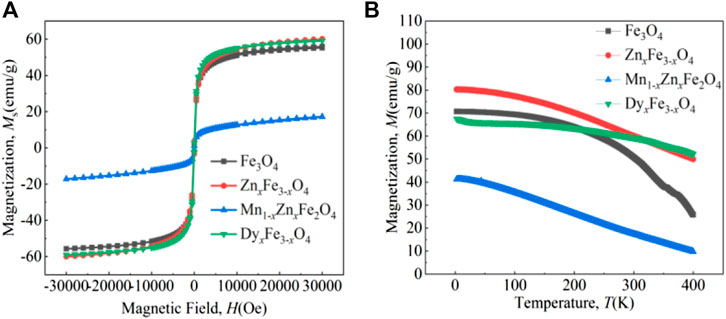
FIGURE 2. The magnetic curves of the ferrite nanoparticles: (A) at room temperature and (B) at a variable temperature.
From the M-T curves of the four kinds of particles tested at 30,000 Oe (Figure 2B), the saturation magnetism of particles reduces with a heating temperature from 1.8 K to 400 K. Research has shown that the magnetization of particles becomes saturated when the field magnitude reaches 30,000 Oe at RT. In a stronger magnetic field, presumably, we could tell that the magnetization of the ferrite nanoparticles will not change significantly. In a weaker magnetic field, the M-T curve will change, and the value of magnetism is probably lower than that of the particle tested under the same temperature. To avoid unknown and complex influences of the field magnitude, we chose to test the M-T curves at 30,000 Oe.
Not only that, the rate of decrease k = ΔM/ΔT of the M-T curves is obviously different, which is related to the Curie temperature of the ferrite nanoparticles. The Curie temperature was related to the interaction effect of A-A, A-B, and B-B, of which the effect of A-B interaction on the Curie temperature was the strongest (Eltabey et al., 2011). It has been reported that the induction of Gd3+ ions into the Fe3O4 nanoferrite matrix results in the weakening of Fe3+(A)–O2––Gd3+(B) superexchange interactions, which are responsible for the lower Curie temperature (Thorat et al., 2016). What is more? The decline rate of the M-T curve is affected by particle size, morphology of particles, particle size distribution, type of ion doping, doping mass of ions, etc., which is very complicated. For the four different kinds and masses of ions doping, it is very difficult to explain the M-T decline rate of the four different kinds of ferrite nanoparticles. The four kinds of particles are ordered by the size of their saturation magnetization as DyxFe3-xO4, ZnxFe3-xO4, Fe3O4, and Mn1-xZnxFe2O4 at ∼348 K.
3.2 The FTIR and TGA of magnetic nanoparticles
Figure 3 shows the TGA and FTIR of coated nanoparticles. From Figure 3A, the particles could be chemically coated by surfactant PFPE acids so that the stretching vibrations of C=O from COOH in the PFPE acid shift from 1777cm-1 to 1685cm-1 in coated particles using PFPE acid, which may be due to the red-shift effect of the covalent bonding with the bare charge on particles (Chen et al., 2018). The stretching vibrations of uncoated ferrite nanoparticles often appear in low peak positions (Mozaffari et al., 2010; Cui et al., 2015). In Figure 3B, ion doping has little effect on the coated mass of particles, and the four kinds of particles are ordered by their coated mass as Mn1-xZnxFe2O4, DyxFe3-xO4, Fe3O4, and ZnxFe3-xO4.
3.3 The SEM images of the four kinds of ferrite ferrofluids
Figure 4 shows the SEM images of the four kinds of ferrite nanoparticle ferrofluids. From the images, the four kinds of ferrite particles were relatively uniformly dispersed in the PFPE oil. The particles were arranged in a short chain length, and no obvious clusters occurred. This was because of the magnetic attraction and repulsion by the coated surfactant between particles (Chen et al., 2021a).
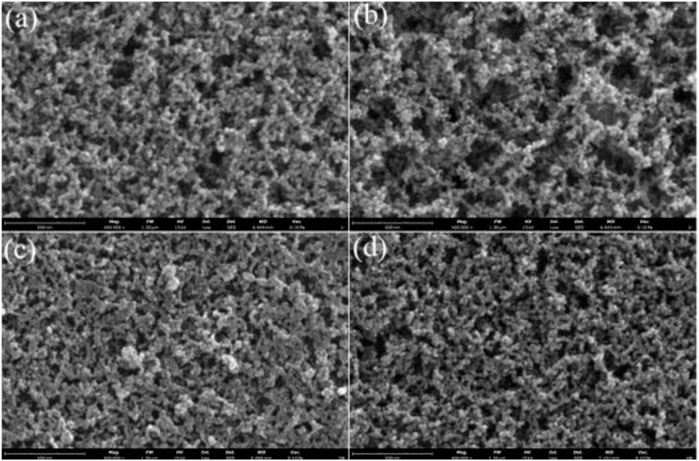
FIGURE 4. The SEM images of the four kinds of ferrite nanoparticles ferrofluids (400000X): (A) Fe3O4, (B) ZnxFe3-xO4, (C) Mn1-xZnxFe2O4, and (D) DyxFe3-xO4.
3.4 The rheological properties of various ferrite nanoparticle ferrofluids
Figure 5 shows the rheological properties of the four kinds of ferrite ferrofluids with and without a magnetic field at RT. In Figure 5A, without a magnetic field, by increasing the shear rate, the viscosity of Fe3O4, ZnxFe3-xO4, and Mn1-xZnxFe2O4 ferrofluid is almost unchanged; nevertheless, the viscosity of the DyxFe3-xO4 ferrofluid firstly decreases and then becomes flat, and shear thinning appears, which is attributed to the arrangement of nanoparticles and PFPE oil molecules along the shear direction (Chen et al., 2021b). The viscosity of ferrite ferrofluids is ordered by the particle type as DyxFe3-xO4, ZnxFe3-xO4, Fe3O4, and Mn1-xZnxFe2O4, which is affected by the particle size, the coated mass of surfactant, and the particle type (Jain et al., 2022).
In Figure 5B, when the applied magnetic field strength is 200 mT, with an increasing shear rate, the viscosity of Fe3O4 and Mn1-xZnxFe2O4 ferrofluid is almost unchanged; nevertheless, the viscosity of the ZnxFe1-xO4 and DyxFe3-xO4 ferrofluid firstly decreases and then becomes flat, and shear thinning appears. The viscosity of ferrite ferrofluids is ordered by the particle type as ZnxFe3-xO4, DyxFe3-xO4, Fe3O4, and Mn1-xZnxFe2O4. It has been shown that the order of the viscosity size of the four kinds of various ferrofluids is the same as the order of the saturation magnetization of corresponding particles at RT. In other words, the type of the ferrite nanoparticle has an effect on the viscosity of the ferrite ferrofluid. Moreover, the viscosity of ferrofluids under a magnetic field is larger than that of the fluids without a field, attributed to the arrangement of micro-structures which will stop the shear action (Chen et al., 2021b). When the applied magnetic field is 200 mT (2000 Oe), the magnetization of particles and ferrofluids has not reached saturation. It has been reported that when the magnetic field strength is less than 200 mT, the viscosity is mainly affected by the field strength, and when the applied field is big enough, the viscosity is mainly affected by the shear rate (Chen et al., 2021b). When the magnetic field is bigger than 200 mT, the viscosity-shear rate curves will make a difference. It has been shown that viscosity is affected by many effects, such as mass of ion doping, particle size, and particle morphology; therefore, it is very difficult to forecast the change of the four kinds of ferrite ferrofluids. Extensive testing of rheological properties is required before the application of ferrofluids.
Figure 6 shows the viscosity of the four kinds of ferrite ferrofluids as a function of shear rate under different temperatures at 400 mT. In Figure 6A, with increasing shear rate, the viscosity of Fe3O4 and Mn1-xZnxFe2O4 ferrofluid decreases slightly; however, the viscosity of ZnxFe1-xO4 and DyxFe3-xO4 ferrofluid decreases greatly. It had been reported that the viscosity stability of ferrofluid was required in some applications (Bai et al., 2017). At 298 K, the viscosity of the ferrofluids is higher than that of the fluids at 348 K (as shown in Figure 6B). In addition, the change rate of viscosity is different, which is affected by the particle type. At 298 K, the viscosity size of ferrite ferrofluids is ordered by the particle type as ZnxFe3-xO4, DyxFe3-xO4, Fe3O4, and Mn1-xZnxFe2O4. However, at 348 K, the order is changed to be DyxFe3-xO4, ZnxFe3-xO4, Fe3O4, and Mn1-xZnxFe2O4, which is the same as that of the saturation magnetization of ferrite nanoparticles. We can infer that the magnetism-temperature of magnetic nanoparticles will affect the viscosity of ferrite ferrofluid.
Research revealed that the force caused by magnetic field strength on magnetic nanoparticles is obtained through the following equation (Odenbach and Storkb, 1998):
Where M0 is the magnetization of nanoparticles and it reaches saturation with an increasing magnetic field, d is the average particle size, b is the shell thickness of coated nanoparticles, and µ0 is the permeability of nanoparticles. According to the equation, the force caused by Fm increases with increasing M02, and meanwhile, the particles arrange to be chain-like structures and even become columns along the magnetic field direction. Research has revealed that the Fm will affect the rheological properties of ferrofluids. According to the equation, the M-T curve of ferrite nanoparticles will affect the force caused by magnetic field strength Fm, and thus the rheological properties are affected by the type of ferrite nanoparticles.
In addition, when the magnetic field is larger than 400 mT, the magnetization of particles will reach saturation. The effect of ion doping in particles on the viscosity-temperature will tend to be a fixed value. When the magnetic field is lower than 400 mT, the effect of ion doping in particles on the viscosity-temperature may be similar so that the magnetization of the particle does not reach saturation. A lot of measurements of rheological properties should be done before the ferrofluids are used in viscosity related applications.
4 Conclusion
Four kinds of ferrite nanoparticles and ferrofluids are obtained through ion doping, and the ferrofluids have high dispersion stability. At 298 K, the size of the saturation magnetization of the four kinds of ferrite particles is ordered by ferrite type as DyxFe3-xO4, ZnxFe3-xO4, Fe3O4, and Mn1-xZnxFe2O4. The saturation magnetization of particles reduces with heating temperature from 1.8 K to 400 K. Not only that, the decline rate of k = ΔM/ΔT is different and greatly affected by ion doping. The kind and mass of doped ions will change the superexchange interactions of A-B, which are responsible for the lower Curie temperature and decline rate of M-T curves. The type and mass of doped ions will change the magnetism-temperature of ferrite nanoparticles, which further affects the viscosity and its variation of the ferrofluid. What is more? The viscosity is also affected by magnetic field strength, temperature, particle size, etc. It is very difficult to predict the change of the four kinds of ferrite ferrofluids. A lot of measurements should be done before the ferrofluids are used in applications.
Data availability statement
The original contributions presented in the study are included in the article/Supplementary Material, further inquiries can be directed to the corresponding authors.
Author contributions
FC: Writing–original draft. JZ: Writing–review and editing. XL: Supervision, Writing–review and editing. SY: Project administration, Writing–review and editing. WL: Writing–review and editing. ZY: Project administration, Writing–review and editing. ZL: Supervision, Writing–review and editing.
Funding
The author(s) declare financial support was received for the research, authorship, and/or publication of this article. This research was financially supported by the National Natural Science Foundation of China (No. 52079118) and the Key Research and Development Plan of Sichuan Province (No. 2020YFH0135).
Conflict of interest
Author ZY was employed by Zigong Zhaoqiang Sealing Products Industrial Co., Ltd.
The remaining authors declare that the research was conducted in the absence of any commercial or financial relationships that could be construed as a potential conflict of interest.
Publisher’s note
All claims expressed in this article are solely those of the authors and do not necessarily represent those of their affiliated organizations, or those of the publisher, the editors and the reviewers. Any product that may be evaluated in this article, or claim that may be made by its manufacturer, is not guaranteed or endorsed by the publisher.
References
Atashrouz, S., Mozaffarian, M., and Pazuki, G. (2016). Viscosity and rheological properties of ethylene glycol+water+Fe3O4 nanofluids at various temperatures: experimental and thermodynamics modeling. Korean J. Chem. Eng. 33 (9), 2522–2529. doi:10.1007/s11814-016-0169-4
Bai, L., Zhang, Z., and Xu, L. (2017). Preparation and performance studies of silicon oil based magnetic fluid. J. Funct. Mater. 48 (11), 5. doi:10.3969/j.issn.1001-9731.2017.11.018
Cantow, M. J. R., Barrall, E. M., Wolf, B. A., and Geerissen, H. (1987). Temperature and pressure dependence of the viscosities of perfluoropolyether fluids. J. Polym. Sci. Polym. Phys. 25, 603–609. doi:10.1002/polb.1987.090250311
Chand, M., Kumar, S., Shankar, A., Porwal, R., and Pant, R. P. (2013). The size induced effect on rheological properties of Co-ferrite based ferrofluid. J. Non-Cryst. Solids 361, 38–42. doi:10.1016/j.jnoncrysol.2012.10.003
Chen, B. (2019). Study on magnetic fluid seals under highspeed condition. China, Beijing: Beijing Jiaotong University.
Chen, F., Li, J., Li, X., Zhou, Q., and Yan, Z. (2019). Splitting regularities of thin ferrofluid layer manipulated by vertical magnetic field. J. Wuhan. Univ. Technol. 34 (1), 6–10. doi:10.1007/s11595-019-2006-1
Chen, F., Iiyas, N., Liu, X. B., and Li, Z. G. (2021a). Size effect of Fe3O4 nanoparticles on magnetism and dispersion stability of magnetic nanofluid. Front. Energy Res. 11, 780008. doi:10.3389/fenrg.2021.780008
Chen, F., Liu, X., Li, Z., Yan, S., Fu, H., and Yan, Z. (2021b). Investigation of the rheological properties of Zn-Ferrite/Perfluoropolyether oil-based ferrofluids. Nanomaterials-basel 11 (10), 92653. doi:10.3390/nano11102653
Chen, F., Liu, Y., and Yan, Z. (2018). Influence of various parameters on the performance of superior PFPE-oil-based ferrofluids. Chem. Phys. 513, 67–72. doi:10.1016/j.chemphys.2018.05.011
Cui, H., and Li, D. (2019). Fabrication and properties research on a novel perfluoropolyether based ferrofluid. J. Magn. Magn. Mater. 473, 341–347. doi:10.1016/j.jmmm.2018.10.039
Cui, H., Li, D., and Zhang, Z. (2015). Preparation and characterization of Fe3O4 magnetic nanoparticles modified by perfluoropolyether carboxylic acid surfactant. Mater Lett. 143 (15), 38–40. doi:10.1016/j.matlet.2014.12.037
Cunha, L., Siqueira, I. R., Oliveira, T. F., and Ceniceros, H. D. (2018). Field-induced control of ferrofluid emulsion rheology and droplet break-up in shear flows. Phys. Fluids 30, 122110. doi:10.1063/1.5055943
Eltabey, M. M., El-shokrofy, K. M., and Gharbia, S. A. (2011). Enhancement of the magnetic properties of Ni–Cu–Zn ferrites by the non-magnetic Al3+ ions substitution. J. Alloy Comp. 509 (5), 2473–2477. doi:10.1016/j.jallcom.2010.11.056
Hong, C. Y., Jang, I. J., Horng, H. E., Hsu, C. J., Yao, Y. D., and Yang, H. C. (1997). Ordered structures in Fe3O4 kerosene-based ferrofluids. J. Appl. Phys. 81, 4275–4277. doi:10.1063/1.364800
Hong, R. Y., Ren, Z. Q., Han, Y. P., Li, H., Zheng, Y., and Ding, J. (2007). Rheological properties of water-based Fe3O4 ferrofluids. Chem. Eng. Sci. 62, 5912–5924. doi:10.1016/j.ces.2007.06.010
Hosseini, S. M., Ghasemi, E., Fazlali, A., and Henneke, D. E. (2012). The effect of nanoparticle concentration on the rheological properties of paraffifin-based Co3O4 ferroflfluids. J. Nanopart. Res. 14, 858–867. doi:10.1007/s11051-012-0858-9
Jain, K., Kumar, P., Pant, R. P., and Basheed, G. A. (2022). Tunning of rheological and magnetic properties of Ni doped magnetite based magnetic nanofluid. Phys. B 643, 414136. doi:10.1016/j.physb.2022.414136
Jiang, C., Jiang, W., and Li, J. (2008). Preparation and rheological property of PSA@Fe3O4 doped CdS. J. Funct. Mater. 39 (12), 2019–2081.
Li, Z., Wang, M., Chen, F., Yan, S., Li, W., and Cheng, J. (2022). Influence of the axial tooth structure on the pressure resistance of the magnetic fluid sealing device. Vacuum 202, 111105. doi:10.1016/j.vacuum.2022.111105
Li, Z. K., Yao, J., and Li, D. C. (2017). Research on the rheological properties of a perfluoropolyether based ferrofluid. J. Magn. Magn. Mater. 424, 33–38. doi:10.1016/j.jmmm.2016.09.080
Liao, Z. H., Zhou, J. X., Zhuang, L., Shen, H., Wu, W. P., and Zhao, Y. X. (2011). Optimization of preparation parameters and characterization of the Mn0.5Zn0.5Fe2O4 thermal-sensitive magnetic ferrofluid prepared by the hydro-thermal method. International Conference on Consumer Electronics. IEEE, September 6 – 8: Berlin, Germany.
Ly, H. V., Reitich, F., Jolly, M. R., Banks, H., and Ito, K. (1999). Simulations of particle dynamics in magnetorheological fluids. J. Comput. Phys. 155 (1), 160–177. doi:10.1006/jcph.1999.6335
Mozaffari, M., Manouchehri, S., Yousefi, M., and Amighian, J. (2010). The effect of solution temperature on crystallite size and magnetic properties of Zn substituted Co ferrite nanoparticles. J. Magn. Magn. Mater. 322 (4), 383–388. doi:10.1016/j.jmmm.2009.09.051
Nilankush, A., Kalidas, D., and Kumar, K. P. (2019). On the heat transport mechanism and entropy generation in a nozzle of liquid rocket engine using ferrofluid: A computational framework. J. Comput. Des. Eng. 6 (4), 739–750. doi:10.1016/j.jcde.2019.02.003
Odenbach, S., and Raj, K. (2000). The influence of large particles and agglomerates on the magnetoviscous effect in ferroflfluids. Magnetohydrodynamics 36, 312–319. doi:10.1023/A:1010496907449
Odenbach, S., and Stork, H. (1998). Shear dependence of field-induced contributions to the viscosity of magnetic fluids at low shear rates. J. Magn. Magn. Mater. 183 (1-2), 188–194. doi:10.1016/S0304-8853(97)01051-2
Odenbach, S., and Storkb, H. (1998). Shear dependence of field-induced contributions to the viscosity of magnetic fluids at low shear rates. J. Magn. Magn. Mater. 183, 188–194. doi:10.1016/S0304-8853(97)01051-2
Srivastava, M., Alla, S. K., Meena, S. S., Gupta, N., Mandal, R. K., and Prasad, N. K. (2018). ZnxFe3-xO4 (0.01 ≤ x ≤ 0.8) nanoparticles for controlled magnetic hyperthermia application. New J. Chem. 42, 7144–7153. doi:10.1039/C8NJ00547H
Taghizadeh, M., Bozorgzadeh, F., and Ghorbani, M. (2021). Designing magnetic field sensor based on tapered photonic crystal fibre assisted by a ferrofluid. Sci. Rep-uk 11 (1), 14325. doi:10.1038/s41598-021-93568-z
Thorat, N. D., Bohara, R. A., Tofail, S. A. M., Alothman, Z. A., Shiddiky, M. J. A., A Hossain, M. S., et al. (2016). Superparamagnetic gadolinium ferrite nanoparticles with controllable curie temperature–cancer theranostics for MR-imaging-guided magneto-chemotherapy. Eur. J. Inorg. Chem. 2016, 4586–4597. doi:10.1002/ejic.201600706
Wang, S. H., Yang, C. C., and Bian, X. F. (2012). Magnetoviscous properties of Fe3O4 silicon oil based ferroflfluid. J. Magn. Magn. Mater. 324, 3361–3365. doi:10.1016/j.jmmm.2012.05.055
Keywords: ion doping, ferrite nanoparticle, magnetization curve, viscosity, ferrofluid
Citation: Chen F, Zhang J, Liu X, Yan S, Li W, Yan Z and Li Z (2023) The effect of ions doping on the rheological properties of ferrite ferrofluids. Front. Mater. 10:1264049. doi: 10.3389/fmats.2023.1264049
Received: 24 July 2023; Accepted: 28 August 2023;
Published: 22 September 2023.
Edited by:
Ehsan Nazarzadeh Zare, Damghan University, IranCopyright © 2023 Chen, Zhang, Liu, Yan, Li, Yan and Li. This is an open-access article distributed under the terms of the Creative Commons Attribution License (CC BY). The use, distribution or reproduction in other forums is permitted, provided the original author(s) and the copyright owner(s) are credited and that the original publication in this journal is cited, in accordance with accepted academic practice. No use, distribution or reproduction is permitted which does not comply with these terms.
*Correspondence: Fang Chen, Y2hlbmZhbmdAbWFpbC54aHUuZWR1LmNu; Zhenggui Li, bHpoZ3VpQG1haWwueGh1LmVkdS5jbg==