- 1Department of Stomatology, The First Affiliated Hospital of Chengdu Medical College, Chengdu, China
- 2Department of Rehabilitation Medicine, Southwest Medical University, Luzhou, China
- 3Department of Cardiothoracic Surgery, The First Affiliated Hospital of Chengdu Medical College, Chengdu, China
Microbial infections continue to pose a significant health challenge, especially with an increase in drug-resistant bacteria. Conventional antibiotic treatments show limited efficacy, prompting researchers to explore alternative treatments. Photodynamic therapy (PDT) has emerged as a promising alternative that uses reactive oxygen species (ROS) to induce oxidative stress, offering the potential for cyclic treatment without fostering new drug resistance mechanisms. The success of PDT relies heavily on the selection of appropriate photosensitizers (PSs). Various nanomaterials are being developed as PSs or carriers to enhance the efficacy of PDT in the antibacterial field. In this comprehensive review, we discuss the four main ROS generated during PDT and outline their corresponding antibacterial mechanisms. Additionally, we highlight the prominent types of nanomaterials used as PSs or carriers in PDT. We analyze the current challenges associated with nanomaterial-based PDT for antibacterial therapy and propose potential strategies for optimizing their applications.
1 Introduction
Microbial infections are a significant threat to human health which are prevalent in both community and hospital settings (Lamy et al., 2020). Bacterial cells possess the ability to secrete a range of toxins that elicit immune responses, disrupt local host environments, and ultimately cause infections (Ahmad-Mansour et al., 2021). These infections can manifest as localized or systemic conditions including soft tissue infections, osteomyelitis, endocarditis, bacteremia, and sepsis. In the absence of timely control measures, infections can progress to multiple organ failure and become fatal. Consequently, effective treatment of bacterial infections has emerged as a prominent focus in the medical field, particularly due to the emergence of antibiotic-resistant bacteria (Chang et al., 2022). This pressing concern has prompted researchers to explore alternative strategies to combat bacterial infections.
Photodynamic therapy (PDT) was discovered more than a century ago, which involves the use of specific agents that interact with light of a particular wavelength to generate reactive oxygen species (ROS). These ROS can eradicate microorganisms present at the site of infection. Compared to traditional antibiotic therapy, PDT offers superior biological safety and flexibility for cyclic administration, as required (Zhi et al., 2020). Notably, PDT has demonstrated remarkable efficacy against antibiotic-resistant bacteria while mitigating the emergence of novel resistance mechanisms. Consequently, PDT has emerged as a compelling strategy for treating diverse infections (Plotino et al., 2019; Correia-Barros et al., 2022). It involves the systemic or localized administration of photosensitizers (PSs), followed by irradiation with light of a specific wavelength. In the ground state, PSs absorb light energy and transfer into a highly energetic singlet state (1s*). Subsequently, the singlet-state PSs release energy and transfer to a triplet state (3s*). During this transition, the triplet-state PSs react with oxygen molecules, leading to ROS generation (Liu et al., 2022). However, conventional drug-based PSs have several inherent limitations including poor water solubility, inadequate control of drug release, and limited selectivity, all of which impede their clinical application. Moreover, certain drug-based PSs may cause unforeseen side effects and damage healthy tissues (Kwiatkowski et al., 2018). Although research efforts have been directed towards the development of novel PSs through organic synthesis, the complexity raises concerns regarding the associated cost and time requirements.
To overcome the limitations of traditional drug-based PSs, considerable research efforts have been directed towards the development of nanomaterials. These nanomaterials hold immense potential as they can serve as PSs or carriers, inducing photodynamic effects and eliciting antibacterial activity. Nanomaterial-based PDT possesses several noteworthy characteristics, including controlled-release capabilities, excellent water solubility, favorable biocompatibility, and the ability to generate significant levels of ROS (Lan et al., 2019; Yu et al., 2022). Therefore, nanomaterial-based PDT is promising for antibacterial therapy (Figure 1). In summary, this study aims to elucidate the mechanisms underlying the role of ROS in photodynamic antibacterial therapy, outline common types of nanomaterial-based PSs or carriers and their associated antibacterial effects, and explore the current limitations along with potential strategies for future optimization.
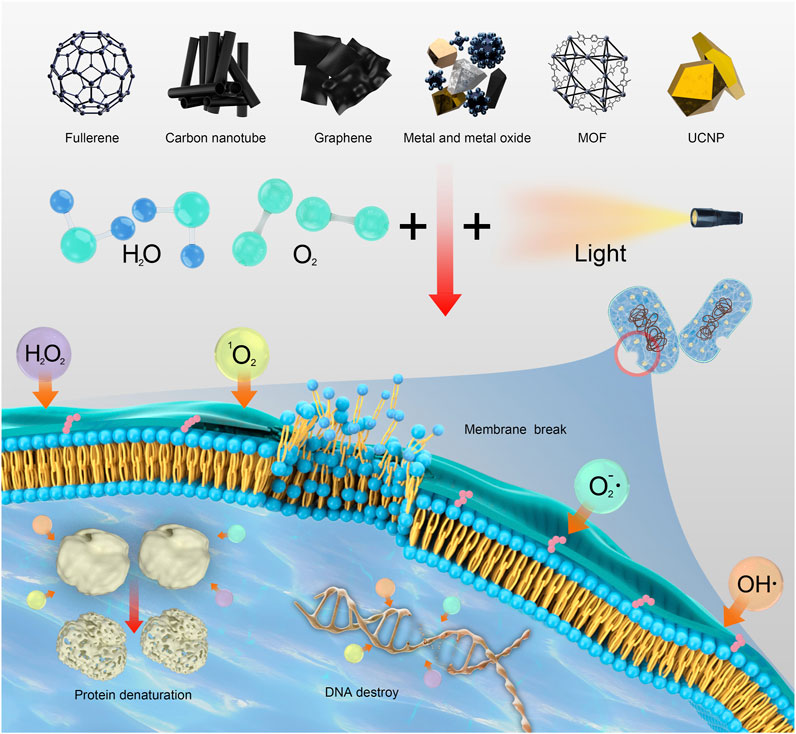
FIGURE 1. Schematics of multiple nanomaterial-based photosensitizers in photodynamic therapy against bacteria.
2 The production modes and antibacterial mechanisms of ROS
Two distinct pathways contribute to the antibacterial effects of PDT. The first pathway involves direct interactions between PSs and the substrate, leading to oxidation. However, this pathway is rare. The second pathway involves the reaction of PSs with ground-state oxygen, which results in the formation of ROS, including singlet oxygen (1O2), superoxide anion radicals (O2−•), hydroxyl radicals (OH•), and hydrogen peroxide (H2O2) (Hou et al., 2020). Excessive accumulation of ROS induces oxidative stress, which subsequently damages bacterial cells.
The ROS generated during the photodynamic process exert non-specific antimicrobial effects. Microbial death is a multifaceted process that has detrimental effects on the cell membrane, cytoplasmic material leakage, and DNA damage (Ezraty et al., 2017). During the photodynamic process, various ROS coexist and undergo transformations, creating a synergistic mechanism for the antibacterial effects of ROS. Notably, ROS represent the pivotal reactant within the photodynamic antibacterial process, and there is a linear correlation between ROS production and bacterial survival rate (Cho et al., 2004). Furthermore, ROS reactivity significantly influences antibacterial efficacy. A comprehensive analysis is warranted to elucidate the distinguishing characteristics of each ROS and discern the production and antibacterial mechanisms of different ROS types.
2.1 The mechanism of ROS generation and the types of ROS
During the photodynamic process, the active sites on the surface of PSs serve as docking sites for oxygen molecules, including O2 and H2O. This interaction facilitates the engagement of high-energy charge carriers, such as photo-generated electrons (e−) and holes (h+), leading to the subsequent generation of ROS (Sangam et al., 2022). When the incident light energy surpasses the band gap of the PSs, electron-hole separation occurs, with electrons being excited from the valence band to the conduction band, while holes remain within the valence band. Consequently, electrons in the conduction band and holes in the valence band possess potent reducing and oxidizing properties, respectively, thereby engaging in redox reactions with oxygen molecules to yield ROS (Wang et al., 2022). In practical settings, both H2O and O2 play crucial roles as reactants in photodynamic processes. Simultaneously, the reduction reaction of O2 with photo-generated electrons and the oxidation reaction of H2O with photo-generated holes occur.
PSs initially absorb light energy, leading to excitation from the ground state to the singlet state (1s*). Subsequently, the short-lived singlet state rapidly undergoes intersystem crossing to attain a more stable state known as the triplet state (3s*) (Xiao et al., 2022). PSs in the triplet excited state can initiate two distinct photodynamic pathways. The type I pathway involves the participation of 3s* PSs in electron transfer or hydrogen abstraction reactions, resulting in the generation of ROS such as O2−•, OH•, and H2O2 (Rajendran, 2016). In contrast, the type II pathway entails the direct transfer of 3s* PSs to the ground-state molecular oxygen, subsequently leading to energy transfer and the production of 1O2 (Yu et al., 2022). However, the type II pathway exhibits a high oxygen dependence, which poses challenges to its therapeutic efficacy in hypoxic microenvironments. In contrast, the type I pathway can efficiently generate ROS through electron or hydrogen transfer, even under severely hypoxic conditions. Nevertheless, the reported number of PSs predominantly associated with the type I pathway is limited, and pure type I PSs are relatively rare. The four primary ROS include O2−•, H2O2, 1O2, and OH• (Table 1), along with other ROS such as ozone (O3), alkoxy radical (RO•), alkyl peroxyl radical (ROO•), and hydroperoxide (ROOH•).
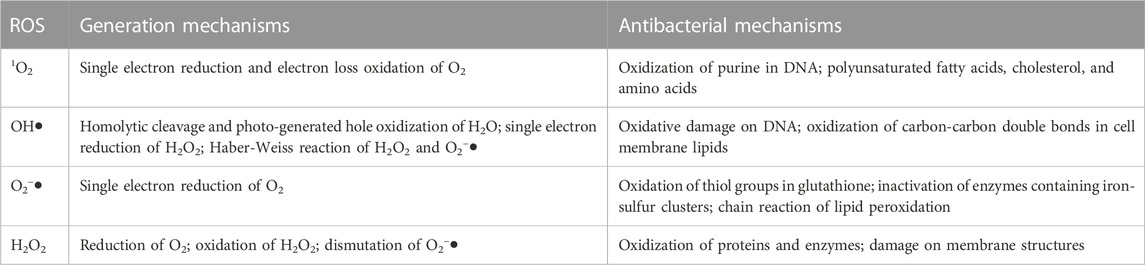
TABLE 1. The summary of four main ROS in generation mechanisms and antibacterial mechanisms during PDT.
The antibacterial effects of ROS manifest as oxidative damage, leading to the disruption of antioxidant defense mechanisms within bacterial cells (Tu et al., 2022). ROS can react with DNA, enzymes, proteins, cell membranes, and cell walls, inducing damage and bacterial death (Song et al., 2022). Although ROS exhibit high reactivity, their lifespan is limited, and their concentration in aquatic systems remains low owing to environmental factors and inherent structural characteristics (Chen et al., 2021). With the exception of the relatively stable H2O2, other ROS can only be detected within a submillisecond timeframe. Nonetheless, they play a crucial role in photodynamic antibacterial processes. Thus, it is imperative to investigate the production processes and antibacterial mechanisms of each type of ROS (Figure 2). This section primarily focuses on the four major types of ROS and elucidates their roles in antibacterial processes.
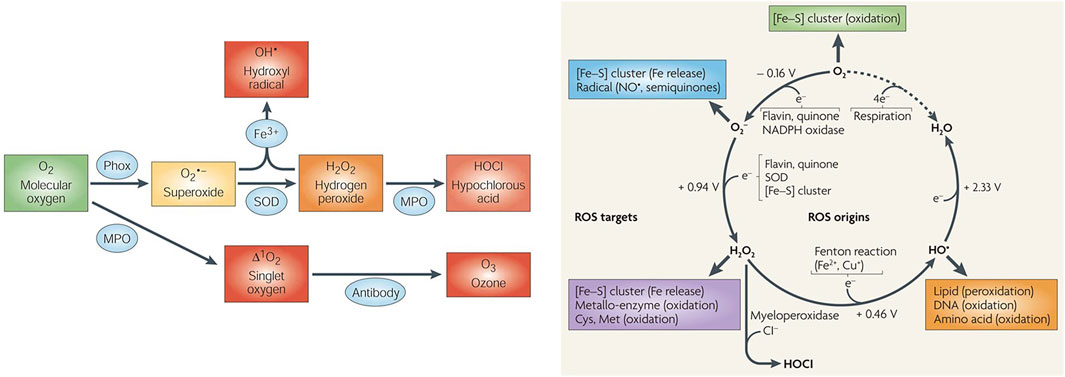
FIGURE 2. Primary types of ROS, the chemistry of ROS in nature, and the interactions of ROS with materials or biomolecules (Yang et al., 2019).
2.2 Singlet oxygen
The generation of 1O2 occurs through the reaction between the ground-state oxygen and PSs. 1O2 represents the excited form of oxygen obtained through the absorption of energy from the 3s* PSs. It exists in two metastable states: 1Δg and 3Δg. In the 1Δg state, the energy of 1O2 is 22 kilocalories higher than that of the ground state, whereas the energy of the 3Δg state is 37 kilocalories higher than that of the ground state. However, the 3Δg state rapidly deactivates in aqueous solutions, hence the primary excited species responsible for PDT is considered to be the 1Δg state (Di Mascio et al., 2019). The production of 1O2 involves three processes: the adsorption of molecular oxygen on the surface of PSs, single-electron reduction, and electron-loss oxidation (Duan et al., 2022). The oxidation-reduction capacity of photo-generated charge carriers represents the rate-determining step in this process. Ordinary molecular oxygen with two parallel electrons in its outermost orbital exhibits strong spin inhibition, which hinders its oxidation. However, excited 1O2 possesses two parallel electrons with opposite spin characteristics, thereby overcoming the inherent inhibition and displaying heightened reactivity (Di Mascio et al., 2019).
1O2 exhibits significant reactivity towards electron-rich organic molecules, including alkenes, dienes, and polycyclic aromatic compounds (Murotomi et al., 2023). Many biological molecules also contain susceptible sites for interaction with 1O2, such as purine bases in DNA, polyunsaturated fatty acids, cholesterol, and certain amino acids (tryptophan, tyrosine, histidine, methionine, and cysteine) (Borisov et al., 2021). 1O2 functions as a highly reactive form of ROS with pronounced electrophilic properties, enabling the oxidation of unsaturated fatty acids, nucleic acids, proteins, and mitochondrial membranes, ultimately leading to cell death (Younis et al., 2021). However, the efficacy of type II PDT is influenced by oxygen availability. At low oxygen concentrations, the generation of a substantial amount of 1O2 is impeded. This limitation is particularly relevant within the body where oxygen is rapidly consumed during type II PDT, frequently resulting in hypoxia and posing challenges in achieving the desired therapeutic outcomes.
2.3 Hydroxyl radical
OH• represents one of the most reactive ROS with the capability to oxidize a wide range of organic compounds derived from the homolytic cleavage of water (Chatgilialoglu et al., 2021). Initially, the capture of water molecules by the PSs at the active sites leads to the generation of surface hydroxyls, which serve as crucial intermediates in the production of OH•. Subsequently, photo-generated holes in the valence band of the PSs oxidize all the surface hydroxyl groups to OH•. Furthermore, OH• can also be formed through the single-electron reduction of H2O2 or the Haber-Weiss reaction involving H2O2 and O2−•.
OH• has an extremely short lifetime, with an estimated lifespan of only about 4 × 10−5 ms in aqueous solutions. Based on this, the diffusion distance is estimated to be only a few molecules in diameter. However, it is noteworthy that the oxidation potential of the OH•/H2O pair is very high, at around +2.30 V (Chatgilialoglu et al., 2021). Because of its strong oxidizing nature, OH• can rapidly capture electrons from almost any adjacent molecule. The most common reaction mediated by OH• is hydrogen abstraction, leading to the formation of H2O and substrate radicals (R•). For example, OH• can trigger lipid peroxidation by reacting with the pentadiene in unsaturated fatty acids to form ROO• via hydrogen abstraction (Chen et al., 2021). In addition, OH• can rapidly interact with various metal cations via electron-transfer reactions. Owing to its powerful oxidizing properties, the development of OH•-generating PSs has received increasing attention with the ultimate aim of improving the efficacy of PDT.
OH• is one of the most potent oxidants in biological systems. It exerts direct oxidative damage on DNA, leading to immediate “site-specific” damage (Xu et al., 2019). Extensive investigations have elucidated the mechanism underlying DNA damage induced by OH•. Specifically, OH• can oxidize guanine residues, resulting in the formation of 8-oxo-7-hydrodeoxyguanosine, which can be incorporated into the DNA strand and paired with adenine, ultimately causing DNA strand breaks, site-specific mutations, and distortions in the double-helix structure (Fleming and Burrows, 2020). Such localized damage is not limited to DNA, but also extends to other biological molecules, including proteins, giving rise to diverse reaction products. Furthermore, the oxidation of unsaturated fatty acids by OH• leads to the generation of aldehyde compounds such as malondialdehyde, which can damage proteins and DNA (Ding et al., 2000). OH• can also affect the cell surface by oxidizing the structural components of the cell membrane or cell wall. For instance, the reaction between OH• and unsaturated fatty acids present in the cell membrane triggers lipid peroxidation, thereby altering membrane properties (Liu et al., 2022). Additionally, OH• functions as an oxidant by reacting with carbon-carbon double bonds in the cell membrane lipids, resulting in the production of H2O2. This facilitates the entry of soluble metal ions or oxidative molecules into the cell, thereby exacerbating ROS-mediated lipid peroxidation, ultimately leading to cell death (Wang et al., 2020). It is important to note that extracellular OH• encounters challenges in penetrating the cell interior owing to its charged nature.
2.4 Superoxide anion radical
In aqueous solutions, O2−• is primarily generated through a three-step process involving the single-electron reduction of O2. This process occurs at the defect sites on PSs and involves adsorption, dissociation, and reduction (Chisté et al., 2015). O2−• exhibits the characteristics of both free-radical chain reactions and anionic charge interactions. Its highly reactive single electron provides it with a high oxidation potential of +2.2 V, rendering it an effective oxidizing agent (Jie et al., 2022). Owing to its strong affinity for accepting electrons, the quantum yield of O2−• is exceptionally high during the initial stages of the photodynamic reactions.
Previous studies have reported that the lifetime of O2−• in aqueous solutions is approximately 50 ms and this duration can be extended by higher pH and lower concentrations. The diffusion distance of O2−• in aqueous solutions is limited to about 40 μm due to its relatively short lifetime (Teng et al., 2021). O2−• can participate in the Haber-Weiss reaction or Fenton reaction, leading to the generation of OH•, which possesses higher biological activity than O2−•. Consequently, these reactions can be exploited to enhance the effectiveness of O2−•-based PDT. Intracellular superoxide dismutase plays a significant role in catalyzing the dismutation reaction of O2−•, resulting in the production of H2O2 and O2 (Li et al., 2018). The accumulated H2O2 can subsequently convert into OH•, thereby intensifying oxidative damage and improving therapeutic outcomes. Another advantage is the recyclability of O2 in these interconnected biological reactions, which contributes to its anti-hypoxia performance (Zhu et al., 2022). Consequently, exogenous O2−• generators hold promise as compelling avenues for developing novel hypoxic therapeutic strategies.
O2−• serves as the primary oxidant in PDT. It exerts deleterious effects on the cellular components by reacting with proteins, DNA, and lipids, leading to irreversible damage and metabolic disturbances. For instance, the excessive presence of O2−• induces the robust oxidation of thiol groups in glutathione, resulting in the formation of disulfide bonds (Winterbourn, 2016). These disulfide bonds critically impair microbial antioxidant systems, exposing cells to a toxic milieu and causing cellular injury. Moreover, O2−• readily oxidizes enzymes containing iron-sulfur clusters in the dehydrogenase family, leading to inactivation. For instance, in E. coli (Escherichia coli), O2−• reacts with iron-sulfur-containing enzymes, inhibiting essential metabolic processes such as the tricarboxylic acid cycle and amino acid biosynthesis (Imlay, 2019).
However, under neutral conditions, O2−• cannot penetrate the cell membrane because of the repulsion between the charges. However, in acidic environments, the oxidation-reduction potential of protonated O2−• becomes more positive, resulting in its transformation into a hydrophobic and neutral species called HO2•. This altered form of O2−• can traverse the cell membrane and accumulate in the hydrophobic region. Consequently, a lipid peroxidation chain reaction is initiated by HO2•, leading to the inactivation of free radical-dependent enzymes (Li et al., 2022). This cascade of events severely compromises biological functions within an organism and ultimately culminates in cell death.
2.5 Hydrogen peroxide
H2O2 is primarily generated by the reduction of O2 and the oxidation of H2O. Another alternative pathway for H2O2 production involves the dismutation of O2−•, which is common in biological systems. Among all ROS, H2O2 exhibits the greatest stability and can persist in aqueous solutions for approximately 10 h (Li et al., 2022). Because of its thermodynamic stability and extended lifespan, H2O2 serves as the principal agent responsible for maintaining the long-lasting antibacterial activity of PDT) Unlike O2−•, H2O2 is a neutral molecule capable of traversing cell membranes. As a small and diffusible molecule, it can penetrate deep into the cells and interact with various proteins, thereby impeding normal cellular functions and ultimately resulting in cell death. For instance, H2O2 can oxidize iron-sulfur clusters present in dehydrogenases. Additionally, H2O2 can oxidize ferrous ions within mononuclear iron enzymes, affecting critical metabolic pathways, such as the pentose phosphate pathway (Winterbourn, 2013).
Because the oxidation potential of H2O2 is relatively low, it is often converted into other highly active ROS for efficient utilization (Sun et al., 2020). The reaction between H2O2 and the cellular iron pool produces OH•, which directly oxidizes most cellular biomolecules including DNA. OH• triggers a series of oxidative chain reactions, attacks membrane structures, exacerbates lipid peroxidation damage, and allows free radicals to enter the cell (Lennicke and Cocheme, 2021). However, the long-term effectiveness of H2O2 under physiological conditions needs to be explored because of its decomposition by endogenous antioxidant enzymes induced by cellular oxidative stress.
3 The antibacterial application of nanomaterials in PDT
Although antibiotics are widely used for the treatment of bacterial infections, their inappropriate usage can result in significant adverse effects, including drug allergies, central nervous system damage, and the emergence of drug-resistant bacterial strains (Pang et al., 2019). PDT has several advantages over traditional antibiotic therapies. PDT is associated with minimal serious adverse effects and does not contribute to the development of drug-resistant bacterial strains. It can be repeated as required, highlighting its versatility and therapeutic potential.
PDT requires three fundamental components: PSs, light, and oxygen. The overall process involves multiple intricate steps, including energy transfer, proton transfer, rearrangement, fluorescence, internal conversion, and intersystem crossing, culminating in the generation of biologically active ROS mentioned above (Lan et al., 2019). The specific properties of the PSs play a pivotal role in determining the type and quantity of ROS produced, thereby directly influencing the antimicrobial efficacy of PDT. Consequently, the careful selection of suitable PSs is of paramount importance. Although various organic small-molecule materials such as porphyrins, synthetic dyes, and natural products have clinical applications, they inherently possess several disadvantages. These drawbacks include poor water solubility, inadequate light stability, uncontrolled drug release, limited selectivity, and low extinction coefficients (Zhou et al., 2021). These shortcomings detrimentally affect ROS production, resulting in diminished efficacy against infections and impeding their widespread clinical use. Therefore, the development of novel PSs capable of overcoming the current limitations is essential for enhancing therapeutic outcomes in combating infections.
To advance the development of PDT in the antibacterial field, the use of nanomaterials as carriers or PSs has been explored (Figure 3). Nanomaterial-based carriers or PSs offer enhanced water solubility and controlled release capabilities, leading to an increased production of ROS (Yang et al., 2019). Notably, nanomaterials can selectively accumulate within infected tissues via enhanced permeability and retention (EPR) effect, thereby facilitating targeted delivery and aggregation at the site of infection. This phenomenon holds significant promise for augmenting therapeutic interventions (Shekhar et al., 2022).
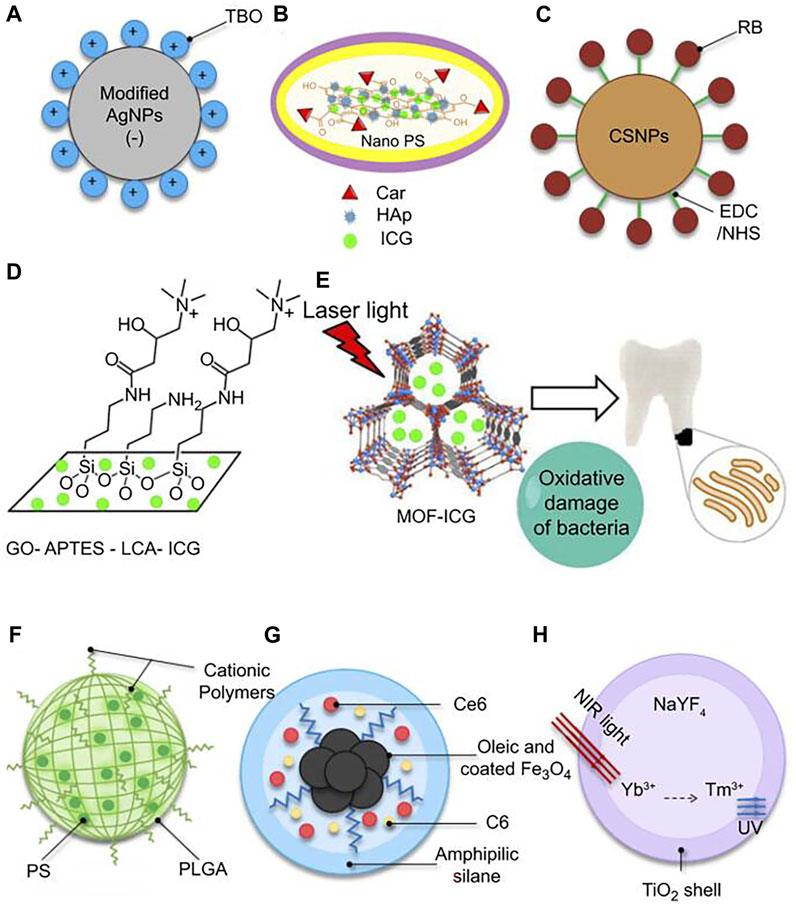
FIGURE 3. Different types of nanomaterials are used as antibacterial agents during the photoconductive process (Qi et al., 2019).
Although extensive research has been conducted on the application of nanomaterial in cancer treatment, their use in antibacterial therapy remains relatively limited. In pursuit of a non-antibiotic-based approach and expanding the scope of PDT, efforts are underway to develop novel nanomaterials as carriers or agents. This section presents a comprehensive overview of various types of nanomaterial-based carriers or PSs (Table 2) that offer innovative concepts for antibacterial therapy.
3.1 Carbon-based nanomaterials
Carbon-based nanomaterials have attracted significant attention in the field of biomedicine. These materials are characterized by π-hybridized carbon atoms, which are typically arranged in hexagonal or pentagonal lattices. The extensive aromatic rings formed by the sp2-hybridized carbon atoms impart exceptional light absorption properties to carbon-based nanomaterials (Ma et al., 2022). Owing to their distinctive physical and chemical attributes, researchers have explored their potential applications in PDT, encompassing various carbon-based nanomaterials, such as fullerenes, graphene and its derivatives, and carbon nanotubes.
Fullerenes are carbon molecules that exhibit a soccer-ball structure composed of sp2-hybridized carbon atoms. The extended-conjugation in fullerenes prolongs the lifetime of the triplet excited state (3s*), enabling the generation of a substantial amount of ROS upon light irradiation (Hashikawa et al., 2022). The specific ROS produced by fullerenes vary depending on the solvent used. In polar solvents, fullerenes generate O2−• and OH•, whereas in nonpolar solvents, the primary ROS generated is 1O2. Fullerenes possess several other advantageous features including high photostability, facile functionalization, and the ability to participate in both type I and type II photodynamic reactions. Numerous studies have reported the broad-spectrum antibacterial photodynamic activities of fullerenes and their derivatives (Hou et al., 2022). For instance, a novel C70-based PS was synthesized through a one-step thiol reaction capable of generating a significant amount of ROS via both type I and type II mechanisms, ultimately leading to the disruption of bacterial cell membranes. These fluorescent C70-COOH nanoparticles can inactivate multiple strains, including methicillin-resistant S. aureus (MRSA) (Tan et al., 2022). Furthermore, composite materials based on fullerenes have demonstrated remarkable photodynamic activities. Researchers have developed a porphyrin-fullerene dyad (TCP-C60) polymer film on an indium tin oxide (ITO) electrode that effectively generated 1O2 and O2−•, leading to a significant reduction in the survival rates of both S. aureus (Staphylococcus aureus) and E. coli (E. coli) (Ballatore et al., 2015). Current research efforts are focused on enhancing the water solubility of fullerenes using functionalization strategies. The introduction of other functional groups (COOH•, OH•, and NH2•) into fullerenes improves their solubility in water and enhances their ROS generation capacity. For instance, water-soluble C60(OH)30 fullerene alcohol was synthesized, which exhibited a high O2−• quantum yield of 0.89, thereby demonstrating a remarkable ROS production capacity and therapeutic effects against multidrug-resistant bacteria (Kuo et al., 2020a). Tegos et al. compared the antibacterial activities of six functionalized C60 compounds and observed that cationic fullerene derivatives exhibited broad-spectrum antibacterial activity under visible-light irradiation, surpassing the antibacterial activity of neutral diene-modified fullerenes. Cationic modification of fullerenes increased their positive charge, facilitating their binding to bacteria and enhancing their cellular permeability (Tegos et al., 2005).
Graphene and its derivatives have gained significant interest in the biomedical field because of their unique properties, such as diverse functional groups, large surface area, and excellent dispersion stability, making them promising materials for various biomedical applications (Yang et al., 2022). Specifically, graphene oxide (GO) and reduced graphene oxide (rGO) have emerged as notable graphene-derived nanomaterials that exhibit considerable potential in drug delivery for PDT owing to their distinctive structures and physicochemical characteristics (Xia et al., 2019). For instance, the silver-graphene oxide (Ag-GO) composite nanomaterial was prepared through the in situ reduction of Ag+ on GO nanosheets, followed by encapsulation with type I collagen (Figure 4). In vivo experiments demonstrated that 20 min of 660 nm visible light irradiation resulted in antibacterial ratios of 96.3% and 99.4% against E. coli and S. aureus on the surface of implants, respectively (Xie et al., 2017). In addition, traditional drug-based PSs can be targeted for drug delivery using graphene-based nanomaterials. Indocyanine green (ICG) was successfully loaded onto the GO nanosheets with encapsulation rates as high as 92.31%. Under near-infrared (NIR) irradiation, the antibacterial efficiency of indocyanine green-graphene oxide (ICG-GO) against MRSA reached 99.86%. NIR irradiation facilitated the activation of both the photothermal and photodynamic effects of ICG-GO, leading to optimal ROS production and a great potential for antibacterial therapies (Chen et al., 2022).
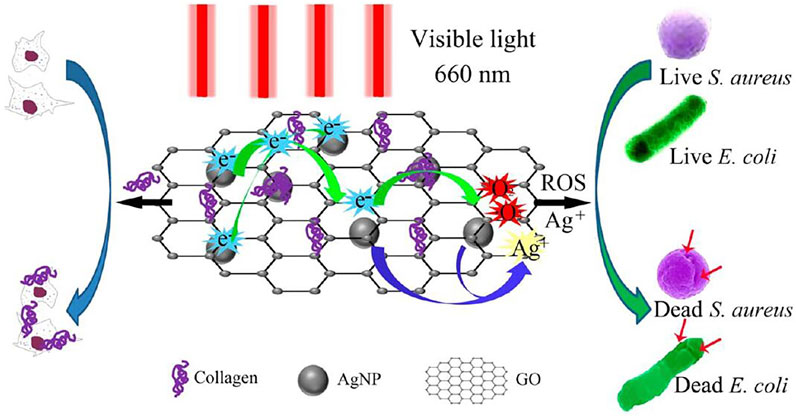
FIGURE 4. The schematical illustration of GO/AgNPs/collagen hybrid coating for photodynamic antibacterial treatment (Xie et al., 2017).
Graphene quantum dots (GQDs), which are graphene nanoparticles smaller than 100 nm, have also gained attention as a novel nanomaterial for biomedical applications due to their favorable biocompatibility, stable photochemical properties, and noticeable quantum confinement effect (Henna and Pramod, 2020). Generally, as the number of carbon atoms per layer or the total number of layers increases, the band gap of GQDs decreases (Chung et al., 2021). A study reported the significant antibacterial activity of GQDs against S. aureus and E. coli, as evidenced by a substantial decrease in bacterial colony count upon co-culture, highlighting the potential of GQDs as antibacterial agents in PDT (Ristic et al., 2014). Functionalization of GQDs, such as nitrogen-doped GQDs (N-GQDs) or amino-functionalized N-GQDs, is a current research direction. Amino-functionalized N-GQDs exhibited notable antibacterial activity in the photodynamic process, with the bonding of amino and nitrogen functional groups playing a crucial role in the antibacterial effect (Kuo et al., 2020). Furthermore, chemically reduced graphene oxide quantum dots (rGOQDs) demonstrated enhanced ROS generation, surpassing the ROS yield of GOQDs under visible light irradiation, including O2−•, H2O2, and 1O2. The lower band gap of rGOQDs facilitated the generation of a higher number of electron-hole pairs, thereby yielding increased ROS production (Zhang et al., 2018). Combining GQDs with other nanomaterials for PDT also holds great potential. Composite nanomaterials consisting of GQDs coupled with silver nanoparticles (Ag NPs) have been employed as an enhanced therapy for tri-modal antibacterial treatment. The incorporation of Ag NPs onto the surface of GQDs significantly enhanced ROS production while enabling photothermal therapy (PTT) through efficient conversion of light energy. After only 10 min of 450 nm laser irradiation, GQD-Ag NPs exhibited effective bactericidal activity against both Gram-negative and Gram-positive bacteria through the combined action of photodynamic ROS and photothermal high temperatures (Yu et al., 2020). Moreover, GQDs loaded with curcumin have also been investigated, showing significant antibacterial effects against P. aeruginosa (Pseudomonas aeruginosa), MRSA, and E. coli under 405 nm blue light irradiation (Mushtaq et al., 2022).
Carbon nanotubes (CNTs) represent a distinct class of carbon-based nanomaterials in which a single layer of graphene is rolled into a cylindrical tube-like nanostructure, exhibiting a high length-to-diameter ratio and large surface area (Negri et al., 2020). CNTs can be categorized as single-walled CNTs (SWCNTs) and multi-walled CNTs (MWCNTs). The remarkable specific surface area of CNTs endows them with exceptional capabilities for PSs immobilization, which is pivotal for drug delivery systems and ROS generation. Sah et al. investigated the photodynamic effects of amine-functionalized porphyrin-conjugated MWCNTs on S. aureus cell membrane damage under visible light irradiation (Sah et al., 2018). In PDT, the generation of highly selective 1O2 is critical owing to its limited lifetime and diffusion distance. To address this, researchers have explored the antibacterial activity of methylene blue-conjugated carbon nanotubes (MBCNTs) against E. coli and S. aureus biofilms using a 670 nm laser. MBCNTs exhibited significant bactericidal effects against both planktonic bacteria of E. coli and S. aureus under laser irradiation. Furthermore, biofilm inhibition and extracellular polymeric substance (EPS) reduction assays revealed that the composite nanomaterials exerted stronger inhibitory effects on both S. aureus and E. coli (Parasuraman et al., 2019). Another approach involves the construction of malachite green-coupled carboxy-functionalized multi-walled carbon nanotubes (MGCNTs) to enhance the PDT effect against P. aeruginosa and S. aureus. Both planktonic bacteria and biofilm experiments have validated the sensitivity of MGCNTs to PDT, suggesting their potential as a simple strategy for designing antibacterial and antibiofilm coatings (Anju et al., 2019).
3.2 Metal nanomaterials
Gold (Au) nanomaterials exhibit diverse properties, such as tunable optical characteristics, high chemical inertness, significant catalytic activity, and excellent biocompatibility (Figure 5) (Zhao et al., 2022). They exist in various forms, including nanoparticles, nanorods, nanoclusters, and nanoshells (Usman et al., 2023). Gold nanomaterials possess strong localized surface plasmon resonance (LSPR) properties that can lead to significant photodynamic effects (Zhou et al., 2021). Among the ROS generated by the photodynamic effect, gold nanomaterials can be directly activated to produce a high concentration of 1O2, making them potential candidates for PDT in the antibacterial field (Younis et al., 2021).
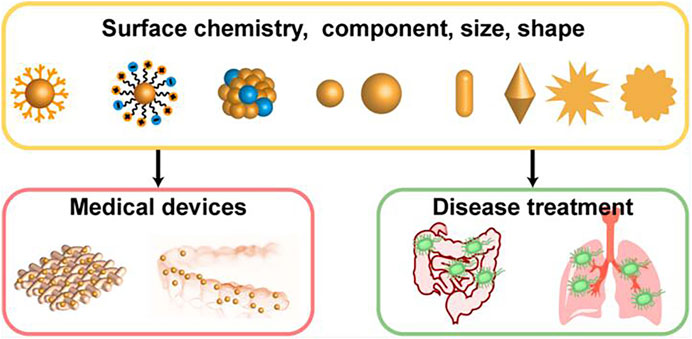
FIGURE 5. The multiple applications of Au-based nanomaterials in combating drug-resistant bacteria (Zhao et al., 2022).
Raviraj et al. discovered that metal nanoparticles can generate 1O2 through a photodynamic process, making them suitable PSs. They synthesized three metal nanoparticles with different structures: Au, Ag, and Pt. These nanoparticles exhibited strong LSPR bands ranging from 398 to 530 nm. When exposed to light within a specific wavelength range, the LSPR of these metal nanoparticles facilitated energy transfer to molecular oxygen, resulting in ROS generation. Researchers have found that the production of 1O2 was highly dependent on the morphology of metal nanoparticles. The Ag nanocubes and Au triangular slabs produced substantial amounts of 1O2 when irradiated at 885 nm, whereas the Au nanocubes yielded negligible 1O2. Mechanistic studies have revealed that the morphology-dependent ROS generation in these nanomaterials can be attributed to the selective binding of molecular oxygen to specific crystal planes (Vankayala et al., 2011). Furthermore, the yield of 1O2 was significantly influenced by the size of the Au NPs. Larger Au NPs generated more 1O2 than smaller Au NPs within a size range of 50 nm. This phenomenon was attributed to the increased generation of hot electrons by the larger Au NPs compared to the smaller ones. The study also demonstrated that in the presence of Au NPs, the photooxidation rate of 9,10-anthracene di(methylene) dimalonic acid by an 808 nm laser was faster than that of indocyanine green or rose bengal. These findings indicate that the two-photon-induced oxygen generation ability of the Au NPs surpasses that of traditional organic PSs (Chadwick et al., 2016).
Recent research has focused on the synthesis of a novel PS consisting of lysozyme-gold nanoclusters/rose bengal (Lys-Au NCs/RB). The antibacterial activity of Lys-Au NCs/RB was significantly enhanced owing to the synergistic effect of resonance energy transfer (RET) associated with Lys in the Au NCs/RB conjugates, which facilitated enhanced 1O2 generation. Photoexcitation of Lys-Au NCs/RB successfully inhibited the formation of S. mutans biofilms (Okamoto et al., 2021). Similarly, other researchers have developed captopril-encapsulated gold nanoclusters (Au25Capt18) using the alkaline NaBH4 reduction method and incorporated them into carrageenan to achieve a dual-mode antibacterial effect through photothermal therapy (PTT) and PDT. Au25Capt18 exhibited excellent thermal effects and 1O2 generation under near-infrared (NIR) light. In vivo experiments confirmed the potent antibacterial effect of the nanomaterial, which promoted wound healing in the S. aureus-infected model. The Au25Capt18-embedded hydrogel exhibited favorable properties, such as water retention, hemostasis, and air permeability, creating a suitable environment for wound healing (Zheng et al., 2023).
Gold nanomaterials have been extensively investigated as promising candidates for PDT; however, silver ions are challenging to reduce to silver nanomaterials and exhibit poor environmental stability. Consequently, controlling ROS generation using Ag nanomaterials has received less attention. Mechanistic studies demonstrated that most Ag NPs undergo intersystem crossing to the triplet state upon photoexcitation. Subsequent transitions to the triplet excited state significantly prolong the lifetime of the electrons, thereby enhancing the excitation ability of the oxygen molecules (Hakimov et al., 2022). Currently, Ag is primarily used as a carrier or enhancer in PDT.
An antibacterial composite membrane was fabricated by electrospinning to produce nanofibers composed of curcumin and Ag NPs. The antibacterial properties of Ag NPs, combined with their metal-enhancing effect, enhanced the production of 1O2 by curcumin. The curcumin@Ag core/shell fiber membrane exhibited a high antibacterial rate of up to 93.04% against S. aureus (Wang et al., 2022). In addition, Ag NPs can enhance the photodynamic antibacterial activity of metal oxides. Ag/Ag@AgCl nanostructures were synthesized within a hydrogel via ultraviolet (UV) photochemical reduction, followed by the incorporation of zinc oxide (ZnO) nanostructures via NaOH precipitation. Ag/Ag@AgCl nanostructures augmented the photodynamic and antibacterial activities of ZnO. Under visible-light irradiation, this hydrogel system achieved a bactericidal efficiency of 95.95% against E. coli and 98.49% against S. aureus within 20 min, demonstrating remarkable antibacterial efficacy (Mao et al., 2017). The silver-assisted enhancement can also be applied to other nanomaterials. Researchers constructed a composite nanomaterial based on quaternary ammonium salt chitosan (QCS)/silver (Ag)/cobalt phosphide (CoP) for rapid antibacterial induction using NIR. Ag NPs were uniformly deposited on the CoP nanoneedles, with a layer of QCS coating the surface of the CoP nanoneedles. Under NIR irradiation, the high-energy hot electrons generated by Ag were transferred to the interface between Ag and CoP, thereby amplifying the photothermal effect of CoP. Furthermore, owing to the Schottky heterojunction structure, Ag loading enhanced the photodynamic effect of CoP, resulting in a synergistic antibacterial activity. The QCS/Ag/CoP ternary nanocomposite achieved a sterilization rate exceeding 99.6% against S. aureus and E. coli within 10 min of NIR irradiation (Han et al., 2022).
3.3 Metal oxide nanomaterials
Metal oxides exhibit desirable characteristics such as enhanced photostability, high extinction coefficients, excellent quantum yields, and enhanced surface activity, making them widely applicable in the field of biomedicine (Balhaddad et al., 2021). Among the metal oxides, semiconductor materials such as titanium dioxide (TiO2) and zinc oxide (ZnO) have demonstrated the ability to directly generate ROS upon light irradiation (Alavi et al., 2022). The band-edge structure of metal oxides is regarded as a crucial factor in ROS generation (Saied et al., 2022).
The TiO2 NPs exhibit excellent photostability and biocompatibility. When exposed to UV irradiation, TiO2 NPs generate abundant ROS, which possess potent bactericidal effects and can be directly employed as PSs in PDT (Iwatsu et al., 2020). The generation of ROS by TiO2 is attributed to charge separation and surface oxidation-reduction reactions. Because of the high oxidation potential of TiO2, species such as H2O and OH− adsorbed on its surface can be readily oxidized, resulting in the generation of OH•. Furthermore, TiO2 can directly transfer electrons to acceptors such as O2 and H+, leading to the production of O2−• and H2O2. In addition, the production of 1O2 by TiO2 was observed in gated photon-counting experiments under light irradiation. The quantum yield of 1O2 decreased as the particle size exceeded 20 nm, providing insights into the precise control of nanomaterials (Nosaka and Nosaka, 2017).
In a recent study, TiO2 nanorod arrays were successfully fabricated, and their combination with 808 nm NIR light demonstrated simultaneous photothermal conversion and ROS generation capabilities (Figure 6). These TiO2 nanorod arrays effectively eradicated bacterial biofilms and treated biofilm infections in bones through synergistic antibacterial effects, while promoting new bone formation around the implant (Zhang et al., 2021). Synergistic antibacterial effects can also be achieved by using composites of TiO2 and other nanomaterials, resulting in enhanced photodynamic antibacterial effects. Another study has focused on the inhibitory effects of adhesive-containing TiO2 NPs on oral bacterial biofilms. The TiO2/hydroxyapatite (TiO2-HAP) composite material inhibited Streptococcus mutans (S. mutans) biofilm formation on teeth when exposed to dental curing light (385–515 nm) and facilitated the surface remineralization of tooth enamel. Notably, sustained photodynamic activity was observed even after the termination of UV irradiation (Wang et al., 2022). In another study, a TiO2/Ag film was synthesized using atomic layer deposition and reduction methods. In vitro antibacterial experiments demonstrated that, after 5 min of light irradiation, the TiO2/Ag film exhibited antibacterial rates of 98.2% and 98.6% against S. aureus and E. coli, respectively. The formation of Schottky junctions at the interface of TiO2 and Ag reduced the band gap of TiO2 from 3.44 eV to 2.61 eV, facilitating enhanced ROS generation for bacterial eradication (Cai et al., 2022). Furthermore, a recent study has proposed a ligand-to-metal charge transfer (LMCT)-mediated TiO2 photodynamic strategy. Inspired by mussels, researchers have developed mesoporous TiO2@PDA nanoparticles (mTiO2@PDA NPs) using polydopamine (PDA). The tightly bound PDA formed an LMCT bridge with TiO2, enabling 808 nm NIR to activate TiO2 and generate ROS. The mTiO2@PDA NPs demonstrated antibacterial efficacy under NIR irradiation without damaging healthy tissues, making them promising nanomaterials for the treatment of infections and rapid healing of infected wounds (Cheng et al., 2023).
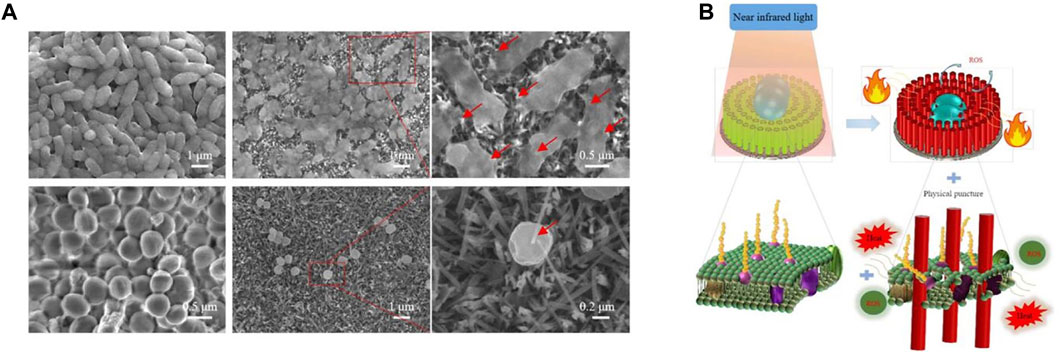
FIGURE 6. The antibacterial mechanism of the TiO2 nanorod arrays against E. coli and S. aureus (Zhang et al., 2021).
ZnO is a semiconductor metal oxide with unique optical properties that can produce ROS upon UV excitation via electron transfer, thereby exerting antimicrobial effects. Recent studies have highlighted the potential of ZnO NPs loaded resin composites for PDT in the management of dental caries (Zhu et al., 2022). The findings demonstrated that the addition of 20 wt% ZnO NPs had a minimal impact on the shear bond strength and flexural strength of dentin while effectively reducing bacterial populations. Thus, resin-based restorative materials loaded with ZnO NPs have promising applications in dentistry (Comeau et al., 2022). This structure can also influence the properties of nanomaterial-based PSs. Nanoflowers, a novel class of nanomaterials characterized by flower-like structures, have garnered significant attention owing to their high stability, facile synthesis, and high efficiency. In a recent study, ZnO nanoflowers were synthesized using both hydrothermal (ZnO-NF1) and chemical methods (ZnO-NF2). A comparative analysis of the two ZnO nanoflowers revealed their photodynamic efficiencies, antibacterial performances, and microbial cell viabilities. Both ZnO-NF1 and ZnO-NF2 demonstrated remarkable photodynamic antibacterial effects; however, ZnO-NF2 exhibited higher activity across all concentrations, underscoring the significance of a precise synthesis route design for nanomaterials (Eskikaya et al., 2022).
Surface modifications, such as doping with molecules, can significantly enhance the antibacterial efficacy. Cobalt-doped zinc oxide nanoparticles (Co-ZnO) coated with chitosan can overcome multiple drug resistance mechanisms in bacteria by inhibiting efflux pumps and exerting photodynamic effects. Notably, the 10 μg/mL concentration of Co-ZnO achieved complete eradication of MRSA following 15 min of visible light irradiation. Furthermore, cobalt doping enhanced the photodynamic and photothermal activities of Co-ZnO, while chitosan effectively blocked the efflux pump of MRSA, facilitating the effective targeting of drug-resistant bacteria through multiple synergistic mechanisms (Iqbal et al., 2019). In another study, Au@ZnO@SiO2-ICG nanocomposites were investigated for their potential use as antibacterial agents. The metal/semiconductor heterostructure of Au@ZnO exhibited a synergistic effect under UV irradiation, regulating the transfer of interfacial electrons and enhancing ROS generation in PDT. The results indicated that Au@ZnO@SiO2-ICG nanomaterials displayed excellent antibacterial properties against both S. aureus and E. coli (Liu et al., 2022).
3.4 Metal-organic frameworks
Organic-inorganic hybrid materials have emerged as promising PSs in the field of nanomedicine. Among these materials, metal-organic frameworks (MOFs) represent an advanced class characterized by porous crystalline structures formed through the self-assembly of organic and inorganic units via covalent bonds. The unique structural attributes of MOFs, including their large surface area, controllable size, and excellent crystallinity, enable the efficient encapsulation of other molecules with high loading capacity (Yang and Yang, 2020). In contrast to conventional inorganic nanomaterials, MOFs offer the advantage of tunable structural compositions and chemical properties at the molecular level while retaining desirable biocompatibility. Over the past decade, numerous MOFs with diverse metal centers and organic ligands have been synthesized, resulting in the development of numerous distinct MOF structures.
The structural properties of MOFs confer significant value for medical applications. Recently, Fe2O3-modified 2D porphyrin MOF nanosheets were designed and synthesized. The presence of metal-connecting bridging units in the nanosheets led to a reduction in the adsorption energy at the interface and an increase in charge transfer, thereby enhancing the photodynamic activity. Through the synergistic effects of ROS and ion release, nanosheets demonstrated broad-spectrum antibacterial activity against various oral pathogenic bacteria (Li et al., 2021). In another study, a zirconium (IV)–benzodiazole-doped MOF (Se-MOF) was synthesized and characterized. The Se-MOF exhibited regular crystallinity and high porosity. Upon visible light irradiation, Se-MOF efficiently generated 1O2 and exhibited pronounced photodynamic effects. Consequently, the Se-MOF inhibited the proliferation of planktonic bacteria and the formation of bacterial biofilms through its photodynamic activity. This study provided a promising strategy for the development of MOFs as PSs for clinical photodynamic antibacterial applications (Luan et al., 2022).
However, the application of MOF-based PDT often faces challenges, such as limited photon absorption and recombination of photo-generated holes with electrons under visible light. To address these limitations, recent studies have focused on developing multifunctional heterostructures by combining MOFs with other nanomaterials. These hybrid structures retain the inherent characteristics of MOFs, while endowing them with additional functionalities. Researchers have designed MOF-based nanosystem that enhanced PDT efficiency by incorporating Ag NPs into MOFs (Figure 7). The Ag NPs@MOFs exhibited remarkable photodynamic effects and efficient separation of photo-generated carriers. Under visible light irradiation, Ag NPs@MOFs primarily disrupted the bacterial translation and metabolism of purines and pyrimidines. Overall, Ag NPs@MOFs demonstrated excellent synergistic antibacterial efficacy through multiple mechanisms, thereby inhibiting bacterial resistance (Xie et al., 2023). In another study, NH2-MIL-101(Fe)@MoS2/ZnO ternary nanocomposites were prepared using a wet chemical method. MoS2 nanosheets were grown on the MIL-101 scaffold, and ZnO was deposited on the surface. Upon visible-light irradiation for 30 min, the NH2-MIL-101(Fe)@MoS2/ZnO nanocomposites exhibited a clearance rate of 98.6% for E. coli and 90% for S. aureus, demonstrating excellent bactericidal performance against both Gram-negative and Gram-positive bacteria. This enhanced bactericidal activity was attributed to the unique electronic band structure of the composite, which facilitated the separation of photo-generated carriers. The interfacial charge-transfer process promoted the generation of O2−• through oxygen reduction and facilitated the formation of OH• through the dismutation reaction of water (Liu et al., 2022).
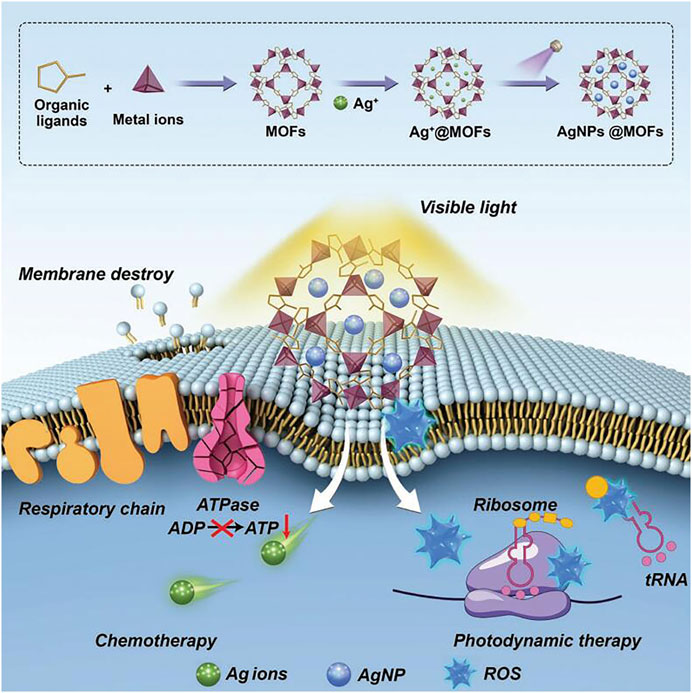
FIGURE 7. The schematic diagram of AgNPs@MOFs nanomaterials combating drug-resistant bacteria through ROS and chemotherapy (Xiu et al., 2022).
3.5 Upconversion nanoparticles
Upconversion nanoparticles (UCNPs) are obtained primarily by doping rare-earth ions into inorganic matrices. The upconversion process involves the conversion of two or more pump photons that are continuously absorbed by the material into high-energy emission photons through radiationless relaxation and transition (Shiby et al., 2022). This process can be attributed to three main mechanisms: excited-state absorption (ESA), energy transfer (ET), and photon avalanche (PA) (Liu, 2015). The unique conversion ability of UCNPs has several advantages, including photostability, the absence of background light interference, and significant penetration depth, thereby rendering them minimally harmful to biological tissues (Sun et al., 2015). To date, UCNPs have exhibited distinctive photochemical properties achieved through size adjustment, lattice modulation, and surface ligand exchange, making them promising materials for therapeutic applications (Figure 8).
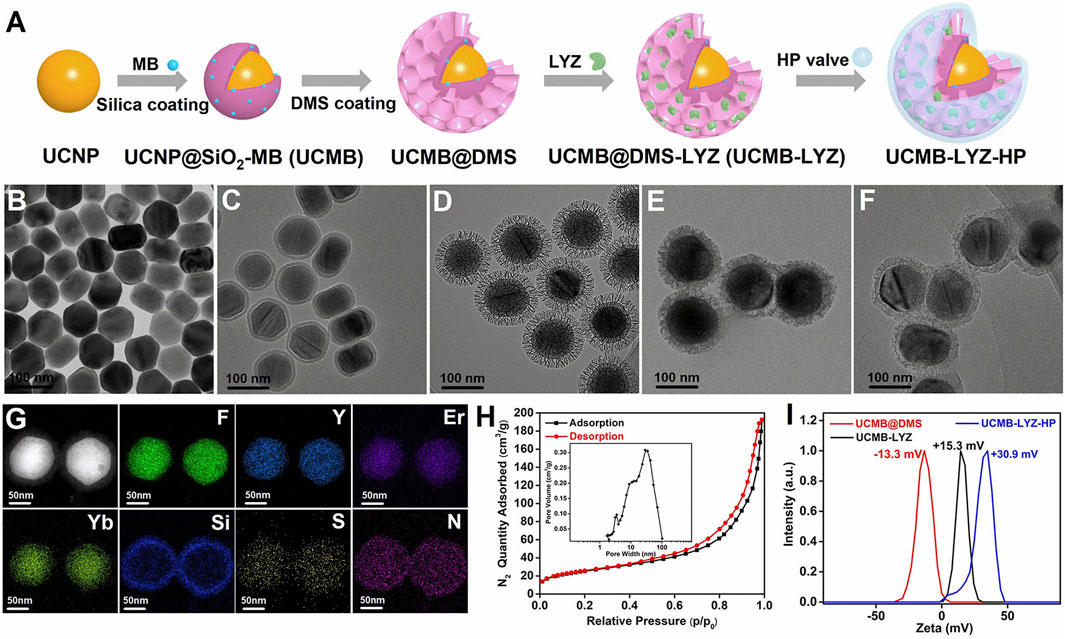
FIGURE 8. The fabrication and characteristics of UCNP-based nanomaterial to treat infection (Li et al., 2021).
In conventional PDT, most PSs can only be activated within the UV or visible light wavelength ranges. However, UCNPs possess the unique ability to sequentially absorb two or more photons under long-wave radiation and convert them into tunable short waves such as UV or visible light emissions. In this context, UCNPs can utilize low-energy NIR radiation as an excitation source that can be converted into the spectrum required for specific PSs. Compared to UV radiation, NIR radiation offers greater penetration depth and causes less tissue damage (Feng et al., 2013). Additionally, UCNPs can be functionalized with PSs or magnetically coated for the targeted treatment of infection sites, thereby enhancing the precision of PDT.
Traditionally, the light absorption of TiO2 has been limited to the UV region, which restricts tissue penetration and may lead to DNA damage or skin cancer. To overcome this limitation, researchers have synthesized core-shell-structured NaYF4:Yb3+,Tm3+@TiO2 nanoparticles (referred to as UCNPs@TiO2). The core of the UCNPs converted NIR light into UV light through upconversion, which further excited TiO2 to generate ROS. Experimental results have demonstrated that UCNPs@TiO2 exhibited excellent antibacterial activity against both planktonic and biofilm states of periodontal pathogens (Qi et al., 2019). In another study, NaYF4:Yb, Tm nanorods were used as the core, TiO2 nanoparticles were used as the shell, and hierarchical UCNPs (UCNPs@TiO2) were synthesized. GO was then doped with UCNPs@TiO2 to obtain UCNPs@TiO2@GO. Subsequently, UCNPs@TiO2@GO were electrospun into a nanocomposite membrane using polyvinylidene fluoride (PVDF). After 5 min of 980 nm NIR irradiation, the membrane exhibited simultaneous production of ROS and temperature elevation, thereby demonstrating the synergistic antibacterial effect of PDT and PTT against both Gram-positive and Gram-negative bacteria (Sun et al., 2019). Moreover, the optical synergy between UCNPs and MOFs can overcome the limitations of each material, ultimately achieving a high drug-loading capacity and broad-spectrum light absorption. Karami et al. (2021) synthesized a composite material by incorporating cetyltrimethylammonium bromide (CTAB)-coated UCNPs into β-NaYF4:Yb/Tm@ZIF-8, which were subsequently transformed into β-NaYF4:Yb/Tm@ZnO nanoparticles via calcination. NIR-activated UCNPs@ZnO exhibited potent bactericidal efficacy against S. aureus and its related mutant forms because of the abundant production of ROS by the newly developed nanoparticles.
4 The limitations of current nanomaterial-based PSs
Nanomaterials have emerged as promising PSs and carriers for photodynamic field of PDT. Successful implementation of PDT relies on the coordinated interplay of three crucial factors: PSs, light sources, and oxygen molecules. However, despite significant advancements, research on nanomaterials in photodynamic antimicrobial therapy encounters various challenges that impede the widespread application of PDT in infection treatment.
First, the applications of nanomaterials are limited by their inherent properties. For instance, fullerenes exhibit low hydrophilicity, a tendency to aggregate, and limited penetration ability (Wilczewska1 et al., 2012). To address these limitations, surface modifications involving the introduction of anionic or cationic functional groups as well as supramolecular approaches, such as PEGylation and encapsulation, have been explored to enhance water solubility. Concerning TiO2, a major obstacle lies in its preferential absorption of UV radiation in the electromagnetic spectrum (Rehman et al., 2016). Most MOFs exhibit high photodynamic efficiencies primarily under UV irradiation. However, prolonged exposure to UV light can have detrimental effects on the surrounding healthy tissue, leading to serious side effects (Totonchy and Chiu, 2014). Additionally, single-phase MOFs often undergo rapid recombination of photo-generated holes and electrons, resulting in reduced PDT efficiency (Caballero-Mancebo et al., 2019). Collectively, the current challenges faced by nanomaterial-based PDT include limited antimicrobial duration, narrow light absorption range, inefficient separation of photogenerated carriers, high recombination rates, and concerns related to biological safety arising from inherent material properties.
A common challenge encountered with nanomaterials is the formation of a protein corona. Upon exposure to physiological fluids, nanomaterials spontaneously adsorb biomolecules, resulting in the formation of a protein corona, which is a significant hurdle for nanomaterial-based therapeutic systems (Jiang et al., 2022). The protein corona exerts a substantial influence on the physicochemical attributes of nanomaterials, including their size, zeta potential, pharmacokinetic profile, and biodistribution (Kopac, 2021). Consequently, the adsorption of biomolecules onto the surface of nanomaterials is widely regarded as a detrimental phenomenon, primarily because of the propensity of adsorbed biomolecules to engage with the mononuclear phagocytic system (MPS), thereby diminishing the circulation duration of nanomaterials in the bloodstream (Jiang et al., 2022). Furthermore, the formation of a protein corona can have additional adverse consequences such as nanomaterial aggregation and impaired active targeting. Notably, a study by Maryam Maghsudi et al. explored the interaction between silver nanoclusters (Ag NCs) and human blood plasma proteins and revealed alterations in the physical and chemical properties of both proteins and Ag NCs (Maghsudi et al., 2018). Similarly, nanomaterials characterized by exceptionally large surface areas, such as graphene and its derivatives, were also susceptible to protein adsorption, resulting in compromised functionalization (Palmieri et al., 2019).
Photodynamic processes rely on the generation of ROS from oxygen molecules to achieve bactericidal effects. However, excessive ROS levels can induce oxidative stress, prompting bacteria to enter a persistent state. During this process, bacteria modulate their oxidative stress response by activating regulators, such as SoxRS, OxyR, and RpoS (Sen and Imlay, 2021). Moreover, bacteria commonly adhere to both biological and nonbiological surfaces, forming biofilms by maturing and producing extracellular polymeric substances (EPS). Biofilms, characterized by their adhesive properties and robust extracellular polysaccharide matrices, protect bacteria against host immune defenses and environmental stress, thereby impeding the penetration of antimicrobial agents (Roy et al., 2018). Bacteria residing deep within biofilms often exhibit distinct phenotypes compared to their planktonic counterparts, and display heightened resistance. Consequently, the persistence of biofilms or the continuous growth of bacteria within them frequently leads to prolonged infections, treatment failure, and potentially fatal outcomes (Del Pozo, 2018). Therefore, the development of novel antibacterial strategies that can effectively inhibit biofilm formation and impede persistent bacterial growth is of the utmost importance (Dong et al., 2022).
5 The approaches for solving the challenges of nanomaterial-based PDT
Over the past few decades, significant progress has been achieved in leveraging nanotechnology to enhance the efficacy of PDT in antimicrobial therapy. However, certain nanomaterials employed in PDT have inherent limitations because of their specific properties. To address these challenges, researchers have developed strategies that present promising avenues for the design and selection of nanomaterials as PSs.
To overcome the inherent limitations associated with nanomaterial properties, targeted surface modifications have been employed to increase ROS production and improve the antimicrobial activity of PDT. The utilization of appropriate nanomaterials with suitable bandgap values for constructing heterojunction structures and synthesizing composite nanomaterials has emerged as an effective approach for enhancing the photodynamic activity of antimicrobial materials. A prominent method involves the decoration of nanomaterials with UCNPs, enabling the regulation of their absorption from the visible/UV to NIR regions, thereby facilitating deeper tissue penetration with reduced biological tissue attenuation (Chen et al., 2018). However, the tunable absorption method based on UCNPs requires further optimization owing to limitations such as limited wavelength selections, low extinction coefficient, and high dependence on laser intensity. Elemental surface doping can also be employed to adjust the inherent crystal and electronic structures of nanomaterials, thereby optimizing their redox abilities. For instance, in the case of metal oxide nanomaterials, the creation of heterojunction structures and the incorporation of noble metals are advantageous strategies for enhancing their activity. Liang et al. successfully prepared the TiO2-ZnO/Au composite nanomaterial with excellent photodynamic hydrogen production performance and achieved an antimicrobial rate of up to 98.2%, in which the p-n heterojunction played a synergistic role in this process (Zhang et al., 2012). Another approach involves the use of near-field enhancement effect to construct composite nanomaterials Researchers employed plasma Au NPs to modify carbon-dot-based nanomaterials, leading to a high production rate of 1O2 under NIR irradiation. Exploring the use of nanomaterials to activate attached PSs may inspire the development of multifunctional PSs. However, this method should be extended to other nanomaterials with careful consideration of the spectral overlap between them (Zhao et al., 2021).
To address the issue of protein corona formation, albumin has been considered as a potential solution. Albumin is an endogenous plasma protein abundantly present in the body that serves essential physiological functions, such as colloid osmotic pressure regulation and transportation of biological compounds (Sleep, 2014). Owing to its numerous binding sites for exogenous ligands, albumin can readily associate with a wide range of biologically active compounds in a relatively non-specific manner (Tiwari et al., 2021). Albumin offers several advantages as a carrier, including biocompatibility, biodegradability, non-toxicity, and non-immunogenicity. It also provides protection to encapsulated drugs or materials against interference in vivo (Rabbani and Ahn, 2019). Human serum albumin (HSA) is a fundamental building block of biomaterials that exhibits minimal cytotoxicity and excellent biocompatibility. HSA has extensive applications as a carrier for nanomaterials both in vitro and in vivo (Figure 9). Peng et al. (2013) demonstrated the successful application of a preformed albumin corona to inhibit plasma protein adsorption and reduce complement activation, leading to prolonged blood circulation time and reduced toxicity of PHBHHx nanoparticles. Moreover, albumin is preferentially taken up by the inflamed tissues, making it a promising candidate for passive targeting via the EPR effect. In cancer research, albumin-based drug delivery systems, such as those combining albumin with paclitaxel, lapatinib, and auristatin, have shown efficacy in tumor suppression. However, only a few studies have investigated albumin as a carrier for photodynamic antibacterial treatments. In 2021, a highly efficient antibacterial agent was synthesized by combining rGO with HSA/polypropylene. This nanomaterial exhibited dual photodynamic effects, generating ROS upon light irradiation and achieving a remarkable antibacterial rate of 96% against antibiotic-resistant bacteria (Amina et al., 2021). In conclusion, the exploitation of albumin as a carrier for photodynamic antibacterial therapy represents a promising avenue for further research.
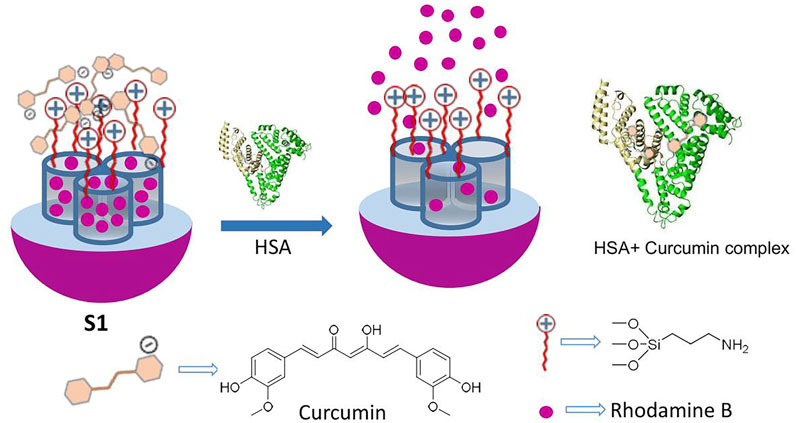
FIGURE 9. The binding sites of HSA have multiple applications (Otri et al., 2022).
To address the challenges posed by antibiotic-resistant bacteria, the exploration of novel treatment modalities involving synergistic light-responsive mechanisms has emerged as a promising approach. Relying solely on PDT is constrained by the limited response of oxygen molecules, resulting in relatively weak therapeutic efficacy. However, combining PDT with other modalities can yield unforeseen advantages (Khan et al., 2022). One such modality is PTT, a non-invasive treatment method that utilizes specific light wavelengths to excite materials and converts them into thermal energy capable of inducing bacterial cell death through thermal ablation (Chen et al., 2020). PTT offers characteristics such as high selectivity, non-toxicity, and ease of operation (Nie et al., 2022). Nonetheless, the use of PTT alone can pose a risk of overheating damage to normal tissue, necessitating the exploration of combined PDT-PTT approaches to mitigate such effects. Moreover, mild heat therapy employed in conjunction with PDT can augment the permeability of bacterial membranes, facilitating the cellular uptake of ROS and consequently enhancing the overall treatment efficacy (Maleki et al., 2021). For instance, Ye et al. developed a synergistic antibacterial strategy by co-modifying chitosan (CS) and bovine serum albumin (BSA) on copper sulfide nanoparticles (CuS NPs), which were further combined with rGO and immobilized on a polydopamine-modified substrate to create the CuS@BSA/rGO-PDA nanocoating. This nanocoating exhibited outstanding antibacterial properties under NIR irradiation, which were achieved through a combination of thermal energy and 1O2 production. Notably, this system demonstrated the integration of antibacterial and bone regeneration capabilities for the treatment of infected implants (Zhang et al., 2021).
Another synergistic treatment utilizes nitric oxide (NO). To overcome the limitations of ROS in PDT as a stand-alone antibacterial treatment, synergistic approaches involving NO have attracted considerable interest. NO has been extensively investigated for its antibacterial properties owing to its extended half-life and large diffusion radius, which provides a broader bactericidal range than ROS (Massoumi et al., 2022). In this context, researchers have developed multifunctional nanoplatforms that combined PDT with NO (Yuan et al., 2020). The platform was based on UCNPs with dual-emission characteristics and coated with mesoporous silica to encapsulate PSs with aggregation-induced emission (AIE) properties. Under 808 nm light irradiation, the UCNPs generated both UV and visible light emissions, triggering the release of NO and ROS. The O2−• generated by PDT reacted with NO to form a potent oxidizing and nitrifying agent, peroxynitrite (ONOO-). ONOO- was the key to the specific synergistic treatment, which led to bacteria killing and biofilm elimination with high efficiency. Experimental data demonstrated that the synergistic interplay between O2−•, NO, and ONOO- significantly enhanced the antibacterial efficacy (Zhang et al., 2022).
6 Conclusion
In recent years, significant advancements have been made in the field of antibacterial PDT through the development of nanomaterials (Sun et al., 2021; Jin et al., 2022; Wu et al., 2022). Nanomaterial-based PDT offer several advantages over traditional organic materials, including higher ROS quantum yields and broader application prospects (Jia et al., 2019). These novel PSs have tremendous potential for achieving remarkable photodynamic antibacterial efficacy. To enhance selectivity, mitigate cellular toxicity, and improve the antibacterial targeting capabilities, it is crucial to develop nanomaterial-based PSs or carriers with multifunctional characteristics. Furthermore, comprehensive nanotoxicological studies of these nanomaterials are of paramount importance. Such studies should consider various parameters, including surface chemical properties, size, zeta potential, biological distribution, and biodegradability, to assess long-term safety implications. With rapid advancements in nanotechnology, it is anticipated that new generations of nanomaterials with multifunctional properties will be developed in the near future, leading to breakthroughs in combating multidrug-resistant bacteria.
Author contributions
YG: Writing–original draft. HL: Writing–original draft. YL: Writing–review and editing. JL: Writing–review and editing. CG: Writing–review and editing. HC: Writing–review and editing. RG: Writing–review and editing.
Funding
The author(s) declare financial support was received for the research, authorship, and/or publication of this article. This research was funded by the Sichuan Medical Association (Q21037), Chengdu Health Commission (2022095), Chengdu Medical College (21Z079), The First Affiliated Hospital of Chengdu Medical College (CYFY-GQ14), Sichuan Education Department (16ZB0290 and 18ZA0156), Sichuan Social Science Base (CSXL-203011), Sichuan Science and Technology Department (2022NSFSC1576 and 2022NSFSC1333).
Conflict of interest
The authors declare that the research was conducted in the absence of any commercial or financial relationships that could be construed as a potential conflict of interest.
Publisher’s note
All claims expressed in this article are solely those of the authors and do not necessarily represent those of their affiliated organizations, or those of the publisher, the editors and the reviewers. Any product that may be evaluated in this article, or claim that may be made by its manufacturer, is not guaranteed or endorsed by the publisher.
References
Ahmad-Mansour, N., Loubet, P., Pouget, C., Dunyach-Remy, C., Sotto, A., Lavigne, J. P., et al. (2021). Staphylococcus aureus toxins: an update on their pathogenic properties and potential treatments. Toxins (Basel) 13 (10), 677. doi:10.3390/toxins13100677
Alavi, M., Kamarasu, P., McClements, D. J., and Moore, M. D. (2022). Metal and metal oxide-based antiviral nanoparticles: properties, mechanisms of action, and applications. Adv. Colloid Interface Sci. 306, 102726. doi:10.1016/j.cis.2022.102726
Amina, M., Al Musayeib, N. M., Alarfaj, N. A., El-Tohamy, M. F., and Al-Hamoud, G. A. (2021). Facile multifunctional-mode of fabricated biocompatible human serum albumin/reduced graphene oxide/Cladophora glomerata nanoparticles for bacteriostatic phototherapy, bacterial tracking and antioxidant potential. Nanotechnology 32 (31), 315301. doi:10.1088/1361-6528/abf457
Anju, V. T., Paramanantham, P., Siddhardha, B., Sruthil Lal, S. B., Sharan, A., Alyousef, A. A., et al. (2019). Malachite green-conjugated multi-walled carbon nanotubes potentiate antimicrobial photodynamic inactivation of planktonic cells and biofilms of Pseudomonas aeruginosa and Staphylococcus aureus. Int. J. Nanomed. 14, 3861–3874. doi:10.2147/IJN.S202734
Balhaddad, A. A., Garcia, I. M., Mokeem, L., Alsahafi, R., Collares, F. M., and Sampaio de Melo, M. A. (2021). Metal oxide nanoparticles and nanotubes: ultrasmall nanostructures to engineer antibacterial and improved dental adhesives and composites. Bioeng. (Basel) 8 (10), 146. doi:10.3390/bioengineering8100146
Ballatore, M. B., Durantini, J., Gsponer, N. S., Suarez, M. B., Gervaldo, M., Otero, L., et al. (2015). Photodynamic inactivation of bacteria using novel electrogenerated porphyrin-fullerene C60 polymeric films. Environ. Sci. Technol. 49 (12), 7456–7463. doi:10.1021/acs.est.5b01407
Borisov, V. B., Siletsky, S. A., Nastasi, M. R., and Forte, E. (2021). ROS defense systems and terminal oxidases in bacteria. Antioxidants (Basel) 10 (6), 839. doi:10.3390/antiox10060839
Caballero-Mancebo, E., Cohen, B., Smolders, S., De Vos, D. E., and Douhal, A. (2019). Unravelling why and to what extent the topology of similar ce-based MOFs conditions their photodynamic: relevance to photocatalysis and photonics. Adv. Sci. (Weinh) 6 (19), 1901020. doi:10.1002/advs.201901020
Cai, P. F., Li, J., Wu, X. B., Li, Z. Y., Shen, J., Nie, J. J., et al. (2022). ALD-induced TiO2/Ag nanofilm for rapid surface photodynamic ion sterilization. Rare Met. 41 (12), 4138–4148. doi:10.1007/s12598-022-02096-w
Chadwick, S. J., Salah, D., Livesey, P. M., Brust, M., and Volk, M. (2016). Singlet oxygen generation by laser irradiation of gold nanoparticles. J. Phys. Chem. C 120 (19), 10647–10657. doi:10.1021/acs.jpcc.6b02005
Chang, R. Y. K., Nang, S. C., Chan, H. K., and Li, J. (2022). Novel antimicrobial agents for combating antibiotic-resistant bacteria. Adv. Drug Deliv. Rev. 187, 114378. doi:10.1016/j.addr.2022.114378
Chatgilialoglu, C., Ferreri, C., Krokidis, M. G., Masi, A., and Terzidis, M. A. (2021). On the relevance of hydroxyl radical to purine DNA damage. Free Radic. Res. 55 (4), 384–404. doi:10.1080/10715762.2021.1876855
Chen, D., Xu, Q., Wang, W., Shao, J., Huang, W., and Dong, X. (2021). Type I photosensitizers revitalizing photodynamic oncotherapy. Small 17 (31), e2006742. doi:10.1002/smll.202006742
Chen, S., Weitemier, A. Z., Zeng, X., He, L., Wang, X., Tao, Y., et al. (2018). Near-infrared deep brain stimulation via upconversion nanoparticle–mediated optogenetics. Science 359, 679–684. doi:10.1126/science.aaq1144
Chen, Y., Gao, Y., Chen, Y., Liu, L., Mo, A., and Peng, Q. (2020). Nanomaterials-based photothermal therapy and its potentials in antibacterial treatment. J. Control. Release 328, 251–262. doi:10.1016/j.jconrel.2020.08.055
Chen, Y., Huang, W., Dong, Y., Yu, X., Mo, A., and Peng, Q. (2022). Enhanced antibacterial activity of indocyanine green-loaded graphene oxide via synergistic contact killing, photothermal and photodynamic therapy. J. Biomed. Nanotechnol. 18 (1), 185–192. doi:10.1166/jbn.2022.3236
Cheng, S., Qi, M., Li, W., Sun, W., Li, M., Lin, J., et al. (2023). Dual-responsive nanocomposites for synergistic antibacterial therapies facilitating bacteria-infected wound healing. Adv. Healthc. Mat. 12 (6), e2202652. doi:10.1002/adhm.202202652
Chisté, R. C., Freitas, M., MercadanteA, Z., and Fernandes, E. (2015). Superoxide anion radical: generation and detection in cellular and non-cellular systems. Curr. Med. Chem. 22, 4234–4256. doi:10.2174/0929867322666151029104311
Cho, M., Chung, H., Choi, W., and Yoon, J. (2004). Linear correlation between inactivation of E. coli and OH radical concentration in TiO2 photocatalytic disinfection. Water Res. 38 (4), 1069–1077. doi:10.1016/j.watres.2003.10.029
Chung, S., Revia, R. A., and Zhang, M. (2021). Graphene quantum dots and their applications in bioimaging, biosensing, and therapy. Adv. Mat. 33 (22), e1904362. doi:10.1002/adma.201904362
Comeau, P., Burgess, J., Malekafzali, N., Leite, M. L., Lee, A., and Manso, A. (2022). Exploring the physicochemical, mechanical, and photocatalytic antibacterial properties of a methacrylate-based dental material loaded with ZnO nanoparticles. Mater. (Basel) 15 (14), 5075. doi:10.3390/ma15145075
Correia-Barros, G., Serambeque, B., Carvalho, M. J., Marto, C. M., Pineiro, M., Pinho, E. M. T., et al. (2022). Applications of photodynamic therapy in endometrial diseases. Bioeng. (Basel) 9 (5), 226. doi:10.3390/bioengineering9050226
Del Pozo, J. L. (2018). Biofilm-related disease. Expert Rev. anti-infect. 16 (1), 51–65. doi:10.1080/14787210.2018.1417036
Di Mascio, P., Martinez, G. R., Miyamoto, S., Ronsein, G. E., Medeiros, M. H. G., and Cadet, J. (2019). Singlet molecular oxygen reactions with nucleic acids, lipids, and proteins. Chem. Rev. 119 (3), 2043–2086. doi:10.1021/acs.chemrev.8b00554
Ding, Y., Gonick, H. C., and Vaziri, N. D. (2000). Lead promotes hydroxyl radical generation and lipid peroxidation in cultured aortic endothelial cells. Am. J. Hypertens. 13, 552–555. doi:10.1016/s0895-7061(99)00226-5
Dong, H., Yang, K., Zhang, Y., Li, Q., Xiu, W., Ding, M., et al. (2022). Photocatalytic Cu2WS4 nanocrystals for efficient bacterial killing and biofilm disruption. Int. J. Nanomedicine 17, 2735–2750. doi:10.2147/IJN.S360246
Duan, J., Li, Y., Gao, J., Cao, R., Shang, E., and Zhang, W. (2022). ROS-mediated photoaging pathways of nano- and micro-plastic particles under UV irradiation. Water Res. 216, 118320. doi:10.1016/j.watres.2022.118320
Eskikaya, O., Ozdemir, S., Tollu, G., Dizge, N., Ramaraj, R., Manivannan, A., et al. (2022). Synthesis of two different zinc oxide nanoflowers and comparison of antioxidant and photocatalytic activity. Chemosphere 306, 135389. doi:10.1016/j.chemosphere.2022.135389
Ezraty, B., Gennaris, A., Barras, F., and Collet, J. F. (2017). Oxidative stress, protein damage and repair in bacteria. Nat. Rev. Microbiol. 15 (7), 385–396. doi:10.1038/nrmicro.2017.26
Feng, W., Han, C., and Li, F. (2013). Upconversion-nanophosphor-based functional nanocomposites. Adv. Mat. 25 (37), 5287–5303. doi:10.1002/adma.201301946
Fleming, A. M., and Burrows, C. J. (2020). On the irrelevancy of hydroxyl radical to DNA damage from oxidative stress and implications for epigenetics. Chem. Soc. Rev. 49 (18), 6524–6528. doi:10.1039/d0cs00579g
Hakimov, S., Kylychbekov, S., Harness, B., Neupane, S., Hurley, J., Brooks, A., et al. (2022). Evaluation of silver nanoparticles attached to methylene blue as an antimicrobial agent and its cytotoxicity. Photodiagnosis Photodyn. Ther. 39, 102904. doi:10.1016/j.pdpdt.2022.102904
Han, H., Xu, X., Kan, H., Tang, Y., Liu, C., Wen, H., et al. (2022). Synergistic photodynamic/photothermal bacterial inactivation over heterogeneous quaternized chitosan/silver/cobalt phosphide nanocomposites. J. Colloid Interface Sci. 616, 304–315. doi:10.1016/j.jcis.2022.02.068
Hashikawa, Y., Fujikawa, N., and Murata, Y. (2022). π-extended fullerenes with a reactant inside. J. Am. Chem. Soc. 144 (51), 23292–23296. doi:10.1021/jacs.2c12259
Henna, T. K., and Pramod, K. (2020). Graphene quantum dots redefine nanobiomedicine. Mat. Sci. Eng. C 110, 110651. doi:10.1016/j.msec.2020.110651
Hou, W., Shi, G., Wu, S., Mo, J., Shen, L., Zhang, X., et al. (2022). Application of fullerenes as photosensitizers for antimicrobial photodynamic inactivation: A review. Front. Microbiol. 13, 957698. doi:10.3389/fmicb.2022.957698
Hou, Y. J., Yang, X. X., Liu, R. Q., Zhao, D., Guo, C. X., Zhu, A. C., et al. (2020). <p>Pathological mechanism of photodynamic therapy and photothermal therapy based on nanoparticles</p>. Int. J. Nanomedicine 15, 6827–6838. doi:10.2147/IJN.S269321
Imlay, J. A. (2019). Where in the world do bacteria experience oxidative stress? Environ. Microbiol. 21 (2), 521–530. doi:10.1111/1462-2920.14445
Iqbal, G., Faisal, S., Khan, S., Shams, D. F., and Nadhman, A. (2019). Photo-inactivation and efflux pump inhibition of methicillin-resistant Staphylococcus aureus using thiolated cobalt doped ZnO nanoparticles. J. Photochem. Photobiol. B 192, 141–146. doi:10.1016/j.jphotobiol.2019.01.021
Iwatsu, M., Kanetaka, H., Mokudai, T., Ogawa, T., Kawashita, M., and Sasaki, K. (2020). Visible light-induced photocatalytic and antibacterial activity of N-doped TiO2. J. Biomed. Mat. Res. 108 (2), 451–459. doi:10.1002/jbm.b.34401
Jia, Q., Song, Q., Li, P., and Huang, W. (2019). Rejuvenated photodynamic therapy for bacterial infections. Adv. Healthc. Mat. 8 (14), e1900608. doi:10.1002/adhm.201900608
Jiang, Z., Chu, Y., and Zhan, C. (2022). Protein corona: challenges and opportunities for targeted delivery of nanomedicines. Expert Opin. Drug Deliv. 19 (7), 833–846. doi:10.1080/17425247.2022.2093854
Jie, Z., Liu, J., Shu, M., Ying, Y., and Yang, H. (2022). Detection strategies for superoxide anion: A review. Talanta 236, 122892. doi:10.1016/j.talanta.2021.122892
Jin, Y., Zhao, B., Guo, W., Li, Y., Min, J., and Miao, W. (2022). Penetration and photodynamic ablation of drug-resistant biofilm by cationic iron oxide nanoparticles. J. Control. Release 348, 911–923. doi:10.1016/j.jconrel.2022.06.038
Karami, A., Farivar, F., de Prinse, T. J., Rabiee, H., Kidd, S., Sumby, C. J., et al. (2021). Facile multistep synthesis of ZnO-coated beta-NaYF4:Yb/Tm upconversion nanoparticles as an antimicrobial photodynamic therapy for persistent Staphylococcus aureus small colony variants. ACS Appl. Bio. Mat. 4 (8), 6125–6136. doi:10.1021/acsabm.1c00473
Khan, S. B., Khan, M. I., and Nisar, J. (2022). Microwave-assisted green synthesis of pure and mn-doped ZnO nanocomposites: in vitro antibacterial assay and photodegradation of methylene blue. Front. Mat. 8. doi:10.3389/fmats.2021.710155
Kopac, T. (2021). Protein corona, understanding the nanoparticle-protein interactions and future perspectives: A critical review. Int. J. Biol. Macromol. 169, 290–301. doi:10.1016/j.ijbiomac.2020.12.108
Kuo, W. S., Chang, C. Y., Liu, J. C., Chen, J. H., So, E. C., and Wu, P. C. (2020). <p>Two-Photon photoexcited photodynamic therapy with water-soluble fullerenol serving as the highly effective two-photon photosensitizer against multidrug-resistant bacteria</p>. Int. J. Nanomedicine 15, 6813–6825. doi:10.2147/IJN.S236897
Kuo, W. S., Yeh, T. S., Chang, C. Y., Liu, J. C., Chen, C. H., So, E. C., et al. (2020). <p>Amino-Functionalized nitrogen-doped graphene quantum dots for efficient enhancement of two-photon-excitation photodynamic therapy: functionalized nitrogen as a bactericidal and contrast agent</p>. Int. J. Nanomedicine 15, 6961–6973. doi:10.2147/IJN.S242892
Kwiatkowski, S., Knap, B., Przystupski, D., Saczko, J., Kedzierska, E., Knap-Czop, K., et al. (2018). Photodynamic therapy - mechanisms, photosensitizers and combinations. Biomed. Pharmacother. 106, 1098–1107. doi:10.1016/j.biopha.2018.07.049
Lamy, B., Sundqvist, M., and Idelevich, E. A. (2020). Bloodstream infections - standard and progress in pathogen diagnostics. Clin. Microbiol. Infect. 26 (2), 142–150. doi:10.1016/j.cmi.2019.11.017
Lan, M., Zhao, S., Liu, W., Lee, C. S., Zhang, W., and Wang, P. (2019). Photosensitizers for photodynamic therapy. Adv. Healthc. Mat. 8 (13), e1900132. doi:10.1002/adhm.201900132
Lennicke, C., and Cocheme, H. M. (2021). Redox metabolism: ROS as specific molecular regulators of cell signaling and function. Mol. Cell 81 (18), 3691–3707. doi:10.1016/j.molcel.2021.08.018
Li, B., Kumar, M., Zhou, C., Li, L., and Francisco, J. S. (2022). Mechanistic insights into criegee intermediate-hydroperoxyl radical chemistry. J. Am. Chem. Soc. 144 (32), 14740–14747. doi:10.1021/jacs.2c05346
Li, J., Song, S., Meng, J., Tan, L., Liu, X., Zheng, Y., et al. (2021). 2D MOF periodontitis photodynamic ion therapy. J. Am. Chem. Soc. 143 (37), 15427–15439. doi:10.1021/jacs.1c07875
Li, M., Xia, J., Tian, R., Wang, J., Fan, J., Du, J., et al. (2018). Near-infrared light-initiated molecular superoxide radical generator: rejuvenating photodynamic therapy against hypoxic tumors. J. Am. Chem. Soc. 140 (44), 14851–14859. doi:10.1021/jacs.8b08658
Li, Y., Du, J., Huang, S., Wang, S., Wang, Y., Cai, Z., et al. (2022). Hydrogen peroxide potentiates antimicrobial photodynamic therapy in eliminating Candida albicans and Streptococcus mutans dual-species biofilm from denture base. Photodiagnosis Photodyn. Ther. 37, 102691. doi:10.1016/j.pdpdt.2021.102691
Li, Z., Lu, S., Liu, W., Dai, T., Ke, J., Li, X., et al. (2021). Synergistic lysozyme-photodynamic therapy against resistant bacteria based on an intelligent upconversion nanoplatform. Angew. Chem. Int. Ed. 60 (35), 19201–19206. doi:10.1002/anie.202103943
Liu, E., Chen, Y., Xu, J., Gu, S., An, N., Xin, J., et al. (2022). Platelets inhibit methicillin-resistant Staphylococcus aureus by inducing hydroxyl radical-mediated apoptosis-like cell death. Microbiol. Spectr. 10 (4), e0244121. doi:10.1128/spectrum.02441-21
Liu, G. (2015). Advances in the theoretical understanding of photon upconversion in rare-earth activated nanophosphors. Chem. Soc. Rev. 44 (6), 1635–1652. doi:10.1039/c4cs00187g
Liu, J., Cheng, W., Zhang, K., Liu, H., Li, J., Tressel, J., et al. (2022). High-efficiency photodynamic antibacterial activity of NH2-MIL-101(Fe)@MoS2/ZnO Ternary Composites. ACS Appl. Bio. Mat. 5 (8), 3912–3922. doi:10.1021/acsabm.2c00439
Liu, M., Chen, Y., Guo, Y., Yuan, H., Cui, T., Yao, S., et al. (2022). Golgi apparatus-targeted aggregation-induced emission luminogens for effective cancer photodynamic therapy. Nat. Commun. 13 (1), 2179. doi:10.1038/s41467-022-29872-7
Liu, X., Liu, H., Zhang, J., Hao, Y., Yang, H., Zhao, W., et al. (2022). Construction of a matchstick-shaped Au@ZnO@SiO2-ICG Janus nanomotor for light-triggered synergistic antibacterial therapy. Biomater. Sci. 10 (19), 5608–5619. doi:10.1039/d2bm00845a
Luan, L., Du, L., Shi, W., Li, Y., and Zhang, Q. (2022). Photodynamic inactivation of bacteria and biofilms with benzoselenadiazole-doped metal-organic frameworks. Molecules 27 (24), 8908. doi:10.3390/molecules27248908
Ma, R., Xue, Y., Ma, Q., Chen, Y., Yuan, S., and Fan, J. (2022). Recent advances in carbon-based materials for adsorptive and photocatalytic antibiotic removal. Nanomaterials 12 (22), 4045. doi:10.3390/nano12224045
Maghsudi, M., Shahabadi, N., Kooshk, M. R. A., Ghaemi, N., Nemati, L., Parvaneh, S., et al. (2018). Nontoxic silver nanocluster-induced folding, fibrillation, and aggregation of blood plasma proteins. Int. J. Biol. Macromol. 119, 838–848. doi:10.1016/j.ijbiomac.2018.07.177
Maleki, A., He, J., Bochani, S., Nosrati, V., Shahbazi, M. A., and Guo, B. (2021). Multifunctional photoactive hydrogels for wound healing acceleration. ACS Nano 15 (12), 18895–18930. doi:10.1021/acsnano.1c08334
Mao, C., Xiang, Y., Liu, X., Cui, Z., Yang, X., Yeung, K. W. K., et al. (2017). Photo-inspired antibacterial activity and wound healing acceleration by hydrogel embedded with Ag/Ag@AgCl/ZnO nanostructures. ACS Nano 11 (9), 9010–9021. doi:10.1021/acsnano.7b03513
Massoumi, H., Kumar, R., Chug, M. K., Qian, Y., and Brisbois, E. J. (2022). Nitric oxide release and antibacterial efficacy analyses of S-nitroso-N-acetyl-penicillamine conjugated to titanium dioxide nanoparticles. ACS Appl. Bio Mat. 5 (5), 2285–2295. doi:10.1021/acsabm.2c00131
Murotomi, K., Umeno, A., Shichiri, M., Tanito, M., and Yoshida, Y. (2023). Significance of singlet oxygen molecule in pathologies. Int. J. Mol. Sci. 24 (3), 2739. doi:10.3390/ijms24032739
Mushtaq, S., Yasin, T., Saleem, M., Dai, T., and Yameen, M. A. (2022). Potentiation of antimicrobial photodynamic therapy by curcumin-loaded graphene quantum dots. Photochem. Photobiol. 98 (1), 202–210. doi:10.1111/php.13503
Negri, V., Pacheco-Torres, J., Calle, D., and Lopez-Larrubia, P. (2020). Carbon nanotubes in biomedicine. Top. Curr. Chem. 378 (1), 15. doi:10.1007/s41061-019-0278-8
Nie, R., Sun, Y., Lv, H., Lu, M., Huangfu, H., Li, Y., et al. (2022). 3D printing of MXene composite hydrogel scaffolds for photothermal antibacterial activity and bone regeneration in infected bone defect models. Nanoscale 14 (22), 8112–8129. doi:10.1039/d2nr02176e
Nosaka, Y., and Nosaka, A. Y. (2017). Generation and detection of reactive oxygen species in photocatalysis. Chem. Rev. 117 (17), 11302–11336. doi:10.1021/acs.chemrev.7b00161
Okamoto, I., Miyaji, H., Miyata, S., Shitomi, K., Sugaya, T., Ushijima, N., et al. (2021). Antibacterial and antibiofilm photodynamic activities of lysozyme-Au nanoclusters/rose bengal conjugates. ACS Omega 6 (13), 9279–9290. doi:10.1021/acsomega.1c00838
Otri, I., Medaglia, S., Aznar, E., Sancenon, F., and Martinez-Manez, R. (2022). Fluorogenic detection of human serum albumin using curcumin-capped mesoporous silica nanoparticles. Molecules 27 (3), 1133. doi:10.3390/molecules27031133
Palmieri, V., Perini, G., De Spirito, M., and Papi, M. (2019). Graphene oxide touches blood: in vivo interactions of bio-coronated 2D materials. Nanoscale Horiz. 4 (2), 273–290. doi:10.1039/c8nh00318a
Pang, Z., Raudonis, R., Glick, B. R., Lin, T. J., and Cheng, Z. (2019). Antibiotic resistance in Pseudomonas aeruginosa: mechanisms and alternative therapeutic strategies. Biotechnol. Adv. 37 (1), 177–192. doi:10.1016/j.biotechadv.2018.11.013
Parasuraman, P., Anju, V. T., Lal, S. B. S., Sharan, A., Busi, S., Kaviyarasu, K., et al. (2019). Synthesis and antimicrobial photodynamic effect of methylene blue conjugated carbon nanotubes on E. coli and S. aureus. Photochem. Photobiol. Sci. 18 (2), 563–576. doi:10.1039/c8pp00369f
Peng, Q., Zhang, S., Yang, Q., Zhang, T., Wei, X. Q., Jiang, L., et al. (2013). Preformed albumin corona, a protective coating for nanoparticles based drug delivery system. Biomaterials 34 (33), 8521–8530. doi:10.1016/j.biomaterials.2013.07.102
Plotino, G., Grande, N. M., and Mercade, M. (2019). Photodynamic therapy in endodontics. Int. Endod. J. 52 (6), 760–774. doi:10.1111/iej.13057
Qi, M., Chi, M., Sun, X., Xie, X., Weir, M. D., Oates, T. W., et al. (2019). <p>Novel nanomaterial-based antibacterial photodynamic therapies to combat oral bacterial biofilms and infectious diseases</p>. Int. J. Nanomedicine 14, 6937–6956. doi:10.2147/IJN.S212807
Qi, M., Li, X., Sun, X., Li, C., Tay, F. R., Weir, M. D., et al. (2019). Novel nanotechnology and near-infrared photodynamic therapy to kill periodontitis-related biofilm pathogens and protect the periodontium. Dent. Mat. 35 (11), 1665–1681. doi:10.1016/j.dental.2019.08.115
Rabbani, G., and Ahn, S. N. (2019). Structure, enzymatic activities, glycation and therapeutic potential of human serum albumin: A natural cargo. Int. J. Biol. Macromol. 123, 979–990. doi:10.1016/j.ijbiomac.2018.11.053
Rajendran, M. (2016). Quinones as photosensitizer for photodynamic therapy: ROS generation, mechanism and detection methods. Photodiagnosis Photodyn. Ther. 13, 175–187. doi:10.1016/j.pdpdt.2015.07.177
Rehman, F. U., Zhao, C., Jiang, H., and Wang, X. (2016). Biomedical applications of nano-titania in theranostics and photodynamic therapy. Biomater. Sci. 4 (1), 40–54. doi:10.1039/c5bm00332f
Ristic, B. Z., Milenkovic, M. M., Dakic, I. R., Todorovic-Markovic, B. M., Milosavljevic, M. S., Budimir, M. D., et al. (2014). Photodynamic antibacterial effect of graphene quantum dots. Biomaterials 35 (15), 4428–4435. doi:10.1016/j.biomaterials.2014.02.014
Roy, R., Tiwari, M., Donelli, G., and Tiwari, V. (2018). Strategies for combating bacterial biofilms: A focus on anti-biofilm agents and their mechanisms of action. Virulence 9 (1), 522–554. doi:10.1080/21505594.2017.1313372
Sah, U., Sharma, K., Chaudhri, N., Sankar, M., and Gopinath, P. (2018). Antimicrobial photodynamic therapy: single-walled carbon nanotube (SWCNT)-porphyrin conjugate for visible light mediated inactivation of Staphylococcus aureus. Colloids Surf. B 162, 108–117. doi:10.1016/j.colsurfb.2017.11.046
Saied, E., Salem, S. S., Al-Askar, A. A., Elkady, F. M., Arishi, A. A., and Hashem, A. H. (2022). Mycosynthesis of hematite (alpha-Fe2O3) nanoparticles using aspergillus Niger and their antimicrobial and photocatalytic activities. Bioeng. (Basel) 9 (8), 397. doi:10.3390/bioengineering9080397
Sangam, S., Jindal, S., Agarwal, A., Banerjee, B. D., Prasad, P., and Mukherjee, M. (2022). Graphene quantum dot-porphyrin/phthalocyanine multifunctional hybrid systems: from interfacial dialogue to application. Biomater. Sci. 10 (7), 1647–1679. doi:10.1039/d2bm00016d
Sen, A., and Imlay, J. A. (2021). How microbes defend themselves from incoming hydrogen peroxide. Front. Immunol. 12, 667343. doi:10.3389/fimmu.2021.667343
Shekhar, S., Chauhan, M., Hu, L., SonaliYadav, B., Dutt, R., et al. (2022). Enhanced permeability and retention effect-focused tumor-targeted nanomedicines: latest trends, obstacles and future perspective. Nanomedicine 17 (18), 1213–1216. doi:10.2217/nnm-2022-0065
Shiby, E., Reddy, K. L., and Kumar, J. (2022). A facile approach for the ligand free synthesis of biocompatible upconversion nanophosphors. Front. Chem. 10, 904676. doi:10.3389/fchem.2022.904676
Sleep, D. (2014). Albumin and its application in drug delivery. Expert Opin. Drug Deliv. 12 (5), 793–812. doi:10.1517/17425247.2015.993313
Song, H., Zhang, M., and Tong, W. (2022). Single-atom nanozymes: fabrication, characterization, surface modification and applications of ROS scavenging and antibacterial. Molecules 27 (17), 5426. doi:10.3390/molecules27175426
Sun, J., Song, L., Fan, Y., Tian, L., Luan, S., Niu, S., et al. (2019). Synergistic photodynamic and photothermal antibacterial nanocomposite membrane triggered by single NIR light source. ACS Appl. Mat. Inter. 11 (30), 26581–26589. doi:10.1021/acsami.9b07037
Sun, Y., Feng, W., Yang, P., Huang, C., and Li, F. (2015). The biosafety of lanthanide upconversion nanomaterials. Chem. Soc. Rev. 44 (6), 1509–1525. doi:10.1039/c4cs00175c
Sun, Y., Sun, X., Li, X., Li, W., Li, C., Zhou, Y., et al. (2021). A versatile nanocomposite based on nanoceria for antibacterial enhancement and protection from a PDT-aggravated inflammation via modulation of macrophage polarization. Biomaterials 268, 120614. doi:10.1016/j.biomaterials.2020.120614
Sun, Y., Zhao, D., Wang, G., Wang, Y., Cao, L., Sun, J., et al. (2020). Recent progress of hypoxia-modulated multifunctional nanomedicines to enhance photodynamic therapy: opportunities, challenges, and future development. Acta Pharm. Sin. B 10 (8), 1382–1396. doi:10.1016/j.apsb.2020.01.004
Tan, Y., Ma, Y., Fu, S., and Zhang, A. (2022). Facile construction of fluorescent C70-COOH nanoparticles with advanced antibacterial and anti-biofilm photodynamic activity. J. Photoch. Photobio. B 234, 112507. doi:10.1016/j.jphotobiol.2022.112507
Tegos, G. P., Demidova, T. N., Arcila-Lopez, D., Lee, H., Wharton, T., Gali, H., et al. (2005). Cationic fullerenes are effective and selective antimicrobial photosensitizers. Chem. Biol. 12 (10), 1127–1135. doi:10.1016/j.chembiol.2005.08.014
Teng, K. X., Chen, W. K., Niu, L. Y., Fang, W. H., Cui, G., and Yang, Q. Z. (2021). BODIPY-Based photodynamic agents for exclusively generating superoxide radical over singlet oxygen. Angew. Chem. Int. Ed. 60 (36), 19912–19920. doi:10.1002/anie.202106748
Tiwari, R., Sethiya, N. K., Gulbake, A. S., Mehra, N. K., Murty, U. S. N., and Gulbake, A. (2021). A review on albumin as a biomaterial for ocular drug delivery. Int. J. Biol. Macromol. 191, 591–599. doi:10.1016/j.ijbiomac.2021.09.112
Totonchy, M. B., and Chiu, M. W. (2014). UV-based therapy. Dermatol. Clin. 32 (3), 399–413. ix-x. doi:10.1016/j.det.2014.03.003
Tu, C., Lu, H., Zhou, T., Zhang, W., Deng, L., Cao, W., et al. (2022). Promoting the healing of infected diabetic wound by an anti-bacterial and nano-enzyme-containing hydrogel with inflammation-suppressing, ROS-scavenging, oxygen and nitric oxide-generating properties. Biomaterials 286, 121597. doi:10.1016/j.biomaterials.2022.121597
Usman, M., Ishafaq, M. U. U., Muhammad, Z., Ali, W., Dastgeer, G., Zhang, X., et al. (2023). Evaporation-induced self-assembly of gold nanorods on a hydrophobic substrate for surface enhanced Raman spectroscopy applications. Front. Mat. 9. doi:10.3389/fmats.2022.1048011
Vankayala, R., Sagadevan, A., Vijayaraghavan, P., Kuo, C. L., and Hwang, K. C. (2011). Metal nanoparticles sensitize the formation of singlet oxygen. Angew. Chem. Int. Ed. 50 (45), 10640–10644. doi:10.1002/anie.201105236
Wang, C., Zhao, P., Jiang, D., Yang, G., Xue, Y., Tang, Z., et al. (2020). In situ catalytic reaction for solving the aggregation of hydrophobic photosensitizers in tumor. ACS Appl. Mat. Interfaces 12 (5), 5624–5632. doi:10.1021/acsami.9b21589
Wang, Q., Liu, S., Lu, W., and Zhang, P. (2022). Fabrication of curcumin@Ag loaded core/shell nanofiber membrane and its synergistic antibacterial properties. Front. Chem. 10, 870666. doi:10.3389/fchem.2022.870666
Wang, R., Jia, C., Zheng, N., Liu, S., Qi, Z., Wang, R., et al. (2022). Effects of photodynamic therapy on Streptococcus mutans and enamel remineralization of multifunctional TiO2-HAP composite nanomaterials. Photodiagnosis Photodyn. Ther. 42, 103141. doi:10.1016/j.pdpdt.2022.103141
Wang, W., Gao, W., Nie, X., Liu, W., Cheng, X., Shang, N., et al. (2022). Photocatalytic selective amines oxidation coupled with H2O2 production over hyper-cross-linked polymers. J. Colloid Interface Sci. 616, 1–11. doi:10.1016/j.jcis.2022.02.055
Wilczewska, A. Z., Niemirowicz, K., Markiewic, K. H., and Car, H. (2012). Nanoparticles as drug delivery systems. Pharmacol. Rep. 64, 1020–1037. doi:10.1016/s1734-1140(12)70901-5
Winterbourn, C. C. (2016). Revisiting the reactions of superoxide with glutathione and other thiols. Arch. Biochem. Biophys. 595, 68–71. doi:10.1016/j.abb.2015.11.028
Winterbourn, C. C. (2013). The biological chemistry of hydrogen peroxide. Methods Enzymol. 528, 3–25. doi:10.1016/B978-0-12-405881-1.00001-X
Wu, X., Abbas, K., Yang, Y., Li, Z., Tedesco, A. C., and Bi, H. (2022). Photodynamic anti-bacteria by carbon dots and their nano-composites. Pharm. (Basel) 15 (4), 487. doi:10.3390/ph15040487
Xia, M. Y., Xie, Y., Yu, C. H., Chen, G. Y., Li, Y. H., Zhang, T., et al. (2019). Graphene-based nanomaterials: the promising active agents for antibiotics-independent antibacterial applications. J. Control. Release. 307, 16–31. doi:10.1016/j.jconrel.2019.06.011
Xiao, Y. F., Chen, W. C., Chen, J. X., Lu, G., Tian, S., Cui, X., et al. (2022). Amplifying free radical generation of AIE photosensitizer with small singlet-triplet splitting for hypoxia-overcoming photodynamic therapy. ACS Appl. Mat. Interfaces 14 (4), 5112–5121. doi:10.1021/acsami.1c23797
Xie, W., Chen, J., Cheng, X., Feng, H., Zhang, X., Zhu, Z., et al. (2023). Multi-mechanism antibacterial strategies enabled by synergistic activity of metal-organic framework-based nanosystem for infected tissue regeneration. Small 19 (14), e2205941. doi:10.1002/smll.202205941
Xie, X., Mao, C., Liu, X., Zhang, Y., Cui, Z., Yang, X., et al. (2017). Synergistic bacteria killing through photodynamic and physical actions of graphene oxide/Ag/collagen coating. ACS Appl. Mat. Interfaces 9 (31), 26417–26428. doi:10.1021/acsami.7b06702
Xiu, W., Wan, L., Yang, K., Li, X., Yuwen, L., Dong, H., et al. (2022). Potentiating hypoxic microenvironment for antibiotic activation by photodynamic therapy to combat bacterial biofilm infections. Nat. Commun. 13 (1), 3875. doi:10.1038/s41467-022-31479-x
Xu, D., Huang, C. H., Xie, L. N., Shao, B., Mao, L., Shao, J., et al. (2019). Mechanism of unprecedented hydroxyl radical production and site-specific oxidative DNA damage by photoactivation of the classic arylhydroxamic acid carcinogens. Carcinogenesis 2019, bgz021. doi:10.1093/carcin/bgz021
Yang, B., Chen, Y., and Shi, J. (2019). Reactive oxygen species (ROS)-based nanomedicine. Chem. Rev. 119 (8), 4881–4985. doi:10.1021/acs.chemrev.8b00626
Yang, J., and Yang, Y. W. (2020). Metal–organic frameworks for biomedical applications. Small 16 (10), e1906846. doi:10.1002/smll.201906846
Yang, Y., Wei, Y., Guo, Z., Hou, W., Liu, Y., Tian, H., et al. (2022). From materials to devices: graphene toward practical applications. Small Methods 6 (10), e2200671. doi:10.1002/smtd.202200671
Yang, Z., Sun, Z., Ren, Y., Chen, X., Zhang, W., Zhu, X., et al. (2019). Advances in nanomaterials for use in photothermal and photodynamic therapeutics (review). Mol. Med. Rep. 20 (1), 5–15. doi:10.3892/mmr.2019.10218
Younis, M. R., He, G., Qu, J., Lin, J., Huang, P., and Xia, X. H. (2021). Inorganic nanomaterials with intrinsic singlet oxygen generation for photodynamic therapy. Adv. Sci. 8 (21), e2102587. doi:10.1002/advs.202102587
Yu, X. T., Sui, S. Y., He, Y. X., Yu, C. H., and Peng, Q. (2022). Nanomaterials-based photosensitizers and delivery systems for photodynamic cancer therapy. Biomater. Adv. 135, 212725. doi:10.1016/j.bioadv.2022.212725
Yu, Y., Mei, L., Shi, Y., Zhang, X., Cheng, K., Cao, F., et al. (2020). Ag-conjugated graphene quantum dots with blue light-enhanced singlet oxygen generation for ternary-mode highly-efficient antimicrobial therapy. J. Mat. Chem. B 8 (7), 1371–1382. doi:10.1039/c9tb02300c
Yu, Y., Wu, S., Zhang, L., Xu, S., Dai, C., Gan, S., et al. (2022). Cationization to boost both type I and type II ROS generation for photodynamic therapy. Biomaterials 280, 121255. doi:10.1016/j.biomaterials.2021.121255
Yuan, Z., Lin, C., He, Y., Tao, B., Chen, M., Zhang, J., et al. (2020). Near-infrared light-triggered nitric-oxide-enhanced photodynamic therapy and low-temperature photothermal therapy for biofilm elimination. ACS Nano 14 (3), 3546–3562. doi:10.1021/acsnano.9b09871
Zhang, H., Jiang, W., Peng, Y., Yang, J., Chu, X., Long, Z., et al. (2022). Killing three birds with one stone: near-infrared light triggered nitric oxide release for enhanced photodynamic and anti-inflammatory therapy in refractory keratitis. Biomaterials 286, 121577. doi:10.1016/j.biomaterials.2022.121577
Zhang, P., Shao, C., Li, X., Zhang, M., Zhang, X., Sun, Y., et al. (2012). In situ assembly of well-dispersed Au nanoparticles on TiO2/ZnO nanofibers: A three-way synergistic heterostructure with enhanced photocatalytic activity. J. Hazard. Mater 237-238, 331–338. doi:10.1016/j.jhazmat.2012.08.054
Zhang, X., Zhang, G., Chai, M., Yao, X., Chen, W., and Chu, P. K. (2021). Synergistic antibacterial activity of physical-chemical multi-mechanism by TiO2 nanorod arrays for safe biofilm eradication on implant. Bioact. Mat. 6 (1), 12–25. doi:10.1016/j.bioactmat.2020.07.017
Zhang, Y., Yang, C., Yang, D., Shao, Z., Hu, Y., Chen, J., et al. (2018). Reduction of graphene oxide quantum dots to enhance the yield of reactive oxygen species for photodynamic therapy. Phys. Chem. Chem. Phys. 20 (25), 17262–17267. doi:10.1039/c8cp01990h
Zhang, Z., Wang, Y., Teng, W., Zhou, X., Ye, Y., Zhou, H., et al. (2021). An orthobiologics-free strategy for synergistic photocatalytic antibacterial and osseointegration. Biomaterials 274, 120853. doi:10.1016/j.biomaterials.2021.120853
Zhao, Q., Zhang, Z., Yan, T., Guo, L., Yang, C., Gao, G., et al. (2021). Synergism of carbon quantum dots and Au nanoparticles with Bi2MoO6 for activity enhanced photocatalytic oxidative degradation of phenol. RSC Adv. 11 (46), 28674–28684. doi:10.1039/d1ra05164d
Zhao, X., Tang, H., and Jiang, X. (2022). Deploying gold nanomaterials in combating multi-drug-resistant bacteria. ACS Nano 16 (7), 10066–10087. doi:10.1021/acsnano.2c02269
Zheng, Y., Zhu, Y., Dai, J., Lei, J., You, J., Chen, N., et al. (2023). Atomically precise Au nanocluster-embedded carrageenan for single near-infrared light-triggered photothermal and photodynamic antibacterial therapy. Int. J. Biol. Macromol. 230, 123452. doi:10.1016/j.ijbiomac.2023.123452
Zhi, D., Yang, T., O'Hagan, J., Zhang, S., and Donnelly, R. F. (2020). Photothermal therapy. J. Control. Release. 325, 52–71. doi:10.1016/j.jconrel.2020.06.032
Zhou, Y., Wang, Z., Peng, Y., Wang, F., and Deng, L. (2021). Gold nanomaterials as a promising integrated tool for diagnosis and treatment of pathogenic infections-a review. J. Biomed. Nanotechnol. 17 (5), 744–770. doi:10.1166/jbn.2021.3075
Zhou, Z., Zhang, L., Zhang, Z., and Liu, Z. (2021). Advances in photosensitizer-related design for photodynamic therapy. Asian J. Pharm. Sci. 16 (6), 668–686. doi:10.1016/j.ajps.2020.12.003
Zhu, K., Xia, W., He, D., Huang, J., He, H., Lei, L., et al. (2022). Facile fabrication of Fe/Fe3C embedded in N-doped carbon nanofiber for efficient degradation of tetracycline via peroxymonosulfate activation: role of superoxide radical and singlet oxygen. J. Colloid. Interface Sci. 609, 86–101. doi:10.1016/j.jcis.2021.11.178
Keywords: nanomaterial, photodynamic, photosensitizer, antibacterial, reactive oxygen species
Citation: Gao Y, Lin H, Luo Y, Li J, Gong C, Chen H and Gong R (2023) Nanomaterial-based photodynamic therapy for antibacterial applications: a comprehensive review. Front. Mater. 10:1260887. doi: 10.3389/fmats.2023.1260887
Received: 18 July 2023; Accepted: 24 August 2023;
Published: 04 September 2023.
Edited by:
Dongliang Yang, Nanjing Tech University, ChinaReviewed by:
Meng Ding, Nanjing University, ChinaZhiyong Song, Huazhong Agricultural University, China
Jia Chen, University of Macau, China
Copyright © 2023 Gao, Lin, Luo, Li, Gong, Chen and Gong. This is an open-access article distributed under the terms of the Creative Commons Attribution License (CC BY). The use, distribution or reproduction in other forums is permitted, provided the original author(s) and the copyright owner(s) are credited and that the original publication in this journal is cited, in accordance with accepted academic practice. No use, distribution or reproduction is permitted which does not comply with these terms.
*Correspondence: Renguo Gong, Z29uZ3Jna3FAMTYzLmNvbQ==