- 1School of Physics and Engineering Technology, Chengdu Normal University, Chengdu, China
- 2Faculty of Civil Engineering, Universiti Teknologi Malaysia, Kuala Lumpur, Malaysia
- 3Department of Civil, Environmental and Natural Resources Engineering, Luleå University of Technology, Luleå, Sweden
- 4Department of Civil Engineering, School of Engineering, Monash University Malaysia, Bandar Sunway, Selangor, Malaysia
- 5Department of Civil Engineering, COMSATS University Islamabad, Abbottabad, Pakistan
- 6Department of Civil Engineering, National Institute of Technology Calicut, Kozhikode, Kerala, India
Recent research has shown that the incorporation of fibres, such as steel and polypropylene fibres, in concrete can significantly improve its resistance to spalling under high-temperature conditions. However, the reported outcomes of studies on the spalling performance of Fibre Reinforced Concrete (FRC) vary significantly due to differences in cementitious matrix and fibre types, mix design, and testing techniques. Existing review studies have struggled to systematically and precisely consolidate the diverse aspects of the literature. To address these limitations, this paper adopts the latest approach for mining, processing, and analyzing data to interpret bibliographic data on the fire resistance of FRC. The primary objective of this study is to comprehensively explore the viability of FRC as a fire-resistant and refractory material. In pursuit of this goal, the paper thoroughly reviews various aspects of FRC behavior at elevated temperatures, including pore pressure behaviors. Moreover, this review also discusses spalling behaviors, mechanisms, and residual mechanical properties under high temperatures. The microstructural analysis of FRC is also discussed comprehensively to gain an in-depth understanding of its behavior under elevated temperatures. By analyzing available data, this study aims to shed light on the potential of FRC as a suitable material for resisting spalling in high-temperature scenarios. Additionally, the research delves into prospects and challenges in achieving sustainable FRC with enhanced spalling resistance, considering both material and structural levels.
1 Introduction
In civil engineering structures, concrete is a primary construction material that is susceptible to fire attack during the design life of that particular structure, resulting in severe damages, associated property loss, and even casualties (Figure 1). Several efforts have been made, such as fibre incorporation (Zheng et al., 2013; Sun et al., 2022), utilization of supplementary cementitious materials (Fang et al., 2022; Wang et al., 2022), geo-polymerization (Zhou et al., 2021), and addition of waste materials (Huang et al., 2022b; Sun et al., 2023), aiming to enhance concrete properties and progress towards sustainable development simultaneously. The researchers are more attentive toward sustainable supplementary cementitious materials for heading toward sustainable development (Khan et al., 2022b; Ahmad et al., 2023; Lao et al., 2023a; Lao et al., 2023b; Khan et al., 2023; Riaz Ahmad et al., 2023). In the near past, considerable interest has been developed globally towards the utilization of fibre reinforced concrete (FRC), as a construction material, in civil engineering structures, owing to the significantly improved mechanical characteristics in comparison to typical cementitious concrete-like; higher strength against compressive and tensile loadings (Farooqi and Ali, 2018c; Shi et al., 2022b), higher strength at first-crack (Branston et al., 2016; Farooqi and Ali, 2018a), higher fracture toughness (Khan and Ali, 2016; Solhmirzaei and Kodur, 2017; Shi et al., 2022a), higher resistance against impact loading (Yang et al., 2018; Yang et al., 2019a; Yang et al., 2019b), and significantly enhanced energy absorption capability (Farooqi and Ali, 2018a; Farooqi and Ali, 2019; Khan et al., 2022a; Farooqi and Ali, 2022). But, the mechanical characteristics of concrete can deteriorate if exposed to elevated temperatures due to physicochemical variations that occur during the heating process (Ma et al., 2015; Varona et al., 2018b; Han et al., 2023). Consequently, evaluating FRC mechanical characteristics at higher temperatures is crucial in dealing with fire threats (Amin and Tayeh, 2020; Huang et al., 2022d; Guo et al., 2022). The FRC performance under elevated temperatures is subjected to several parameters like; the addition of substitute materials and the incorporation of fibres as dispersed reinforcement (Kalifa et al., 2001; Han et al., 2005; Khaliq and Kodur, 2012; Serrano et al., 2016). It has also been reported in the literature (Sideris et al., 2009; Bangi and Horiguchi, 2011; Bošnjak et al., 2013; Choumanidis et al., 2016) that different types of fibre influence FRC residual properties after the exposure to elevated temperature.
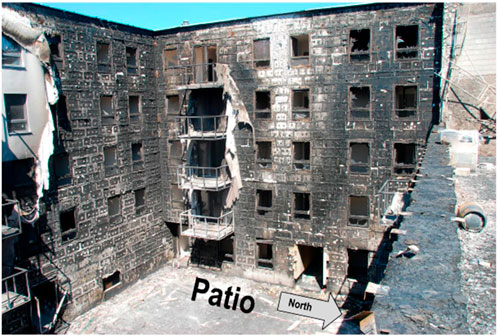
FIGURE 1. Building damaged due to fire (Wróblewski and Stawiski, 2020).
Polypropylene and steel fibres have been mostly explored (Huang et al., 2021; Zhang et al., 2022b; Sun et al., 2022). In addition, polymer fibre (Sim and Park, 2005; Arisoy and Wu, 2008; Hossain et al., 2013), glass fibre (Hossain et al., 2013; Khan and Ali, 2016), natural plant fibre (Savastano Jr et al., 2009; Farooqi and Ali, 2018b), and basalt fibre (Bhat et al., 2015; Khan et al., 2018; Khan et al., 2022a), have also been utilized, as dispersed reinforcement, in concrete to achieve various characteristics, like improvement in resistance against cracking and concrete toughness, exposure to elevated temperature (Colombo et al., 2010; Sukontasukkul et al., 2010; Huang et al., 2022a). The different types of fibres under general classification are shown in Figure 2. Fibres are broadly classified into two categories depending upon the concrete functions in terms of resistance against fire. One category comprising carbon, steel, and basalt fibres generally depicts virtuous mechanical characteristics at normal/room temperature and improved thermal stability at elevated temperatures. When incorporated into concrete, the fibres improve strength even against fire and resist the cracks’ initiation and propagation due to the bridging effect. The second group’s fibres, such as PVA and PP fibres, usually have lower melting points. After heating the FRC, having these fibres up to a specific temperature, melting fibres take place, ultimately resulting in water vaporization. Consequently, the decreased inner vapor pressure protects the concrete microstructure. Further, FRC’s temperature-based material characteristics also vary due to variations in fibre contents, size, shape, and orientation in a similar manner as at room temperature (Gao et al., 2012; Kim et al., 2013; Wu et al., 2016; Yoo et al., 2016).
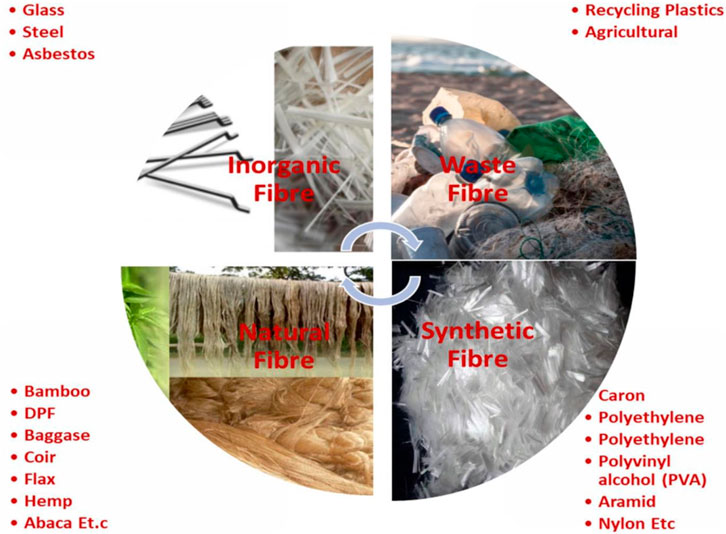
FIGURE 2. Generic classification of fibres (Adamu et al., 2022).
However, the utilization of natural fibres as a sustainable alternative to synthetic and steel fibres presents an exceptional opportunity to enhance the resistance of FRC against spalling significantly (Zhang et al., 2022a; Zhang et al., 2021b; Krishna et al., 2022). Natural fibres, such as hemp, jute, sisal, and coir, possess a multitude of advantageous properties, including renewability, low carbon footprint, and biodegradability (Khan and Ali, 2018b; Khan and Ali, 2019; Khan et al., 2022a; Farooqi and Ali, 2023), as illustrated in Figure 3 by Mohamad Moasas et al. (2022). When incorporated into the concrete matrix, these fibres effectively reinforce the structure, improving mechanical properties and heightened resistance to spalling, especially under elevated temperature conditions (Krishna et al., 2022). The unique attributes of natural fibres, such as their high tensile strength, thermal stability, and inherent fire-retardant qualities, make them exceptionally suitable for integration into FRC applications (Farooqi and Ali, 2019). Beyond their performance benefits, the adoption of natural fibres in FRC fosters a reduction in reliance on non-renewable resources while simultaneously contributing to a reduced environmental impact throughout the entire lifecycle of concrete structures (Ahmad et al., 2022; Boumaaza et al., 2022; Khatib et al., 2022; Shah et al., 2022). As the construction industry increasingly prioritizes sustainability, utilizing natural fibres as a sustainable alternative in FRC signifies a notable stride forward in achieving enhanced spalling resistance while promoting environmentally-friendly practices.
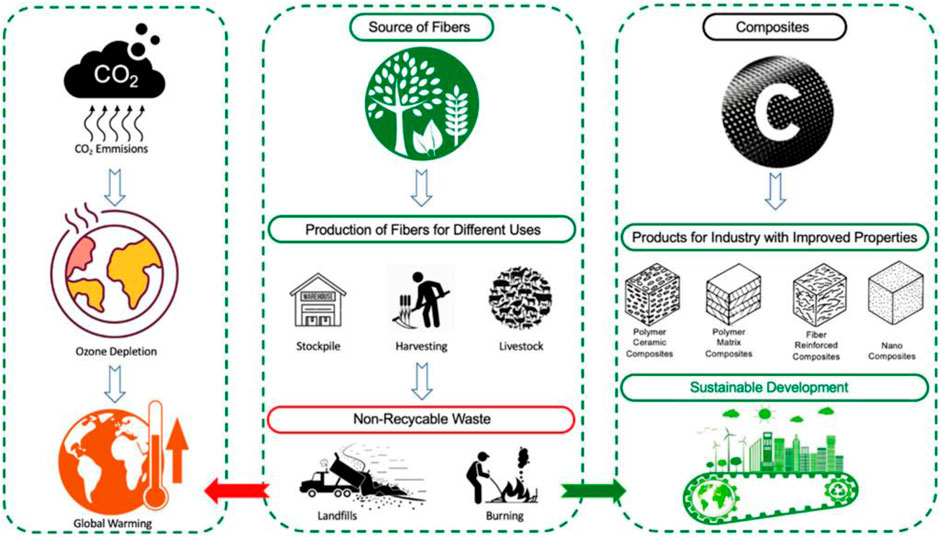
FIGURE 3. Concept of sustainable development by using fibres in concrete (Mohamad Moasas et al., 2022).
At the same time, the incorporation of supplementary materials, like fly ash (Xu et al., 2001; Afroughsabet et al., 2016; Jang et al., 2016; Jawahar et al., 2016; Abid et al., 2017), silica fume (Afroughsabet et al., 2016; Gupta et al., 2016; Jang et al., 2016; Abid et al., 2017; Ali et al., 2017) ground granulated blast furnace slag (GGBFS) (Afroughsabet et al., 2016; Jang et al., 2016; Jawahar et al., 2016; Abid et al., 2017), slag powder (Abid et al., 2017), and metakaolin (Afroughsabet et al., 2016; Abid et al., 2017) also plays a vital role on FRC performance at elevated temperature. Such supplementary materials usually generate a denser microstructure. Despite the improvement in strength, the internal vapor pressure is hard to release upon heating, which leads to concrete spalling (Behnood and Ziari, 2008). Concrete spalling has a considerable impact on its fire performance and may be a governing parameter in defining the fire resistance of an FRC structural member (Çavdar, 2013).
Moreover, the residual properties of concrete may also be affected by aggregates (Yang et al., 2009; Marques et al., 2013; Xie et al., 2015; Li et al., 2018; Ozawa et al., 2019). Varied chemical components of aggregate (Yang et al., 2009; Xie et al., 2015) and aggregate size (Li et al., 2018) would result in the varied performance of FRC. For instance, there is more risk of spalling in the case of siliceous aggregates at elevated temperatures compared to carbonate ones (Kodur and Dwaikat, 2008). In addition to the mix design of FRC, the heating curve design in testing material, like the highest temperature, heating rate, cooling method, and temperature holding time, would also considerably affect the FRC properties. The FRC microstructure would be more damaged in case of the highest heating temperature, leading to the FRC’s worse residual properties (Poon et al., 2004a). The heating rate is also critical for concrete spalling (Choe et al., 2019). The more severe spalling in FRC would be caused by fast heating, as per the fire curve of the ISO-834 standard (Zhao et al., 2017). Furthermore, the evaluated FRC compressive strength has decreased because of a longer holding time at peak temperature (Al Qadi and Al-Zaidyeen, 2014). Moreover, the different methods for cooling, such as water and nature cooling, may lead to varied residual strengths for FRC after being exposed to elevated temperatures (Luo et al., 2000). Figure 4 shows the condition of a concrete structure after firefighting.
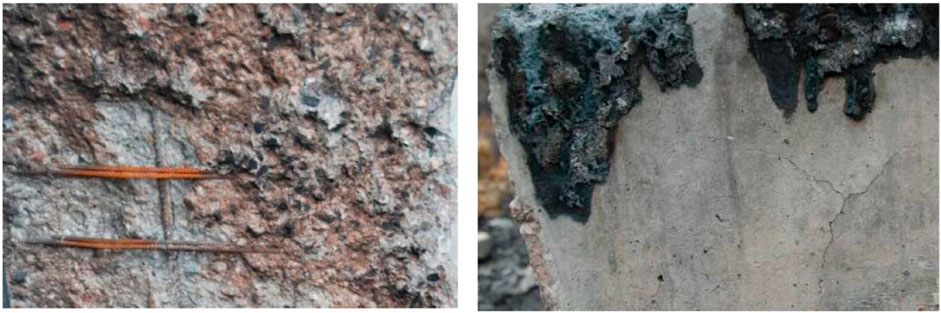
FIGURE 4. Concrete condition after firefighting (Wróblewski and Stawiski, 2020).
Extensive investigations have explored fibre reinforced concrete’s residual properties at high temperatures. However, its fire resistance remains uncertain, necessitating thorough investigation before using it in fire-prone structures. It is challenging to assess its significance compared to steel fibre reinforced concrete due to scattered research on polymeric/natural fibres. Understanding its performance in preventing spalling damage and mechanical deterioration under high temperatures is also lacking and complex due to variations in materials, mix design, and testing techniques. Additionally, available research outcomes on fibre reinforced concrete’s fire performance vary significantly due to differences in the cementitious matrix and fibre types. Synthetic fibres like steel and polypropylene have shown noteworthy improvements in spalling resistance under high-temperature conditions. Conventional review studies have limitations in systematically and precisely connecting various aspects of literature. To address this issue, this study adopts a novel approach to mine, process, and analyze bibliographic data concerning fibre reinforced concrete fire resistance. This methodology comprehensively explains the material’s potential as a refractory material. In this study, we utilize an innovative review method with specialized software, which helps overcome these limitations. Our focus is on conducting a scientometric analysis of bibliographic data related to the fire resistance applications of FRC, aiming to establish cohesive links between different literature aspects that are challenging to achieve through traditional review-based studies. For recent research, co-occurrence, scientific visualization, and co-citations are highly complex features (Ahmad et al., 2021a; Amin et al., 2022; Zhang et al., 2022a; Huang et al., 2022c). The analysis reveals the primary publication sources and authors with the highest number of articles and related citations, the co-occurrence of keywords, noteworthy contributing countries, and the most cited articles in the FRC fire resistance applications field. Furthermore, a comprehensive review thoroughly investigates the feasibility of FRC as a material with fire resistance and refractory properties. This in-depth exploration covers various aspects, including pore pressure analysis, spalling behavior and mechanism, residual mechanical properties, and microstructural characteristics of FRC. Additionally, the study extensively reviews existing literature concerning the performance of FRC in terms of spalling under elevated temperature conditions. Moreover, the study examines the potential for sustainable FRC with improved spalling resistance, exploring prospects and challenges in this regard. The statistical and graphical representation of bibliometric data facilitates knowledge exchange among young scientists, enabling them to share state-of-the-art concepts and methods and collaborate on joint ventures.
2 Methodology
In the current study, a scientometric analysis is performed on bibliographic data (Zhong et al., 2019; Darko et al., 2020; Afgan and Bing, 2021; Antwi-Afari et al., 2021; Amin et al., 2022; Huang et al., 2022c) to quantify its numerous properties. Scientific mapping is used by scientometric analysis, a method researchers invented to explore bibliometric data (Markoulli et al., 2017; Amin et al., 2022). As several articles are available in the understudied research area; therefore, the engagement of a reliable search engine is necessary. Scopus and Web of Science are the two highly suggested databases to attain the study objective (Afgan and Bing, 2021; Huang et al., 2022c). Accordingly, Scopus is used to collect bibliographic data relevant to the fire resistance application of FRC research. Several filters are also employed to scrutinize irrelevant data. A comprehensive flowchart depicting all the methodical steps, like retrieving data, data analysis, and applying different filters in the analysis, is shown in Figure 5.
In literature, several types of research have reported the employment of the same method (Oraee et al., 2017; Jin et al., 2018; Park and Nagy, 2018). The Scopus database’s stocking is done using CSV format to perform added evaluation by employing suitable software. An open-source, freely accessible mapping tool, i.e., VOS viewer (version 1.6.18), is applied to conduct the quantitative assessment and scientific visualization of gathered data (Zuo and Zhao, 2014; Darko et al., 2017; Ahmad et al., 2021b). Thus, the objectives of this research are accomplished by using a VOS viewer. The subsequent CSV file is updated on the VOS viewer and added assessment is done on data consistency and dependability. The scientometric analysis evaluates widely used keywords, publication sources, the authors having the most citations and articles, the contributions of different countries, and the highly cited papers. The features, their co-occurrences, and interconnectivity are developed using maps. To identify a particular element in the map, allocation of colors is done for the groups. Likewise, specific colors, such as rainbow, depict density mapping, and plasma, rainbow, and Viridis are used for density visualization.
3 Results and analysis
3.1 Relevant subject areas and annual publications
A Scopus analyzer is used to identify the most relevant research areas. Figure 6 shows that most documents, i.e., 49%, are generated in the “Engineering” discipline, followed by “Materials Science” with almost 32% of documents. As a whole, 81% of papers are generated by these two disciplines. Furthermore, the analysis of the Scopus database is also conducted to determine the type of publication in the understudied research domain (Figure 7). It is extracted from this evaluation that the percentage of journal articles is 58, 27% are conference articles, and approximately 10% are conference review papers. It is pretty inspiring to note that the fire resistance application of FRC is gaining the researchers’ attention.
3.2 Sources of publications
VOS viewer is applied to bibliographic data to determine the sources of publication. The most active journals in the area of fire-resistant application of FRC are “Construction and Building Materials,” “Engineering Structures,” “Applied Mechanics and Materials,” and “Composites Part B: Engineering.” “Journal of Structural Fire Engineering” also receives considerable citations in the same context. Figure 8 shows the visualization mapping for publication sources having a minimum of five articles. The circle dimensions demonstrate the impact of the source on this research field in terms of document count, depicting that the bigger the circle, the more the effect is. In this scenario, it may be noted that “Construction and Building Materials” has a bigger circle than others, showing its greater impact in the fire resistance application of the SFRC research area. Four groups are formed, and a specific color is allocated to every group on the map (red, green, blue, and yellow). The level of publication source and co-citation frequency is the basis for forming each group (Wuni et al., 2019). The group formation for journals is made by applying VOS viewer, depending upon the capacity of co-citation in published articles. For example, in the green group, eight journals have recurrent co-citations in the same field of research. Moreover, the distance between each group shows the linkage between publication sources. As can be seen, “Construction and Building Materials” is more closely related to “Journal of Materials in Civil Engineering” as compared to “Journal of Building Engineering” or “Cement and Concrete Composites.”
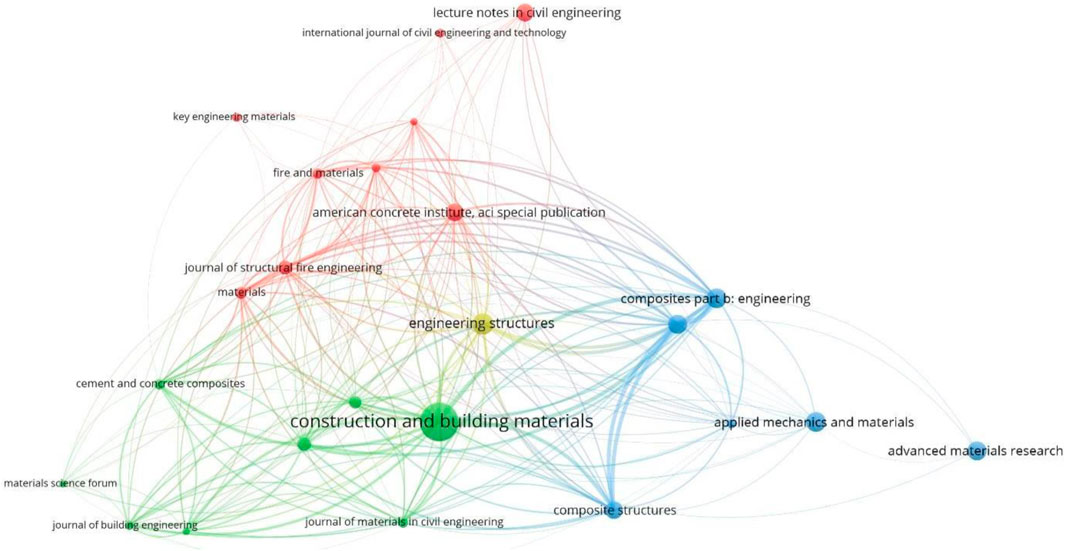
FIGURE 8. Scientific visualization for sources of publication having a minimum of five publications in the relevant research domain.
3.3 Keyword cooccurrence
In research, the keywords are essential for differentiating and emphasizing the main theme of a specific research study (Song et al., 2021). “Fire Resistance,” “Reinforced Concrete,” “Concrete,” “Fires,” and “Steel Fibres” are among the most widely utilized keywords in the fire resistance application of the FRC research field. It is found from the analysis of keywords that fire resistance applications of FRC have been explored to have fire resistance materials. Figure 9 illustrates a map for the frequency of occurrence depending on density and connections. Once again, in Figure 9A, the circle dimensions for a particular keyword represent the keyword frequency, and the circle’s position depicts its co-occurrence in articles. Moreover, the circle size is relatively bigger in the case of leading keywords than others, depicting their impact in exploring literature on the fire resistance application of FRC. Again, the group formation represents keywords co-occurrences in various publications. Several colors are assigned to each distinct group to indicate the co-occurrences of keywords in published documents. The different colors show four discrete groups (Figure 9A). Likewise, diverse, unique colors signify the density concentrations of the keyword (Figure 9B). The color schemes are organized based on density like green being applied for maximum density followed by blue, red, yellow, and purple colors representing further lesser densities. Fire resistance and concretes are important keywords shown by green colors, demonstrating their higher density of occurrences. This finding would benefit young scholars in selecting keywords that can minimize the efforts to explore this particular research area-relevant publications.
3.4 Authors
The impact of any author in a particular research area is revealed from their citations (Yu and Hayes, 2018). An author’s standing is difficult to get by simultaneously considering all the parameters like publication quantity, citations, etc. Instead, the assessment of their ranking may be done for each parameter independently. Hence, it is revealed from the analysis that Kodur V., Correia J.R., Firmo J.P, and Green M.F.are among the prominent researchers in the considered field, i.e., fire resistance application of the FRC research field. Figure 10 shows the connectivity among different authors having a minimum of five publications. It is summarized in this analysis that there is substantial inter-connectivity between authors in terms of citations who are working on FRC fire resistance application.
3.5 Documents
The importance of a particular research paper in a specific research field is revealed from the received citations. The pioneer articles in a particular research domain have the highest citations. The density concentration and scientific visualization of the citations for inter-related articles in fire resistance application of FRC research are presented in Figure 11. The connected articles based on citations extracted from the VOS viewer are presented in Figure 11A. The circle size for a particular article is directly related to the impact of that article in the considered research domain. Furthermore, the enhanced density concentrations for the leading articles based on citations are extracted from the density mapping, as depicted in Figure 11B.
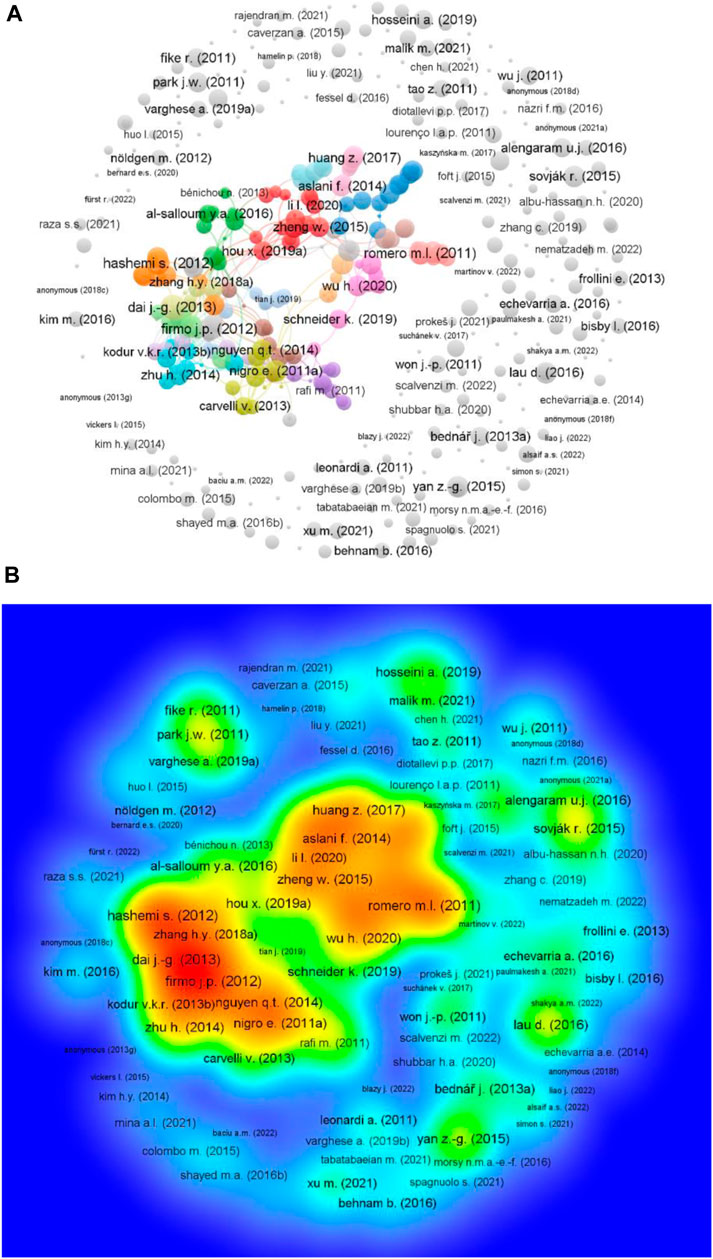
FIGURE 11. Scientific mapping of citations for published papers in considered research area till 2022; (A) Citations based connected articles (B) Connected articles density.
3.6 Countries
The contribution of some countries in terms of research on fire resistance application of FRC is comparatively significant. Systematic mapping is developed for bibliophiles to assess the most contributing countries on fire resistance application of FRC research to predict its characteristics. It may be noted that China, the United States, and India are among the leading countries. Figure 12 depicts the citation mapping depending on the inter-connected countries and density concentration visualization. The respective circle’s dimension shows a specific country’s impact on the considered research field (Figure 12A). Similarly, it is extracted from Figure 12B that countries with more publications show higher density. Representing statistical and graphical output for contributing countries would benefit young researchers in developing scientific collaborations joint ventures, and exchanging novel/innovative concepts/techniques. Academics from various countries interested in working on the fire resistance application of FRC may team up with related field professionals to benefit from their expertise.
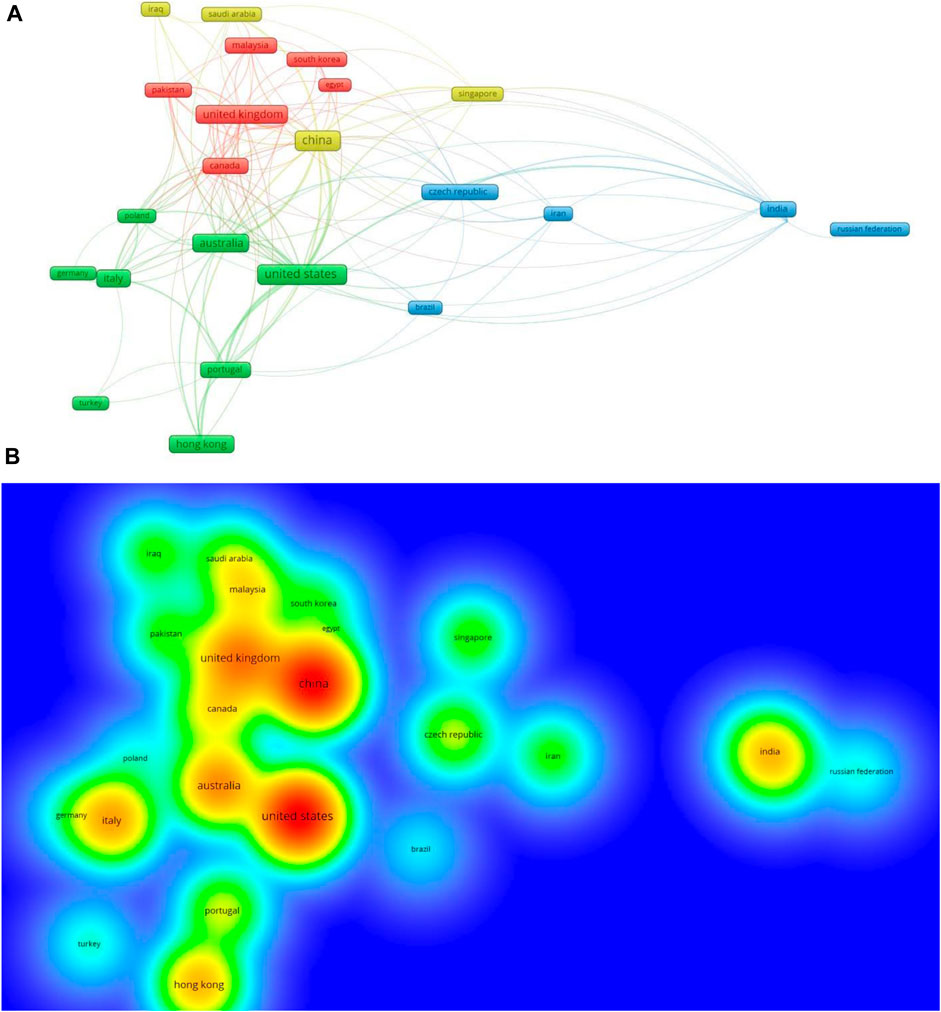
FIGURE 12. Scientific visualization of countries in considered research domain till 2022: (A) Network visualization; (B) Density visualization.
4 Spalling behavior of FRC at elevated temperature
4.1 Behavior of FRC at high temperature
Concrete is a widely used construction material worldwide, with annual production exceeding ten billion tons (Meyer, 2009). Anticipations suggest that the demand for concrete will reach 18 billion tons by 2050 (Monteiro, 2006). Concrete finds extensive applications in structures such as buildings, bridges, parking structures, and industrial pavements. Due to the ongoing progress in the construction and infrastructure sectors, there has been a rapid surge in the construction of tall buildings and underground structures. Nevertheless, the vulnerability of these edifices to fire has escalated due to the extensive utilization of gas and electrical appliances, along with the intricate nature of their functionalities (Zhang et al., 2020c). Over the past few years, there has been a noticeable rise in the number of fire incidents in such structures across the globe. These incidents pose a significant risk to the safety of individuals and properties. Consequently, researchers have begun to give more attention and engage in discussions regarding the residual performance of concrete when subjected to high temperatures, as well as the mechanical properties of concrete under such conditions (Noumowe et al., 1995; Arioz, 2007). Studies have revealed that when exposed to elevated temperatures or fire, concrete undergoes various physical and chemical reactions, such as dehydration and decomposition. The elevated temperature can impact the durability and strength of concrete structures, as it induces physical deterioration processes (Huang et al., 2017; Chidighikaobi, 2019). These reactions not only cause significant harm to the microstructure of concrete but also reduce its strength and stiffness. Consequently, this can lead to explosive spalling and a decline in the mechanical integrity of the concrete (Handoo et al., 2002; Li et al., 2004; Caetano et al., 2019; Krishna et al., 2019). Kim et al. (2023) evaluated the effect of natural minerals, i.e., ‘hwangto’ in concrete at elevated temperatures. The mechanism of such concrete at elevated temperatures is shown in Figure 13, as presented by Kim et al. (2023).
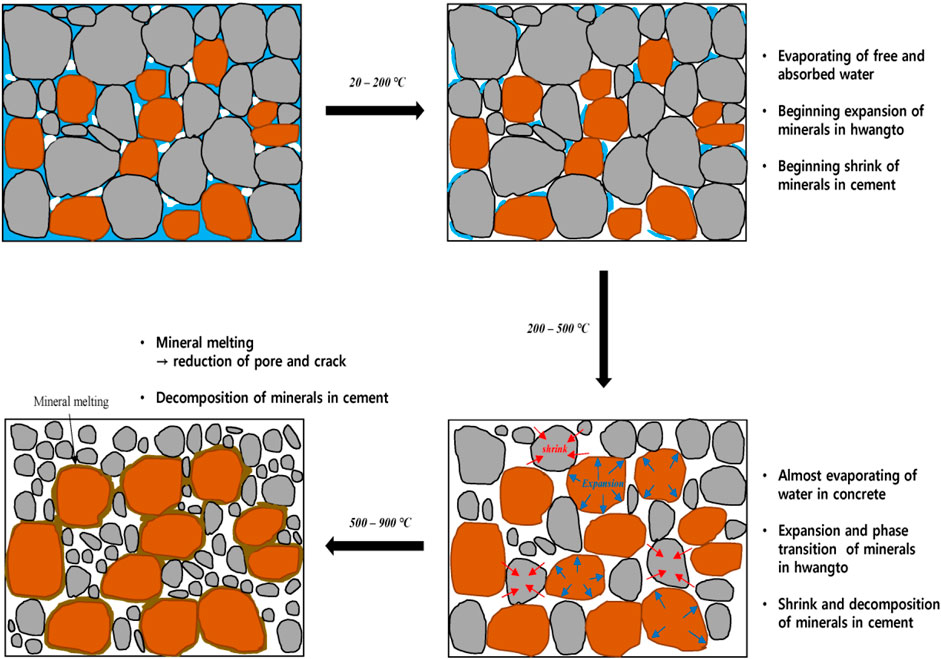
FIGURE 13. Concrete mechanism at elevated temperature (Kim et al., 2023).
After being exposed to high temperatures, concrete’s chemical composition and physical properties can undergo alterations. This can lead to permanent damage within the structures, affecting the serviceability and safety of concrete. In severe cases, such damage can even result in the collapse of the structure (Arioz, 2007; Huang et al., 2017; Chidighikaobi, 2019). Multiple prior research endeavors have demonstrated that the build-up of vapor pressure within concrete pores, coupled with thermal stresses, plays a crucial role in the mechanism of strength degradation and the occurrence of explosive spalling during fire incidents. These factors greatly compromise the mechanical properties of concrete (Janotka and Bagel, 2002; Fu et al., 2006; Peng et al., 2006; Ma et al., 2015; Chen et al., 2016). Heat-induced spalling is an irreversible form of damage that concrete experiences when exposed to high temperatures (Zhang et al., 2020b; Holan et al., 2020). This phenomenon arises from the development of thermal stresses and rapid evaporation of water, increasing pore pressure (Liu et al., 2018). It is a widely recognized fact that concrete, as a heterogeneous and multi-scale composite material, acquires its properties from the various elements it comprises. When subjected to fire, these constituent ingredients undergo disintegration caused by the phenomenon referred to as ‘thermal inconsistency of the ingredients,’ resulting in a decline in mechanical properties (Sancak et al., 2008). The construction industry has made significant advancements in research and development, resulting in high-performance concrete mixes that exhibit enhanced microstructure and improved mechanical properties, including increased strength, toughness, and durability. Nevertheless, recent studies have revealed that these high-performance concretes do not possess equivalent fire endurance compared to traditional concrete (Kodur and Khaliq, 2011; Hulin et al., 2016; Xiao et al., 2017). Changes in the microstructure of concrete occur in correspondence with the heating process, ultimately resulting in structural failure of the concrete. These modifications begin at the onset of the heating process, even at a temperature increase as low as 105°C (Hager, 2013). Nonetheless, concrete can generally be regarded as relatively secure and stable at temperatures ranging from 200°C to 300°C (Ma et al., 2015), minimizing potential hazards. However, as the temperature rises over a specific exposure period, irreversible changes occur in the concrete microstructure, resulting in structural damage (Cree et al., 2013). Cree et al. (2013) examined the physical and chemical changes that occur in concrete across different temperature ranges, as presented in Figure 14.
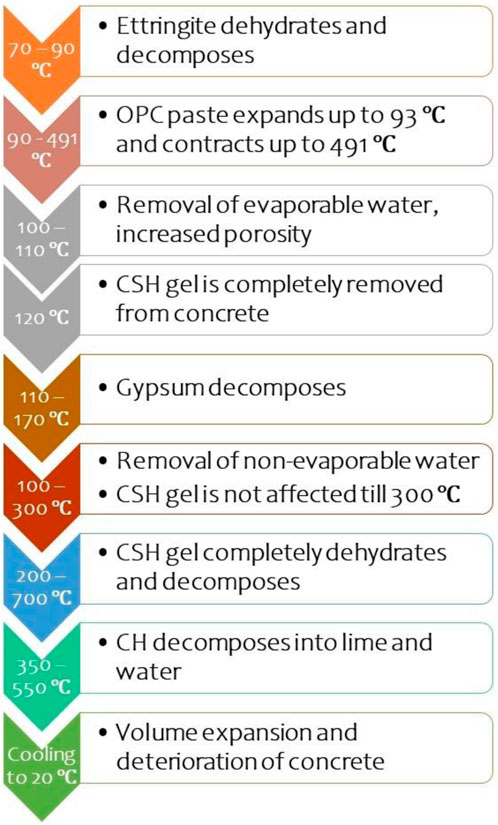
FIGURE 14. Effects of various temperatures on concrete [Statistics by Kim et al. (2023)].
Thermal conductivity, thermal diffusivity, and specific heat are regarded as fundamental thermo-physical properties of concrete. Among these, thermal conductivity is paramount as the principal factor impacting heat conduction within concrete (Bhattacharjee and Krishnamoorthy, 2004). Using concrete with a low thermal conductivity contributes to reducing heat transfer and energy consumption in buildings. Thermal conductivity refers to a material’s ability to conduct heat. When examining heat transfer, steady-state and transient conditions are recognized as distinct modes of heat transfer across various materials (Bindiganavile et al., 2012). Concrete is a solid material that exhibits heterogeneity and permeability. At typical operational temperatures, heat transfer within concrete primarily occurs through conduction. The properties of concrete are significantly influenced by its constituents’ distribution and volume ratio, including aggregate, water-cement ratio, and voids (Asadi et al., 2018). The presence of voids within concrete plays a crucial role in shaping its mechanical and thermal characteristics (Chung et al., 2016). Variations in specific properties of concrete give rise to distinct thermal conductivity values. Kim et al. (2003) investigated the impact of seven variables on the thermal conductivity of cement paste, mortar, and concrete. These factors include the humidity level of the specimen, age, temperature, water-cement (w/c) ratio, fine aggregate fraction, type of admixture, and total volume fraction of aggregates. The primary influential factors on the thermal conductivity of concrete were found to be the condition of the specimen, moisture, and the volume fraction of aggregates. Past research has indicated that the thermal conductivity value of concrete is predominantly influenced by various factors such as the aggregate type, moisture content, density, percentage of cement paste, porosity, presence of supplementary cementitious materials (SCMs), environmental temperature, and the specific measurement method and equipment utilized (Bentz et al., 2011; Shin and Kodide, 2012).
4.2 Pore pressure of FRC at high temperatures
Pore pressure accumulates from moisture movement up to temperatures reaching 320°C. The moisture content within concrete exists in various states: free, adsorbed, and bonded (Rawat et al., 2021). During stage I (ranging from 30°C to 100°C), moisture can be attributed to the evaporation of unbound water. In stage II (ranging from 100°C to 300°C), the moisture content is derived from a combination of unbound water, absorbed water, a portion of chemically bonded water, and inter-layered water originating from the C-S-H gel. During stage III (ranging from 300°C to 500°C), moisture results from the decomposition of Ca (OH)2, forming CaO and H2O. As for stage IV (ranging from 500°C to 700°C), any remaining moisture within the C-S-H layers will be released due to the decomposition of C-S-H. Spalling at high temperatures is primarily attributed to thermally induced stress (Liu et al., 2018). This stress arises from the temperature disparity between the surface and interior of UHPC when exposed to elevated temperatures. Several studies suggest that both pore pressure and thermally induced stress contribute to spalling (Liu et al., 2018), working in conjunction. Between the 220°C–320°C temperature range, thermo-hydraulic spalling transpires due to thermally induced stress and pore pressure. This specific type of spalling is triggered by the expansion of evaporated pore water caused by elevated temperatures, leading to heightened internal pressure that can result in cracking and spalling. During this stage, a portion of the C-S-H undergoes dehydration.
One potential approach to enhance the resilience of concrete against high temperatures is introducing fibre reinforcement. Extensive research has confirmed that the inclusion of randomly dispersed fibres in concrete leads to a notable enhancement in its compressive strength, tensile strength, ductility, and impact resistance under ambient conditions (Romualdi et al., 1968; Ding et al., 2012; Eik et al., 2015; Abdallah et al., 2016). Fibre reinforced concrete (FRC) finds broad applications in construction, including ground-supported slabs, road paving, and tunnel linings, thanks to its remarkable ability to improve concrete’s tensile strength and effectively manage crack propagation (Laranjeira et al., 2010; Zīle and Zīle, 2013; Abdallah et al., 2016). Hence, in recent years, there has been a growing interest in studying the impact of fibres on the remaining mechanical properties of concrete following exposure to high temperatures and their influence on concrete’s susceptibility to explosive spalling during fires or elevated temperatures. Numerous research studies have been carried out to investigate the residual properties and explosive spalling behavior of FRC (Zheng et al., 2013; Varona et al., 2018b; Li et al., 2019b). During a fire or high-temperature exposure, fibres in concrete have several beneficial effects. It helps to decrease water vapor pressure within concrete pores, bridges internal cracks, and mitigates temperature gradients by facilitating heat absorption into the concrete. These factors collectively reduce the risk of explosive spalling and cracking, thereby enhancing the fire resistance of concrete (Purkiss, 1984; Faiyadh and Al-Ausi, 1989b; Poon et al., 2004b; Gao et al., 2012). Furthermore, there is substantial potential for significant enhancement in the residual mechanical properties of concrete in a post-fire setting (Purkiss, 1984; Faiyadh and Al-Ausi, 1989a; Lau and Anson, 2006). However, inconsistencies in international regulations and standards hinder the widespread adoption of FRC in high-temperature environments.
Incorporating natural or artificial fibres is a proven method to enhance the strength of composites, both at room temperature and following exposure to high temperatures (Khan and Ali, 2016; Khan and Ali, 2018a; Farooqi and Ali, 2018c; Cao et al., 2019; Khan and Ali, 2019; Khan et al., 2020; Farooqi and Ali, 2022). In the construction industry, there has been significant progress in utilizing hybrid fibre reinforced concrete (HFRC) in recent decades (Machovič et al., 2013; Li et al., 2020). Moreover, combining basalt fibre with synthetic fibres represents a novel approach with vast potential applications within hybrid fibres (Machovič et al., 2013). Earlier investigations have demonstrated that incorporating basalt fibre into composites substantially improves their mechanical properties and toughness. Basalt fibre is a compatible reinforcing material for cementitious composites (Jiang et al., 2014; Campione et al., 2015; Yang et al., 2019c; Khan and Cao, 2021). Steel fibres exhibit superior thermal conductivity and thermal stability, along with crack-resistant characteristics that mitigate thermal stress, even at elevated temperatures. These properties arise from steel fibres’ effective heat transfer phenomena (Cao et al., 2019). Steel fibres offer a bridging effect in fibre reinforced concrete across a wide temperature range and retain their structural integrity even at temperatures below 1,300°C (Deshpande et al., 2019). Moreover, the micro-level performance of calcium carbonate (CaCO3) whiskers, functioning as micro-fibres, has been notable, and they decompose within the range of 800°C–900°C in composites (Cao et al., 2019).
4.3 Spalling behavior of FRC at high temperature
Spalling in concrete occurs due to the interplay of thermal, hydraulic, and mechanical degradation processes (Gawin et al., 2005). When exposed to fires or high temperatures, concrete undergoes substantial deterioration in its mechanical properties, leading to a rapid decline in the bearing capacity of concrete elements. This poses a significant threat to concrete structures’ overall safety and stability (Fu et al., 2006). The concrete’s explosive spalling phenomenon at elevated temperatures is attributed to two primary factors, which have been extensively recognized in the literature. One of the factors contributing to the accumulation of pore pressure in concrete is the internal vaporization of water within the material (Chen et al., 2016; Li et al., 2021a). Free water, water chemically bonded in calcium hydroxide (CH), and interlayer water in C-S-H all coexist in concrete. The evaporation of free water begins at about 100°C, which causes the pore pressure within the matrix to rise. The dehydration process affects the interlayer and chemically bonded water in the C-S-H gel as the temperature increases from 300°C to 400 °C. Subsequently, between 400°C and 500°C, calcium hydroxide dehydration occurs, leading to concrete shrinkage and a significant reduction in strength. Ultimately, at approximately 900°C, the C–S–H gel undergoes complete decomposition (Fletcher et al., 2007; Henry et al., 2014; Lim, 2015). The free and chemical water in the concrete’s pore structure undergoes evaporation with the temperature rise. While a significant portion of the water evaporates and moves towards the center of the heated concrete, some are ejected to the surface. Due to the lower temperature inside the concrete, the vapor gathers and starts to condense. Over time, a saturated layer starts to develop within the concrete, hindering the movement of vapor toward its inner parts. Consequently, the vapor preferentially migrates toward the concrete’s surface and is released into the atmosphere on a larger scale. This leads to a gradual increase in pore pressure within the porous network, and if the tensile stress of the concrete cannot withstand this pressure, it results in the explosive spalling of concrete (Consolazio et al., 1998; Kalifa et al., 2000). By taking measurements of the temperature field and pore pressure, Kalifa et al. (2000) verified that a quasi-saturated layer in concrete existed before the drying front. Furthermore, it has been observed that concrete with lower permeability develops this saturated layer more rapidly, and it is located closer to the heated surface, resulting in higher pore pressure and pressure gradient. A graphical representation of the entire process of concrete spalling with and without fibre caused by pore vapor pressure is illustrated in Figure 15, as presented by Hager and Mróz (2019a).
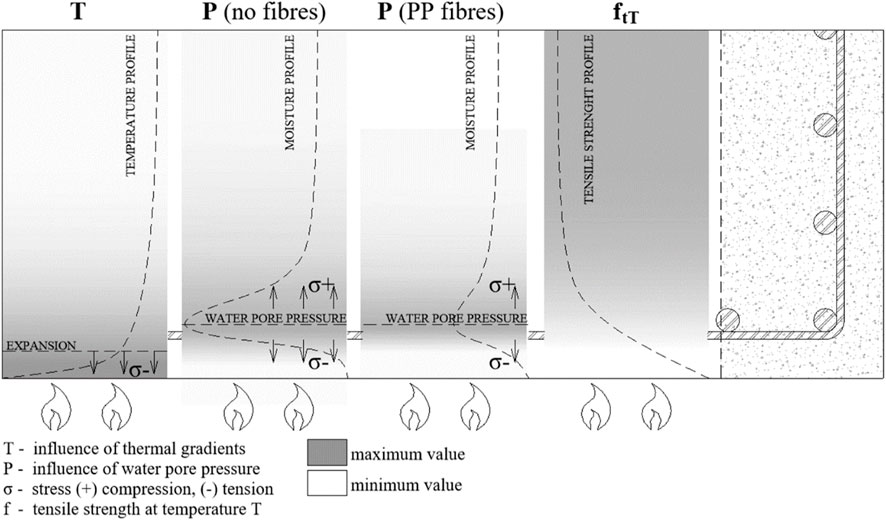
FIGURE 15. Mechanism of pore pressure distribution and spalling at elevated temperature in concrete with and without fibres (Hager and Mróz, 2019a).
The development of cracks brought on by thermal strains brought on by temperature gradients is the second reason causing the concrete to spall (Chen et al., 2016). A thermal gradient, also called “thermal shock,” is produced between the heated surface and the inside of the concrete when exposed to high temperatures or flames. Rapid increases in the surface temperature of concrete lead to its shrinkage, resulting in the generation of compression stress parallel to the heated concrete’s surface. Additionally, tensile stress is induced perpendicular to the surface. The critical point for concrete spalling lies when the compressive stress surpasses the tensile stress (Debicki et al., 2012; Ozawa et al., 2012). The mechanism for thermal stress-induced explosive spalling in concrete is shown in Figure 16. Additionally, heterogeneity is brought on by the different ways that different concrete components behave at high temperatures, resulting in considerable thermal cracking at the interfaces (Varona et al., 2018a). The porous network makes it easy for water vapor to move through it and quickly evaporate. The moisture blockage forms earlier and closer to the heated surface in denser and less porous concrete. As shown in the pictogram in Figure 17 a nearly impenetrable layer that is entirely saturated forms (Hager and Mróz, 2019b). Over time, a dry zone emerges in the concrete subjected to the most significant temperature rise, where the cement paste undergoes dehydration. After that, a drying zone where no free water is left forms next to the saturated and impermeable concrete layer.
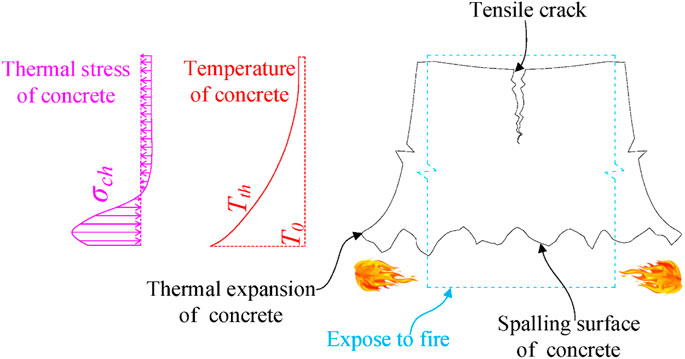
FIGURE 16. Mechanism of concrete spalling due to thermal stress (Duan et al., 2023).
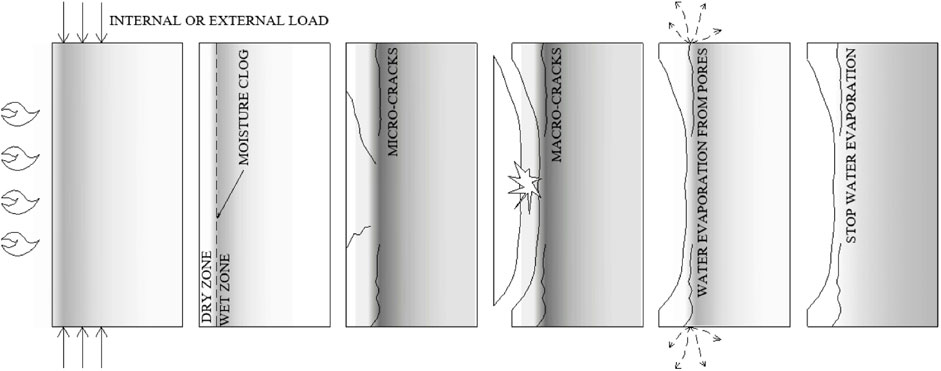
FIGURE 17. Evolution of hydraulic processes in fire-exposed concrete (Hager and Mróz, 2019b).
The impact of steel fibres on concrete’s resistance to explosive spalling under high temperatures or fires is still uncertain. Several studies (Hertz, 1992; Aydın et al., 2008; Sideris et al., 2009) have suggested that adding steel fibres did not decrease the concrete’s tendency to undergo explosive spalling. Using experimental data, Hertz (1992) concluded that adding steel fibres did not reduce the risk of explosion. Surprisingly, specimens with a larger concentration of steel fibres showed a higher likelihood of explosive spalling. Contrary to this conclusion, some investigations have suggested that using steel fibres may help prevent explosive spalling in concrete exposed to high temperatures. This is mainly due to the steel fibres’ contribution to lowering the pore pressure within the concrete at high temperatures. Peng et al. (2006) provided evidence of steel fibres’ ability to mitigate the accumulation of steam pressure. Bangi and Horiguchi (2012) reported that incorporating steel fibres in heated concrete enhanced its resistance to pore pressure. In addition, the application of steel fibres decreased the pore pressure in high-strength concrete specimens exposed to high temperatures, according to Düğenci et al. (2015) and Bangi and Horiguchi (2012). Furthermore, the influence of steel fibres in decreasing pore pressure in the inner regions of concrete was notably more pronounced during rapid heating than gradual heating. This is explained by the fact that steel fibres have better thermal conductivity than cement substrates and coarse aggregates. As a result, cracks brought on by thermal gradients within the concrete are diminished as heat is more evenly distributed throughout the steel fibre reinforced concrete. Felicetti (2007) found that the heat diffusion coefficient of SFRC was higher than that of traditional concrete in his investigation. Additionally, it was found by Gao et al. (2012) and Zheng et al. (2013) that adding steel fibres improved heat transport within the concrete, resulting in fewer temperature gradients and lessening the effects of thermal shock at the concrete surface. This result is consistent with earlier studies by Zhang and Ju (2005) and Zheng et al. (2012b). Due to its ability to lower temperature gradients, SFRC demonstrated remarkable resistance to explosive spalling. Moreover, the incorporation of steel fibres has been shown to enhance the tensile properties of concrete. Concrete’s tensile strength is essential in preventing internal vapor pressure and thermal stress brought on by high temperatures, thus protecting it from explosive spalling. Additionally, the random distribution of steel fibres within the concrete matrix contributes to reduced crack tip opening displacement, leading to delayed crack formation and restricted crack propagation. Consequently, this diminishes the likelihood of explosive spalling, as supported by previous findings (Zheng et al., 2013). Under high-temperature conditions, the accumulation of pore pressure and thermal stress brought on by temperature gradients is the main cause of explosive spalling in concrete. Hossain et al. (2021) reported the exposure of hybrid and polyvinyl alcohol fibre reinforced concrete specimens to 60 cycles of high temperature (i.e., 175°C). Figure 18 illustrates the specimen condition of hybrid and polyvinyl alcohol fibre reinforced concrete spalling after exposure to 60 cycles of set temperature and the cracking pattern on the spalled specimens. The reported basic cause for concrete spalling is the dense microstructure, that do not allow moisture to escape upon exposure to elevated temperatures. By incorporating fibres into the concrete mix, the risk of such explosions can be mitigated. This is achieved through improvements in the concrete’s thermal conductivity and tensile properties, as well as the reduction of pore pressure at elevated temperatures.
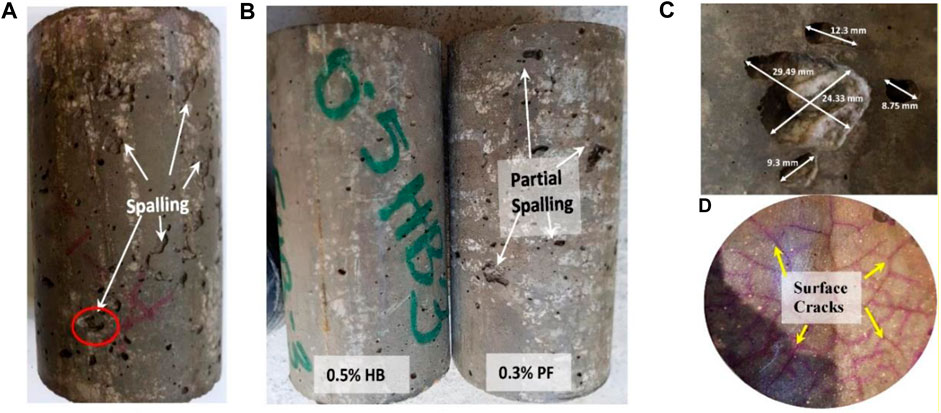
FIGURE 18. Concrete spalling (A) reference specimens, (B) slight spalling in hybrid fibre reinforced specimen and partial spalling in polyvinyl alcohol fibre reinforced specimen, (C) spalled reference specimen, and (D) cracking at spalled specimens (Hossain et al., 2021).
5 Spalling mechanism of FRC at elevated temperature
The working mechanisms of different fibres in cementitious composites may vary. The different spalling damages observed in concrete at elevated temperatures are shown in Figure 19. Two mechanisms have frequently been reported in the literature to explain how different fibres work in concrete, i.e., vapor migration via pressure-induced tangential space (Khoury and Willoughby, 2008) and vapor migration via melting of fibres (Zhang et al., 2021a). Khoury and Willoughby (2008) first proposed this mechanism to explain the working mechanism of PP fibre. The polarity difference between PP fibre and matrix produces poor/weak interfacial adhesion. This weak bond region, called PITS, is susceptible to disruption by steam pressure at high temperatures. PITS can create a space for water vapor migration and offer a pressure release route even before fibre melting. Besides PP fibre, it has been reported that this mechanism also exists in steel fibre and jute fibre reinforced concrete. Ozawa et al. (2019) stated that the carbonation of the straw-like structure of jute fibre results in PITS between the fibre and matrix interface. The release of vapor pressure through PITS of steel fibre was also stated in a previous study (Li et al., 2021b). In summary, the PITS mechanism provides a viable explanation for the working mechanism of different fibres in concrete, which can help to improve spalling performance. The mechanism of PITS is the same regardless of the type of fibre used.
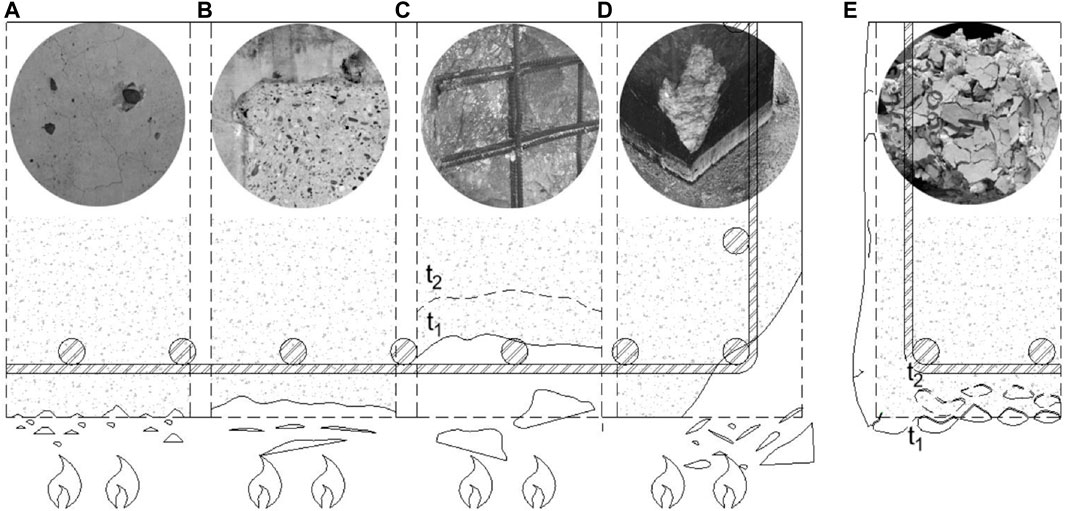
FIGURE 19. Manifestations of damage observed in diverse concrete types during fire exposure (A) aggregate spalling, (B) continuous and progressive surface spalling, (C) discrete and progressive explosive spalling, (D) corner spalling, (E) surface peeling during cooling (Hager and Mróz, 2019b).
However, in the case of vapor migration through the channels formed by the melting of fibres, Table 1 enlists the melting points of various types of fibres. Specifically, LLDPE, UHMWPE, PP, PA, and PET (polyethylene terephthalate) fibres exhibited melting points of 121.4°C, 144.3°C, 164.6°C, 257.8°C, and 250.5°C, respectively. When these polymer fibres undergo melting, empty channels can be created that allow for the release of trapped vapor pressure. These empty channels may also be connected by microcracks in concrete, facilitating vapor migration. No significant cracking was observed at room temperature, but microcracks in the radial direction at the PP fibre interface were observed at 105°C. The significant dimensional changes of PP fibres at 105°C (41 times greater than cement paste) resulted in radial microcracks (Zhang et al., 2021a), which could be widened with an increase in temperature or applied load. The interconnecting network of microcracks along the radial direction of PP fibres was observed at 150°C. Furthermore, debonding of fibres from the matrix was reported, and microcracks expanded in width and length at 170°C after melting PP fibres. Most PP fibres disappeared with further temperature increases to 200°C and 300°C, and the microcracks continued to widen. This study emphasizes the importance of an interconnected microcracks network both before and after the melting point of fibres in enabling vapor migration via empty channels created by the melting of polymer fibres. Moreover, Bian et al. (2016) found that melting PP/PVA fibres was the primary reason for improved spalling resistance, and no significant contribution of steel fibres was observed. Thus, the findings suggest that the channels formed by the melting of polymer fibres can facilitate vapor migration, and an interconnected microcracks network is crucial for this process, highlighting the potential of hybridization with steel fibre to control cracking under elevated temperatures.
6 Residual compressive strength of FRC at elevated temperature
Past research has extensively explored the compressive strength of FRC (fibre reinforced concrete) under room temperature conditions. Overall, the impact of fibres is considered to be minimal. When steel and basalt fibres are introduced, FRC’s compressive strength is slightly improved, while including PP fibres may reduce FRC’s compressive strength (Poon et al., 2004a). The investigations that have already been done on FRC at higher temperatures have mostly focused on FRC with reinforcement composed of PP and steel fibres. The effect of high temperatures on the compressive strength of steel fibre reinforced concrete has been documented in several previous studies (Chan et al., 2000; Zheng et al., 2012a; Zheng et al., 2012b; Chen et al., 2014a; Guo et al., 2014). The residual compressive strength of steel fibre reinforced concrete is notably influenced by the temperature to which it is exposed. In general, it is reported that there is a significant linear decline in the relative compressive strength of steel fibre reinforced concrete as the temperature increases. Moreover, the extent of strength reduction can be influenced by the type of replacement material used (Chan et al., 2000; Poon et al., 2004a; Zheng et al., 2015). Additionally, it has been found that all steel fibre reinforced concrete samples exhibit comparable relative compressive strength drop rates at relatively low temperatures (between room temperature and 200°C). However, as the temperature surpasses this range, steel fibre reinforced concrete containing 20% silica fume and 10% slag (Zheng et al., 2015) exhibits a notably more rapid decline in relative compressive strength than other mixtures. Steel fibre reinforced concrete without any replacement material demonstrates the highest relative compressive strength at elevated temperatures. The compressive strength of steel fibre reinforced concrete is influenced by the volume fraction of steel fibres, particularly at room temperature. In an experimental study conducted by Zheng et al. (2012b) and Zheng et al. (2015), it was observed that steel fibre reinforced concrete with 1% steel fibres exhibited the lowest compressive strength, while steel fibre reinforced concrete with 3% steel fibres demonstrated the highest strength among the three volume fractions tested (1%, 2%, and 3%). Additionally, when steel fibre reinforced concrete is exposed to elevated temperatures, the fibres’ dosage may also impact its compressive strength. Other studies (Tai et al., 2011a; Zheng et al., 2012a; Guo et al., 2014; Xie et al., 2018) have similarly indicated enhanced compressive strength for steel fibre reinforced concrete with 1%–2% steel fibres. However, the dosage of steel fibres does not seem to have a significant effect when steel fibre reinforced concrete comprises 20% SF and 10% slag (Zheng et al., 2012b). Notably, a fibre dose of 2% demonstrates the best residual compressive strength at 600°C. Figure 20 presents the findings from the literature on the relative compressive strength of steel fibre reinforced concrete following exposure to high temperatures (Lau and Anson, 2006; Zhao et al., 2006; Chen et al., 2010; Tai et al., 2011b; Zheng et al., 2012a; Chen et al., 2014b; Ismail et al., 2014; Düğenci et al., 2015; Shaikh and Hosan, 2016; Xie et al., 2018; Li et al., 2019a).
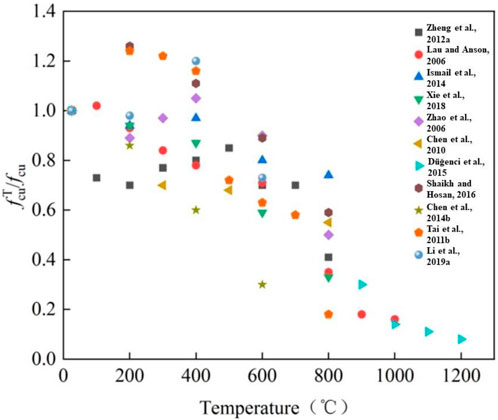
FIGURE 20. Reported relative compressive strength of FRC at higher temperatures (Zhang et al., 2020c).
In previous research, PP fibre has been a significant area of interest as a concrete reinforcement material. According to (Aslani and Kelin, 2018), a modest amount of PP fibres added to FRC at room temperature increases the material’s compressive strength. Additionally, PP fibres prevent FRC from spalling damage when subjected to high temperatures, improving residual compressive strength (Canbaz, 2014; Sanchayan and Foster, 2016; Eidan et al., 2019). The residual compressive strength of PP fibre reinforced concrete with 25% FA and 10% SF (Zheng et al., 2012a) gradually increases to 400°C. The remaining compressive strength, however, gradually decreases above this temperature and eventually approaches a level comparable to the other mixes. Similar behavior is shown by PP FRC with 30% FA, 22.5% slag, and 7.5% SF (Aslani and Kelin, 2018), with the highest residual compressive strength being attained at 300°C. The increased C-S-H production during the initial phases of heating at high temperatures during this event could be related to the rapid hydration. Drzymała et al. (2017)’s experimental testing on PP FRC with 0.17% PP fibres and aggregated basalt chippings revealed a similar trend. Their investigation found that the compressive strength increased initially as the temperature rose, reaching 350°C. However, there was a minor decline in temperature between 350°C and 450°C, followed by a sharp drop when the temperature hit 450°C. There is a wide range in how PP fibre affects the remaining compressive strength of FRC. A fibre dosage of 0.05% shows the optimum performance in the case of PP FRC with 22% FA (Al Qadi and Al-Zaidyeen, 2014), and this is also true for PP FRC with 30% FA, 22.5% slag, and 7.5% SF (Aslani and Kelin, 2018). However, additional test findings indicate that the effect of PP fibre dose is negligible (Zheng et al., 2012a; Eidan et al., 2019). Interestingly, 0.2% PP fibre offers greater relative compressive strength for PFRC with 25% FA and 10% SF at 800°C (Zheng et al., 2012a), but a declining trend is seen for PFRC with 10% silica fume (Poon et al., 2004a). These variances could be explained by differences in the tests’ curing and heating procedures. Figure 21 shows PP FRC’s compressive strengths, as Li et al. (2023) reported, following exposure to high temperatures.
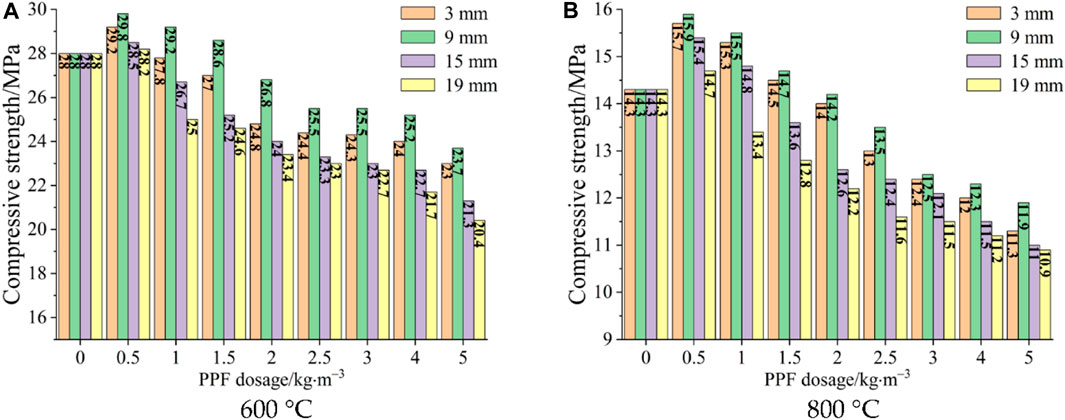
FIGURE 21. Compressive strengths of PP FRCs at higher temperatures (A) 600°C (B) 800°C (Li et al., 2023).
Different types of reinforcing fibres can play specific functions in improving fire resistance in FRC. For example, steel fibres limit the development and spread of microcracks, whereas PP fibres open channels to release water vapor. Researchers are interested in hybrid fibre reinforced concrete to take advantage of various fibres. Several researchers have reported on the combined effect of steel fibre and PP fibre on FRC performance (Zheng et al., 2012a; Canbaz, 2014; Yermak et al., 2017). According to Poon et al. (2004a), HFRC’s compressive strength at room temperature and 800°C is marginally higher than PP FRC’s but lower than SFRC’s. HFRC contains 1% steel fibres and 0.222% PP fibres. HFRC reinforced with steel and PP fibres demonstrated greater residual compressive strength than regular concrete in an experimental investigation by Yermak et al. (2017) in the temperature range of 20°C–300°C. Even at 300°C, the residual compressive strength of FRC containing 0.77% steel fibres and 0.08% PP fibres increased by 16%. The residual compressive strength of all FRC specimens made with hybrid steel and PP fibres increased from 20°C to 200°C compared to the strength at room temperature, according to research by Zheng et al. (2012a). They continued to have an equivalent compressive strength to that seen in ambient circumstances, even at 300°C. When reinforced with blended fibres, the types, shapes, doses, and concrete mix designs can all impact the fire resistance of FRC. Most recent experimental studies have concentrated on examining the interactions between PP and steel fibres, with relatively little attention paid to alternative forms of HFRC.
7 Microstructural (SEM) analysis of FRC at elevated temperature
The available research on the fire resistance of FRC has primarily considered the combined and individual inclusion of steel and polymer fibres. Polymer fibres, like low-density polyethylene, polypropylene, polyvinyl alcohol, and polyamide, are highly effective against spalling (Liu and Tan, 2018b; Zhang and Tan, 2020). Such fibres help to enhance permeability at higher temperatures and reduce vapor pressure. Numerous studies have confirmed that elevated temperatures can significantly impair concrete’s chemical composition and microstructure. However, incorporating steel fibres offers a potential solution to enhance the concrete’s microstructure to a certain extent. Huang et al. (2018), using Scanning Electron Microscopy (SEM) observations, noted alterations in the diameter of steel fibres and observed an increase in the oxygen content within the steel fibres as the temperature rose. This phenomenon could be attributed to small pores surrounding the steel fibre, hindering water evaporation. Consequently, a considerable amount of water vapor accumulates within the matrix, leading to the rusting of steel and the deposition of oxidation products on the fibre’s surface. Kalifa et al. (2001) demonstrated that at 400°C, ordinary concrete exhibits a significantly lower microcrack density than FRC. Microcracks in FRC typically have a thickness of 1 mm and occur between and around the skeleton of the sand and aggregate. On the other hand, conventional concrete often has microcracks that are around 10 mm thick and typically form between large aggregates. According to a study by Lau and Anson (2006), the porosity of concrete increased as the maximum heating temperature rose. However, compared to concrete without fibres, steel FRC had reduced porosity and a smaller average pore diameter. The microstructure of the matrix-fibre interface in steel FRC subjected to high temperatures was studied by Ahmad et al. (2019). The study’s findings showed that the binding strength between the matrix and the fibres gradually deteriorated as exposure duration increased. After prolonged exposure to high temperatures, the matrix-fibre bond eventually underwent progressive rupture, which caused a slow decline in the activation effectiveness of the steel fibres. This phenomenon may be due to the constriction brought on by the capillary water discharge and the prolonged oxidation of certain hydrates at high temperatures. The influence of steel fibres on the high-temperature performance of concrete may be lessened as a result. Still, it is crucial to remember that this does not completely eliminate the effect. Figure 22 shows the SEM analysis of FRC conducted by Hager and Mróz (2019b). Two conjectures have been proposed to explain the occurrence of additional cracking in FRC. Firstly, during the heating process, the fibres experience a dilation of approximately 7%–10% (Pasquini and Addeo, 2005), generating local stresses and initiating cracks. Secondly, the arrangement of fibres within the concrete contributes to localized crack formation, as depicted in Figure 22. The growth of fractures near the fibres helps to lower the pressure of water vapour in the pores of the concrete. According to a numerical analysis by Bentz (2000), this phenomenon is thought to be caused by the interaction between the melted fibre network with the material’s inherent porosity.
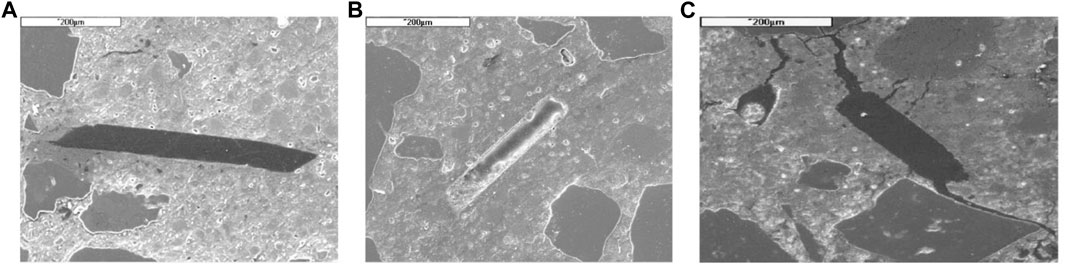
FIGURE 22. SEM analysis of FRC specimens: (A) untreated, (B) heated to 150°C, and (C) post-exposure to 600°C (Hager and Mróz, 2019b).
The effect of steel fibre hybridization with polyvinyl alcohol and independent polyvinyl alcohol fibre in concrete exposure to elevated temperature is explored at micro-structural level by Hossain et al. (2021). The fibre-matrix interaction was investigated by conducting SEM analysis of samples from its core. There was a significant difference in the porosity of polyvinyl alcohol and steel fibre due to its repeated exposure to 175°C temperature (Figure 23). Incorporating natural fibres as an alternative to artificial/synthetic fibres has also been studied after exposure to high-temperature applications. For example, Zhang et al. (2020a) reported a 64% weight loss between 250°C and 400°C of jute fibre and henceforth also owns a considerable possibility of enhancing the permeability for vapor pressure releasing. Similarly, the micro-analysis of jute fibre reinforced concrete at ambient and elevated temperatures (i.e., 200°C) is conducted. The shrinkage of jute fibre is enhanced with increasing temperature. Furthermore, the application of ultra-high molecular weight polyethylene fibres is also enhancing to attain both high ductility and high strength at the same time and at the normal temperature (Yu et al., 2018). But, the sole incorporation of these fibres is inadequate to resist spalling (Liu and Tan, 2018a). It may also be noted that the melting point of all polymer fibres mentioned above is very low (i.e., 120°C–250°C), which can badly affect the FRC mechanical performance at elevated temperatures. In past research, it has been reported that using a combination of high melting point steel fibres and polymer fibres can be proved as an effective approach for overcoming the said lacking and may also enhance ductility and strength after being exposed to elevated temperature (Liu et al., 2017; Deshpande et al., 2019). In addition, steel fibres also help resist crack propagation at higher temperatures (Tai et al., 2011a). A comparatively novel category of fire-resistant fibres is polybenzimidazole fibres, which suffer weight loss of only 35% up to 600°C (Fares et al., 2015). However, research on these fibres or other fire-resistive fibres such as basalt and glass is still limited to validate their performance merits.
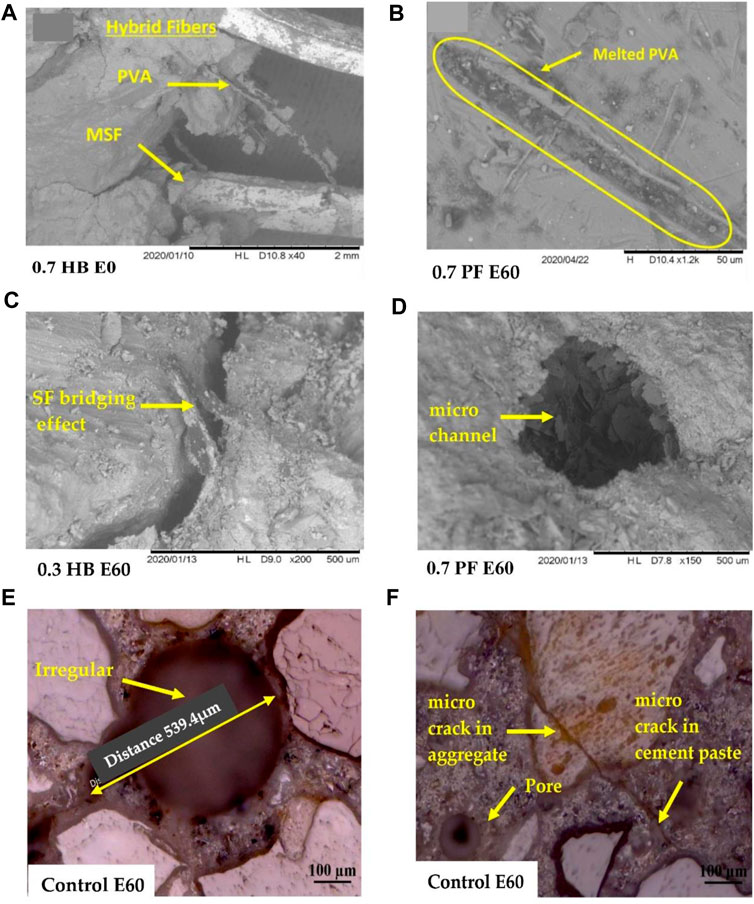
FIGURE 23. SEM analysis of fibre-matrix bonding (A) steel and polyvinyl alcohol before melting, (B) steel and polyvinyl alcohol after melting, (C) sewing effect due to steel fibres, (D) development of microchannel due to melting of polyvinyl alcohol fibre, (E) irregular voids, and (F) developed micro-cracks (Hossain et al., 2021).
8 Future research prospects
Furthermore, the available review-based conventional research studies are not able to connect different literature aspects in a precise manner. Subsequently, the systematic review conducted in the current study provides the mapping among bibliographic data and its statistical analysis on the fire resistance application of FRC research. In this manner, the identification of frequently used keywords, the countries that are contributing the most relevant articles, the sources that are producing the most relevant articles, and the most credible authors in terms of articles and citations in the fire resistance application of FRC research domain are made in the current study. The keywords assessment shows that the fire resistance application of FRC has been explored to have fire resistance materials. Similarly, the highly contributing countries in the research domain are characterized by literature with citation-based linkages. This analysis would aid the researchers in collaborating to advance research in this field. With the help of scientometric analysis to evaluate keywords and inter-linked literature, the current study further emphasizes the future perspectives in research of fire resistance application of FRC. Accordingly, the following prospects are proposed for this research area.
• Studies on the determination of FRC thermal properties at higher temperatures are limited. Specific heat is enhanced upon increasing temperature, whereas heat conductivity usually reduces. But, the variation pattern is considerably influenced by mix ingredients and fibre’s capability to conduct heat; hence, there are different observations in the case of various studies that need to be explored regarding behavior prediction.
• It is well-known that the impact of supplementary cementitious materials depends on the content and type. Hence, it is vital to devise vibrant standards/guidelines regarding the quantity with minimum/maximum limitations of various supplementary material types to achieve enhanced properties at higher temperatures and FRC strength and durability at normal temperatures.
• The available research primarily considers the polymer fibres volume to assess performance improvement at the higher temperature. However, FRC mechanical characteristics are observed to rely on both aspect ratio volume and aspect ratio. Hence, further study is required to optimize the volume for various aspect ratios and lengths of fibre in FRC.
• The literature on polyvinyl alcohol fibre reinforced concrete is still very limited in developing a clear interpretation of their general performance. So, their performance at higher temperatures requires further examination of the cementitious matrix interdependency with fibre and the independent behavior.
• Existing research on the spalling performance of concrete under high temperatures mainly focuses on the volume of natural fibres used. However, the mechanical properties of fibres, such as aspect ratio, volume, and coefficient of thermal expansion, also play a crucial role. Therefore, further studies are required to standardize the appropriate natural fibre type, volume, fibre length, aspect ratio, and coefficient of thermal expansion in concrete.
• Extensive experimental investigations have been conducted on the fire resistance of hybrid fibre reinforced concrete incorporating steel and polypropylene fibres. However, limited research exists on the fire resistance of HFRC reinforced with alternative fibre types. Exploring the synergistic effects of different fibre combinations on enhancing the fire resistance of FRC would be advantageous.
• The available findings on the impact of the cooling method type and size of the specimen are also not dependable, and further investigation is required to indicate their exact effects.
• Furthermore, future work should establish standard testing procedures to reduce the variables’ impact on the output.
• Eurocode 2 (Code, 2005) only stipulates the propylene fibres minimum volume for decreasing spalling risk in high-strength concrete. However, resistance against spalling relies on fibre volume and aspect ratio as well as on the ingredients of the matrix. Consequently, the current requirements stated in Eurocode 2 (Code, 2005) may not be acceptable. A detailed standard is obligatory for optimum volume for various fibre types for an appropriate mix design and a particular aspect ratio.
• Moreover, numerical and experimental research is also needed to validate the analogy of the collective effect of different fibre types and micro-defects in improving the spalling resistance.
• Likewise, the sole addition of ultra-high molecular weight polyethylene fibres, although they provide higher ductility and strength simultaneously, their sole incorporation for better resistance against spalling, remains an investigative area for future research.
• The available research on thermally stable fibres like polybenzimidazole, basalt glass, etc., is also quite limited in validating the respective FRC performance, indicating a gap for future research.
• An in-depth study is required to improve the spalling behavior of UHPC with hybrid steel and natural fibres, promoting its use as a sustainable material for elevated temperatures. Future studies should focus on the MIP analysis of natural fibres in UHPC to understand their swelling/shrink effect, which can influence pore size/diameter at high temperatures.
9 Conclusion
Fibre reinforced concrete (FRC) has significant advantages, but its fire resistance remains a critical concern. The scientific community has leveraged abundant information and innovative communication networks to propose a reliable bibliometric metric based on statistical analysis. This metric allows evaluating FRC-related publications from research groups, institutions, individuals, and countries to draw scientifically sound conclusions. A review-based study is ideal for identifying emerging trends in the literature on FRC’s fire resistance application, providing valuable insights for young scholars and researchers. The main aim is to conduct an in-depth analysis of the existing literature, delving into various aspects of FRC behavior at elevated temperatures. This includes discussing pore pressure behaviors, spalling behavior and mechanisms, residual mechanical properties, and microstructural analysis under high temperatures, as it pertains to FRC’s application in fire resistance. The drawn conclusions are as follows.
• The widespread use of concrete in various structures has increased fire vulnerability, leading to physical and chemical alterations, reduced strength, explosive spalling, and safety issues; thermal conductivity and specific heat are crucial thermo-physical properties affected by factors like aggregate type, moisture content, density, porosity, SCMs, environmental temperature, and measurement methods. Fire-induced spalling, which can result from increased pore pressure, heat-induced stress, or a combination of these variables, is a serious problem for concrete. High tensile strength fibres, like steel fibres, can prevent the development and spread of microcracks by serving as bridges during heating and cooling cycles. Conversely, fibres with lower melting points, like polypropylene fibres, might open up channels for water vapour to escape when temperatures are high. This enhances concrete’s resistance to spalling by reducing the harm done by temperature gradients and internal pore pressure.
• When subjected to high temperatures, concrete undergoes physical and chemical reactions that result in strength degradation and the risk of explosive spalling. Introducing fiber reinforcement, particularly in HFRC with steel and synthetic fibers, holds potential for enhancing concrete’s resistance to elevated temperatures and improving its mechanical properties. The thermal conductivity of FRC is a crucial aspect affecting heat conduction, which can be influenced by factors such as aggregate type, moisture content, and density. Gaining insights into these factors can aid in the development of more fire-resistant and thermally efficient FRC structures.
• Fiber reinforcement in concrete improves its resilience against high temperatures, reducing spalling caused by thermally induced stress and pore pressure accumulation. FRC, especially HFRC with steel and synthetic fibers, exhibits enhanced mechanical properties, crack management, and fire resistance. Steel fibers play a crucial role in mitigating thermal stress due to their thermal stability and crack-resistant characteristics. However, challenges in international regulations and standards hinder the widespread adoption of fiber-reinforced concrete for high-temperature applications.
• Spalling in concrete under high-temperature conditions results from the interplay of thermal, hydraulic, and mechanical degradation processes. Vaporization of water within the concrete leads to the accumulation of pore pressure, while thermal gradients cause thermal stress-induced cracking. The explosive spalling of concrete can be mitigated by using steel fibers, which lower pore pressure, improve thermal conductivity, and enhance the concrete’s tensile properties. The incorporation of fibers into the concrete mix offers potential benefits in reducing the risk of explosive spalling by improving its behavior under elevated temperatures.
• The working mechanisms of different fibers in cementitious composites may vary. Two mechanisms have been reported in the literature, i.e., vapor migration via pressure-induced tangential space (PITS) and vapor migration through channels formed by the melting of fibers. The PITS mechanism, independent of the fiber type used, provides a viable explanation for fiber behavior in concrete, enhancing spalling performance. On the other hand, the melting of polymer fibers can create empty channels for vapor pressure release and facilitate vapor migration through an interconnected network of microcracks. Hybridization with steel fibers can control cracking under elevated temperatures, further enhancing spalling resistance. Understanding these mechanisms offers valuable insights for improving concrete’s behavior during fire exposure.
• The addition of fibers to concrete significantly enhances its residual compressive strength, and incorporating steel fibers along with a higher content of natural fibers improves spalling resistance. The study emphasizes that the compressive strength of FRC is affected by the type and volume fraction of fibers and the exposure temperature. Steel fibers generally demonstrate a pronounced linear decline in relative compressive strength as the temperature increases, while the influence of polypropylene (PP) fibers depends on the fiber dosage and replacement materials. Hybrid fiber-reinforced concrete shows promise in enhancing fire resistance. Significant variations in the mechanical properties of FRC have been observed in reported studies, mainly due to the utilization of diverse heating and cooling techniques. Therefore, it is crucial to establish a standardized testing method to ensure consistency and facilitate future research in this field.
• The SEM analyses indicate that polymer fibers effectively mitigate spalling and enhance permeability at elevated temperatures, whereas steel fibers improve FRC’s microstructure and reduce porosity, enhancing its high-temperature performance. Nevertheless, prolonged exposure to high temperatures may lead to a weakening of the matrix-fiber bond in steel FRC, presenting potential drawbacks. Additional cracking during heating is attributed to fiber dilation and arrangement. According to SEM analysis, steel fibers restrict crack propagation and enhance connectivity between voids and interfacial gaps in concrete. Conversely, polymer and natural fibers produce radial microcracks that facilitate vapor migration. The hybridization of steel fibers with polyvinyl alcohol and natural fibers results in diverse effects on FRC properties at elevated temperatures.
Author contributions
FG: Conceptualization, Funding acquisition, Methodology, Visualization, Writing–original draft. XJ: Conceptualization, Funding acquisition, Investigation, Project administration, Validation, Writing–review and editing. YG: Investigation, Resources, Software, Supervision, Validation, Writing–review and editing. BI: Writing–original draft. BT: Data curation, Methodology, Visualization, Writing–review and editing.
Funding
The author(s) declare that no financial support was received for the research, authorship, and/or publication of this article.
Conflict of interest
The authors declare that the research was conducted in the absence of any commercial or financial relationships that could be construed as a potential conflict of interest.
Publisher’s note
All claims expressed in this article are solely those of the authors and do not necessarily represent those of their affiliated organizations, or those of the publisher, the editors and the reviewers. Any product that may be evaluated in this article, or claim that may be made by its manufacturer, is not guaranteed or endorsed by the publisher.
References
Abdallah, S., Fan, M., Zhou, X., and Le Geyt, S. (2016). Anchorage effects of various steel fibre architectures for concrete reinforcement. Int. J. Concr. Struct. Mater. 10, 325–335. doi:10.1007/s40069-016-0148-5
Abid, M., Hou, X., Zheng, W., and Hussain, R. R. (2017). High temperature and residual properties of reactive powder concrete–A review. Constr. Build. Mater. 147, 339–351. doi:10.1016/j.conbuildmat.2017.04.083
Adamu, M., Alanazi, F., Ibrahim, Y. E., Alanazi, H., and Khed, V. C. (2022). A comprehensive review on sustainable natural fiber in cementitious composites: the date palm fiber case. Sustainability 14 (11), 6691. doi:10.3390/su14116691
Afgan, S., and Bing, C. (2021). Scientometrics review of international research trends on thermal energy storage cement based composites via integration of phase change materials from 1993 to 2020. Constr. Build. Mater. 278, 122344. doi:10.1016/j.conbuildmat.2021.122344
Afroughsabet, V., Biolzi, L., and Ozbakkaloglu, T. (2016). High-performance fiber-reinforced concrete: A review. J. Mater. Sci. 51 (14), 6517–6551. doi:10.1007/s10853-016-9917-4
Ahmad, H., Chhipi-Shrestha, G., Hewage, K., and Sadiq, R. (2022). A comprehensive review on construction applications and life cycle sustainability of natural fiber biocomposites. Sustainability 14 (23), 15905. doi:10.3390/su142315905
Ahmad, M. R., Das, C. S., Khan, M., and Dai, J.-G. (2023). Development of low-carbon alkali-activated materials solely activated by flue gas residues (FGR) waste from incineration plants. J. Clean. Prod. 397, 136597. doi:10.1016/j.jclepro.2023.136597
Ahmad, S., Rasul, M., Adekunle, S. K., Al-Dulaijan, S. U., Maslehuddin, M., and Ali, S. I. (2019). Mechanical properties of steel fiber-reinforced UHPC mixtures exposed to elevated temperature: effects of exposure duration and fiber content. Compos. Part B Eng. 168, 291–301. doi:10.1016/j.compositesb.2018.12.083
Ahmad, W., Ahmad, A., Ostrowski, K. A., Aslam, F., and Joyklad, P. (2021a). A scientometrics review of waste material utilization in concrete for sustainable construction. Case Stud. Constr. Mater. 15, e00683. doi:10.1016/j.cscm.2021.e00683
Ahmad, W., Khan, M., and Smarzewski, P. (2021b). Effect of short fiber reinforcements on fracture performance of cement-based materials: A systematic review approach. Materials 14 (7), 1745. doi:10.3390/ma14071745
Al Qadi, A. N., and Al-Zaidyeen, S. M. (2014). Effect of fibre content and specimen shape on residual strength of polypropylene fibre self-compacting concrete exposed to elevated temperatures. J. King Saud University-Engineering Sci. 26 (1), 33–39. doi:10.1016/j.jksues.2012.12.002
Ali, M. H., Dinkha, Y. Z., and Haido, J. H. (2017). Mechanical properties and spalling at elevated temperature of high performance concrete made with reactive and waste inert powders. Eng. Sci. Technol. Int. J. 20 (2), 536–541. doi:10.1016/j.jestch.2016.12.004
Amin, M. N., Ahmad, W., Khan, K., and Sayed, M. M. (2022). Mapping research knowledge on rice husk ash application in concrete: A scientometrics review. Materials 15 (10), 3431. doi:10.3390/ma15103431
Amin, M., Tayeh, B. A., and agwa, I. s. (2020). Investigating the mechanical and microstructure properties of fibre-reinforced lightweight concrete under elevated temperatures. Case Stud. Constr. Mater. 13, e00459. doi:10.1016/j.cscm.2020.e00459
Antwi-Afari, P., Ng, S. T., and Hossain, M. U. (2021). A review of the circularity gap in the construction industry through scientometrics analysis. J. Clean. Prod. 298, 126870. doi:10.1016/j.jclepro.2021.126870
Arioz, O. (2007). Effects of elevated temperatures on properties of concrete. Fire Saf. J. 42 (8), 516–522. doi:10.1016/j.firesaf.2007.01.003
Arisoy, B., and Wu, H.-C. (2008). Material characteristics of high performance lightweight concrete reinforced with PVA. Constr. Build. Mater. 22 (4), 635–645. doi:10.1016/j.conbuildmat.2006.10.010
Asadi, I., Shafigh, P., Abu Hassan, Z. F. B., and Mahyuddin, N. B. (2018). Thermal conductivity of concrete – a review. J. Build. Eng. 20, 81–93. doi:10.1016/j.jobe.2018.07.002
Aslani, F., and Kelin, J. (2018). Assessment and development of high-performance fibre-reinforced lightweight self-compacting concrete including recycled crumb rubber aggregates exposed to elevated temperatures. J. Clean. Prod. 200, 1009–1025. doi:10.1016/j.jclepro.2018.07.323
Aydın, S., Yazıcı, H., and Baradan, B. (2008). High temperature resistance of normal strength and autoclaved high strength mortars incorporated polypropylene and steel fibers. Constr. Build. Mater. 22 (4), 504–512. doi:10.1016/j.conbuildmat.2006.11.003
Bangi, M. R., and Horiguchi, T. (2012). Effect of fibre type and geometry on maximum pore pressures in fibre-reinforced high strength concrete at elevated temperatures. Cem. Concr. Res. 42 (2), 459–466. doi:10.1016/j.cemconres.2011.11.014
Bangi, M. R., and Horiguchi, T. (2011). Pore pressure development in hybrid fibre-reinforced high strength concrete at elevated temperatures. Cem. Concr. Res. 41 (11), 1150–1156. doi:10.1016/j.cemconres.2011.07.001
Behnood, A., and Ziari, H. (2008). Effects of silica fume addition and water to cement ratio on the properties of high-strength concrete after exposure to high temperatures. Cem. Concr. Compos. 30 (2), 106–112. doi:10.1016/j.cemconcomp.2007.06.003
Bentz, D. P. (2000). Fibers, percolation, and spalling of high-performance concrete. Mater. J. 97 (3), 351–359. doi:10.14359/9878
Bentz, D. P., Peltz, M. A., Duran-Herrera, A., Valdez, P., and Juarez, C. (2011). Thermal properties of high-volume fly ash mortars and concretes. J. Build. Phys. 34 (3), 263–275. doi:10.1177/1744259110376613
Bhat, T., Chevali, V., Liu, X., Feih, S., and Mouritz, A. (2015). Fire structural resistance of basalt fibre composite. Compos. Part A Appl. Sci. Manuf. 71, 107–115. doi:10.1016/j.compositesa.2015.01.006
Bhattacharjee, B., and Krishnamoorthy, S. (2004). Permeable porosity and thermal conductivity of construction materials. J. Mater. Civ. Eng. 16 (4), 322–330. doi:10.1061/(asce)0899-1561(2004)16:4(322)
Bian, H., Hannawi, K., Takarli, M., Molez, L., and Prince, W. (2016). Effects of thermal damage on physical properties and cracking behavior of ultrahigh-performance fiber-reinforced concrete. J. Mater. Sci. 51 (22), 10066–10076. doi:10.1007/s10853-016-0233-9
Bindiganavile, V., Batool, F., and Suresh, N. (2012). Effect of fly ash on thermal properties of cement based foams evaluated by transient plane heat source. Indian Concr. J. 86 (11), 7.
Bošnjak, J., Ožbolt, J., and Hahn, R. (2013). Permeability measurement on high strength concrete without and with polypropylene fibers at elevated temperatures using a new test setup. Cem. Concr. Res. 53, 104–111. doi:10.1016/j.cemconres.2013.06.005
Boumaaza, M., Belaadi, A., and Bourchak, M. (2022). Systematic review on reinforcing mortars with natural fibers: challenges of environment-friendly option. J. Nat. Fibers 19 (16), 14262–14286. doi:10.1080/15440478.2022.2060408
Branston, J., Das, S., Kenno, S. Y., and Taylor, C. (2016). Mechanical behaviour of basalt fibre reinforced concrete. Constr. Build. Mater. 124, 878–886. doi:10.1016/j.conbuildmat.2016.08.009
Caetano, H., Ferreira, G., Rodrigues, J. P. C., and Pimienta, P. (2019). Effect of the high temperatures on the microstructure and compressive strength of high strength fibre concretes. Constr. Build. Mater. 199, 717–736. doi:10.1016/j.conbuildmat.2018.12.074
Cai, R., Liu, J.-C., and Ye, H. (2021). Spalling prevention of ultrahigh-performance concrete: comparative effectiveness of polyethylene terephthalate and polypropylene fibers. J. Mater. Civ. Eng. 33 (12), 04021344. doi:10.1061/(asce)mt.1943-5533.0003980
Campione, G., La Mendola, L., Monaco, A., Valenza, A., and Fiore, V. (2015). Behavior in compression of concrete cylinders externally wrapped with basalt fibers. Compos. Part B Eng. 69, 576–586. doi:10.1016/j.compositesb.2014.10.008
Canbaz, M. (2014). The effect of high temperature on reactive powder concrete. Constr. Build. Mater. 70, 508–513. doi:10.1016/j.conbuildmat.2014.07.097
Cao, M., Ming, X., Yin, H., and Li, L. (2019). Influence of high temperature on strength, ultrasonic velocity and mass loss of calcium carbonate whisker reinforced cement paste. Compos. Part B Eng. 163, 438–446. doi:10.1016/j.compositesb.2019.01.030
Çavdar, A. (2013). The effects of high temperature on mechanical properties of cementitious composites reinforced with polymeric fibers. Compos. Part B Eng. 45 (1), 78–88. doi:10.1016/j.compositesb.2012.09.033
Chan, Y., Luo, X., and Sun, W. (2000). Compressive strength and pore structure of high-performance concrete after exposure to high temperature up to 800 C. Cem. Concr. Res. 30 (2), 247–251. doi:10.1016/s0008-8846(99)00240-9
Chen, G., He, Y., Yang, H., Chen, J., and Guo, Y. (2014a). Compressive behavior of steel fiber reinforced recycled aggregate concrete after exposure to elevated temperatures. Constr. Build. Mater. 71, 1–15. doi:10.1016/j.conbuildmat.2014.08.012
Chen, G. M., He, Y. H., Yang, H., Chen, J. F., and Guo, Y. C. (2014b). Compressive behavior of steel fiber reinforced recycled aggregate concrete after exposure to elevated temperatures. Constr. Build. Mater. 71, 1–15. doi:10.1016/j.conbuildmat.2014.08.012
Chen, G., Yang, H., Lin, C., Chen, J., He, Y., and Zhang, H. (2016). Fracture behaviour of steel fibre reinforced recycled aggregate concrete after exposure to elevated temperatures. Constr. Build. Mater. 128, 272–286. doi:10.1016/j.conbuildmat.2016.10.072
Chen, H.-G., Liu, Y.-F., Sun, B., Wang, M., and Cheng, P.-J. (2010). Impact of steel fibre dosage on mechanical properties of concrete under high temperature. Chongqing Jiot. Daxue Xuebao(Ziran Kexue Ban. 29 (4).
Chidighikaobi, P. C. (2019). Thermal effect on the flexural strength of expanded clay lightweight basalt fiber reinforced concrete. Mater. Today Proc. 19, 2467–2470. doi:10.1016/j.matpr.2019.08.110
Choe, G., Kim, G., Yoon, M., Hwang, E., Nam, J., and Guncunski, N. (2019). Effect of moisture migration and water vapor pressure build-up with the heating rate on concrete spalling type. Cem. Concr. Res. 116, 1–10. doi:10.1016/j.cemconres.2018.10.021
Choumanidis, D., Badogiannis, E., Nomikos, P., and Sofianos, A. (2016). The effect of different fibres on the flexural behaviour of concrete exposed to normal and elevated temperatures. Constr. Build. Mater. 129, 266–277. doi:10.1016/j.conbuildmat.2016.10.089
Chung, S.-Y., Han, T.-S., Kim, S.-Y., Kim, J.-H. J., Youm, K. S., and Lim, J.-H. (2016). Evaluation of effect of glass beads on thermal conductivity of insulating concrete using micro CT images and probability functions. Cem. Concr. Compos. 65, 150–162. doi:10.1016/j.cemconcomp.2015.10.011
Code, P. (2005). Eurocode 2: design of concrete structures-part 1–1: general rules and rules for buildings. London: British Standard Institution.
Colombo, M., Di Prisco, M., and Felicetti, R. (2010). Mechanical properties of steel fibre reinforced concrete exposed at high temperatures. Mater. Struct. 43 (4), 475–491. doi:10.1617/s11527-009-9504-0
Consolazio, G. R., McVay, M. C., and Rish, J. W. (1998). Measurement and prediction of pore pressures in saturated cement mortar subjected to radiant heating. Mater. J. 95 (5), 525–536.
Cree, D., Green, M., and Noumowé, A. (2013). Residual strength of concrete containing recycled materials after exposure to fire: A review. Constr. Build. Mater. 45, 208–223. doi:10.1016/j.conbuildmat.2013.04.005
Darko, A., Chan, A. P., Adabre, M. A., Edwards, D. J., Hosseini, M. R., and Ameyaw, E. E. (2020). Artificial intelligence in the AEC industry: scientometrics analysis and visualization of research activities. Automation Constr. 112, 103081. doi:10.1016/j.autcon.2020.103081
Darko, A., Zhang, C., and Chan, A. P. (2017). Drivers for green building: A review of empirical studies. Habitat Int. 60, 34–49. doi:10.1016/j.habitatint.2016.12.007
Debicki, G., Haniche, R., and Delhomme, F. (2012). An experimental method for assessing the spalling sensitivity of concrete mixture submitted to high temperature. Cem. Concr. Compos. 34 (8), 958–963. doi:10.1016/j.cemconcomp.2012.04.002
Deshpande, A. A., Kumar, D., and Ranade, R. (2019). Influence of high temperatures on the residual mechanical properties of a hybrid fiber-reinforced strain-hardening cementitious composite. Constr. Build. Mater. 208, 283–295. doi:10.1016/j.conbuildmat.2019.02.129
Ding, Y., Zhang, F., Torgal, F., and Zhang, Y. (2012). Shear behaviour of steel fibre reinforced self-consolidating concrete beams based on the modified compression field theory. Compos. Struct. 94 (8), 2440–2449. doi:10.1016/j.compstruct.2012.02.025
Drzymała, T., Jackiewicz-Rek, W., Tomaszewski, M., Kuś, A., Gałaj, J., and Šukys, R. (2017). Effects of high temperature on the properties of high performance concrete (HPC). Procedia Eng. 172, 256–263. doi:10.1016/j.proeng.2017.02.108
Du, Y., Qi, H.-H., Huang, S.-S., and Liew, J. R. (2020). Experimental study on the spalling behaviour of ultra-high strength concrete in fire. Constr. Build. Mater. 258, 120334. doi:10.1016/j.conbuildmat.2020.120334
Duan, J., Qiu, P., Liu, J., and Wu, X. (2023). Experimental study of the concrete cracking behavior of an immersed tunnel under fire. Buildings 13 (6), 1412. [Online]. doi:10.3390/buildings13061412
Düğenci, O., Haktanir, T., and Altun, F. (2015). Experimental research for the effect of high temperature on the mechanical properties of steel fiber-reinforced concrete. Constr. Build. Mater. 75, 82–88. doi:10.1016/j.conbuildmat.2014.11.005
Eidan, J., Rasoolan, I., Rezaeian, A., and Poorveis, D. (2019). Residual mechanical properties of polypropylene fiber-reinforced concrete after heating. Constr. Build. Mater. 198, 195–206. doi:10.1016/j.conbuildmat.2018.11.209
Eik, M., Puttonen, J., and Herrmann, H. (2015). An orthotropic material model for steel fibre reinforced concrete based on the orientation distribution of fibres. Compos. Struct. 121, 324–336. doi:10.1016/j.compstruct.2014.11.018
Faiyadh, F., and Al-Ausi, M. (1989a). Effect of elevated temperature on splitting tensile strength of fibre concrete. Int. J. Cem. Compos. Lightweight Concr. 11 (3), 175–178. doi:10.1016/0262-5075(89)90090-0
Faiyadh, F. I., and Al-Ausi, M. A. (1989b). Effect of elevated temperature on splitting tensile strength of fibre concrete. Int. J. Cem. Compos. Lightweight Concr. 11 (3), 175–178. doi:10.1016/0262-5075(89)90090-0
Fang, B., Hu, Z., Shi, T., Liu, Y., Wang, X., Yang, D., et al. (2022). Research progress on the properties and applications of magnesium phosphate cement. Ceram. Int. 49, 4001–4016. doi:10.1016/j.ceramint.2022.11.078
Fares, G., Khan, M. I., Mourad, S., and Abbass, W. (2015). Evaluation of PVA and PBI-based engineered-cementitious composites under different environments. Constr. Build. Mater. 85, 109–118. doi:10.1016/j.conbuildmat.2015.03.080
Farooqi, M. U., and Ali, M. (2022). A study on natural fibre reinforced concrete from materials to structural applications. Arabian J. Sci. Eng. 48, 4471–4491. doi:10.1007/s13369-022-06977-1
Farooqi, M. U., and Ali, M. (2023). A study on natural fibre reinforced concrete from materials to structural applications. Arabian J. Sci. Eng. 48 (4), 4471–4491. doi:10.1007/s13369-022-06977-1
Farooqi, M. U., and Ali, M. (2018a). Contribution of plant fibers in improving the behavior and capacity of reinforced concrete for structural applications. Constr. Build. Mater. 182, 94–107. doi:10.1016/j.conbuildmat.2018.06.041
Farooqi, M. U., and Ali, M. (2018b). “Effect of fibre content on compressive strength of wheat straw reinforced concrete for pavement applications,” in IOP conference series: Materials science and engineering (IOP Publishing).012014.
Farooqi, M. U., and Ali, M. (2018c). “Effect of fibre content on splitting-tensile strength of wheat straw reinforced concrete for pavement applications,” in Key engineering materials (Trans Tech Publ), 349–354.
Farooqi, M. U., and Ali, M. (2019). Effect of pre-treatment and content of wheat straw on energy absorption capability of concrete. Constr. Build. Mater. 224, 572–583. doi:10.1016/j.conbuildmat.2019.07.086
Felicetti, R. (2007). Assessment of the equivalent thermal diffusivity for fire analysis of concrete structures. Proceeding Fib Task Group 4, 49–158.
Fletcher, I. A., Welch, S., Torero, J. L., Carvel, R. O., and Usmani, A. (2007). Behaviour of concrete structures in fire. Therm. Sci. 11 (2), 37–52. doi:10.2298/tsci0702037f
Fu, Y. F., Wong, Y. L., Poon, C. S., and Tang, C. A. (2006). Literature review of study on mechanism of explosive spalling in concrete at elevated temperatures. Jianzhu Cailiao Xuebao/Journal Build. Mater. 9 (3), 323–329.
Gao, D., Yan, D., and Li, X. (2012). Splitting strength of GGBFS concrete incorporating with steel fiber and polypropylene fiber after exposure to elevated temperatures. Fire Saf. J. 54, 67–73. doi:10.1016/j.firesaf.2012.07.009
Gawin, D., Alonso, C., Andrade, C., Majorana, C., and Pesavento, F. (2005). Effect of damage on permeability and hygro-thermal behaviour of HPCs at elevated temperatures: part 1. Experimental results. Comput. Concr. Int. J. 2 (3), 189–202. doi:10.12989/cac.2005.2.3.189
Guo, M., Huang, H., Zhang, W., Xue, C., and Huang, M. (2022). Assessment of RC frame capacity subjected to a loss of corner column. J. Struct. Eng. 148 (9), 04022122. doi:10.1061/(asce)st.1943-541x.0003423
Guo, Y.-c., Zhang, J.-h., Chen, G.-m., and Xie, Z.-h. (2014). Compressive behaviour of concrete structures incorporating recycled concrete aggregates, rubber crumb and reinforced with steel fibre, subjected to elevated temperatures. J. Clean. Prod. 72, 193–203. doi:10.1016/j.jclepro.2014.02.036
Gupta, T., Chaudhary, S., and Sharma, R. K. (2016). Mechanical and durability properties of waste rubber fiber concrete with and without silica fume. J. Clean. Prod. 112, 702–711. doi:10.1016/j.jclepro.2015.07.081
Hager, I. (2013). Behaviour of cement concrete at high temperature. Bulletin of the Polish Academy of Sciences: Technical Sciences.
Hager, I., and Mróz, K. (2019a). Role of polypropylene fibres in concrete spalling risk mitigation in fire and test methods of fibres effectiveness evaluation. Materials 12 (23), 3869. doi:10.3390/ma12233869
Hager, I., and Mróz, K. (2019b). Role of polypropylene fibres in concrete spalling risk mitigation in fire and test methods of fibres effectiveness evaluation. Materials 12 (23), 3869. [Online]. doi:10.3390/ma12233869
Han, C.-G., Hwang, Y.-S., Yang, S.-H., and Gowripalan, N. (2005). Performance of spalling resistance of high performance concrete with polypropylene fiber contents and lateral confinement. Cem. Concr. Res. 35 (9), 1747–1753. doi:10.1016/j.cemconres.2004.11.013
Han, Y., Shao, S., Fang, B., Shi, T., Zhang, B., Wang, X., et al. (2023). Chloride ion penetration resistance of matrix and interfacial transition zone of multi-walled carbon nanotube-reinforced concrete. J. Build. Eng. 72, 106587. doi:10.1016/j.jobe.2023.106587
Handoo, S., Agarwal, S., and Agarwal, S. (2002). Physicochemical, mineralogical, and morphological characteristics of concrete exposed to elevated temperatures. Cem. Concr. Res. 32 (7), 1009–1018. doi:10.1016/s0008-8846(01)00736-0
Henry, M., Darma, I. S., and Sugiyama, T. (2014). Analysis of the effect of heating and re-curing on the microstructure of high-strength concrete using X-ray CT. Constr. Build. Mater. 67, 37–46. doi:10.1016/j.conbuildmat.2013.11.007
Hertz, K. D. (1992). Danish investigations on silica fume concretes at elevated temperatures. Mater. J. 89 (4), 345–347.
Holan, J., Novak, J., Müller, P., and Štefan, R. (2020). Experimental investigation of the compressive strength of normal-strength air-entrained concrete at high temperatures. Constr. Build. Mater. 248, 118662. doi:10.1016/j.conbuildmat.2020.118662
Hossain, K., Lachemi, M., Sammour, M., and Sonebi, M. (2013). Strength and fracture energy characteristics of self-consolidating concrete incorporating polyvinyl alcohol, steel and hybrid fibres. Constr. Build. Mater. 45, 20–29. doi:10.1016/j.conbuildmat.2013.03.054
Hossain, M. M., Al-Deen, S., Hassan, M. K., Shill, S. K., Kader, M. A., and Hutchison, W. (2021). Mechanical and thermal properties of hybrid fibre-reinforced concrete exposed to recurrent high temperature and aviation oil. Materials 14 (11), 2725. doi:10.3390/ma14112725
Huang, H., Huang, M., Zhang, W., Guo, M., Chen, Z., and Li, M. (2021). Progressive collapse resistance of multistory RC frame strengthened with HPFL-BSP. J. Build. Eng. 43, 103123. doi:10.1016/j.jobe.2021.103123
Huang, H., Huang, M., Zhang, W., Guo, M., and Liu, B. (2022a). Progressive collapse of multistory 3D reinforced concrete frame structures after the loss of an edge column. Struct. Infrastructure Eng. 18 (2), 249–265. doi:10.1080/15732479.2020.1841245
Huang, H., Li, M., Yuan, Y., and Bai, H. (2022b). Theoretical analysis on the lateral drift of precast concrete frame with replaceable artificial controllable plastic hinges. J. Build. Eng. 62, 105386. doi:10.1016/j.jobe.2022.105386
Huang, S., Wang, H., Ahmad, W., Ahmad, A., Ivanovich Vatin, N., Mohamed, A. M., et al. (2022c). Plastic waste management strategies and their environmental aspects: A scientometrics analysis and comprehensive review. Int. J. Environ. Res. Public Health 19 (8), 4556. doi:10.3390/ijerph19084556
Huang, Y., Zhang, W., and Liu, X. (2022d). Assessment of diagonal macrocrack-induced debonding mechanisms in FRP-strengthened RC beams. J. Compos. Constr. 26 (5), 04022056. doi:10.1061/(asce)cc.1943-5614.0001255
Huang, Z., Liew, J. R., and Li, W. (2017). Evaluation of compressive behavior of ultra-lightweight cement composite after elevated temperature exposure. Constr. Build. Mater. 148, 579–589. doi:10.1016/j.conbuildmat.2017.04.121
Huang, Z., Padmaja, K., Li, S., and Liew, J. R. (2018). Mechanical properties and microstructure of ultra-lightweight cement composites with fly ash cenospheres after exposure to high temperatures. Constr. Build. Mater. 164, 760–774. doi:10.1016/j.conbuildmat.2018.01.009
Hulin, T., Maluk, C., Bisby, L., Hodicky, K., Schmidt, J. W., and Stang, H. (2016). Experimental studies on the fire behaviour of high performance concrete thin plates. Fire Technol. 52, 683–705. doi:10.1007/s10694-015-0486-x
Ismail, R., Zakwan, F. A. A., Petrus, C., Marzuki, N. A., Hashim, N. H., and Mustafa, M. F. (2014). “Compressive behavior of steel fiber reinforced concrete after exposed to high temperatures,” in InCIEC 2013: Proceedings of the international civil and infrastructure engineering conference 2013 (Springer), 731–740.
Jang, H.-s., So, H.-s., and So, S. (2016). The properties of reactive powder concrete using PP fiber and pozzolanic materials at elevated temperature. J. Build. Eng. 8, 225–230. doi:10.1016/j.jobe.2016.09.010
Janotka, I., and Bagel, L. (2002). Pore structures, permeabilities, and compressive strengths of concrete at temperatures up to 800 C. Mater. J. 99 (2), 196–200. doi:10.14359/11713
Jawahar, J. G., Lavanya, D., and Sashidhar, C. (2016). Performance of fly ash and ggbs based geopolymer concrete in acid environment. Int. J. Res. Sci. Innov. 3 (8), 101–104.
Jiang, C., Fan, K., Wu, F., and Chen, D. (2014). Experimental study on the mechanical properties and microstructure of chopped basalt fibre reinforced concrete. Mater. Des. 58, 187–193. doi:10.1016/j.matdes.2014.01.056
Jin, R., Gao, S., Cheshmehzangi, A., and Aboagye-Nimo, E. (2018). A holistic review of off-site construction literature published between 2008 and 2018. J. Clean. Prod. 202, 1202–1219. doi:10.1016/j.jclepro.2018.08.195
Kalifa, P., Chene, G., and Galle, C. (2001). High-temperature behaviour of HPC with polypropylene fibres: from spalling to microstructure. Cem. Concr. Res. 31 (10), 1487–1499. doi:10.1016/s0008-8846(01)00596-8
Kalifa, P., Menneteau, F.-D., and Quenard, D. (2000). Spalling and pore pressure in HPC at high temperatures. Cem. Concr. Res. 30 (12), 1915–1927. doi:10.1016/s0008-8846(00)00384-7
Khaliq, W., and Kodur, V. (2012). High temperature mechanical properties of high-strength fly ash concrete with and without fibers. ACI Mater. J. 109 (6). doi:10.14359/51684164
Khan, M., and Ali, M. (2018a). Effect of super plasticizer on the properties of medium strength concrete prepared with coconut fiber. Constr. Build. Mater. 182, 703–715. doi:10.1016/j.conbuildmat.2018.06.150
Khan, M., and Ali, M. (2018b). Effectiveness of hair and wave polypropylene fibers for concrete roads. Constr. Build. Mater. 166, 581–591. doi:10.1016/j.conbuildmat.2018.01.167
Khan, M., and Ali, M. (2019). Improvement in concrete behavior with fly ash, silica-fume and coconut fibres. Constr. Build. Mater. 203, 174–187. doi:10.1016/j.conbuildmat.2019.01.103
Khan, M., and Ali, M. (2016). Use of glass and nylon fibers in concrete for controlling early age micro cracking in bridge decks. Constr. Build. Mater. 125, 800–808. doi:10.1016/j.conbuildmat.2016.08.111
Khan, M., Cao, M., Ai, H., and Hussain, A. (2022a). Basalt fibers in modified whisker reinforced cementitious composites. Period. Polytech. Civ. Eng. 66 (2), 344–354. doi:10.3311/ppci.18965
Khan, M., Cao, M., and Ali, M. (2018). Effect of basalt fibers on mechanical properties of calcium carbonate whisker-steel fiber reinforced concrete. Constr. Build. Mater. 192, 742–753. doi:10.1016/j.conbuildmat.2018.10.159
Khan, M., and Cao, M. (2021). Effect of hybrid basalt fibre length and content on properties of cementitious composites. Mag. Concr. Res. 73 (10), 487–498. doi:10.1680/jmacr.19.00226
Khan, M., Lao, J., Ahmad, M. R., Kai, M.-F., and Dai, J.-G. (2023). The role of calcium aluminate cement in developing an efficient ultra-high performance concrete resistant to explosive spalling under high temperatures. Constr. Build. Mater. 384, 131469. doi:10.1016/j.conbuildmat.2023.131469
Khan, M., Lao, J., and Dai, J.-G. (2022b). Comparative study of advanced computational techniques for estimating the compressive strength of UHPC. J. Asian Concr. Fed. 8 (1), 51–68. doi:10.18702/acf.2022.6.8.1.51
Khan, M., Rehman, A., and Ali, M. (2020). Efficiency of silica-fume content in plain and natural fiber reinforced concrete for concrete road. Constr. Build. Mater. 244, 118382. doi:10.1016/j.conbuildmat.2020.118382
Khatib, J., Machaka, M., and Elkordi, A. (2022). Natural fibers. Handb. Sustain. Concr. Industrial Waste Manag., 85–107. doi:10.1016/b978-0-12-821730-6.00014-0
Khoury, G., and Willoughby, B. (2008). Polypropylene fibres in heated concrete. Part 1: molecular structure and materials behaviour. Mag. Concr. Res. 60 (2), 125–136. doi:10.1680/macr.2008.60.2.125
Kim, K.-H., Jeon, S.-E., Kim, J.-K., and Yang, S. (2003). An experimental study on thermal conductivity of concrete. Cem. Concr. Res. 33 (3), 363–371. doi:10.1016/s0008-8846(02)00965-1
Kim, W., Choi, H., and Lee, T. (2023). Investigating ultrasonic pulse velocity method for evaluating high-temperature properties of non-sintered hwangto-mixed concrete as a cement replacement material. Materials 16 (3), 1099. [Online]. doi:10.3390/ma16031099
Kim, Y.-s., Lee, T.-g., and Kim, G.-y. (2013). An experimental study on the residual mechanical properties of fiber reinforced concrete with high temperature and load. Mater. Struct. 46 (4), 607–620. doi:10.1617/s11527-012-9918-y
Kodur, V., and Dwaikat, M. (2008). A numerical model for predicting the fire resistance of reinforced concrete beams. Cem. Concr. Compos. 30 (5), 431–443. doi:10.1016/j.cemconcomp.2007.08.012
Kodur, V., and Khaliq, W. (2011). Effect of temperature on thermal properties of different types of high-strength concrete. J. Mater. Civ. Eng. 23 (6), 793–801. doi:10.1061/(asce)mt.1943-5533.0000225
Krishna, A., Kaliyaperumal, S. R. M., and Kathirvel, P. (2022). Compressive strength and impact resistance of hybrid fiber reinforced concrete exposed to elevated temperatures. Struct. Concr. 23 (3), 1611–1624. doi:10.1002/suco.202100566
Krishna, D. A., Priyadarsini, R., and Narayanan, S. (2019). Effect of elevated temperatures on the mechanical properties of concrete. Procedia Struct. Integr. 14, 384–394. doi:10.1016/j.prostr.2019.05.047
Lao, J.-C., Huang, B.-T., Xu, L.-Y., Khan, M., Fang, Y., and Dai, J.-G. (2023a). Seawater sea-sand Engineered Geopolymer Composites (EGC) with high strength and high ductility. Cem. Concr. Compos. 138, 104998. doi:10.1016/j.cemconcomp.2023.104998
Lao, J.-C., Xu, L.-Y., Huang, B.-T., Zhu, J.-X., Khan, M., and Dai, J.-G. (2023b). Utilization of sodium carbonate activator in strain-hardening ultra-high-performance geopolymer concrete (SH-UHPGC). Front. Mater. 10, 1–12. doi:10.3389/fmats.2023.1142237
Laranjeira, F., Molins, C., and Aguado, A. (2010). Predicting the pullout response of inclined hooked steel fibers. Cem. Concr. Res. 40 (10), 1471–1487. doi:10.1016/j.cemconres.2010.05.005
Lau, A., and Anson, M. (2006). Effect of high temperatures on high performance steel fibre reinforced concrete. Cem. Concr. Res. 36 (9), 1698–1707. doi:10.1016/j.cemconres.2006.03.024
Li, B., Zheng, W., and Zhang, Y. (2023). Study on the compressive stress–strain curve and performance of low-slump polypropylene fiber concrete after high temperature. Appl. Sci. 13 (12), 7222. [Online]. doi:10.3390/app13127222
Li, D., Niu, D., Fu, Q., and Luo, D. (2020). Fractal characteristics of pore structure of hybrid Basalt–Polypropylene fibre-reinforced concrete. Cem. Concr. Compos. 109, 103555. doi:10.1016/j.cemconcomp.2020.103555
Li, L., Khan, M., Bai, C., and Shi, K. (2021a). Uniaxial tensile behavior, flexural properties, empirical calculation and microstructure of multi-scale fiber reinforced cement-based material at elevated temperature. Materials 14 (8), 1827. doi:10.3390/ma14081827
Li, L., Zhang, R., Jin, L., Du, X., Wu, J., and Duan, W. (2019a). Experimental study on dynamic compressive behavior of steel fiber reinforced concrete at elevated temperatures. Constr. Build. Mater. 210, 673–684. doi:10.1016/j.conbuildmat.2019.03.138
Li, M., Qian, C., and Sun, W. (2004). Mechanical properties of high-strength concrete after fire. Cem. Concr. Res. 34 (6), 1001–1005. doi:10.1016/j.cemconres.2003.11.007
Li, Y., Pimienta, P., Pinoteau, N., and Tan, K. H. (2019b). Effect of aggregate size and inclusion of polypropylene and steel fibers on explosive spalling and pore pressure in ultra-high-performance concrete (UHPC) at elevated temperature. Cem. Concr. Compos. 99, 62–71. doi:10.1016/j.cemconcomp.2019.02.016
Li, Y., Tan, K. H., and Yang, E.-H. (2018). Influence of aggregate size and inclusion of polypropylene and steel fibers on the hot permeability of ultra-high performance concrete (UHPC) at elevated temperature. Constr. Build. Mater. 169, 629–637. doi:10.1016/j.conbuildmat.2018.01.105
Li, Y., Yang, E.-H., Zhou, A., and Liu, T. (2021b). Pore pressure build-up and explosive spalling in concrete at elevated temperature: A review. Constr. Build. Mater. 284, 122818. doi:10.1016/j.conbuildmat.2021.122818
Lim, S. (2015). Effects of elevated temperature exposure on cement-based composite materials. University of Illinois at Urbana-Champaign.
Liu, J.-C., and Tan, K. H. (2018a). Fire resistance of ultra-high performance strain hardening cementitious composite: residual mechanical properties and spalling resistance. Cem. Concr. Compos. 89, 62–75. doi:10.1016/j.cemconcomp.2018.02.014
Liu, J.-C., and Tan, K. H. (2018b). Mechanism of PVA fibers in mitigating explosive spalling of engineered cementitious composite at elevated temperature. Cem. Concr. Compos. 93, 235–245. doi:10.1016/j.cemconcomp.2018.07.015
Liu, J.-C., Tan, K. H., and Yao, Y. (2018). A new perspective on nature of fire-induced spalling in concrete. Constr. Build. Mater. 184, 581–590. doi:10.1016/j.conbuildmat.2018.06.204
Liu, J.-C., Tan, K. H., and Zhang, D. (2017). Multi-response optimization of post-fire performance of strain hardening cementitious composite. Cem. Concr. Compos. 80, 80–90. doi:10.1016/j.cemconcomp.2017.03.001
Luo, X., Sun, W., and Chan, S. Y. N. (2000). Effect of heating and cooling regimes on residual strength and microstructure of normal strength and high-performance concrete. Cem. Concr. Res. 30 (3), 379–383. doi:10.1016/s0008-8846(99)00264-1
Ma, Q., Guo, R., Zhao, Z., Lin, Z., and He, K. (2015). Mechanical properties of concrete at high temperature—a review. Constr. Build. Mater. 93, 371–383. doi:10.1016/j.conbuildmat.2015.05.131
Machovič, V., Lapčák, L., Borecka, L., Lhotka, M., Andertova, J., Kopecký, L., et al. (2013). Microstructure of interfacial transition zone between pet fibres and cement paste. Acta Geodyn. Geomaterialia 10 (1), 121–127. doi:10.13168/agg.2013.0012
Markoulli, M. P., Lee, C. I., Byington, E., and Felps, W. A. (2017). Mapping human resource management: reviewing the field and charting future directions. Hum. Resour. Manag. Rev. 27 (3), 367–396. doi:10.1016/j.hrmr.2016.10.001
Marques, A., Correia, J., and De Brito, J. (2013). Post-fire residual mechanical properties of concrete made with recycled rubber aggregate. Fire Saf. J. 58, 49–57. doi:10.1016/j.firesaf.2013.02.002
Meyer, C. (2009). The greening of the concrete industry. Cem. Concr. Compos. 31 (8), 601–605. doi:10.1016/j.cemconcomp.2008.12.010
Mohamad Moasas, A., Amin, M. N., Ahmad, W., Khan, K., Al-Hashem, M. N., Qureshi, H. J., et al. (2022). Bibliographic trends in mineral fiber-reinforced concrete: A scientometrics analysis. Front. Mater. 9, 1100276. doi:10.3389/fmats.2022.1100276
Noumowe, A., Clastres, P., Debicki, G., and Costaz, J. (1995). “High performance concrete for severe thermal conditions,” in Proceedings of the international conference on concrete under severe conditions, CONSEC), 1129–1140.
Oraee, M., Hosseini, M. R., Papadonikolaki, E., Palliyaguru, R., and Arashpour, M. (2017). Collaboration in BIM-based construction networks: A bibliometric-qualitative literature review. Int. J. Proj. Manag. 35 (7), 1288–1301. doi:10.1016/j.ijproman.2017.07.001
Ozawa, M., Parajuli, S. S., Uchida, Y., and Zhou, B. (2019). Preventive effects of polypropylene and jute fibers on spalling of UHPC at high temperatures in combination with waste porous ceramic fine aggregate as an internal curing material. Constr. Build. Mater. 206, 219–225. doi:10.1016/j.conbuildmat.2019.02.056
Ozawa, M., Uchida, S., Kamada, T., and Morimoto, H. (2012). Study of mechanisms of explosive spalling in high-strength concrete at high temperatures using acoustic emission. Constr. Build. Mater. 37, 621–628. doi:10.1016/j.conbuildmat.2012.06.070
Park, J.-J., Yoo, D.-Y., Kim, S., and Kim, S.-W. (2019). Benefits of synthetic fibers on the residual mechanical performance of UHPFRC after exposure to ISO standard fire. Cem. Concr. Compos. 104, 103401. doi:10.1016/j.cemconcomp.2019.103401
Park, J. Y., and Nagy, Z. (2018). Comprehensive analysis of the relationship between thermal comfort and building control research-A data-driven literature review. Renew. Sustain. Energy Rev. 82, 2664–2679. doi:10.1016/j.rser.2017.09.102
Peng, G.-F., Yang, W.-W., Zhao, J., Liu, Y.-F., Bian, S.-H., and Zhao, L.-H. (2006). Explosive spalling and residual mechanical properties of fiber-toughened high-performance concrete subjected to high temperatures. Cem. Concr. Res. 36 (4), 723–727. doi:10.1016/j.cemconres.2005.12.014
Poon, C. S., Shui, Z., and Lam, L. (2004a). Compressive behavior of fiber reinforced high-performance concrete subjected to elevated temperatures. Cem. Concr. Res. 34 (12), 2215–2222. doi:10.1016/j.cemconres.2004.02.011
Poon, C. S., Shui, Z., Lam, L., Fok, H., and Kou, S. (2004b). Influence of moisture states of natural and recycled aggregates on the slump and compressive strength of concrete. Cem. Concr. Res. 34 (1), 31–36. doi:10.1016/s0008-8846(03)00186-8
Purkiss, J. (1984). Steel fibre reinforced concrete at elevated temperatures. Int. J. Cem. Compos. Lightweight Concr. 6 (3), 179–184. doi:10.1016/0262-5075(84)90006-x
Rawat, S., Lee, C., and Zhang, Y. (2021). Performance of fibre-reinforced cementitious composites at elevated temperatures: A review. Constr. Build. Mater. 292, 123382. doi:10.1016/j.conbuildmat.2021.123382
Riaz Ahmad, M., Khan, M., Wang, A., Zhang, Z., and Dai, J.-G. (2023). Alkali-activated materials partially activated using flue gas residues: an insight into reaction products. Constr. Build. Mater. 371, 130760. doi:10.1016/j.conbuildmat.2023.130760
Romualdi, J. P., Ramey, M., and Sanday, S. C. (1968). Prevention and control of cracking by use of short random fibers. Spec. Publ. 20, 179–204.
Sancak, E., Sari, Y. D., and Simsek, O. (2008). Effects of elevated temperature on compressive strength and weight loss of the light-weight concrete with silica fume and superplasticizer. Cem. Concr. Compos. 30 (8), 715–721. doi:10.1016/j.cemconcomp.2008.01.004
Sanchayan, S., and Foster, S. J. (2016). High temperature behaviour of hybrid steel–PVA fibre reinforced reactive powder concrete. Mater. Struct. 49, 769–782. doi:10.1617/s11527-015-0537-2
Savastano, H., Santos, S., Radonjic, M., and Soboyejo, W. (2009). Fracture and fatigue of natural fiber-reinforced cementitious composites. Cem. Concr. Compos. 31 (4), 232–243. doi:10.1016/j.cemconcomp.2009.02.006
Serrano, R., Cobo, A., Prieto, M. I., and de las Nieves González, M. (2016). Analysis of fire resistance of concrete with polypropylene or steel fibers. Constr. Build. Mater. 122, 302–309. doi:10.1016/j.conbuildmat.2016.06.055
Shah, I., Li, J., Yang, S., Zhang, Y., and Anwar, A. (2022). Experimental investigation on the mechanical properties of natural fiber reinforced concrete. J. Renew. Mater. 10 (5), 1307–1320. doi:10.32604/jrm.2022.017513
Shaikh, F. U. A., and Hosan, A. (2016). Mechanical properties of steel fibre reinforced geopolymer concretes at elevated temperatures. Constr. Build. Mater. 114, 15–28. doi:10.1016/j.conbuildmat.2016.03.158
Shi, T., Liu, Y., Hu, Z., Cen, M., Zeng, C., Xu, J., et al. (2022a). Deformation performance and fracture toughness of carbon nanofiber-modified cement-based materials. ACI Mater. J. 119 (5), 119–128. doi:10.14359/51735976
Shi, T., Liu, Y., Zhao, X., Wang, J., Zhao, Z., Corr, D. J., et al. (2022b). Study on mechanical properties of the interfacial transition zone in carbon nanofiber-reinforced cement mortar based on the PeakForce tapping mode of atomic force microscope. J. Build. Eng. 61, 105248. doi:10.1016/j.jobe.2022.105248
Shin, A. H.-C., and Kodide, U. (2012). Thermal conductivity of ternary mixtures for concrete pavements. Cem. Concr. Compos. 34 (4), 575–582. doi:10.1016/j.cemconcomp.2011.11.009
Sideris, K., Manita, P., and Chaniotakis, E. (2009). Performance of thermally damaged fibre reinforced concretes. Constr. Build. Mater. 23 (3), 1232–1239. doi:10.1016/j.conbuildmat.2008.08.009
Sim, J., Park, C., and Moon, D. Y. (2005). Characteristics of basalt fiber as a strengthening material for concrete structures. Compos. Part B Eng. 36 (6-7), 504–512. doi:10.1016/j.compositesb.2005.02.002
Solhmirzaei, R., and Kodur, V. (2017). Modeling the response of ultra high performance fiber reinforced concrete beams. Procedia Eng. 210, 211–219. doi:10.1016/j.proeng.2017.11.068
Song, H., Liu, J., He, K., and Ahmad, W. (2021). A comprehensive overview of jute fiber reinforced cementitious composites. Case Stud. Constr. Mater. 15, e00724. doi:10.1016/j.cscm.2021.e00724
Sukontasukkul, P., Pomchiengpin, W., and Songpiriyakij, S. (2010). Post-crack (or post-peak) flexural response and toughness of fiber reinforced concrete after exposure to high temperature. Constr. Build. Mater. 24 (10), 1967–1974. doi:10.1016/j.conbuildmat.2010.04.003
Sun, L., Wang, C., Zhang, C., Yang, Z., Li, C., and Qiao, P. (2022). Experimental investigation on the bond performance of sea sand coral concrete with FRP bar reinforcement for marine environments. Adv. Struct. Eng. 26 (3), 533–546. doi:10.1177/13694332221131153
Sun, X., Chen, Z., Sun, Z., Wu, S., Guo, K., Dong, Z., et al. (2023). High-efficiency utilization of waste shield slurry: A geopolymeric flocculation-filtration-solidification method. Constr. Build. Mater. 387, 131569. doi:10.1016/j.conbuildmat.2023.131569
Tai, Y.-S., Pan, H.-H., and Kung, Y.-N. (2011a). Mechanical properties of steel fiber reinforced reactive powder concrete following exposure to high temperature reaching 800 C. Nucl. Eng. Des. 241 (7), 2416–2424. doi:10.1016/j.nucengdes.2011.04.008
Tai, Y.-S., Pan, H.-H., and Kung, Y.-N. (2011b). Mechanical properties of steel fiber reinforced reactive powder concrete following exposure to high temperature reaching 800°C. Nucl. Eng. Des. 241 (7), 2416–2424. doi:10.1016/j.nucengdes.2011.04.008
Varona, F., Baeza, F. J., Bru, D., and Ivorra, S. (2018a). Evolution of the bond strength between reinforcing steel and fibre reinforced concrete after high temperature exposure. Constr. Build. Mater. 176, 359–370. doi:10.1016/j.conbuildmat.2018.05.065
Varona, F. B., Baeza, F. J., Bru, D., and Ivorra, S. (2018b). Influence of high temperature on the mechanical properties of hybrid fibre reinforced normal and high strength concrete. Constr. Build. Mater. 159, 73–82. doi:10.1016/j.conbuildmat.2017.10.129
Wang, M., Yang, X., and Wang, W. (2022). Establishing a 3D aggregates database from X-ray CT scans of bulk concrete. Constr. Build. Mater. 315, 125740. doi:10.1016/j.conbuildmat.2021.125740
Wróblewski, R., and Stawiski, B. (2020). Ultrasonic assessment of the concrete residual strength after a real fire exposure. Buildings 10 (9), 154. doi:10.3390/buildings10090154
Wu, Z., Shi, C., He, W., and Wu, L. (2016). Effects of steel fiber content and shape on mechanical properties of ultra high performance concrete. Constr. Build. Mater. 103, 8–14. doi:10.1016/j.conbuildmat.2015.11.028
Wuni, I. Y., Shen, G. Q., and Osei-Kyei, R. (2019). Scientometrics review of global research trends on green buildings in construction journals from 1992 to 2018. Energy Build. 190, 69–85. doi:10.1016/j.enbuild.2019.02.010
Xiao, J., Xie, Q., Li, Z., and Wang, W. (2017). Fire resistance and post-fire seismic behavior of high strength concrete shear walls. Fire Technol. 53, 65–86. doi:10.1007/s10694-016-0582-6
Xie, J.-h., Guo, Y.-c., Liu, L.-s., and Xie, Z.-h. (2015). Compressive and flexural behaviours of a new steel-fibre-reinforced recycled aggregate concrete with crumb rubber. Constr. Build. Mater. 79, 263–272. doi:10.1016/j.conbuildmat.2015.01.036
Xie, J., Zhang, Z., Lu, Z., and Sun, M. (2018). Coupling effects of silica fume and steel-fiber on the compressive behaviour of recycled aggregate concrete after exposure to elevated temperature. Constr. Build. Mater. 184, 752–764. doi:10.1016/j.conbuildmat.2018.07.035
Xu, Y., Wong, Y., Poon, C. S., and Anson, M. (2001). Impact of high temperature on PFA concrete. Cem. Concr. Res. 31 (7), 1065–1073. doi:10.1016/s0008-8846(01)00513-0
Yang, L., Lin, X., and Gravina, R. J. (2018). Evaluation of dynamic increase factor models for steel fibre reinforced concrete. Constr. Build. Mater. 190, 632–644. doi:10.1016/j.conbuildmat.2018.09.085
Yang, L., Lin, X., Li, H., and Gravina, R. J. (2019a). A new constitutive model for steel fibre reinforced concrete subjected to dynamic loads. Compos. Struct. 221, 110849. doi:10.1016/j.compstruct.2019.04.021
Yang, L., Qi, C., Lin, X., Li, J., and Dong, X. (2019b). Prediction of dynamic increase factor for steel fibre reinforced concrete using a hybrid artificial intelligence model. Eng. Struct. 189, 309–318. doi:10.1016/j.engstruct.2019.03.105
Yang, S., Millard, S., Soutsos, M., Barnett, S., and Le, T. T. (2009). Influence of aggregate and curing regime on the mechanical properties of ultra-high performance fibre reinforced concrete (UHPFRC). Constr. Build. Mater. 23 (6), 2291–2298. doi:10.1016/j.conbuildmat.2008.11.012
Yang, Y., Zhou, Q., Li, X., Lum, G. C., and Deng, Y. (2019c). Uniaxial compression mechanical property and fracture behavior of hybrid inorganic short mineral fibers reinforced cement-based material. Cem. Concr. Compos. 104, 103338. doi:10.1016/j.cemconcomp.2019.103338
Yermak, N., Pliya, P., Beaucour, A.-L., Simon, A., and Noumowé, A. (2017). Influence of steel and/or polypropylene fibres on the behaviour of concrete at high temperature: spalling, transfer and mechanical properties. Constr. Build. Mater. 132, 240–250. doi:10.1016/j.conbuildmat.2016.11.120
Yoo, D.-Y., Banthia, N., Kang, S.-T., and Yoon, Y.-S. (2016). Effect of fiber orientation on the rate-dependent flexural behavior of ultra-high-performance fiber-reinforced concrete. Compos. Struct. 157, 62–70. doi:10.1016/j.compstruct.2016.08.023
Yu, F., and Hayes, B. E. (2018). Applying data analytics and visualization to assessing the research impact of the cancer cell biology (CCB) program at the university of North Carolina at chapel hill. J. eScience Librariansh. 7 (1), e1123. doi:10.7191/jeslib.2018.1123
Yu, K.-Q., Yu, J.-T., Dai, J.-G., Lu, Z.-D., and Shah, S. P. (2018). Development of ultra-high performance engineered cementitious composites using polyethylene (PE) fibers. Constr. Build. Mater. 158, 217–227. doi:10.1016/j.conbuildmat.2017.10.040
Zhang, B., Ahmad, W., Ahmad, A., Aslam, F., and Joyklad, P. (2022a). A scientometrics analysis approach to analyze the present research on recycled aggregate concrete. J. Build. Eng. 46, 103679. doi:10.1016/j.jobe.2021.103679
Zhang, D., and Ju, L. (2005). Effects of hybrid fiber on HPC properties under hightemperature. Ind. Constr. 35, 8–14.
Zhang, D., Liu, Y., and Tan, K. H. (2021a). Spalling resistance and mechanical properties of strain-hardening ultra-high performance concrete at elevated temperature. Constr. Build. Mater. 266, 120961. doi:10.1016/j.conbuildmat.2020.120961
Zhang, D., Tan, G. Y., and Tan, K. H. (2021b). Combined effect of flax fibers and steel fibers on spalling resistance of ultra-high performance concrete at high temperature. Cem. Concr. Compos. 121, 104067. doi:10.1016/j.cemconcomp.2021.104067
Zhang, D., Tan, K. H., Dasari, A., and Weng, Y. (2020a). Effect of natural fibers on thermal spalling resistance of ultra-high performance concrete. Cem. Concr. Compos. 109, 103512. doi:10.1016/j.cemconcomp.2020.103512
Zhang, D., and Tan, K. H. (2020). Effect of various polymer fibers on spalling mitigation of ultra-high performance concrete at high temperature. Cem. Concr. Compos. 114, 103815. doi:10.1016/j.cemconcomp.2020.103815
Zhang, H. Y., Qiu, G. H., Kodur, V., and Yuan, Z. S. (2020b). Spalling behavior of metakaolin-fly ash based geopolymer concrete under elevated temperature exposure. Cem. Concr. Compos. 106, 103483. doi:10.1016/j.cemconcomp.2019.103483
Zhang, P., Kang, L., Wang, J., Guo, J., Hu, S., and Ling, Y. (2020c). Mechanical properties and explosive spalling behavior of steel-fiber-reinforced concrete exposed to high temperature—a review. Appl. Sci. 10 (7), 2324. [Online]. doi:10.3390/app10072324
Zhang, W., Liu, X., Huang, Y., and Tong, M.-N. (2022b). Reliability-based analysis of the flexural strength of concrete beams reinforced with hybrid BFRP and steel rebars. Archives Civ. Mech. Eng. 22 (4), 171. doi:10.1007/s43452-022-00493-7
Zhao, J., Gao, D., and Wang, B. (2006). The experimental study on mechanical property of steel fiber reinforced high strength concrete after high temperature. Concrete 28, 4–6.
Zhao, J., Zheng, J.-J., Peng, G.-F., and van Breugel, K. (2017). Numerical analysis of heating rate effect on spalling of high-performance concrete under high temperature conditions. Constr. Build. Mater. 152, 456–466. doi:10.1016/j.conbuildmat.2017.07.023
Zheng, W., Li, H., and Wang, Y. (2012a). Compressive behaviour of hybrid fiber-reinforced reactive powder concrete after high temperature. Mater. Des. 41, 403–409. doi:10.1016/j.matdes.2012.05.026
Zheng, W., Li, H., and Wang, Y. (2012b). Compressive stress–strain relationship of steel fiber-reinforced reactive powder concrete after exposure to elevated temperatures. Constr. Build. Mater. 35, 931–940. doi:10.1016/j.conbuildmat.2012.05.031
Zheng, W., Luo, B., and Wang, Y. (2013). Compressive and tensile properties of reactive powder concrete with steel fibres at elevated temperatures. Constr. Build. Mater. 41, 844–851. doi:10.1016/j.conbuildmat.2012.12.066
Zheng, W., Luo, B., and Wang, Y. (2015). Stress–strain relationship of steel-fibre reinforced reactive powder concrete at elevated temperatures. Mater. Struct. 48, 2299–2314. doi:10.1617/s11527-014-0312-9
Zhong, B., Wu, H., Li, H., Sepasgozar, S., Luo, H., and He, L. (2019). A scientometrics analysis and critical review of construction related ontology research. Automation Constr. 101, 17–31. doi:10.1016/j.autcon.2018.12.013
Zhou, S., Lu, C., Zhu, X., and Li, F. (2021). Preparation and characterization of high-strength geopolymer based on BH-1 lunar soil simulant with low alkali content. Engineering 7 (11), 1631–1645. doi:10.1016/j.eng.2020.10.016
Zīle, E., and Zīle, O. (2013). Effect of the fiber geometry on the pullout response of mechanically deformed steel fibers. Cem. Concr. Res. 44, 18–24. doi:10.1016/j.cemconres.2012.10.014
Keywords: fire resistance, spalling resistance, spalling mechanism, residual strength, fibre reinforced concrete, refractory material
Citation: Gong F, Jiang X, Gamil Y, Iftikhar B and Thomas BS (2023) An overview on spalling behavior, mechanism, residual strength and microstructure of fiber reinforced concrete under high temperatures. Front. Mater. 10:1258195. doi: 10.3389/fmats.2023.1258195
Received: 13 July 2023; Accepted: 10 August 2023;
Published: 08 September 2023.
Edited by:
Sahar A. Mostafa, Beni-Suef University, EgyptReviewed by:
Junfeng Guan, North China University of Water Conservancy and Electric Power, ChinaRawaz Kurda, Erbil Polytechnic University, Iraq
Copyright © 2023 Gong, Jiang, Gamil, Iftikhar and Thomas. This is an open-access article distributed under the terms of the Creative Commons Attribution License (CC BY). The use, distribution or reproduction in other forums is permitted, provided the original author(s) and the copyright owner(s) are credited and that the original publication in this journal is cited, in accordance with accepted academic practice. No use, distribution or reproduction is permitted which does not comply with these terms.
*Correspondence: Fang Gong, Z29uZ2ZhbmcxOTgzQDE2My5jb20=; Yaser Gamil, eWFzZXIuZ2FtaWxAbHR1LnNl