- 1Department of Medicine, Western Michigan University Homer Stryker M. D. School of Medicine, Kalamazoo, MI, United States
- 2Division of Biomedical Engineering, Department of Orthopedic Surgery, Western Michigan University Homer Stryker M. D. School of Medicine, Kalamazoo, MI, United States
Repair after injury in mammalian tissue involves a complex cascade of events, with the formation of local blood clots being essential for the initial phases of wound healing. As a result, emerging research has sought to harness this biological activity to generate a pro-regenerative biomaterial to speed up wound healing. According to recent studies, “blood clots” created in vitro can be employed as an orthobiologic-based biomaterial for promoting tissue regeneration. Even though such research is still in its early phases, numerous studies show encouraging results that suggest autologous blood clots created in vitro might be a valuable treatment for soft tissue and orthopedic injuries. In this article, we discuss the function of blood clots in physiologic healing, how exogenous material can affect this process, and the most recent clinical research that proposes the use of autologous blood clots as a therapeutically beneficial biomaterial.
1 Introduction
Through the processes of primary, secondary, and tertiary hemostasis, whole blood creates a natural, in vivo biomaterial that not only reduces hemorrhage, but also provides a robust scaffold for the healing of injured tissues. This scaffold is also biologically active, establishing a pro-regenerative local environment and recruiting adjacent cells to foster tissue remodeling (Weisel, 2010; Tran et al., 2013). Therefore, the proposition that topical application of exogenously prepared autologous blood clots (BCs) can aid in wound healing has become an exciting focus of translational research. The unique mechanical characteristics of BCs allow the economical and rapid construction of a biocompatible scaffold with antiseptic and pro-regenerative properties (Snyder, 2005). Additionally, autologous BCs can be supplemented with exogenous material to further augment their mechanical properties, facilitating more significant clinical benefits. Thus, this review summarizes the structural properties that give autologous BCs the characteristics of a biomaterial, how such properties can be modulated, and current clinical investigations for BC’s therapeutic implications.
2 Mechanical properties of blood clots
The structural formation of BCs is a complex and dynamic process involving a series of biochemical and cellular events, which can be divided into several distinct stages that are crucial for their potential use as a biomaterial. More specifically, fibrin, a key biomaterial isolated from whole blood, has been researched since the 1940s for its distinct mechanical properties and positive implication across various tissue engineering applications (Park and Woo, 2018). Critical aspects of BC structure include formation and composition of the clot’s fibrin matrix, as well as the role of various proteins in the clotting process. Furthermore, the biomechanical properties of the fibrin matrix in the BC—such as its high tensile strength and viscoelastic behavior—make the BC a promising candidate for various biomedical applications. While significant research exists regarding the biochemistry of clot formation, the mechanical properties of clots as a whole substance have received limited investigation despite their potential significance in therapeutic applications and tissue regeneration.
2.1 Structural formation of blood clots
The overall structure of a BC is determined by the arrangement of individual fibrin fibers, which interlace to create a complex fibrin meshwork. During the clotting process, fibrinogen, a 340-kDa soluble glycoprotein, is cleaved by thrombin into an insoluble fibrin polymer, resulting in the release of fibrinopeptide FpA and FpB (Kattula et al., 2017). The release of FpB then triggers fibrin monomers to polymerize into half-staggered oligomers that aggregate to form protofibrils, which is the structural foundation of the clot’s fibrin meshwork (Weisel, 1986). As these protofibrils elongate to a critical length, they associate laterally, forming thicker fibrin fibers through staggered, half-molecule overlaps (Weisel, 2005). The interplay between branching and lateral aggregation of the fibers results in a three-dimensional network that defines a fibrin BC’s mechanical and structural properties (Undas and Ariëns, 2011) (Figure 1).
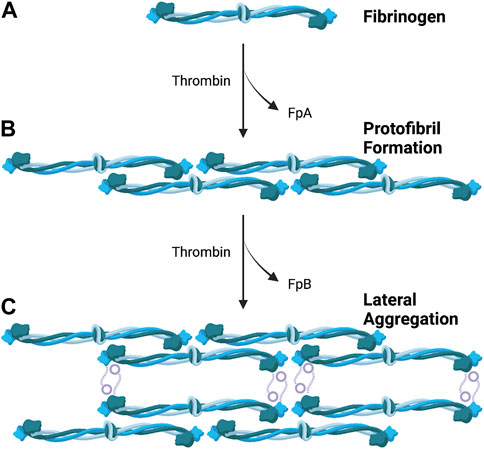
FIGURE 1. Formation of Fibrin Meshwork in Blood Clot. (A) Fibrinogen is cleaved by thrombin, leading to the release of fibrinopeptides (FpA and FpB). (B) Fibrin monomers polymerize into half-staggered oligomers, which aggregate to form protofibrils. (C) Protofibrils elongate and laterally associate, resulting in the formatting of thicker fibrin fibers through staggered, half-molecule overlaps. Figure made with BioRender.com.
Although this current model for clot formation has been extensively studied and is widely accepted, the mechanisms by which fibrin gel networks form from protofibrils—which are critical in the structural development of clots—remain less understood. Weisel and Nagaswami found that increased lateral association during BC formation resulted in clots composed of thicker fibers with fewer branches, whereas reduced lateral association led to the formation of clots with thinner fibers and more branches (Weisel and Nagaswami, 1992). More recently, a thrombin-free gelation pathway of fibrinogen was observed in acidic conditions in vitro, demonstrating a behavior that differed from the classical formation of fibrin BCs (Rieu et al., 2020). Given its autologous origin, fibrinogen provides a natural scaffold for clots intended as a biomaterial. Investigating the underlying mechanisms of fibrin formation could provide valuable insights into developing novel approaches for utilizing BCs as a biomaterial in various clinical settings.
The architecture of BCs exhibits variability contingent upon clot composition. Plasma clots have been extensively studied, but only limited studies have investigated the impact of RBCs on the structural characteristics of the formed clot. Notably, clots formed from platelet-rich plasma exhibit distinct structural arrangements compared to those from platelet-poor plasma (Roessler et al., 2011). Moreover, red blood cells have been found to enhance the thickness of fibers and promote the formation of a more aligned fibrin network within clots formed from whole blood (Gersh et al., 2009). Whole blood clots also exhibit increased resistance to lysis when compared to plasma clots (Varin et al., 2013). These findings highlight the importance of considering the role of red blood cells in clot architecture and warrant further investigation.
2.2 Viscoelastic and proelastic characteristics of blood clots
In the past few decades, extensive research has focused on the viscoelastic properties of BCs, specifically the varying degrees of elasticity and viscosity exhibited by fibrin—the main component of BCs (Litvinov and Weisel, 2016). Understanding these mechanical properties is crucial due to their significant implications for biological and clinical applications. The reversible elastic properties of the fibrin polymer contribute to its stiffness, while the irreversible characteristics of viscosity describe its inelastic behavior (Weisel, 2004). Such viscoelastic parameters—determined by the structural composition of BCs—play a vital role in defining the extent of deformation observed under stress (Johnson et al., 2021). As a result, comprehensive studies have utilized compression, deformation, and tension tests to establish well-defined mechanisms to characterize the mechanical characteristics of blood clots (Sugerman et al., 2020).
Although the mechanical behavior of BCs has received significant attention, one area that remains underexplored is the poroelastic behavior of clots. Remarkably, both viscoelasticity and poroelasticity have been found to coexist in regenerative structures, including BCs (Gersh et al., 2009). The presence of hallmark features of poroelasticity, such as permeability and fluid transport, has been observed in BCs—emphasizing their poroelastic nature (Chaudhuri et al., 2020). Previous studies have demonstrated the migration of fluids through the porous matrix of BCs, composed of RBCs within a fibrin network, providing further evidence of their poroelastic behavior (Ghezelbash et al., 2022). Moreover, the specific contributions of viscoelasticity and poroelasticity to the function of BCs remain largely unknown. In recent developments, viscoelastic biomaterials have emerged as potential for tissue development and regeneration (Wu et al., 2022). Given the coexistence of poroelasticity and viscoelasticity, exploring the role of poroelastic behavior in regenerative medicine and healing could provide valuable insight. A deeper understanding of the interplay between viscoelastic and poroelastic influences in BCs may have implications for their future use as biomaterials.
2.3 Alignment of fibrin network
Fibrin fiber alignment within BCs plays a crucial role in determining their mechanical properties and, thus, potential applications in regenerative medicine. Several mechanical and physical techniques have been investigated to achieve controlled alignment. Previous studies have primarily focused on platelet-poor or platelet-free clots when investigating fibrin fiber alignment. Magnetic fields of various strengths have shown promising results in aligning fibrin fibers (Torbet et al., 1981; Yamagishi et al., 1990). Fluid dynamics have also been investigated, with Campbell et al. demonstrating significant alignment of fibrin fibers parallel to flow when plasma clots flowed over fibroblasts (Campbell et al., 2010). Additionally, incorporating human mesenchymal stem cells (MSCs) into plasma clots, combined with electric fields, has achieved unidirectional alignment of fibrin fibers and MSCs (Gessmann et al., 2016).
Despite the success in orientating fibrin fibers using various techniques, the impact of mechanical alterations on BC properties and their potential therapeutic applications remains largely understudied. Nevertheless, longitudinal orientation of fibrin within autologous BCs makes them highly suitable scaffolds for axonal regrowth following traumatic peripheral nerve injuries (Gessmann et al., 2016). However, the underlying mechanisms of the effects of these techniques on BCs remain unclear. In addition to further research on plasma clots, autologous clots prepared from whole blood provide a natural reservoir of healing factors, making them a valuable subject for investigation (Snyder et al., 2020). Additional research is needed to enhance our understanding and optimize the design of aligned fibrin scaffolds to fully exploit their potential in therapeutic settings.
3 Conjugation of blood clots with exogenous materials
Given the potential for BCs to serve as an immunologically native biomaterial, growing research has focused on conjugating BCs with exogenous materials to create an improved scaffold for wound healing. One candidate currently being investigated is “blood for reconstruction of bone” (BRB), which contains biphasic calcium phosphate (BCP) microparticles embedded in an autologous BC (Balaguer et al., 2010). BCPs are a class of calcium phosphate ceramics with osteoinductive properties that have been investigated as potential surgical cement for endodontic, maxillofacial, and orthopedic procedures (Lobo and Livingston Arinzeh, 2010). Compared to BCP microparticles alone, BRB can be molded into segmental bone defects, creating a seal. As such, animal studies have demonstrated the ability of BRB to induce woven bone formation and defect repair (Balaguer et al., 2010; Lobo and Livingston Arinzeh, 2010). Furthermore, in 2020, a proof-of-concept study designed a BCP-BC composite mandibular plate, which was surgically implanted into a dog with a sequestered, unstable mandibular fracture. The surgery was considered a success, with radiologic evidence of osteointegration observed within 6 months and complete regeneration of alveolar ridge continuity (Girard et al., 2020). These results warrant further investigation of the material with potential application in human subjects.
3.1 Biphasic calcium phosphate microparticles
The concentration of BCP within the BC appears to play a role in directing tissue differentiation. Jing et al. demonstrated that, in mouse models, BCs containing 80–200 µm of BCP microparticles induced formation of ectopic woven bone, while concentrations of 200–500 µm induced mainly fibrous tissue (Jing et al., 2021). Such BCP-BC composites likely induce osteogenesis as they strongly upregulate the TLR4 signaling pathway and fibrinogen (FGN). FGN acts upon TLR4 through MyD88-dependent nuclear factor-κB activation. BCP particles also induce production of proteins involved in the HGF and IGF osteogenic pathways, likely through the TLR4 signaling cascade (Jing et al., 2021). Therefore, modulating the concentration of BCP within autologous blood preparations to tissue-specific levels may provide further benefit in wound healing.
3.2 Bone morphogenetic protein
Other investigations have sought to use BCs as a vehicle for therapeutic delivery, in addition to wound repair scaffolds. BMP-2, given orally or as a systemic injection, has been used in clinical practice to improve bone fracture repair and bone regeneration (Ho-Shui-Ling et al., 2018). However, systemic reviews have found fracture healing times to be comparable between BMP-2 and control groups, raising questions about its significance or benefits in bone repair (Garrison et al., 2010). Such conclusions may be due to current delivery methods, which result in relatively low BMP-2 concentration at the lesion site. Therefore, Fan et al. utilized a BC loaded with BMP-2 to supply high local concentrations (Fan et al., 2021). Essentially, the BMP-2-loaded BCs served as a moldable biomaterial that could fuse to the shape of the lesion, supplying high concentrations of the therapeutic BMP-2. As a result, BMP-2-loaded BCs stimulated osteogenesis, as demonstrated by elevated expression of the osteogenic proteins RUNX2 and Ostreix, and improved healing area of rat skull defects (Fan et al., 2021).
Similar investigations have designed BC calcium phosphate ceramic constructs (CPC) loaded with both BMP-2 and dexamethasone (Liu et al., 2022). Initial attempts to use CPC or BMP-2 alone as a scaffold to repair bone defects in mice resulted in delayed fracture healing and wound exudation in a non-significant portion of subjects (Lin et al., 2016). The hope was that combining Dex and BMP-2 within an immunologically native vector would quell the early-stage inflammatory response and promote fracture healing across a larger patient population. Indeed, the Dex/blood/BMP-2/CPC scaffold (6:1 DEX/BMP-2 ratio) yielded better bone mineralization and wound healing when applied to a rat calvaria defect than either DEX/BM.P-2/CPC (6:1 DEX/BMP-2 ratio) or blood clot alone. This result depended on Dex concentration, with a 9:1 DEX/BMP ratio resulting in decreased bone volume (Liu et al., 2022).
4 Therapeutic applications of autologous blood clots
Due to the ability of autologous BCs to serve as proteinaceous, immune-compatible scaffolds, a growing body of research has examined possible therapeutic applications of this orthobiologic as a natural biomaterial. A wide variety of studies—ranging from in vitro experiments to human trials—have suggested that autologous BCs may improve and accelerate tissue repair (Figure 2). Most notably, literature points to a role for autologous BCs as an orthobiologic therapeutic material for the treatment of specific orthopedic injuries, ulcers, and soft-tissue trauma.
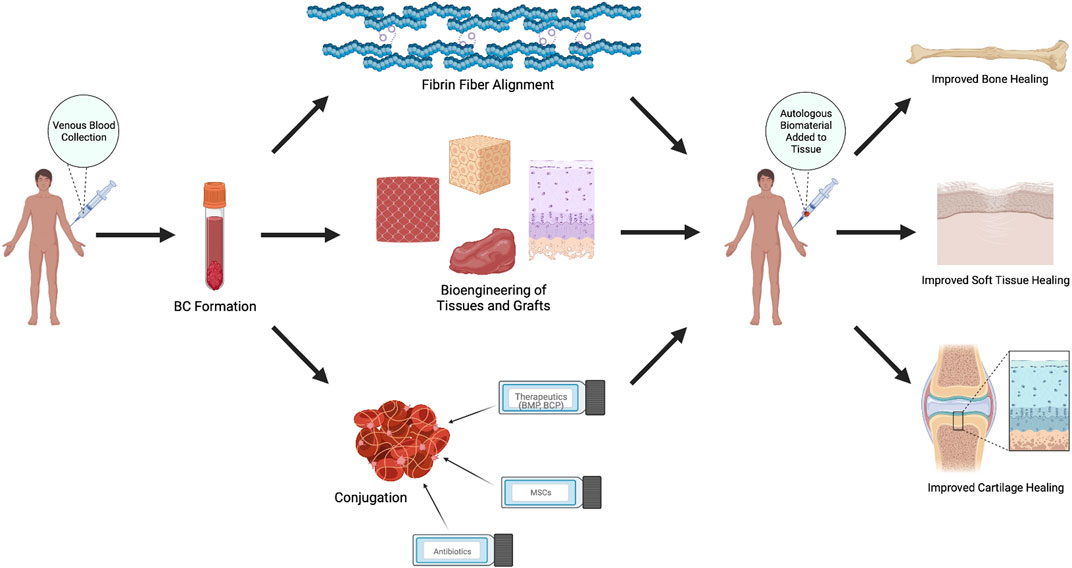
FIGURE 2. Therapeutic Applications of Autologous Blood Clots. Autologous blood clots are used in various animal and human models to induce healing and tissue regeneration through creation of stronger, unidirectional fibrin alignments, synthesis of biologic tissues and grafts, or conjugation with other materials, including therapeutics, ceramics, mesenchymal stem cells, drugs, and antibiotics. Figure made with BioRender.com.
4.1 Application of blood clots for the treatment of orthopedic injuries
Injury to articular cartilage fibrocartilage presents a particularly alluring application for autologous BCs due to this tissue’s limited capacity for repair (Armiento et al., 2019) and the high incidence of meniscus tears (Luvsannyam et al., 2022). Arzoczky et al. (1988) was the first to demonstrate the ability of autologous BCs to serve as a scaffold for meniscal repair in an animal model (Arnoczky et al., 1988). This technique was translated to humans by Kamimura and Kimura (2011), achieving restoration of horizontal cleavage tears of the meniscus following implantation of exogenously-clotted peripheral blood (Kamimura and Kimura, 2011). In a subsequent 10-patient case series, Kamimura and Kimura demonstrated complete healing in 70% of patients, in addition to statistically significant improvements in mean Lysholm score and International Knee Documentation Committee (IKDC) subjective knee scores (Kamimura and Kimura, 2014). Ra et al. applied a similar technique to complete radial tears of the meniscus, with 91.7% of patients showing complete resolution by MRI 30 months post-operation (Ra et al., 2013).
Furthermore, BCs are suggested to underscore the efficacy of microfracture surgery—a procedure used to stimulate repair of damaged articular cartilage of the knee joint. Following microfracture, blood clots serve as proteinaceous scaffolds for pluripotent cells, which differentiate into chondrocyte-like cells and produce cartilaginous tissue that results in injury repair (Steadman et al., 2010). Numerous animal studies have investigated the use of adjuvant compounds to enhance clot formation following microfracture, with mixed results (Schizas et al., 2019). Notably, Hoemann et al. demonstrated microfracture in combination with administration of chitosan-glycerol phosphate-supplemented BCs to be superior to microfracture alone (Hoemann et al., 2005).
In addition to therapeutic applications for cartilage repair, emerging evidence points to a potential therapeutic role for autologous BCs in bone repair. Following bone injury, blood clots play a crucial role in the early stages of tissue repair by serving as a biologically active scaffold (Yang and Xiao, 2020). In addition to the ability of BCs to serve as a biomaterial, autologous clots may also serve as a vehicle for delivery of repair-promoting factors. Thus, numerous animal studies have examined supplemented autologous blood as a potential therapeutic to accelerate tissue healing following fracture. Notably, Fan et al. (2021) examined the therapeutic potential of BMP-2-loaded autologous BCs for the treatment of large-area bone defects. Six weeks post-treatment, BMP-2-loaded autologous BCs resulted in >95% closure of skull defects in mouse models (Fan et al., 2021). Similarly, Grgurevic et al. found autologous BCs to be an effective delivery vehicle for BMP-6, resulting in improved healing of critical size segmental defects of the ulna in rabbit models (Grgurevic et al., 2019). Furthermore, a case report by Girad et al. showed autologous blood mixed with biphasic calcium phosphate microparticles to be an effective therapeutic for sizable mandibular bone defects in dog models (Girard et al., 2020). However, it is important to note that Gao et al. found autologous BCs to be inferior to fibrin sealants as a scaffold for promoting bone regeneration (Gao et al., 2021); thus, more research is needed to elucidate the ideal candidate for bone healing before progressing to clinical studies. That said, autologous BCs are theoretically advantageous over commercially available fibrin sealants. BCs contain both fibrin and other pro-healing factors and, thus, serve as a biologically active pro-healing orthobiologic that can be quickly prepared from patients’ own blood. Contrarily, fibrin sealants, alone, simply serve as a “patch” and must be supplemented to foster wound healing.
4.2 Application of autologous BCs for the treatment of soft tissue injury
While the use of autologous BCs for orthopedic injury is an emerging field, a role for BCs in soft tissue injury is more established. Interestingly, a consensus panel recently recommended employing autologous BCs to treat certain complex soft-tissue wounds (Snyder et al., 2023). As autologous BCs can be prepared quickly, they can be applied to function as biologically active wound dressings, bolstering the healing process and preventing infection (VanZweden et al., 2022). As pressure ulcers are difficult to treat and represent a significant cause of morbidity and mortality (Mervis and Phillips, 2019), numerous studies have attempted to elucidate the role of autologous BCs in this context. In numerous case studies, autologous BCs have been associated with favorable outcomes in pressure ulcers up to 42 cm2 and sizable healing in the context of significant injury—such as fibronecrotic wounds with exposed bone (Landau et al., 2023). A subsequent pilot study found that application of autologous BCs achieved complete wound healing in 78% of ulcers (Kushnir et al., 2016). Furthermore, in a small, randomized study, autologous BCs significantly reduced healing time and wound surface area (Singh et al., 2014). Although such data suggest autologous BCs may be an effective treatment for ulceration, larger clinical studies are needed to provide a definitive answer.
5 Limitations of autologous blood clots as a therapeutic
While autologous BCs are an appealing therapeutic, they are not without limitations. Presently, no rigorous studies have investigated the correlation between wound size, BC size, and therapeutic outcomes. The effectiveness of autologous BCs is inherently constrained by the volume of blood that can be extracted from a single patient, posing potential challenges in treating large traumatic injuries. Nevertheless, utilization to treat small to moderate-sized defects could circumvent this limitation. Furthermore, autologous BC size may have minimal significance on the healing process; rather, released factors from the BC may diffuse throughout the defect and underscore healing. This aspect has not been extensively explored and could be a promising topic for future investigations. Clinical use of autologous BCs may also be limited by time constraints imposed by blood coagulation. However, the use of chemical agents to reduce coagulation time could be investigated to achieve consistent results with BC therapy.
6 Conclusion
A crucial component of the physiologic response to tissue injury is the formation of blood clots, which are the foundation for tissue healing. Although it is well known that BCs promote the repair of many tissue types, their therapeutic value has not yet been fully elucidated. According to initial investigations, autologous BCs could be utilized as a biomaterial that accelerates wound healing, primarily because of their distinctive structural characteristics and capacity to complement exogenous pro-healing elements. Given these results, additional research is necessary to determine the clinical value of autologous blood clots in the healing of different tissues.
Author contributions
DR, JK, KK, LB, GA, and YL contributed to conception and design of this review. DR, JK, KK, and LB wrote sections of the manuscript. YL and GA supervised and structured drafting of the manuscript. All authors contributed to the article and approved the submitted version.
Funding
This review is partially supported by a pioneer grant from the Western Michigan University Homer Stryker M. D. School of Medicine.
Conflict of interest
YL is an executive editor for Journal of Cellular Biochemistry.
The remaining authors declare that the research was conducted in the absence of any commercial or financial relationships that could be construed as a potential conflict of interest.
Publisher’s note
All claims expressed in this article are solely those of the authors and do not necessarily represent those of their affiliated organizations, or those of the publisher, the editors and the reviewers. Any product that may be evaluated in this article, or claim that may be made by its manufacturer, is not guaranteed or endorsed by the publisher.
References
Armiento, A. R., Alini, M., and Stoddart, M. J. (2019). Articular fibrocartilage - why does hyaline cartilage fail to repair? Adv. Drug Deliv. Rev. 146, 289–305. doi:10.1016/j.addr.2018.12.015
Arnoczky, S. P., Warren, R. F., and Spivak, J. M. (1988). Meniscal repair using an exogenous fibrin clot. An experimental study in dogs. J. Bone Jt. Surg. Am. 70 (8), 1209–1217. doi:10.2106/00004623-198870080-00012
Balaguer, T., Boukhechba, F., Clavé, A., Bouvet-Gerbettaz, S., Trojani, C., Michiels, J. F., et al. (2010). Biphasic calcium phosphate microparticles for bone formation: Benefits of combination with blood clot. Tissue Eng. Part A 16 (11), 3495–3505. doi:10.1089/ten.tea.2010.0227
Campbell, R. A., Aleman, M., Gray, L. D., Falvo, M. R., and Wolberg, A. S. (2010). Flow profoundly influences fibrin network structure: Implications for fibrin formation and clot stability in haemostasis. Thromb. Haemost. 104 (6), 1281–1284. doi:10.1160/th10-07-0442
Chaudhuri, O., Cooper-White, J., Janmey, P. A., Mooney, D. J., and Shenoy, V. B. (2020). Effects of extracellular matrix viscoelasticity on cellular behaviour. Nature 584 (7822), 535–546. doi:10.1038/s41586-020-2612-2
Fan, Q., Bai, J., Shan, H., Fei, Z., Chen, H., Xu, J., et al. (2021). Implantable blood clot loaded with BMP-2 for regulation of osteoimmunology and enhancement of bone repair. Bioact. Mater 6 (11), 4014–4026. doi:10.1016/j.bioactmat.2021.04.008
Gao, X., Cheng, H., Sun, X., Lu, A., Ruzbarsky, J. J., Wang, B., et al. (2021). Comparison of autologous blood clots with fibrin sealant as scaffolds for promoting human muscle-derived stem cell-mediated bone regeneration. Biomedicines 9 (8), 983. doi:10.3390/biomedicines9080983
Garrison, K. R., Shemilt, I., Donell, S., Ryder, J. J., Mugford, M., Harvey, I., et al. (2010). Bone morphogenetic protein (BMP) for fracture healing in adults. Cochrane Database Syst. Rev. 2010, CD006950. doi:10.1002/14651858.cd006950.pub2
Gersh, K. C., Nagaswami, C., and Weisel, J. W. (2009). Fibrin network structure and clot mechanical properties are altered by incorporation of erythrocytes. Thromb. Haemost. 102 (6), 1169–1175. doi:10.1160/th09-03-0199
Gessmann, J., Seybold, D., Peter, E., Schildhauer, T. A., and Köller, M. (2016). Alignment of the fibrin network within an autologous plasma clot. Tissue Eng. Part C Methods 22 (1), 30–37. doi:10.1089/ten.tec.2015.0207
Ghezelbash, F., Liu, S., Shirazi-Adl, A., and Li, J. (2022). Blood clot behaves as a poro-visco-elastic material. J. Mech. Behav. Biomed. Mater 128, 105101. doi:10.1016/j.jmbbm.2022.105101
Girard, N., Cauvin, E. R. J., Gauthier, O., and Gault, S. (2020). Biphasic calcium phosphate microparticles mixed with autologous blood: Application for the reconstruction of a large mandibular bone defect in a dog. J. Vet. Dent. 37 (4), 201–209. doi:10.1177/0898756421990909
Grgurevic, L., Oppermann, H., Pecin, M., Erjavec, I., Capak, H., Pauk, M., et al. (2019). Recombinant human bone morphogenetic protein 6 delivered within autologous blood coagulum restores critical size segmental defects of ulna in rabbits. JBMR Plus 3 (5), e10085. doi:10.1002/jbm4.10085
Ho-Shui-Ling, A., Bolander, J., Rustom, L. E., Johnson, A. W., Luyten, F. P., and Picart, C. (2018). Bone regeneration strategies: Engineered scaffolds, bioactive molecules and stem cells current stage and future perspectives. Biomaterials 180, 143–162. doi:10.1016/j.biomaterials.2018.07.017
Hoemann, C. D., Hurtig, M., Rossomacha, E., Sun, J., Chevrier, A., Shive, M. S., et al. (2005). Chitosan-glycerol phosphate/blood implants improve hyaline cartilage repair in ovine microfracture defects. J. Bone Jt. Surg. 87 (12), 2671–2686. doi:10.2106/jbjs.d.02536
Jing, L., Rota, S., Olivier, F., Momier, D., Guigonis, J. M., Schaub, S., et al. (2021). Proteomic analysis identified LBP and CD14 as key proteins in blood/biphasic calcium phosphate microparticle interactions. Acta Biomater. 127, 298–312. doi:10.1016/j.actbio.2021.03.070
Johnson, S., McCarthy, R., Gilvarry, M., McHugh, P. E., and McGarry, J. P. (2021). Investigating the mechanical behavior of clot analogues through experimental and computational analysis. Ann. Biomed. Eng. 49 (1), 420–431. doi:10.1007/s10439-020-02570-5
Kamimura, T., and Kimura, M. (2014). Meniscal repair of degenerative horizontal cleavage tears using fibrin clots. Orthop. J. Sports Med. 2 (11), 232596711455567. doi:10.1177/2325967114555678
Kamimura, T., and Kimura, M. (2011). Repair of horizontal meniscal cleavage tears with exogenous fibrin clots. Knee Surg. Sports Traumatol. Arthrosc. 19 (7), 1154–1157. doi:10.1007/s00167-011-1404-5
Kattula, S., Byrnes, J. R., and Wolberg, A. S. (2017). Fibrinogen and fibrin in hemostasis and thrombosis. Arteriosclerosis, Thrombosis, Vasc. Biol. 37, e13–e21. Lippincott Williams and Wilkins. doi:10.1161/ATVBAHA.117.308564
Kushnir, I., Kushnir, A., Serena, T. E., and Garfinkel, D. (2016). Efficacy and safety of a novel autologous wound matrix in the management of complicated, chronic wounds: A pilot study. Wounds 28 (9), 317–327.
Landau, Z., Whitacre, K. L., Leewood, C., Hawkins, J., and Wachuku, C. D. (2023). Utilization of a topical autologous blood clot for treatment of pressure ulcers. Int. Wound J. 20 (3), 806–812. doi:10.1111/iwj.13927
Lin, D., Zhang, J., Bai, F., Cao, X., Fan, C., Yuan, Y., et al. (2016). Fabrication and clinical application of easy-to-operate pre-cured CPC/rhBMP-2 micro-scaffolds for bone regeneration. Am. J. Transl. Res. 8 (3), 1379–1396.
Litvinov, R., and Weisel, J. (2016). What is the biological and clinical relevance of fibrin? Semin. Thromb. Hemost. 42 (04), 333–343. doi:10.1055/s-0036-1571342
Liu, Y., Lin, D., Li, B., Hong, H., Jiang, C., Yuan, Y., et al. (2022). BMP-2/CPC scaffold with dexamethasone-loaded blood clot embedment accelerates clinical bone regeneration. Am. J. Transl. Res. 14 (5), 2874–2893.
Lobo, S. E., and Livingston Arinzeh, T. (2010). Biphasic calcium phosphate ceramics for bone regeneration and tissue engineering applications. Materials 3 (2), 815–826. doi:10.3390/ma3020815
Luvsannyam, E., Jain, M. S., Leitao, A. R., Maikawa, N., and Leitao, A. E. (2022). Meniscus tear: Pathology, incidence, and management. San Francisco, CA: Cureus.
Mervis, J. S., and Phillips, T. J. (2019). Pressure ulcers: Pathophysiology, epidemiology, risk factors, and presentation. J. Am. Acad. Dermatol 81 (4), 881–890. doi:10.1016/j.jaad.2018.12.069
Park, C. H., and Woo, K. M. (2018). Fibrin-based biomaterial applications in tissue engineering and regenerative medicine. Adv. Exp. Med. Biol. 1064, 253–261. doi:10.1007/978-981-13-0445-3_16
Ra, H. J., Ha, J. K., Jang, S. H., Lee, D. W., and Kim, J. G. (2013). Arthroscopic inside-out repair of complete radial tears of the meniscus with a fibrin clot. Knee Surg. Sports Traumatol. Arthrosc. 21 (9), 2126–2130. doi:10.1007/s00167-012-2191-3
Rieu, C., Mosser, G., Haye, B., Sanson, N., Coradin, T., and Trichet, L. (2020). Thrombin-free polymerization leads to pure fibrin(ogen) materials with extended processing capacity. bioRxiv [Internet] Available at: http://biorxiv.org/content/early/2020/05/14/2020.05.12.091793.abstract.
Roessler, F. C., Ohlrich, M., Marxsen, J. H., Stellmacher, F., Sprenger, A., Dempfle, C. E., et al. (2011). The platelet-rich plasma clot. Blood Coagulation Fibrinolysis 22 (5), 407–415. doi:10.1097/mbc.0b013e3283468a60
Schizas, N., Savvidou, O., Triantafyllopoulos, I., Papadakis, S., Dontas, I., and Papagelopoulos, P. (2019). Adjuvant therapies for the enhancement of microfracture technique in cartilage repair. Orthop. Rev. (Pavia) 11 (3), 7950.doi:10.4081/or.2019.7950
Singh, R., Rohilla, R. K., Dhayal, R. K., Sen, R., and Sehgal, P. K. (2014). Role of local application of autologous platelet-rich plasma in the management of pressure ulcers in spinal cord injury patients. Spinal Cord. 52 (11), 809–816.doi:10.1038/sc.2014.144
Snyder, R., Driver, V., Cole, W., Joseph, W., Reyzelman, A., Lantis, J., et al. (2023). Topical autologous blood clot therapy: Consensus panel recommendations to guide use in the treatment of complex wound types. Wounds 35 (1), 2–8. doi:10.25270/wnds/22061
Snyder, R. J., Schultz, G., Wachuku, C., Rashid, A. M., and Ead, J. K. K. (2020). Proposed mechanism of action of topically applied autologous blood clot tissue: A quintessential cellular and tissue based therapy. J. Am. Podiatr. Med. Assoc, 20-140. doi:10.7547/20-140
Snyder, R. J. (2005). Treatment of nonhealing ulcers with allografts. Clin. Dermatol 23 (4), 388–395. doi:10.1016/j.clindermatol.2004.07.020
Steadman, J. R., Rodkey, W. G., and Briggs, K. K. (2010). Microfracture. Cartil. 1 (2), 78–86. doi:10.1177/1947603510365533
Sugerman, G. P., Parekh, S. H., and Rausch, M. K. (2020). Nonlinear, dissipative phenomena in whole blood clot mechanics. Soft Matter 16 (43), 9908–9916. doi:10.1039/d0sm01317j
Torbet, J., Freyssinet, J. M., and Hudry-Clergeon, G. (1981). Oriented fibrin gels formed by polymerization in strong magnetic fields. Nature 289 (5793), 91–93. doi:10.1038/289091a0
Tran, R., Myers, D. R., Ciciliano, J., Trybus Hardy, E. L., Sakurai, Y., Ahn, B., et al. (2013). Biomechanics of haemostasis and thrombosis in health and disease: From the macro- to molecular scale. J. Cell Mol. Med. 17 (5), 579–596. doi:10.1111/jcmm.12041
Undas, A., and Ariëns, R. A. S. (2011). Fibrin clot structure and function: A role in the pathophysiology of arterial and venous thromboembolic diseases. Arteriosclerosis, Thrombosis, Vasc. Biol. 31.doi:10.1161/ATVBAHA.111.230631
VanZweden, E., Tolsma, R., Hung, V., Awad, P., Sawyer, R., and Li, Y. (2022). The advances of blood clots used as biomaterials in regenerative medicine. Regen. Med. 17 (12), 957–969. doi:10.2217/rme-2022-0035
Varin, R., Mirshahi, S., Mirshahi, P., Klein, C., Jamshedov, J., Chidiac, J., et al. (2013). Whole blood clots are more resistant to lysis than plasma clots - greater efficacy of rivaroxaban. Thromb. Res. 131 (3), e100–e109. doi:10.1016/j.thromres.2012.11.029
Weisel, J. W. (2010). Biomechanics in hemostasis and thrombosis. J. Thrombosis Haemostasis 8 (5), 1027–1029. doi:10.1111/j.1538-7836.2010.03808.x
Weisel, J. W. (1986). Fibrin assembly. Lateral aggregation and the role of the two pairs of fibrinopeptides. Biophys. J. 50 (6), 1079–1093. doi:10.1016/s0006-3495(86)83552-4
Weisel, J. W., and Nagaswami, C. (1992). Computer modeling of fibrin polymerization kinetics correlated with electron microscope and turbidity observations: Clot structure and assembly are kinetically controlled. Biophys. J. 63 (1), 111–128. doi:10.1016/s0006-3495(92)81594-1
Weisel, J. W. (2004). The mechanical properties of fibrin for basic scientists and clinicians. Biophys. Chem. 112 (2-3), 267–276. doi:10.1016/j.bpc.2004.07.029
Wu, D. T., Jeffreys, N., Diba, M., and Mooney, D. J. (2022). Viscoelastic biomaterials for tissue regeneration. Tissue Eng. Part C Methods 28 (7), 289–300. doi:10.1089/ten.tec.2022.0040
Yamagishi, A., Takeuchi, T., Higashi, T., and Date, M. (1990). Magnetic field effect on the polymerization of fibrin fibers. Phys. B Condens Matter 164 (1–2), 222–228. doi:10.1016/0921-4526(90)90080-e
Keywords: tissue injury, wound healing, blood clots, regeneration, biomaterial
Citation: Richter DM, Ku JC, Keckler KE, Burke LR, Abd GM and Li Y (2023) Autologous blood clots: a natural biomaterial for wound healing. Front. Mater. 10:1250013. doi: 10.3389/fmats.2023.1250013
Received: 29 June 2023; Accepted: 11 July 2023;
Published: 25 July 2023.
Edited by:
Chengchen Guo, Westlake University, ChinaCopyright © 2023 Richter, Ku, Keckler, Burke, Abd and Li. This is an open-access article distributed under the terms of the Creative Commons Attribution License (CC BY). The use, distribution or reproduction in other forums is permitted, provided the original author(s) and the copyright owner(s) are credited and that the original publication in this journal is cited, in accordance with accepted academic practice. No use, distribution or reproduction is permitted which does not comply with these terms.
*Correspondence: Yong Li, eW9uZy5saUB3bWVkLmVkdQ==