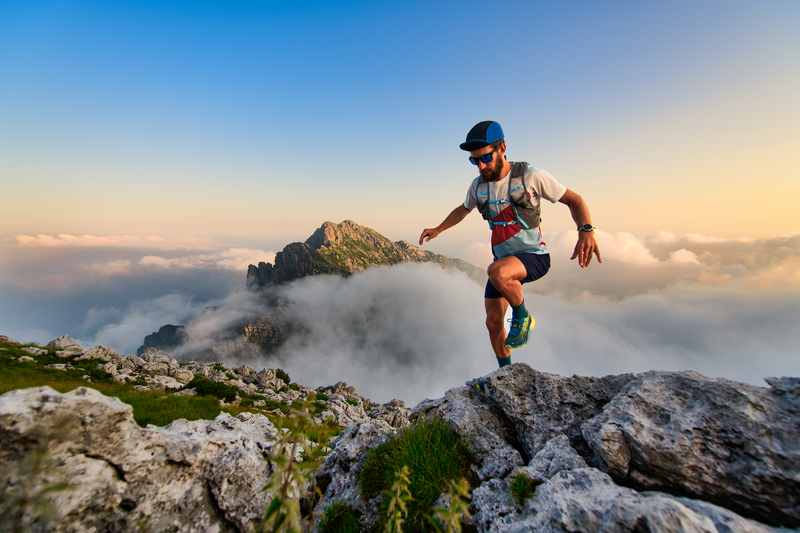
95% of researchers rate our articles as excellent or good
Learn more about the work of our research integrity team to safeguard the quality of each article we publish.
Find out more
ORIGINAL RESEARCH article
Front. Mater. , 25 July 2023
Sec. Biomaterials and Bio-Inspired Materials
Volume 10 - 2023 | https://doi.org/10.3389/fmats.2023.1233136
Biomaterials will have far reaching effects on solving the ever-rising environmental problems caused by the presently used toxic materials. Disposal of hazardous materials based electronic devices is causing a huge environmental problem in the form of electronic wastes (E-wastes). This study is an effort to contribute in the reduction of E-wastes by presenting the design, fabrication, and performance evaluation of a fully biocompatible humidity sensor with gelatin as the active layer. Gelatin is an excellent choice for humidity sensing because it is a hygroscopic material with the natural ability to attract and absorb water molecules, allowing for an effective detection and measurement of humidity levels in its surrounding environment. We patterned highly conductive (2.05 μΩ-cm) gold (Au) interdigitated electrode (IDE) (50 nm thickness) structure on a glass substrate by using thermal vacuum evaporation followed by the deposition of gelatin thin film by using cost effective technique of spin coating (at 1,500 rpm for 15 s). The device size was 25 mm × 22 mm with a line spacing of 1.50 mm between IDEs. Electrical characterisation was carried out to determine necessary performance parameters of the fully biocompatible humidity sensor and the obtained results exhibited a fast response/recovery time (4/6.30 s), wide operating range (15 %RH to 86 %RH), highly repeatable behaviour of 3 different samples and long lifetime (1 month). Wide variation in the resistance of sensor was observed at extreme humidity sensors ranging from 2.45 MΩ (15 %RH) to 0.12 MΩ (86 %RH). The fabricated sensor was used for the practical applications of health monitoring, touch less sensing, and environment monitoring. Moisture content of multiple commercially available moisturizers was determined by using gelatin based fully biocompatible humidity sensor and the obtained results showed that moisturizing cream had highest moisture content of 87 %RH (0.1 MΩ), followed by 80 %RH (0.30 MΩ) of rose water, and 72 %RH (0.67 MΩ) of baby oil.
Humidity sensing plays a crucial role in various fields and applications due to its significant impact on human comfort, health, and the environment. Understanding and monitoring humidity levels is essential in a wide range of industries, including meteorology, agriculture, HVAC (heating, ventilation, and air Conditioning) systems, food processing, pharmaceuticals, and materials science. In meteorology and climate studies, accurate humidity measurements are vital for weather forecasting, climate modelling, and understanding atmospheric phenomena. In agriculture, humidity sensing helps optimize crop irrigation and disease control. In the field of HVAC, humidity sensing is essential for maintaining indoor air quality and comfort. In the food and pharmaceutical industries, humidity sensors play a critical role in ensuring product quality and safety. In pharmaceutical manufacturing, humidity monitoring is essential for preserving the stability and efficacy of medications. Materials science and engineering rely on humidity sensing to evaluate the moisture absorption properties of various materials, such as polymers, coatings, and composites. Understanding how materials interact with moisture is crucial for product development, quality control, and durability assessments. Advances in humidity sensing technology have the potential to enhance various industries, contribute to sustainable practices, and improve the wellbeing of individuals in different environments. Existing humidity sensing technologies encompass a range of principles and techniques that offer diverse approaches to measuring and monitoring humidity levels. These technologies can be broadly categorized into two main types: 1) relative humidity (RH) sensors (Yang et al., 2022) and 2) absolute humidity (AH) sensors (He et al., 2021). RH sensors can be further classified into (a) Capacitive RH Sensors and (b) Resistive RH Sensors while (c) Thermal RH Sensors (d) Gravimetric RH Sensors. Among all these type of sensors, resistive sensors are cost-effective and suitable for low-cost applications. Resistive RH sensors use a moisture-sensitive material, typically a polymer or ceramic, to alter the electrical resistance based on humidity-induced changes in material conductivity and measure resistance changes to determine RH levels.
In recent years, emerging materials such as graphene-based sensors (Khan et al., 2021) (Khan et al., 2021; Saqib et al., 2021; Rahman et al., 2022), nanomaterial-based sensors (Saqib et al., 2021), and flexible sensors (Rahman et al., 2022) have shown promise in humidity sensing, offering improved sensitivity, miniaturization, and integration capabilities. However, serious problems have been reported off late due to electronic wastes (E-wastes) caused by using non-environment friendly materials therefore use of biocompatible materials is inevitable for sustainable future of electronics (Rehman et al., 2020; Khan et al., 2022). The selection of an appropriate sensing material is crucial for the accurate and reliable detection of humidity levels. The choice of the humidity-sensitive material is critical for the sensing performance of IDE-based humidity sensors. The sensing material should exhibit a high sensitivity to changes in humidity, low hysteresis, and fast response time. In the case of resistive humidity sensors, the sensing material should have high electrical conductivity and be responsive to changes in moisture content. Biomaterials with appropriate characteristics can play a vital role in the sustainable future of electronics without causing any burden on environment (Mutee Ur Rehman et al., 2021; Rehman et al., 2021; 2023; Mutee ur Rehman et al., 2022). In this regard, gelatin, derived from collagen, has gained attention as a potential sensing due to its unique properties (Liu et al., 2019; Pereira et al., 2020; Yang et al., 2020; Afnas et al., 2022; Zhong et al., 2022). Gelatin is a protein-based biomaterial derived from collagen, primarily obtained from animal sources such as bovine or porcine skin and bones. It has been widely used in various industries due to its unique properties, including its biocompatibility, biodegradability, and gel-forming ability. Gelatin has found applications in food, pharmaceuticals, cosmetics, and biomedical fields. In recent years, researchers have explored the potential of gelatin for humidity sensing applications due to its moisture-sensitive characteristics and ease of processing. Properties of Gelatin relevant to humidity sensing include (a) Hygroscopic nature due to its high affinity for water absorption leading to changes in its physical and chemical properties. (b) Swelling Behaviour: Gelatin can undergo significant swelling or shrinkage in response to changes in humidity levels. By absorbing/desorbing moisture. (c) Electrical Conductivity: Gelatin-based sensors can exploit the changes in electrical conductivity that occur with variations in humidity leading to the idea of fabricating resistive type humidity sensor. (d) Mechanical Flexibility: Gelatin possesses excellent mechanical flexibility and can be easily formed into various shapes, including films, fibres, or coatings that can further be used as wearable electronics (e) Biocompatibility: Gelatin is biocompatible, meaning it is non-toxic and compatible with living tissues. This property is advantageous for applications where the sensor may meet biological systems, such as biomedical devices or human-centric applications. (f) Processing Versatility: gelatin can be processed using various techniques, including casting, electrospinning, and inkjet printing, allowing for the fabrication of humidity sensors with different geometries and structures.
In this study we have spin coated the thin film of gelatin as the active layer of a humidity sensor on the surface of sputtered Au IDEs to form a fully biocompatible humidity sensor that exhibited interesting results with variations in relative humidity. The utilization of gelatin in humidity sensing opens possibilities for applications in environmental monitoring, health monitoring, food storage, touchless sensing to avoid the spread of chronic diseases and above all promoting green solution to increasing problems caused by E-wastes (Chakraborty et al., 2022; Roy et al., 2022).
Gelatin ink was purchased from Sigma Aldrich that was derived from lime-cured tissue bovine skin and was in the form of a 2% solution in water. It was used as it is without any further processing and its thin film was spin coated at a speed of 1,500 rpm for 15 s because spin coating is a widely used technique for the deposition of thin films or coatings with the advantages of precise control over film thickness owing to control over rotation speed, uniformity, and ease of operation. 99.99% pure Au powder (2.05 μΩ-cm) was purchased from Sigma Aldrich for the patterning of Au IDEs to attain high conductivity with the help of a customized mask.
The glass substrate was carefully cleaned using a series of cleaning agents, namely, ethanol, acetone, and deionized water. This cleaning process aimed to eliminate any dust particles and contaminants present on the surface of the substrate. After cleaning, the substrate underwent a treatment of UV ozone for a duration of 10 min. This treatment enhanced the adhesion of the substrate’s surface and increased its hydrophilicity. For sensing humidity, we chose to pattern the planar structure of Interdigitated Electrodes (IDEs) based on the anticipation that IDEs would provide greater sensitivity to changes in the characteristics of the biopolymer-based functional layer in response to variations in relative humidity (RH%). IDEs can be understood as two chemristors connected in parallel. To fabricate the IDEs, we utilized state-of-the-art thermal vacuum evaporation technology, as it offered excellent control and efficient results. To enhance the adhesion of the Au IDEs to the surface of the glass substrate, a thin layer of chromium (10 nm) was deposited prior to the Au IDEs. The thickness of the Au IDEs was 50 nm. The deposition rates for the chromium layer and Au IDEs were maintained at 1 A/sec and 2 A/sec, respectively. The spacing and width of each IDE finger plays a crucial role in determining the performance of the humidity sensor. Hence, we optimized these dimensions and customized a mask whose detailed labelling is presented in Figure 1A. The dimensions chosen for the IDEs were the smallest achievable using our proposed fabrication technology. This selection aimed to highlight the device’s potential for integration into CMOS technology. To complete the fabrication process of the biopolymer-based humidity sensor, a diluted ink solution of the biopolymer, gelatin, was deposited on Au IDEs by using the widely used technique of spin-coating (Kashif et al., 2013) at a speed of 1,500 rpm for 15 s. The optical image of our fabricated device is shown in the inset of Figure 1B.
FIGURE 1. (A) Detailed labeling of mask used for patterning IDEs (B) Resistance variation of gelatin-based humidity sensor with change in %RH. Inset shows the optical image of fabricated sensor.
Biomaterial-based humidity sensors require appropriate substrate materials and electrode materials to ensure their high performance, reliability, and compatibility with the sensing mechanism. The selection of the substrate and electrode materials is crucial to achieve good sensor sensitivity, mechanical stability, repeatability, and compatibility with the gelatin sensing layer therefore, transparent glass was used as a substrate for fabricating fully biocompatible and mechanically stable humidity sensor. The chemical inertness of glass substrates prevents unwanted interactions between the substrate and the gelatin layer, preserving the integrity of the sensing mechanism (Li H. et al., 2022). Gold (Au) was selected as the electrode material as it is a widely used electrode material in humidity sensors due to its excellent electrical conductivity, corrosion resistance, and biocompatibility. The choice of gold electrodes for gelatin-based humidity sensors offered several advantages (Yan et al., 2022) like (a) high electrical conductivity enabling efficient charge transfer between the sensing layer and the external circuitry to promote accurate and reliable measurements of humidity changes by minimizing electrical losses and signal distortions. (b) Au is highly resistant to oxidation and corrosion, ensuring the stability and longevity of the electrode performance. This resistance is particularly important in humidity sensing applications, where the electrodes are exposed to moisture and potentially corrosive environments. Gold electrodes maintain their conductivity and integrity over time, contributing to the sensor’s long-term reliability. (c) Gold is biocompatible and non-toxic, making it suitable for applications involving contact with biological systems or living tissues. In biomedical or wearable humidity sensors, gold electrodes ensure compatibility with the human body, minimizing any adverse effects or allergic reactions. Interdigitated electrode (IDE) design was preferred over other electrode structures due to their simplicity, sensitivity, and low cost (Li M. et al., 2022). The geometry of the IDEs played a crucial role in the sensing performance of our humidity sensors. The spacing and width of the electrode fingers determine the effective sensing area and the sensitivity of the sensor. Smaller finger spacing (0.25 mm) enhanced sensitivity as it increases the interaction between the humidity-sensitive layer and the electrodes, resulting in a larger change in resistance.
Thin film of gelatin was characterized to find its structural, morphological, and electrical characteristics. These characterizations also helped in determining the conduction mechanism. The dimensions of the patterned Au IDEs were determined using an optical microscope manufactured by Olympus (model BX60, Tokyo, Japan). The FESEM image of the active layer for the morphological studies of its surface was captured using a scanning electron microscope (TESCON MIRA 3).To investigate the chemical composition and structure of the gelatin biopolymer, Fourier transform infrared (FTIR) spectroscopy was employed. The FTIR analysis was conducted using an ALPHA II FTIR spectrometer. Raman spectroscopy was also carried out utilizing the LABRAM HR EV Micro Raman Spectrometer System (Horiba, Kyoto, Japan). To record the changes in resistance response corresponding to variations in humidity, a standard technique was employed. This technique involved the use of airtight jars saturated with different salt solutions. By exposing the humidity sensor to these controlled environments, the corresponding changes in resistance values were measured and recorded.
The surface of gelatin thin film was examined by using FESEM image as shown in Figure 2A. Obtained results exhibited that the surface of thin film was compact and uniform, and having no prominent surface defects, which showed the robust adhesion of gelatin with the glass substate. The FESEM image also shows presence of a few cracks that can enhance the surface area and ease the ingress/egress of moisture.
FIGURE 2. Characterization results of gelatin thin film (A) Surface morphology through FESEM image (B) FTIR characterization (C) Raman spectrum.
In the analysis of gelatin using Fourier-transform infrared spectroscopy (FTIR), specific peaks or wavenumbers were observed, indicating different regions of the gelatin’s molecular structure as shown in Figure 2B. These peaks include wavenumbers at 3,500–2,300 cm−1 (Amide A), 1,656–1,644 cm−1 (Amide I), 1,560–1,335 cm−1 (Amide II), and 1,240–670 cm−1 (Amide III). Various studies have reported similar results, noting the presence of vibrations related to the stretching of the C-N bond in these regions. Among these peaks, the Amide I band between 1,600 and 1700 cm−1 is particularly valuable for analysing the secondary protein structures using FTIR. The Amide II peak arises from the bending vibration of NH and the stretching vibration of C-N bonds. The Amide III peak represents vibrations within the plane of the C-N and N-H groups of bound amides or the vibration of the CH2 group. The absorption at around 2,900 cm−1 was attributed to the vibration of the C-H group in the protein, which is commonly found in various organic compounds. Thus, this peak cannot be used to differentiate between porcine and bovine gelatins since both types contain C-H groups. The lower intensity of this peak in may be due to the presence of free water, which overlaps with the absorption spectrum of C-H, thereby reducing its intensity. On the other hand, the FTIR spectrum around 700 cm−1 was in the fingerprint region, where small changes in the sample environment can affect the absorption spectrum.
Raman spectroscopy is a vibrational molecular spectroscopy technique that involves inelastic light scattering. In the case of gelatin, Raman spectroscopy was used to identify their functional groups, as shown in Figure 2C. The Raman spectra of gelatin exhibit characteristic peaks related to specific molecular vibrations. These include weak peaks for O-H and N-H stretching around 3,200–3,600 cm−1, with a centre at 3,250 cm−1. Intense peaks for C-H stretching are observed around 2,800–3,000 cm−1, centred at 2,950 cm−1. A weak peak for C=O stretching (amide I) is observed at 1,665 cm−1, and a weak peak for NH bending is observed at 1,613 cm−1. The C-C vibration of tyrosine is represented by peaks at 1,615–1,618 cm−1. Strong peaks for CH stretching and scissoring (methyl (CH3) and methylene (CH2)) are observed around 1,420–1,470 cm−1, centred at 1,450 cm−1. There are also strong peaks for CH wagging at 1,335–1,343 cm−1 and CH twisting at 1,300–1,315 cm−1. Other identified peaks in the Raman spectra include amide III (C-N stretching) at 1,223 cm−1, C-H bending at 1,173 cm−1, C-N stretching in proline at 1,127–1,150 cm−1, C-C skeletal vibrations in the backbone at 1,064–1,080 cm−1, symmetric ring breathing of phenylalanine at 1,003–1,030 cm−1, C-H in-plane bending at 1,031 cm−1, phenylalanine at 620 cm−1, and N-H bending at 599 cm−1. Additionally, a weak peak at around 1,640 cm−1 is correlated with water (H2O).
The conduction mechanism of a resistive-type gelatin-based humidity sensor relies on the alterations in electrical resistance resulting from the absorption or desorption of moisture by the gelatin material. These sensors use impedance measurement principles, where changes in humidity levels cause variations in the sensor’s resistance, which can be correlated with the corresponding humidity value. As humidity increases, the gelatin material absorbs moisture from the environment. This causes gelatin to swell as water molecules interact with its polymer structure. The swelling leads to an increase in intermolecular spacing within the gelatin, allowing for ionic conduction. The increased intermolecular spacing alters the electrical resistance of gelatin. The conductivity of the gelatin depends on the mobility of ions within its matrix. The presence of moisture enhances ionic mobility, resulting in a decrease in resistance. When humidity decreases, gelatin releases moisture back into the environment. The evaporation of water molecules causes the gelatin matrix to contract, reducing the intermolecular spacing. This contraction restricts ion movement, leading to an increase in electrical resistance. By monitoring the change in electrical resistance, humidity levels can be determined using calibration curves or mathematical models derived from experimental data. The resistance of the gelatin-based sensor serves as a direct indicator of the moisture content in the surrounding environment.
Electrical response of gelatin-based humidity sensor was evaluated with respect to changes in relative humidity. The humidity sensor experiment involved testing various salts’ ability to control humidity levels. The standard technique of using supersaturated solutions salts contained in airtight jars was used to determine electrical response of fabricated sensor with respect to variations in %RH with an operating range of 15–86 %RH. These salts included lithium chloride (LiCl-15%), potassium acetate (CH3COOK-23%), calcium chloride (CaCl2-32%), potassium carbonate (K2CO3-46%), sodium bromide (NaBr-60%), copper chloride (CuCl2-67%), sodium chloride (NaCl-72%), potassium chloride (KCl-80%), and potassium sulphate (K2SO4-86%). Commercially available HTU21D was used as a reference sensor inside those jars and Arduino board was used for the calibration of %RH inside airtight jars of super saturated salt solutions. LCR meter (KEYSIGHT 2911A) was used to precisely determine the variations in resistance of fabricated sensor with different levels of relative humidity. Figure 1B shows the adsorption/desorption graph of gelatin based biocompatible humidity sensor in the operating range of 15–86 %RH. The graph clearly shows the variation of resistance with change in %RH hence signifying the use of gelatin as the biocompatible humidity sensitive material. At low humidity level (15 %RH), resistance was higher (2.45 MΩ) but with increase in %RH, the resistance kept decreasing with the minimum resistance recorded as 120 kΩ at 86 %RH. Adsorption of water molecules forms conductive channels for charge carriers to flow within the active layer of gelatin hence, resulting in an increase of electrical conductivity. Gelatin, being a hydrophilic biomaterial, can absorb moisture from the environment. When gelatin absorbs water molecules, it swells and forms a gel-like structure. Within this gel, water molecules dissociate into ions, such as hydrogen ions (H+) and hydroxide ions (OH−). The presence of these ions enables the conduction of electricity within the gelatin material. Gelatin contains a significant number of functional groups, such as amine and carboxyl groups, which can facilitate protonic conduction. Water molecules absorbed by the gelatin can interact with these functional groups, resulting in the transfer of protons (H+) through the gelatin matrix. This protonic conduction contributes to the electrical response of the humidity sensor. In addition to ion and proton conduction, the gelatin-based humidity sensor may exhibit electron conduction. This can occur through the formation of conductive pathways within the gelatin matrix. For example, the presence of metallic nanoparticles or conductive additives within the gelatin structure can facilitate electron transfer and electrical conduction. We prepared 3 different samples by using same fabrication technique and evaluated their electrical response towards changes in %RH to check repeatability in their response and highly repeatable results were obtained as shown in Figure 3A.
FIGURE 3. (A) Highly repeatable response of 3 different sensors in the operating range of 15–86 %RH. (B) Stability of gelatin-based humidity sensor over a period of 30 days.
Biomaterials have the drawback of stability over longer period and gelatin, being a hydrophilic material, can absorb moisture from the environment. This absorption can lead to changes in the physical and electrical properties of the gelatin, potentially affecting the sensor’s stability. Therefore, we checked the response of our biocompatible sensor over a period of 1 month and the obtained response was highly stable as shown in Figure 3B. Our sensor exhibited its ability to maintain consistent and reliable performance over time.
The response time and recovery time of a humidity sensor refer to how quickly the sensor can detect changes in humidity and return to its initial state after exposure to a different humidity level. The specific response time and recovery time of a humidity sensor can vary depending on the sensor technology and design. The response time of a humidity sensor is the time it takes for the sensor to detect and indicate a change in humidity after being exposed to a new environment. It is influenced by factors such as the sensing material, sensor construction, and the method used to measure humidity. The recovery time of a humidity sensor refers to the time it takes for the sensor to return to its initial state after being exposed to a different humidity level. It is influenced by factors such as the sensing material’s ability to release or absorb moisture and the design of the sensor. We evaluated response time and recovery time of our fabricated sensor through its dynamic response. Obtained results showed that a fast response time (4 s) and fast recovery time (6.3 s) can be achieved by using gelatin as the active layer as shown in Figure 4A. We also checked the response of our sensor towards mouth breathing to evaluate the change of its resistance with exhaling (730 kΩ) and inhaling (2.1 MΩ) air as shown in Figure 4B. A mouth breathing test serves as a valuable evaluation method for a humidity sensor, providing insights into its specific capabilities and potential applications. Mouth breathing is a common breathing pattern, especially during physical exertion or when nasal congestion occurs. The mouth breathing test allows for the examination of the sensor’s stability and robustness in a dynamic environment. This characterization result ensures the significance of our sensor in health/respiratory monitoring. Comparison of biomaterial-based humidity sensors is shown in Table 1.
FIGURE 4. (A) Reaction time of gelatin-based humidity sensor with a fast response/recovery time (B) Mouth breathing test of fabricated sensor.
The humidity response of a sensor can vary depending on the breathing rate, particularly between fast and slow breathing. It's important to note that individual variations in breathing patterns and physiology can also influence the specific humidity response. Factors such as lung capacity, respiratory conditions, or environmental conditions can further impact the moisture content and dynamics of exhaled breath. We studied the response of our sensor toward slow and fast breathing rates. The obtained results showed a clear distinction between both breathing rates as shown in Figure 5A. The results confirm that during slow breathing, the exhaled air tends to have a higher moisture content due to the longer exhalation duration and increased airflow. This higher moisture content can result in a more significant change in humidity levels (50–70 %RH). Fast breathing involves smaller exhalation durations and reduced airflow. As a result, the moisture content in exhaled breath during fast breathing may be lower compared to fast breathing. This lower moisture content can lead to relatively smaller changes in humidity levels. Consequently, a humidity sensor may exhibit slower response times and smaller fluctuations in humidity readings during slow breathing. The sensor’s sensitivity to humidity changes may be less prominent due to the reduced moisture content in the exhaled breath (60–65 %RH). Secondly, we tested the response of our biocompatible humidity sensor towards different commercially available moisturizers including baby oil, moisturizing cream, and rose water to determine the content of moisture in them whose results are presented in Figure 5B. The response of a humidity sensor towards commercially available moisturizers can vary depending on several factors, including the specific formulation of the moisturizer and the sensing mechanism of the humidity sensor. Moisturizers, particularly those with higher water or humectant content, can release moisture into the air when applied to the skin. This increased humidity may be detected by the sensor, leading to an elevated humidity reading. Moisturizers typically contain water or ingredients that attract and retain moisture. When applied to the skin, these moisturizers can release moisture into the air, leading to an increase in humidity levels. The humidity sensor may detect this increased moisture and register a higher humidity reading. From the obtained results it was verified that the moisture content was in the order of baby oil (87 %RH), moisturizing cream (80 %RH), rose water (72 %RH), and wet skin (62 %RH). Moisturizing creams typically contain a combination of water, oils, humectants, emollients, and other ingredients. The moisture content of a moisturizing cream can vary depending on its formulation. However, in general, moisturizing creams tend to have a higher water content compared to oil-based products. The presence of water or water-based ingredients allows moisturizing creams to provide hydration to the skin. The water content helps to replenish moisture and improve skin hydration levels. Rose water is a product derived from the distillation of rose petals. It is primarily composed of water with trace amounts of essential oils and rose extract. Rose water is known for its soothing and hydrating properties. While rose water does contain water, its moisture content may be lower compared to moisturizing creams. However, it still provides some level of hydration and can help maintain the skin’s moisture balance. Baby oil is a mineral oil-based product that is typically used for moisturizing and protecting the skin. Unlike moisturizing creams and rose water, baby oil does not contain water. It is purely composed of mineral oil or other types of oils. As a result, the moisture content of baby oil is significantly lower compared to moisturizing creams and rose water. Baby oil primarily acts as an emollient, creating a barrier on the skin’s surface to prevent moisture loss rather than adding moisture. Thirdly, we tested our humidity sensor for the application of touchless sensing by keeping finger at 3 different distances (2, 5, and 10 cm) as shown in Figure 5C. Resistive type humidity sensors typically rely on a direct contact between the sensing element and the moisture source, making them primarily contact-based sensors. However, it is possible to achieve a form of non-contact sensing. When the finger was brought close to the sensor without touching it, the moisture content in the surrounding air influenced the resistance of the sensing element. The presence of the finger alters the local humidity in the vicinity of the sensor. Finally, we tested our gelatin-based humidity sensor to measure the real time humidity of the laboratory environment. Obtained results clearly showed that as the day passed by from morning to night, humidity increased and as a result resistance of our sensor kept decreasing as shown in Figure 6.
FIGURE 5. Practical applications of gelatin-based humidity sensor (A) Breathing rate evaluation by monitoring the difference between fast and slow breathing (B) Evaluation of moisture content in multiple commercially available moisturizers (C) Touchless sensing response of gelatin-based humidity sensor at variable distances.
In this study, we fabricated a fully biocompatible gelatin-based humidity sensor that exhibited reliable and sensitive response towards changes in humidity levels. The sensor was fabricated using a glass substrate with a patterned interdigitated electrode (IDE) structure made of highly conductive gold (Au). A highly uniform and compact thin film of gelatin was deposited on the IDE structure using a cost-effective spin coating technique. The fabricated biocompatible humidity sensor demonstrated excellent performance, including fast response/recovery times (4/6.3 s), a wide operating range (15–90 %RH), high stability, and repeatability (3 samples) over a long period of time (1 month). The electrical characterization of the sensor revealed a significant variation in resistance at extreme humidity levels, ranging from 2.45 MΩ at 15% relative humidity (RH) to 0.12 MΩ at 86% RH. The sensor was successfully applied in practical scenarios such as health monitoring (fast and slow breathing), touchless sensing (2, 5, and 10 cm), and environmental monitoring (12 h on a rainy day). Additionally, the moisture content of commercially available moisturizers was evaluated using the gelatin-based humidity sensor, showing varying levels of moisture content in different products including moisturizing cream, baby oil, and rose water. Overall, the gelatin-based fully biocompatible humidity sensor demonstrated promising performance characteristics and practical applications in moisture detection. It offers potential for addressing environmental concerns associated with toxic materials while providing reliable and versatile humidity sensing capabilities. Following on the footsteps of this study, more biomaterials can be explored for their potential use in electronics that will certainly reduce burden on our environment caused by E-wastes. Furthermore, the performance of gelatin-based humidity sensor can be further enhanced by exploring its composites with other biomaterials. Such sensors can be used in biomedical applications like implants due to their biocompatibility.
The original contributions presented in the study are included in the article/Supplementary material, further inquiries can be directed to the corresponding authors.
Conception and design of study: MK and MR. Acquisition of data: MK, MR, SK, and MS. Analysis and/or interpretation of data: MR, SK, and WK. Drafting the manuscript: MK and MR. Revising the manuscript critically for important intellectual content: MK, MR, and WK. Approval of the version of the manuscript to be published: MK, MR, SK, MS, and WK. All authors contributed to the article and approved the submitted version.
This research was funded by [National Research Foundation of Korea (NRF)- Korea Government (Ministry of Science and ICT)] grant number (NRF- 2020H1D3A1A04081545, 2021R1A4A2000934, 2021R1F1A106 2800). This research has also been supported as Project Open Innovation R&D (21-DC-004) and supported by K-water.
The authors declare that the research was conducted in the absence of any commercial or financial relationships that could be construed as a potential conflict of interest.
All claims expressed in this article are solely those of the authors and do not necessarily represent those of their affiliated organizations, or those of the publisher, the editors and the reviewers. Any product that may be evaluated in this article, or claim that may be made by its manufacturer, is not guaranteed or endorsed by the publisher.
Afnas, V. M., Unnikrishnan, G., Budhe, S., Manaf, O., and Ameen, J. (2022). PVA/gelatin/chitin ternary blend as a humidity sensing material. J. Mater. Sci. Mater. Electron. 33, 2031–2043. doi:10.1007/s10854-021-07406-z
Barmpakos, D., Segkos, A., Tsamis, C., and Kaltsas, G. (2017). A disposable flexible humidity sensor directly printed on paper for medical applications. J. Phys. Conf. Ser. 931, 012003. doi:10.1088/1742-6596/931/1/012003
Chakraborty, M., Kettle, J., and Dahiya, R. (2022). Electronic waste reduction through devices and printed circuit boards designed for circularity. IEEE J. Flex. Electron. 1, 4–23. doi:10.1109/JFLEX.2022.3159258
Duan, Z., Jiang, Y., Yan, M., Wang, S., Yuan, Z., Zhao, Q., et al. (2019). Facile, flexible, cost-saving, and environment-friendly paper-based humidity sensor for multifunctional applications. ACS Appl. Mater. Interfaces 11, 21840–21849. doi:10.1021/acsami.9b05709
He, C., Korposh, S., Correia, R., Liu, L., Hayes-Gill, B. R., and Morgan, S. P. (2021). Optical fibre sensor for simultaneous temperature and relative humidity measurement: Towards absolute humidity evaluation. Sensors Actuators B Chem. 344, 130154. doi:10.1016/j.snb.2021.130154
Kashif, M., Ali, M. E., Ali, S. M. U., and Hashim, U. (2013). Sol–gel synthesis of Pd doped ZnO nanorods for room temperature hydrogen sensing applications. Ceram. Int. 39, 6461–6466. doi:10.1016/j.ceramint.2013.01.075
Khan, M., Mutee Ur Rehman, H. M., Tehreem, R., Saqib, M., Rehman, M. M., and Kim, W.-Y. (2022). All-Printed flexible memristor with metal–non-metal-doped TiO2 nanoparticle thin films. Nanomaterials 12, 2289. doi:10.3390/nano12132289
Khan, S. A., Saqib, M., Rehman, M. M., Mutee Ur Rehman, H. M., Rahman, S. A., Yang, Y., et al. (2021). A full-range flexible and printed humidity sensor based on a solution-processed P(VDF-TrFE)/Graphene-Flower composite. Nanomaterials 11, 1915. doi:10.3390/nano11081915
Li, H., Li, Z., Li, N., Zhu, X., Zhang, Y., Sun, L., et al. (2022a). 3D printed high performance silver mesh for transparent glass heaters through liquid sacrificial substrate electric-field-driven jet. Small 18, 2107811. doi:10.1002/smll.202107811
Li, M., Lv, Y., Jin, Y., and Li, Y. (2022b). Simulation Research on Interdigitated Electrodes based sensors in water solution monitoring. J. Phys. Conf. Ser. 2417, 012026. doi:10.1088/1742-6596/2417/1/012026
Liu, Y., Zhou, A., and Yuan, L. (2019). Gelatin-coated michelson interferometric humidity sensor based on a multicore fiber with helical structure. J. Light. Technol. 37, 2452–2457. doi:10.1109/JLT.2019.2907568
Mutee ur Rehman, H. M., Khan, M., Rehman, M. M., Khan, S. A., and Kim, W. Y. (2022). High-performance humidity sensor for multipurpose applications by recycling of potato peel bio-waste. Sensors Actuators A Phys. 343, 113662. doi:10.1016/j.sna.2022.113662
Mutee Ur Rehman, H. M., Rehman, M. M., Saqib, M., Ali Khan, S., Khan, M., Yang, Y., et al. (2021). Highly efficient and wide range humidity response of biocompatible egg white thin film. Nanomaterials 11, 1815. doi:10.3390/nano11071815
Pereira, P. F. M., Picciani, P. H. S., Calado, V. M. A., and Tonon, R. V. (2020). Gelatin-based nanobiocomposite films as sensitive layers for monitoring relative humidity in food packaging. Food Bioprocess Technol. 13, 1063–1073. doi:10.1007/s11947-020-02462-5
Rahman, S. A., Khan, S. A., Rehman, M. M., and Kim, W.-Y. (2022). Highly sensitive and stable humidity sensor based on the Bi-layered PVA/graphene flower composite film. Nanomaterials 12, 1026. doi:10.3390/nano12061026
Rehman, H. M. M. U., Prasanna, A. P. S., Rehman, M. M., Khan, M., Kim, S., and Kim, W. Y. (2023). Edible rice paper-based multifunctional humidity sensor powered by triboelectricity. Sustain. Mater. Technol. 36, e00596. doi:10.1016/j.susmat.2023.e00596
Rehman, M. M., Rehman, H. M. M. U., Gul, J. Z., Kim, W. Y., Karimov, K. S., and Ahmed, N. (2020). Decade of 2D-materials-based RRAM devices: A review. Sci. Technol. Adv. Mater. 21, 147–186. doi:10.1080/14686996.2020.1730236
Rehman, M. M., ur Rehman, H. M. M., Kim, W. Y., Sherazi, S. S. H., Rao, M. W., Khan, M., et al. (2021). Biomaterial-based nonvolatile resistive memory devices toward ecofriendliness and biocompatibility. ACS Appl. Electron. Mater. 3, 2832–2861. doi:10.1021/acsaelm.1c00078
Roy, J. J., Rarotra, S., Krikstolaityte, V., Zhuoran, K. W., Cindy, Y. D., Tan, X. Y., et al. (2022). Green recycling methods to treat lithium-ion batteries E-waste: A circular approach to sustainability. Adv. Mater. 34, 2103346. doi:10.1002/adma.202103346
Saqib, M., Ali Khan, S., Mutee Ur Rehman, H. M., Yang, Y., Kim, S., Rehman, M. M., et al. (2021). High-performance humidity sensor based on the graphene flower/zinc oxide composite. Nanomaterials 11, 242. doi:10.3390/nano11010242
Shapardanis, S., Hudpeth, M., and Kaya, T. (2014). Gelatin as a new humidity sensing material: Characterization and limitations. AIP Adv. 4, 127132. doi:10.1063/1.4904724
Yan, L., Hu, T., Li, X., Ding, F., Wang, B., Wang, B., et al. (2022). Graphdiyne and ionic liquid composite modified gold electrode for sensitive voltammetric analysis of rutin. Electroanalysis 34, 286–293. doi:10.1002/elan.202100258
Yang, C. C., Wu, C. C., Hsiao, Y. L., Wu, Z. H., Chang, S. H., Wang, H. Y., et al. (2022). Dielectric properties of zinc oxide sensing film with nitrogen doped in capacitance relative humidity sensor. IEEE Trans. Dielectr. Electr. Insul. 29, 1. doi:10.1109/TDEI.2022.3163798
Yang, J., Guan, C., Yu, Z., Yang, M., Shi, J., Wang, P., et al. (2020). High sensitivity humidity sensor based on gelatin coated side-polished in-fiber directional coupler. Sensors Actuators B Chem. 305, 127555. doi:10.1016/j.snb.2019.127555
Keywords: biomaterial, gelatin, humidity sensor, multiple applications, fast reaction time
Citation: Khan M, Rehman MM, Khan SA, Saqib M and Kim WY (2023) Characterization and performance evaluation of fully biocompatible gelatin-based humidity sensor for health and environmental monitoring. Front. Mater. 10:1233136. doi: 10.3389/fmats.2023.1233136
Received: 01 June 2023; Accepted: 07 July 2023;
Published: 25 July 2023.
Edited by:
Farooq Sher, Nottingham Trent University, United KingdomReviewed by:
Muhammad Kashif, Tianjin University, ChinaCopyright © 2023 Khan, Rehman, Khan, Saqib and Kim. This is an open-access article distributed under the terms of the Creative Commons Attribution License (CC BY). The use, distribution or reproduction in other forums is permitted, provided the original author(s) and the copyright owner(s) are credited and that the original publication in this journal is cited, in accordance with accepted academic practice. No use, distribution or reproduction is permitted which does not comply with these terms.
*Correspondence: Muhammad Muqeet Rehman, bXVxZWV0MTk4OEBqZWp1bnUuYWMua3I=; Woo Young Kim, c2VtaWd1bWlAamVqdW51LmFjLmty
Disclaimer: All claims expressed in this article are solely those of the authors and do not necessarily represent those of their affiliated organizations, or those of the publisher, the editors and the reviewers. Any product that may be evaluated in this article or claim that may be made by its manufacturer is not guaranteed or endorsed by the publisher.
Research integrity at Frontiers
Learn more about the work of our research integrity team to safeguard the quality of each article we publish.