- 1Rochester Institute of Technology, Rochester, NY, United States
- 2Rochester Institute of Technology, Golisano Institute of Sustainability, Rochester, NY, United States
- 3Thomas H. Gosnell School of Life Sciences, Rochester Institute of Technology, Rochester, NY, United States
- 4Department of Packaging and Graphic Media Science, Rochester Institute of Technology, Rochester, NY, United States
- 5Department of Manufacturing and Mechanical Engineering Technology, Rochester Institute of Technology, Rochester, NY, United States
Biodegradable Mulch Films (BMFs) offer a sustainable alternative to traditional non-degradable (Polyethylene) PE mulch films. However, their slow rate of biodegradation can lead to plastics accumulation in soil. In this study, a commercially available BMF based on poly (butylene adipate co-terephthalate) (PBAT) and poly (lactic acid) (PLA) is examined. Here the effects of gliding arc plasma treatment on the bulk and surface properties, as well as its degradation behavior in soil and compost is studied. An increase in surface oxygen containing species and hydrophilicity was observed following plasma treatment. Only a small hydrophobic recovery was noted over 30 days. No changes in the bulk polymer molecular weight or thermal properties following treatment were noted. However, a decrease in mechanical strength was observed following gliding arc plasma treatment. The onset of film fragmentation in both soil and compost occurred earlier for a plasma treated film and we attribute this to an improvement in the initial adhesion of bacteria on the surface.
1 Introduction
Plastic usage is common in agriculture and can be seen everywhere from mulch films, storage silos and greenhouse covering (Dilara and Briassoulis, 2000; Espí et al., 2006; Sander, 2019). Mulch films consist of about 50% (Soil Degradable Malinconico, 2017) of the entire plastic usage in agricultural applications. Mulch films are used to create a more suitable environment for crop growth by providing a physical barrier thus aiding in soil moisture retention, preventing weed growth, and maintaining a desirable soil temperature. Previous studies have reported a significant improvement in crop yield after using plastic mulch films: For example, a 45%–47% improvement in musk melon outputs and up to 369% increment in bell pepper yields have been reported (James et al., 2019). In addition, water savings of 12%–35% resulting from the use of mulch films have been reported (Liu et al., 2014a). All of this provides a strong argument for the use of plastic mulch in agricultural applications and why it is needed to keep up with increasing food demands.
Traditional poly (ethylene) (PE) mulch films are cheap, flexible and tend to do well in harsh outdoor conditions (Kasirajan and Ngouajio, 2012; Sander, 2019). However, due to their non-biodegradable nature, PE based films must be removed from the field and this gives rise to added labor costs. From an environmental point of view, this also poses a potential problem of microplastic accumulation in the field. Likewise, films must be disposed of following removal which might include landfill tipping fees. Also, there have been reports of farmers burning the plastic (Levitan, 2005; Brodhagen et al., 2015) which is not a desirable end of life outcome from an environmental point of view.
Biodegradable mulch films (BMFs) provide alternative end of life strategies over non-biodegradable mulches. BMFs are either soil degradable, wherein they could be tilled with the soil and allowed to degrade and/or compostable thus allowing them to be removed from the field and placed into a composter. Commercially available mulch films are generally composed of polysaccharides and aliphatic or aromatic polyesters which are prone to hydrolysis (Müller et al., 2001; Brodhagen et al., 2015). In particular, the usage of PHA (poly (hydroxyalkanoates)), PBAT (poly (butylene adipate-co-terephthalate)), PLA (poly (lactic acid)) and starch is fairly common in biodegradable/compostable mulch films (Serrano-Ruíz et al., 2018). Blending PLA/PBAT results in better modulus of elasticity (as compared to PBAT) and improved toughness (as compared to PLA) (Su et al., 2020).
Soil degradation of BMFs are estimated to take as many as 6 years to full degrade (Sintim et al., 2020; Gao et al., 2021). Not surprisingly, the geographic location where the mulch film is used influences the degradation rate, where cooler climates generally reduces the rate of degradation (Li et al., 2014a). Lamparelli et al. (Study of the Biodegradation of, 2021) express concerns with low temperatures affecting the rate of degradation of PBAT/PLA blends. They further report that the presence of microbes in soil makes it the faster method of degradation over hydrolytic degradation under these conditions.
The biodegradation of polymers in soil is described as a three-step process consisting of film surface colonization by soil microorganisms, followed by enzymatic depolymerization of the polymer into small molecules (monomers/oligomers) and finally their utilization for energy production and biomass formation (Zumstein et al., 2018; Sander, 2019). Given that the initial degradation phase of polymers is dominated by surface colonization of soil microbes (Cai et al., 2019; Sander, 2019), bacteria attachment on the film surface can be an important determining factor in dictating the rate of degradation. Bacterial attachment on plastics has been studied in detail and results suggest that polymer surfaces can serve as a substrate for microbe environment (Zettler et al., 2013; De Tender et al., 2017; Urbanek et al., 2018) and this has been further strengthened by research in biofilm formation on plastic surfaces (Garrett et al., 2008; Feng et al., 2015; Delacuvellerie et al., 2021).
Generally, polymers have relatively hydrophobic surface properties and this limits their rate of biodegradation (Pathak and Navneet, 2017). Engineering of the polymer surface to enhance favorable microbial interactions may be a means to increase the environmental biodegradation of polymers. This was shown by Lea et al. (Gazvoda et al., 2021) who demonstrated an increase in the wettability of PLLA films following alkaline etching and this resulted in favorable enzymatic degradation. The authors reported this happens due to the ability of the surface to permit water, enzymes, and aqueous solutions to easily colonize and access the surface of the polymer. In the current study, plasma surface treatment will be utilized to alter the surface hydrophobicity of a polymer film in an effort to understand its influence on soil microbial attachment and degradation in soil.
Surface treatment of polymers is a commonly applied industrial practice to improve adhesion, printability, and dye uptake of polymer surfaces (Grace and Gerenser, 2003; James et al., 2019). Plasma treatment is one of the most versatile methods of surface modification when compared to other techniques such as physical modification, flame and chemical surface etching (Komvopoulos, 2005). Plasma treatment can result in a variety of surface changes including functionalization, deposition, etching and crosslinking (James et al., 2019). Unlike other methods, plasma surface treatment does not involve the use of harsh chemicals or release toxins, which makes it an environmentally friendly method of polymer surface modification (Komvopoulos, 2005; Kusano, 2014). Atmospheric plasma specifically can be used to incorporate a variety of nitrogen and oxygen containing species on polymeric surfaces (Ramkumar et al., 2018). In this study, we utilize a gliding arc plasma as our surface treatment process. Gliding arc plasma is generated between diverging electrodes at high voltages and is quenched by gas flow. It achieves high reactivity and efficient productivity (high energy density) and is an environmentally clean process (Fridman et al., 1999). Plasma treatment has been identified as an AOP (Advanced Oxidation Processes) to enhance biodegradation of common microplastics such as poly (ethylene), poly (propylene) and poly (styrene). (Bule Možar et al., 2023).
In this work, we investigate the effects of atmospheric pressure, gliding arc plasma on microbial attachment and degradation performance of a commercially available mulch film based on a blend of PBAT and PLA. Here we test the hypothesis that surface treatment of a biodegradable mulch film can aid in colonization of a BMF and enhance its degradation rate in both soil and a low temperature compost. It has been well established in the biomedical field that surface treatment, including plasma treatment, greatly enhances plastic material/cell interactions and can lead to enhanced degradation but to the best of our knowledge this is the first time that this has been applied to an agricultural mulch film application.
In this work the surface of an untreated and plasma treated film is characterized using X-ray Photoelectron Spectroscopy (XPS) and contact angle measurements to assess the efficacy of the plasma treatment to modify the film surface. Differential Scanning Calorimetry (DSC) and Gel Permeation Chromatography (GPC) was used to characterize the influence of plasma treatment on the bulk condition of the film. Additionally, tensile testing was performed on an untreated and treated film (1/4’ working distance, 20 s exposure time) to assess the impact of the plasma treatment on the mechanical properties. These two films were subjected to aerobic soil and low temperature aerated compost degradation. Samples were retrieved throughout the course of these experiments and resulting film surfaces were characterized using optical microscopy and Scanning Electron Microscopy (SEM) with select samples examined via GPC. Finally, microbial adhesion was evaluated by comparing cellular adhesion of Pseudomonas Guariconesis spp. on the surfaces of a plasma treated and an untreated film and differences in cellular adhesion is viewed in the context of film degradation results.
2 Materials and methods
2.1 Materials
Organix™ A.G. film was sourced from Berry Hill Irrigation Specialists and used as purchased. The film is described to be “OK biodegradable” per the manufacturer and is TUV-Austria certified for soil biodegradability. Further, the film is based on BASF’s EcoVio. Differential scanning calorimetry (DSC), Thermogravimetric Analysis (TGA) and Fourier Transform Infrared Spectroscopy (FTIR) agrees with that reported by other researchers (Anunciado et al., 2021) and confirms the presence of PBAT and PLA) (Figure 1A) and an inorganic fraction (See Supplementary Figure S1 for details). The thickness of the film is ∼20 µm and one side of the film is black while the other side is white.
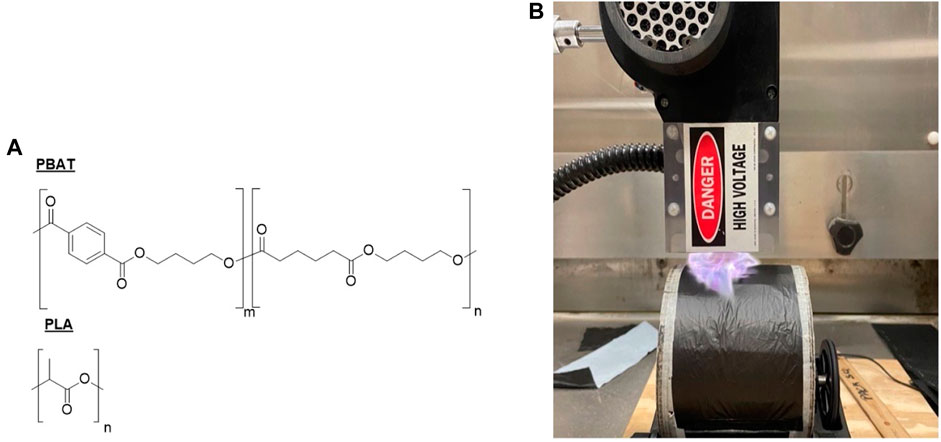
FIGURE 1. Experimental approach to examine effect of plasma treatment on biodegradable mulch films. (A) Structure of PBAT and PLA materials used in this investigation; (B) Plasma treatment method where films were wrapped around a rotating cylinder (100 mm diameter, 130RPM) and placed ¼″ from the plasma head.
2.2 Plasma treatment
A gliding arc plasma treater PJ-2 from Corotec was used for the treatment of the films (Figure 1B). The plasma head inputs are 8 A and 20 V with the output being at 0.06 A and 12 kV. This is a commercially available instrument and lacks the ability to adjust the inputs for the plasma discharge, hence the distance from the plasma head and total exposure to plasma were used to find a suitable set of conditions. The films were wiped down with a Kimwipe and wrapped around a rotating cylinder (130 RPM) measuring ∼75 mm in width and 100 mm in diameter. No additional pre-treatments were performed prior to plasma treatment. The low thermal mass of the film required that it be treated quickly; this was accomplished by treating through multiple passes to allow the surface to cool down after each pass. Treatment time is a popular technique to control the plasma exposure as can also be seen in the work by Darvish et al. (2020). The treatment was carried out for up to 20 s with the sample placed 6.4 mm from the plasma head. These conditions were chosen using a trial-and-error method with the criteria that we did not overheat the specimen and/or induce obvious surface damage (as indicated using optical microscopy and SEM analysis) while still achieving a change in wettability (as indicated by contact angle measurements). For this study only the black side of the film was treated which allowed better visualization of the treatment.
2.3 Film characterization
2.3.1 Contact angle
Contact angle measurements for the films were made using a Ramé-Hart goniometer combined with DROPimage advanced image analysis software. A 1 µL deionized water droplet was placed on the film at three different sections of the surface. The sections were selected at random, and droplets were placed using the Ramé-Hart automated dispensing system, as shown in Supplementary Figure S2. 10 measurements were taken per drop to minimize the error in the image-processing algorithm. The angle reported is the average of 30 measurements. Contact angle measurements were made 2 h after treatment. Measurements were also made after 15 and 30 days where the samples were stored in an airtight container at room temperature away from any source of light to study hydrophobic recovery of the treatment.
2.3.2 X-ray photon spectroscopy (XPS)
X-ray photon spectroscopy (XPS) was performed on films using an XPS–K-Alpha spectrometer (ThermoFisher Scientific, United Kingdom). XPS data was collected (analysis chamber pressure = 4.5 × 10−6 Pa) using a micro-focused, monochromatic Al Kα X-ray source (1,486.6 eV) with a lateral resolution of 30 μm. The atomic concentrations of all the elements were calculated by determining the relevant integral peak intensities using Shirley background.
2.3.3 Thermal characterization
Thermal characterization of films was carried out using a TA Instruments Q250 DSC in a nitrogen environment. ∼ 9 mg of the sample was packed in a hermetically sealed pan and was heated to 200°C at 15°C/min to get rid of the thermal history, cooled to −50°C at 10°C/min and then heated back again to 200°C. The melting, glass and crystallization transitions were observed, and the enthalpies were calculated using TA Instruments TRIOS software (version 5.1).
2.3.4 Gel permeation chromatography (GPC)
Gel permeation chromatography was performed on an untreated film and two plasma treated films (10 s and 20 s exposure times) using a Shimadzu LC 2030 plus detector system containing two Agilent ResiPore, 300 × 7.5 mm columns. In this experiment a refractive index detector (RI) was used. HPLC grade Chloroform (Chloroform, HPLC Grade, 99.5+% min, stab. with amylene, Thermo Scientific™) was used as the mobile phase and calibration was carried out using poly (styrene) (PS) standards (Sigma Aldrich, range of 500–1,000,000 g/mol). Sample concentration was 2.5 mg/mL and the solutions were filtered using a 0.2-micron PTFE filter. Experiments were carried out at 1 mL/min and at a temperature of 30°C.
3 Soil and composting experiments
3.1 Soil degradation
Soil degradation of 75 mm × 75 mm samples (untreated film and a plasma treated film (1/4″ working distance, 20 s exposure time)) was first carried out for 3 months in a non-aerated soil burial test (measured in triplicate) and a detailed description of the soil burial trial can be found in Supplementary Figure S3. This experimental approach was adapted from an article that uses a similar lab technique to determine biodegradability of starch based films (Luchese et al., 2018). Samples were tested as whole films so as to better assess the influence of the plasma treatment process; Grinding the films would generate untreated surfaces introducing a complexity to interpret the results post degradation. A 19 L bin with dimensions 457 mm × 355 mm x 127 mm (18″ x 14″ x 5″) was used for each time trial. All bins were first filled halfway with soil (about 60 mm high) whereupon film samples were placed atop 500 mm × 100 mm mesh screens. The use of mesh bags is a commonly used method (Li et al., 2014a; Li et al., 2014b; Adamcová et al., 2018; Ghimire et al., 2020; Zhang et al., 2020) as it helps to categorize samples and enable their efficient retrieval. An additional 60 mm layer of soil was placed atop the films to complete the sample preparation process. Moisture was maintained throughout the exposure in the range of 50%–60% and was carried out indoors at 21°C ± 1°C. For sample retrieval, the soil from the top of the bin was carefully removed using a small hand shovel after which the mesh screen was pulled out. The samples were then removed from the mesh bag, cleaned using a deionized water spray and then dried at 60°C overnight. To minimize damage to films during cleaning, samples were first placed onto an aluminum plate to provide mechanical reinforcement during spraying. Deionized water was slowly sprayed onto the surface of the sample using a hand pump. Samples were not mechanically manipulated (scrubbed, wiped, etc.) to further minimize damage during this step. Sample removal was performed once every month for a total of 3 months. The soil used for testing is a commercially available high nutrient garden amendment (Bovung Manure Blend). The soil was characterized, and these results are included in the Supplementary Table S1. Notably, CO2 burst (124 mg/L) and Solvita Labile Amino-Nitrogen (SLAN) (387 mg/kg) indicate high microbial life/activity in the soil, well supplied amounts of organic matter and a good capacity to supply nitrogen. Further, in-house bacterial and fungal isolations (using the soil) at 30°C and 40°C were performed which revealed high microbial diversity and colony counts [see Supplementary Figures S4, S5 for results and Plate count agar (PCA)/Potato dextrose agar (PDA) with streptomycin sulfate at 1 μg/mL experimental details]. Summary of the results showed that the chosen soil has parameters suitable to be used as both a soil and as a key ingredient in the compost inoculum (see below).
3.2 Degradation under composting conditions
Degradation under composting conditions (up to 248 days) was performed on an untreated film and a plasma treated film (1/4″ working distance, 20 s exposure time) in a 190 L forced aerated static pile composter (see Supplementary Figure S6 for details). We anticipate that degradation in soil would occur primarily at mesophilic temperatures, either during the growing season or afterward, where the BMF has been tilled into the field. As such, the mild compositing conditions used in this study were selected to accelerate degradation without significantly changing the mesophilic microbes present in the soil. Forced aeration is a common way to introduce oxygen into a closed compost system ensuring proper maturity of the compost over. The forced air method was developed based on pre-testing of the pile using cellulose films (Supplementary Figure S7) which indicated an increased rate of degradation as compared to naturally aired studies. The design for the compost experiment was based on previously published articles that investigated the impacts of aeration using small scale forced air systems (Bhave and Kulkarni, 2019). As with soil degradation experiments, samples measured 75 mm × 75 mm and were tested in triplicate. The compost consisted of 15% spent coffee grounds (SCG) and 15% coffee chaff (CC) with 70% of the Bovung manure blend (see above). Using standard substrate like SCG and CC helps establish a good baseline scenario as the nutrients in the substrate remain consistent over time and are not affected by the location of procurement. In this experiment, films were buried in soil and periodically removed for visual inspection and SEM analysis (see below) to assess degradation. The temperature was monitored throughout the experiment using a glass tube thermometer and found to be typically 5°C–6°C above ambient for the first 5–6 weeks and mimicking ambient temperature for the rest of the experiment. Testing was performed on three films which were placed in custom mesh bags with opening of 1 mm2 (made by sealing Saint-Gobain ADFORS screens on three sides) and taken out during the time of measurement (∼15–30 days) without disrupting the rest of the pile. To minimize damage during cleaning, samples were first placed onto an aluminum plate to provide mechanical reinforcement during spraying. Deionized water was slowly sprayed onto the surface of the sample using a hand pump. Samples were not mechanically manipulated (scrubbed, wiped, etc.) to further minimize damage during this step.
3.3 Surface morphology
Surface morphology of an untreated film and a treated film (1/4″ working distance, 20 s exposure time) was observed using a TESCAN SEM at 10 keV at 20 mm working distance at ×500 magnification. Samples were stuck on SEM stages with carbon tape and then sputter coated with Palladium at 2 Mbar using a SPI-module sputter coater in an argon environment.
3.4 Mechanical properties
Mechanical properties of an untreated film and a treated film (1/4″ working distance, 20 s exposure time) were tested (in tension) using an Instron model 5,542 mechanical tester utilizing ASTM D882 (standard for thin films) at a rate of 1 mm/s. Films were cut using a shear cutter with a 25.4 mm width and 150 mm length and a grip separation of 100 mm. Six tests were conducted for each sample.
3.5 Cell adhesion
Cell adhesion can be measured on the surface by various methods as described by Ungai-Salánki et al. (2019). In this study cell attachment was assessed an untreated film and a treated film (1/4″ working distance, 20 sexposure time) using a modified “centrifugal assay” method (McClay et al., 1981; Lotz et al., 1989; Chu et al., 1994). The culture Pseudomonas guariconensis spp. used in this study was isolated from test soil mentioned in Section 3.1 above. The culture was isolated and identified using an enrichment of cultures technique and 16S rRNA sequencing. Culture was maintained on PCA plates and stored in the refrigerator before its use in cell adhesion tests. Cells were transferred monthly into PCA agar to maintain culture efficacy. For cell adhesion each cell type was grown in 20 mL of tryptic soy broth (TSB) at 28°C–30°C overnight. The samples (measured in triplicate) were stuck inside a 6-well plate using a double-sided 3M tape as shown in Supplementary Figure S8. 9 mL of TSB media is transferred to the plate. 1 mL of 108 CFU/mL of bacteria cells were incubated into each well. The plate is statically incubated at 30°C for 48 h. After the growth cycle, the plate is taken out and centrifuged at 800G.The plate is then inverted and centrifuged again to remove any lightly attached cells on the surface. Finally, films were allowed to dry in an incubator for 20 min before sputter coating with gold palladium prior to imaging via SEM. Cell adhesion before and after treatment was observed using a TESCAN SEM using a 10 KeV electron beam, at ×2000 magnification at 12 mm working distance. Image post-processing was performed using ImageJ software.
4 Results and discussion
4.1 Film characterization
4.1.1 Surface properties: XPS, contact angle, hydrophobic recovery
XPS is a highly sensitive analysis technique commonly used to characterize the surfaces of plasma treated films. XPS survey results are presented in Table 1 and Supplementary Figure S9. For the untreated film, peaks attributed to the O1s (535.08 eV), Ca2p (350.08 eV), C1s (287.08 eV) and S12p (101.08 eV) were observed in the survey scan. For plasma treated films, an increase in both oxygen and nitrogen containing species were observed on the surface with increasing plasma treatment time. For example, the O/C atomic ratio of an untreated film was found to be 0.225. For plasma treatment times of 10 s, the O/C atomic ratio increased by about 95% and for treatment times of 15 s and 20 s the O/C ratio increased to 124% and 107% respectively. An untreated film exhibited no N1s peak (401eV) whereas one was observed for plasma treated films and the N/C ratio of these films was found to be in the range of 0.054–0.055. Similar trends have been observed for plasma treated conventional polymers such as PTFE, acetylene and PE (Chen et al., 2003; Ren et al., 2008; Kuroki et al., 2020; Chiper, 2021). As for biodegradable plastics, Laput et al. (2019) noted an increase in O/C atomic ratio from 0.515 to 1.326 for PLA treated using atmospheric pressure low temperature glow discharge argon plasma with pulse durations of 5 μs Likewise, under these same conditions they observed an increase in O/C atomic ratio from 0.696 to 1.560 for a PLA polymer matrix composite containing 30wt% hydroxyapatite. Jordá-Vilaplana et al. (2014) found a similar result for PLA with both O/C and N/C ratio increasing as a result of atmospheric plasma treatment. Slepička et al. (2012) found that the O/C increased for poly-(4-methyl-1-pentene) (PMP) treated using an Ar plasma treatment from 0.003 for untreated to 0.401 following treatment at 8 W for 240 s. An increase in O/C and N/C ratio was also observed for oxygen and nitrogen, low pressure, non-thermal plasma treated PLA films (Davoodi et al., 2020).

TABLE 1. Elemental composition and C1s regional analysis for samples treated at ¼″ working distance at various treatment times.
High resolution XPS results (C1s) are presented in Figure 2A for an untreated film. As shown, C1s core-level spectra were curve-fitted to the two most prominent peaks corresponding to C-C/C-H (285eV) and C=O peaks (288.7 eV) for untreated films. Peaks at 285.6 eV and 288.7 eV are observed for plasma treated samples (Figure 2B.) and indicate an increase in the presence of oxygen containing species regions attributed to C-O (e.g., C-O/C-O-C) and C=O, respectively. The concentration of these oxygen containing species increases with increasing plasma treatment time as summarized in Table 1. For example, an increase in relative oxygen containing species associated with C-O to 14% is observed at the 10 s treatment condition and this increases further to a maximum of approximately 18% with increased treatment time.
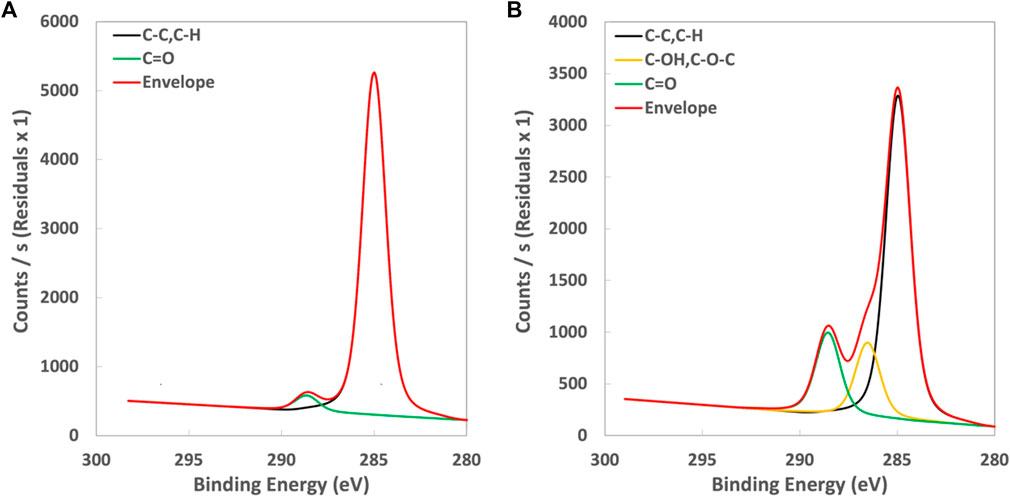
FIGURE 2. Counts/s vs. binding energy plot for (A) untreated and (B) plasma treated film (EV14200) shows additional peak at 286 eV.
Polymers usually are hydrophobic in nature and generally have a contact angle >80° (Homayoun et al., 2018). Increasing polymer hydrophilicity is generally regarded as advantageous in biomedical applications (e.g., to enhance drug delivery, biocompatibility, etc.) (Schmidt, 2019) and we hypothesize that, in this application, it will enhance microbial adhesion during the early stages of degradation in soil and compost. An increase in the concentration of oxygen containing species on the surface was observed as a result of plasma treatment (Figure 3A) and this correlates with a contact angle reduction from 94° to 54° (also see Figure 4).
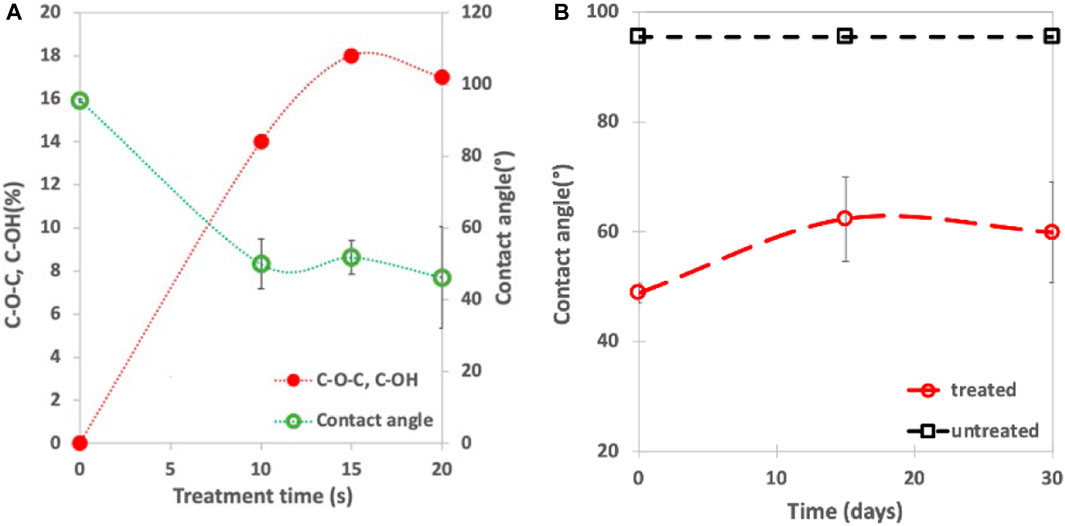
FIGURE 3. Surface properties of biodegradable mulch film are affected by plasma treatment. (A) Contact angle and oxygen containing species change with treatment time. (B) Hydrophobic recovery of a plasma treated sample (EV14200) is limited, thus enabling pre-treatment of mulch. Note: Errors bars for contact angle expressed as ± one standard deviation.
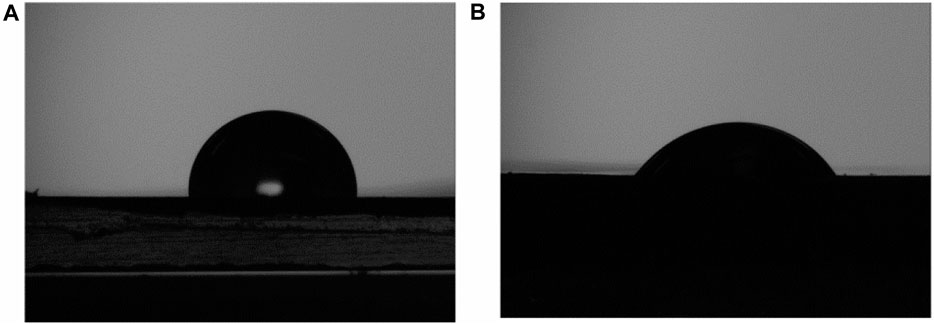
FIGURE 4. Reduction of contact angle was followed the surface plasma treatment, the contact angle of (A) untreated BMF was measured to be 94° whereas (B) a plasma treated mulch (sample EV14200) measured at 54°.
It is well established that the surface properties change with post-plasma treatment aging time and can return back to their original, untreated state (Mortazavi and Nosonovsky, 2012). Several mechanisms have been advanced to explain this so-called hydrophobic recovery process and include surface recontamination, the diffusion of polar groups on the surface into the bulk and the diffusion of un-modified molecules to the surface (Morent et al., 2007; Ren et al., 2017). As shown in Figures 3A, B film treated for 20 s at a 1/4″ working distance (i.e., sample EV14200 as indicated in Table 1) shows only an ∼18% hydrophobic recovery over the first 15 days that remains stable for the remainder of the 30-day observation time. This stability is desirable from an applications point of view in that it allows the pre-treating of mulch films well in advance of field application.
4.1.2 Bulk properties
The thermal properties of an untreated and treated film (sample EV14200) are presented in the DSC second heat thermograms presented in Figure 5; Table 2. Here two melting transitions corresponding to the PBAT (∼127°C) and the PLA (∼150°C) components are observed. This agrees with that reported in the literature (Arruda et al., 2015; Su et al., 2020) and the presence of two melting peaks results from the immiscibility of the two polymers. No significant differences in the DSC scans suggests that plasma treatment does not significantly alter the bulk material of the polymer film, and this was observed for samples subjected to shorter treatment times as well (Supplementary Figure S10). The number average molecular weight (Mn) of films treated for 10 s and 20 s was determined using GPC to be 52,020 g/mol (PDI = 1.7) and 50,400 g/mol (PDI = 1.7) respectively and showed only a small difference as compared to an untreated film (Mn = 51,000 g/mol; PDI = 1.8). This observation, combined with the DSC results, indicates that the gliding arc plasma treatment has little to no effect on the constituent polymers in the bulk film. That plasma treatment influences only the surface of a polymer while leaving the bulk properties unaffected is not an uncommon observation (Yousefi Rad et al., 1998; Morent et al., 2008; Vesel et al., 2011; Meemusaw and Magaraphan, 2016). Given the observation that long treatment times exhibit the highest level of surface activation without inducing bulk degradation of the polymer led us to select the EV14200 sample for further study. Consequently, we will limit the discussion to the 20 s plasma treated sample, referring to it simply as the plasma treated sample for the remainder of this paper.
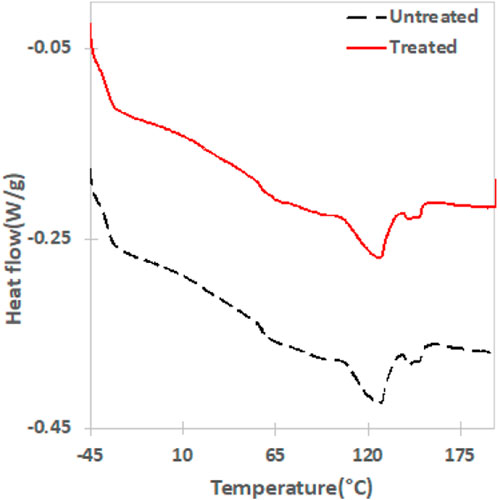
FIGURE 5. Second heat DSC thermograms (endothermic down) following plasma treatment (sample EV14200 shown) exhibits no differences in thermal transitions of the constituent polymers as compared to untreated film.
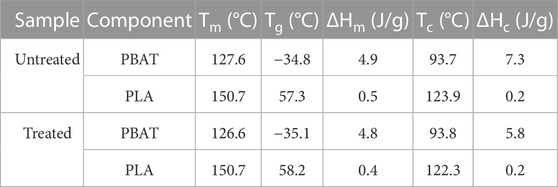
TABLE 2. Consolidated DSC results for an untreated (EV00) and plasma treated sample (sample EV14200).
4.2 Mechanical properties
Mechanical properties of a BMF are an important factor to consider as they provide a physical barrier from the external environment. Moreover, BMFs are subject to stress during their application onto the field and therefore their mechanical properties are of primary concern. To assess this, the tensile behavior of an untreated and plasma treated BMF were evaluated in the machine direction. As shown in Figure 6; Supplementary Figure S11, the untreated BMF displays a tensile yield strength of approximately 8 MPa, an ultimate tensile strength of approximately 15 MPa and strain at break of about 3.2 mm/mm. This generally agrees with that reported in the literature. Xin Wang et al. report an elongation of break of 357%–480% for blends of PBAT/PLA (Mechanical properties, 1016). Similarly, Guocheng Han et al. report a tensile strength of 18–20 MPa and an initial elongation of break of 600% (Han et al., 2020).
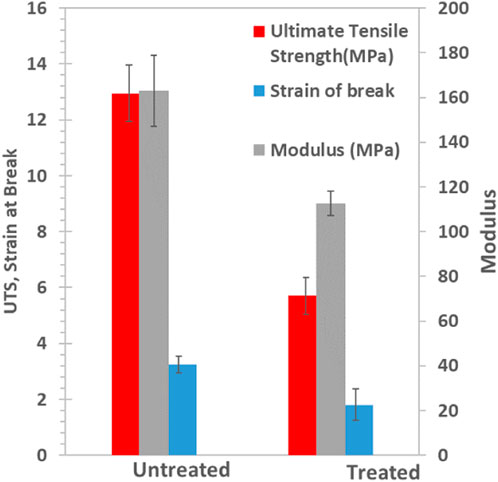
FIGURE 6. A reduction in mechanical properties was observed in the films following plasma treatment (EV14200). This is due to the higher power of the plasma source used in the study which introduces small defects on the films.
A reduction in mechanical properties was observed following plasma treatment (Figure 6; Supplementary Figure S11). Here a reduction in strain at break (44% decrease), modulus (31% decrease) and ultimate strength (56% decrease) was observed for a sample subjected to 20 s treatment time (sample EV14200). The reduction in the mechanical properties is not due to changes in the chemical composition of the bulk film material as confirmed by the DSC and GPC results presented earlier. As shown in Supplementary Figure S12, untreated samples are dull whereas plasma treated surfaces exhibit a shiny appearance along with the presence of surface irregularities. We suspect that the reduction in properties for the plasma treated sample results from localized damage to the surface of the film and it is these micro-defects that initiate failure following the application of stress. Interestingly, the elongation at break of the treated film showed higher variability as compared to untreated films (Supplementary Table S2) and is taken as a reflection of the variability of the surface treatment process itself.
We postulate that the loss in mechanical property is due to the high intensities of the plasma treatment as has been stated in previous studies (Kim and Masuoka, 2009; Meemusaw and Magaraphan, 2016). For example, Meemusaw and Magaraphan (2016) report only a slight reduction in the elongation at break of HDPE that had been treated using an atmospheric plasma treatment using nitrogen flushing. Kim et al. also discuss the presence of pin holes post treatment (Kim and Masuoka, 2009). Given that the bulk properties of the materials are unaffected by the plasma treatment and the sporadic nature of the defects observed here may indicate that optimizing the plasma treatment process would reduce the effect that treatment has on mechanical properties. More work would be required to understand if this level of property loss would present issues during handling and application in the field. As an alternative, milder treatments such as dielectric barrier discharge (DBD) plasma treatment may result in better mechanical properties of these thin films, though additional testing to confirm the other effects (e.g., degradation, cellular adhesion, etc.) would be required as this is a different type of plasma treatment and therefore will likely result in a different surface chemistry. Furthermore, for applications where the plastic is not the major stress bearing member (e.g., paper coated with such materials) or in cases where material thickness is > 20 μm, optimizing the gliding arc plasma treatment process may not be necessary.
4.3 Degradation in soil and compost
Soil degradation of the BMFs was carried out in static pile soil bins for 3 months. Images of treated and untreated films in soil are presented in Figure 7. Here significant difference in the surfaces of the films is noted even after this short time period of 1 and 2 months. Untreated mulch film shows little to no signs of deterioration with only a small amount of residual organic media remaining on the surface to signify its exposure to soil. In contrast, plasma treated mulch after 2 months in soil shows cracks about 2–3 µm in width. This confirms the claim that surface modification of these plastic mulches positively affects the rate of degradation. It was also noteworthy to mention that the process of degradation in soil is a slow process and agrees with that found by others for this same film type (Anunciado et al., 2021).
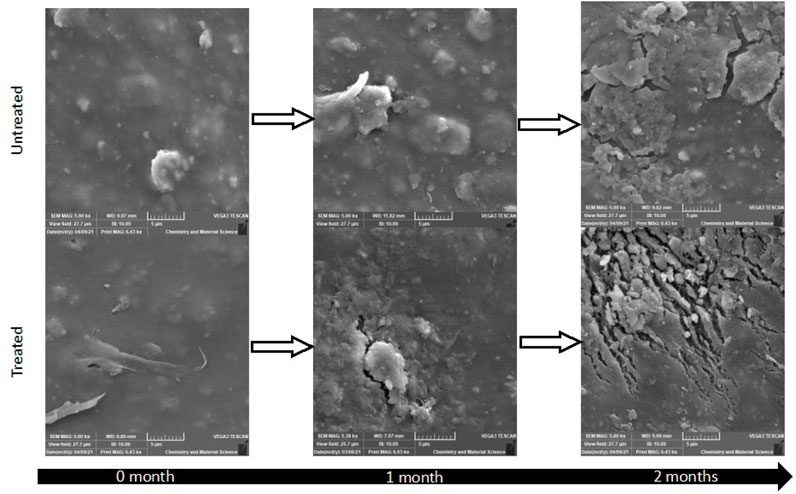
FIGURE 7. SEM images of untreated (top) and plasma treated (EV14200, bottom) films. Two months after soil burial, no surface damage is observed on the untreated film apart from soil/dirt adhered on it whereas clear signs of damage can be observed on the treated films in the form of surface cracks.
Under low temperature composting conditions, plasma treated samples also exhibit enhanced degradation as compared to untreated films. Surface morphology of untreated and plasma treated films before and after soil degradation are presented in Figure 8. A clear distinction can be made between the two with the amount of physical damage on the surface. As shown in Figure 8A an untreated film is free from cracks after 65 days in compost whereas a plasma treated film (Figure 8B) shows large holes measuring ∼120 µm in length and 25 µm in width following the same amount of exposure time.
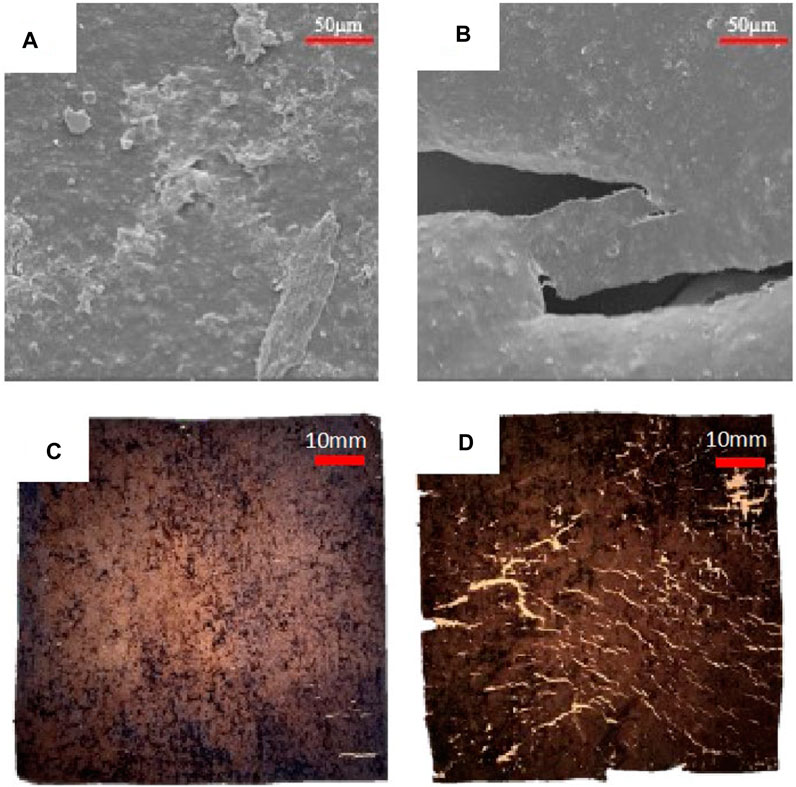
FIGURE 8. Plasma treated film (EV14200) exhibits enhanced biodegradation under low temperature composting conditions as compared to an untreated film. SEM images following 65 days of exposure to composting for (A) untreated film and (B) treated film. After 248 days, the difference in the extent of degradation is evident without the need for magnification. For example, an untreated film (C) shows some minor signs of degradation such as a few small cracks whereas the plasma treated film (D) exhibits large cracks and is easily fragmented.
We observed relatively slow degradation under composting conditions in this experiment and this is suspected of being due to the low temperature, mesophilic conditions employed. A similar observation was made by Nomadolo et al. (2022) who looked at biodegradation of PBAT-PLA blended films in home composting (mesophilic) conditions of 28°C incubation. They reported a 50% rate of biodegradation measuring CO2 evolution from films in 200 days. Gioia et al. (2021) reported on composting studies that also showed low biodegradation rates under mesophilic conditions. They also explained that in blended plastics, the PBAT component takes much longer to assimilate by composting biota. This is supported by observations made by Jian et al. (2020), who showed much faster degradation when composting PBAT under thermophilic conditions (complete degradation of the polymer in 90 days). As described earlier, the mild compositing conditions used in this study were selected to accelerate degradation without significantly changing the mesophilic microbes present in the soil. We suspect that degradation would be hastened by the use of industrial composter operating at elevated temperatures, thus providing a potential alternative to the approach taken in this study.
At longer times, untreated films exhibit signs of deterioration after 180 days of composting; at this point the treated films are heavily damaged and have holes and tears (Supplementary Figure S13). Significant changes are observed 248 days after composting, as presented in Figures 8C, D. As shown, only small cracks are observed on an untreated film whereas the surface of the plasma treated film is covered with cracks and the films are fragile and easily fragmented. No visible changes can be observed on the untreated film otherwise.
Similar molecular weight changes were observed for untreated and treated films throughout the course of the experiment. Over the first 65 days of exposure, no major reduction in polymer molecular weight was observed for either sample. For example, the untreated film molecular weight prior to exposure (Mn = 50,967 g/mol) is nearly the same after 65 days of composting (51,280 g/mol). The fact that there are clear differences in fragmentation rate between plasma treated and untreated films but no significant differences in Mn would seem to indicate a surface degradation mechanism dominance at this time period. It is hypothesized that this may be exacerbated by inconsistencies of the plasma treatment process resulting in very localized areas where degradation is accelerated. At longer treatment times (180 days), a reduction in Mn to ∼40,000 g/mol (PDI = 1.87) (Supplementary Table S3) was observed for both untreated and treated films and, at this reduced molecular weight, the untreated sample is beginning to also show signs of embrittlement (Supplementary Figure S13) though still much less than the plasma treated sample.
4.4 Cell adhesion
Cellular adhesion to BMFs is a key factor in determining the rate of soil degradation. The procedure we used to study microbial attachment on surface of a treated and untreated film is shown in Figure 9. Here films were adhered on a 6 well plate (see also Supplementary Figure S8) and then filled with a cell suspension. Following 48 h of incubation the plate was centrifuged, first in a right side up configuration to promote cellular attachment and then subsequently in an inverted configuration to remove any loosely attached cells. The cell culture was poured out and the surface was allowed to dry in an incubator set at 30°C for 15 min. As indicated in the experimental section, Pseudomonas guariconesis (Toro et al., 2013) were isolated from our test soil and were used for cell adhesion studies. This bacteria was selected for this study as Pseudomonas species have been identified as being able to degrade various plastics (Wilkes and Aristilde, 2017). As shown in Figure 9B, about 2/3 of the untreated film surface is covered whereas nearly the entire surface of the treated film is covered (Figure 9C). We attribute this to an increased hydrophilicity of the film making it easier for the bacteria to attach to the surface.
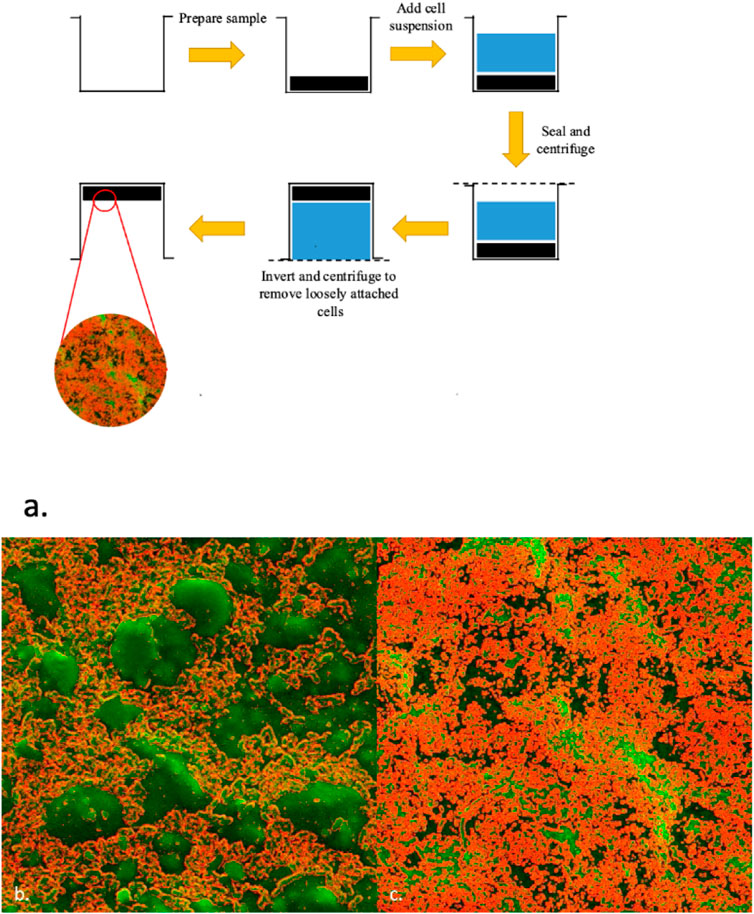
FIGURE 9. Cellular adhesion is enhanced following plasma treatment. (A) Schematic showing steps involved in the study of cell attachment on surface of the films. Films are first stuck to a 6 well plate (black) and filled with cell suspension (blue) where they remain for 48 h in an incubator. The plate is then sealed and centrifuged right side up (to promote adhesion) followed by centrifugation in an inverted orientation to remove loosely attached cells. The resulting surface was dried, sputter coated and observed under the SEM right. SEM images (enhanced using ImageJ) of (B) untreated film as compared to (C) treated film (EV14200) demonstrates improved cellular adhesion (see Supplementary Figure S14 for unprocessed images).
Cell attachment and biocompatibility of plastics and films have shown to improve following various types of plasma treatment (Chen et al., 2003; Vesel et al., 2011; Davoodi et al., 2020; Sundriyal et al., 2020). Chen et al. (2003) investigated the effect of ammonia and oxygen plasma on cell attachment on PTFE surfaces and reports a reduction of contact angle resulting from plasma treatment and a concomitant increase in the number of bovine aorta endothelial cells on the surface. In another study, argon plasma treated PEEK showed enhanced mouse fibroblasts and human osteoblast cell adhesion, proliferation and metabolic activity over that of untreated PEEK (Novotna et al., 2015). Liu W. et al. (2014) in their study on adhesion report reduction of contact angle as a result of O2 plasma treatment; this resulted in significant increase in the porcine mesenchymal stem cells (pMSCs) count on the surface of electrospun PLLA scaffolds. In our study, we hypothesize that this initial colonization of cells is a major contributing factor of improved degradation and translates to lower lag phase in plastic surface degradation.
5 Conclusion
In this work, gliding arc plasma treatment was used as a means to accelerate soil biodegradation for a commercially available mulch film based on PBAT and PLA. These films showed increased hydrophilicity because of plasma treatment (contact angle reduced from 94° to 54°) and this was accompanied by a 107% increase in the oxygen containing functional groups observed on the surface as confirmed using XPS. It is envisioned that mulch films can be pretreated before laying in the fields with only minimal loss in surface activation as indicated by minimal hydrophobic recovery (contact angle change from 54° to 62° after 30 days for a film that had been treated for 20 s). There was no modification made to the bulk properties of the polymer such as the melting, crystallization, and glass transition temperatures. In addition, no changes in molecular weight were observed following plasma treatment. A reduction in mechanical strength of a film (e.g., 44% decrease in elongation at break) was observed following treatment for 20 s and this could be attributed to the presence of pin holes due to the aggressive gliding arc plasma treatment utilized in this study. We hypothesize that optimization of the gliding arc plasma treatment or the use of a milder surface treatment (e.g., dielectric barrier discharge, DBD) may help to mitigate mechanical property losses.
A plasma treated film (20 s treatment time) showed early signs of degradation such as cracking and fragmentation upon exposure to both soil and compost. While this was also true for the untreated film, the differences between the two differed greatly. Surface degradation in compost dominated over the first 65 days, as no change in molecular weight (GPC results) or thermal characteristics (DSC results) was observed whereas at longer times (180 days), ∼20% reduction in the molecular weight was observed. This can be attributed to better microbial adhesion following surface treatment. This was demonstrated by showing that bacteria exhibited improved adhesion to plasma treated films using a modified “centrifugal assay” method.
Plasma treatment is a cheap and environmentally friendly method of plastic film surface modification, and these results indicate that it can improve biodegradation of a BMF. In addition to BMFs, single-use products such as plastic-coated paper cups, plastic silverware and take-out food containers may also benefit from this approach.
Data availability statement
The original contributions presented in the study are included in the article/Supplementary Material, further inquiries can be directed to the corresponding author.
Author contributions
Conceptualization, JL, CD, and CL; formal analysis, SB, HK, and CL; funding acquisition, JL, CD, and CL; investigation, SB and HK; methodology, SB, HK, JL, CD, and CL; project administration, JL, CD, and CL; resources, JL, CD, and CL; supervision, JL, CD, and CL; writing–original draft, SB, HK, and CL; writing–review and editing, SB, HK, JL, CD, and CL. All authors contributed to the article and approved the submitted version.
Funding
The authors gratefully acknowledge the support of Foundation for Food and Agriculture Research (FFAR) grant #CA19-SS-0000000013.
Acknowledgments
The authors would like to acknowledge Nishant Singh and Alex Scott for running GPC measurements and Celina Alvarado for running DSC measurements. Special thanks to Alexander Leo Bardenstein and Yukihiro Kusano (Danish Institute of Technology) who supplied X-ray photon spectroscopy (XPS) results and for discussions concerning plasma technology and data interpretation. A portion of these results were presented at the Society of Plastics Engineers Annual Technical Conference 2022 (Bhattacharya et al.).
Conflict of interest
The authors declare that the research was conducted in the absence of any commercial or financial relationships that could be construed as a potential conflict of interest.
Publisher’s note
All claims expressed in this article are solely those of the authors and do not necessarily represent those of their affiliated organizations, or those of the publisher, the editors and the reviewers. Any product that may be evaluated in this article, or claim that may be made by its manufacturer, is not guaranteed or endorsed by the publisher.
Supplementary material
The Supplementary Material for this article can be found online at: https://www.frontiersin.org/articles/10.3389/fmats.2023.1232577/full#supplementary-material
References
Adamcová, D., Radziemska, M., Zloch, J., Dvořáčková, H., Elbl, J., Kynický, J., et al. (2018). SEM analysis and degradation behavior of conventional and bio-based plastics during composting. Acta Univ. Agric. Silvic. mendel. Brun. 66 (2), 349–356. doi:10.11118/actaun201866020349
Anunciado, M. B., Hayes, D. G., Wadsworth, L. C., English, M. E., Schaeffer, S. M., Sintim, H. Y., et al. (2021). Impact of agricultural weathering on physicochemical properties of biodegradable plastic mulch films: Comparison of two diverse climates over four successive years. J. Polym. Environ. 29 (1), 1–16. doi:10.1007/s10924-020-01853-1
Arruda, L. C., Magaton, M., Bretas, R. E. S., and Ueki, M. M. (2015). Influence of chain extender on mechanical, thermal and morphological properties of blown films of PLA/PBAT blends. Polym. Test. 43, 27–37. doi:10.1016/j.polymertesting.2015.02.005
Bhattacharya, S., Kansara, H. J., Alvarado, C. E., Acosta, C. D., Lodge, J., and Lewis, C. L. “Effect of plasma treatment on mulch film degradation of biodegradable mulch film,” in Society of plastics Engineers annual technical conference (North Carolina: Society of Plastics Engineers: Charlotte).
Bhave, P. P., and Kulkarni, B. N. (2019). Effect of active and passive aeration on composting of household biodegradable wastes: A decentralized approach. Int. J. Recycl. Org. Waste Agric. 8 (S1), 335–344. doi:10.1007/s40093-019-00306-7
Brodhagen, M., Peyron, M., Miles, C., and Inglis, D. A. (2015). Biodegradable plastic agricultural mulches and key features of microbial degradation. Appl. Microbiol. Biotechnol. 99 (3), 1039–1056. doi:10.1007/s00253-014-6267-5
Bule Možar, K., Miloloža, M., Martinjak, V., Cvetnić, M., Kušić, H., Bolanča, T., et al. (2023). Potential of advanced oxidation as pretreatment for microplastics biodegradation. Separations 10 (2), 132. doi:10.3390/separations10020132
Cai, L., Wu, D., Xia, J., Shi, H., and Kim, H. (2019). Influence of physicochemical surface properties on the adhesion of bacteria onto four types of plastics. Sci. Total Environ. 671, 1101–1107. doi:10.1016/j.scitotenv.2019.03.434
Chen, M., Zamora, P. O., Som, P., Peña, L. A., and Osaki, S. (2003). Cell attachment and biocompatibility of polytetrafluoroethylene (PTFE) treated with glow-discharge plasma of mixed ammonia and oxygen. J. Biomater. Sci. Polym. Ed. 14 (9), 917–935. doi:10.1163/156856203322381410
Chiper, A. S. (2021). Tailoring the working gas flow to improve the surface modification of plasma-treated polymers. Mat. Lett. 305, 130832. doi:10.1016/j.matlet.2021.130832
Chu, L., Tempelman, L. A., Miller, C., and Hammer, D. A. (1994). Centrifugation assay of IgE-mediated cell adhesion to antigen-coated gels. AIChE J. 40 (4), 692–703. doi:10.1002/aic.690400412
Darvish, F., Mostofi Sarkari, N., Khani, M., Eslami, E., Shokri, B., Mohseni, M., et al. (2020). Direct plasma treatment approach based on non-thermal gliding arc for surface modification of biaxially-oriented polypropylene with post-exposure hydrophilicity improvement and minus aging effects. Appl. Surf. Sci. 509, 144815. doi:10.1016/j.apsusc.2019.144815
Davoodi, A., Zadeh, H. H., Joupari, M. D., Sahebalzamani, M. A., Khani, M. R., and Shahabi, S. (2020). Physicochemical- and biocompatibility of oxygen and nitrogen plasma treatment using a PLA scaffold. AIP Adv. 10 (12), 125205. doi:10.1063/5.0022306
De Tender, C., Devriese, L. I., Haegeman, A., Maes, S., Vangeyte, J., Cattrijsse, A., et al. (2017). Temporal dynamics of bacterial and fungal colonization on plastic debris in the north sea. Environ. Sci. Technol. 51 (13), 7350–7360. doi:10.1021/acs.est.7b00697
Delacuvellerie, A., Benali, S., Cyriaque, V., Moins, S., Raquez, J.-M., Gobert, S., et al. (2021). Microbial biofilm composition and polymer degradation of compostable and non-compostable plastics immersed in the marine environment. J. Hazard. Mat. 419, 126526. doi:10.1016/j.jhazmat.2021.126526
Dilara, P. A., and Briassoulis, D. (2000). Degradation and stabilization of low-density polyethylene films used as greenhouse covering materials. J. Agric. Eng. Res. 76 (4), 309–321. doi:10.1006/jaer.1999.0513
Espí, E., Salmerón, A., Fontecha, A., García, Y., and Real, A. I. (2006). PLastic films for agricultural applications. J. Plast. Film. Sheeting 22 (2), 85–102. doi:10.1177/8756087906064220
Feng, G., Cheng, Y., Wang, S.-Y., Borca-Tasciuc, D. A., Worobo, R. W., and Moraru, C. I. (2015). Bacterial attachment and biofilm formation on surfaces are reduced by small-diameter nanoscale pores: How small is small enough? Npj Biofilms Microbiomes 1 (1), 15022. doi:10.1038/npjbiofilms.2015.22
Fridman, A., Nester, S., Kennedy, L. A., Saveliev, A., and Mutaf-Yardimci, O. (1999). Gliding Arc gas discharge. Prog. Energy Combust. Sci. 25 (2), 211–231. doi:10.1016/S0360-1285(98)00021-5
Gao, X., Xie, D., and Yang, C. (2021). Effects of a PLA/PBAT biodegradable film mulch as a replacement of polyethylene film and their residues on crop and soil environment. Agric. Water Manag. 255, 107053. doi:10.1016/j.agwat.2021.107053
Garrett, T. R., Bhakoo, M., and Zhang, Z. (2008). Bacterial adhesion and biofilms on surfaces. Prog. Nat. Sci. 18 (9), 1049–1056. doi:10.1016/j.pnsc.2008.04.001
Gazvoda, L., Višić, B., Spreitzer, M., and Vukomanović, M. (2021). Hydrophilicity affecting the enzyme-driven degradation of piezoelectric poly-l-lactic films. Polymers 13 (11), 1719. doi:10.3390/polym13111719
Ghimire, S., Flury, M., Scheenstra, E. J., and Miles, C. A. (2020). Sampling and degradation of biodegradable plastic and paper mulches in field after tillage incorporation. Sci. Total Environ. 703, 135577. doi:10.1016/j.scitotenv.2019.135577
Gioia, C., Giacobazzi, G., Vannini, M., Totaro, G., Sisti, L., Colonna, M., et al. (2021). End of life of biodegradable plastics: Composting versus Re/upcycling. ChemSusChem 14 (19), 4167–4175. doi:10.1002/cssc.202101226
Grace, J. M., and Gerenser, L. J. (2003). Plasma treatment of polymers. J. Dispers. Sci. Technol. 24 (3–4), 305–341. doi:10.1081/DIS-120021793
Han, G., Yu, Z., Guo, R., and Chen, G.Effect of ambient environment on mechanical properties of PBAT/PLA fully-degradable packaging films. In Advanced graphic communication, printing and packaging technology; P. Zhao, Z. Ye, M. Xu, and L. Yang, Eds.; lecture notes in electrical engineering; Springer Singapore: Singapore, 2020; Vol. 600, pp 757–763. doi:10.1007/978-981-15-1864-5_103
Homayoun, M.-R., Golchin, A., and Emami, N. (2018). Effect of hygrothermal ageing on tribological behaviour of PTFE-based composites. Lubricants 6 (4), 103. doi:10.3390/lubricants6040103
James, J., Joseph, B., Shaji, A., Nancy, P., Kalarikkal, N., Thomas, S., et al. (2019). “Microscopic analysis of plasma-activated polymeric materials,” in Non-thermal plasma technology for polymeric materials (USA: Elsevier), 287–317. doi:10.1016/B978-0-12-813152-7.00011-1
Jian, J., Xiangbin, Z., and Xianbo, H. (2020). An overview on synthesis, properties and applications of poly(butylene-adipate-Co-Terephthalate)–PBAT. Adv. Ind. Eng. Polym. Res. 3 (1), 19–26. doi:10.1016/j.aiepr.2020.01.001
Jordá-Vilaplana, A., Fombuena, V., García-García, D., Samper, M. D., and Sánchez-Nácher, L. (2014). Surface modification of polylactic acid (PLA) by air atmospheric plasma treatment. Eur. Polym. J. 58, 23–33. doi:10.1016/j.eurpolymj.2014.06.002
Kasirajan, S., and Ngouajio, M. (2012). Polyethylene and biodegradable mulches for agricultural applications: A review. Agron. Sustain. Dev. 32 (2), 501–529. doi:10.1007/s13593-011-0068-3
Kim, M. C., and Masuoka, T. (2009). Degradation properties of PLA and PHBV films treated with CO2-plasma. React. Funct. Polym. 69 (5), 287–292. doi:10.1016/j.reactfunctpolym.2009.01.013
Komvopoulos, K. (2005). “Plasma-enhanced surface modification of biopolymers,” in World tribology congress III (Washington, D.C., USA: ASMEDC), 701–702. doi:10.1115/WTC2005-64235
Kuroki, T., Nakamura, M., Hori, K., and Okubo, M. (2020). Effect of monomer concentration on adhesive strength of PTFE films treated with atmospheric-pressure nonthermal plasma graft polymerization. J. Electrost. 108, 103526. doi:10.1016/j.elstat.2020.103526
Kusano, Y. (2014). Atmospheric pressure plasma processing for polymer adhesion: A review. J. Adhes. 90 (9), 755–777. doi:10.1080/00218464.2013.804407
Laput, O., Vasenina, I., Salvadori, M. C., Savkin, K., Zuza, D., and Kurzina, I. (2019). Low-temperature plasma treatment of polylactic acid and PLA/HA composite material. J. Mat. Sci. 54 (17), 11726–11738. doi:10.1007/s10853-019-03693-4
Levitan, L. (2005). Reducing dioxin emissions by recycling agricultural plastics: Creating viable alternatives to open burning. New York, NY: Great Lakes Regional Pollution Prevention Roundtable (GLRPPR).
Li, C., Moore-Kucera, J., Lee, J., Corbin, A., Brodhagen, M., Miles, C., et al. (2014b). Effects of biodegradable mulch on soil quality. Appl. Soil Ecol. 79, 59–69. doi:10.1016/j.apsoil.2014.02.012
Li, C., Moore-Kucera, J., Miles, C., Leonas, K., Lee, J., Corbin, A., et al. (2014a). Degradation of potentially biodegradable plastic mulch films at three diverse U.S. Locations. Agroecol. Sustain. Food Syst. 38 (8), 861–889. doi:10.1080/21683565.2014.884515
Liu, E. K., He, W. Q., and Yan, C. R. (2014a). ‘White revolution’ to ‘white pollution’—agricultural plastic film mulch in China. Environ. Res. Lett. 9 (9), 091001. doi:10.1088/1748-9326/9/9/091001
Liu, W., Zhan, J., Su, Y., Wu, T., Wu, C., Ramakrishna, S., et al. (2014b). Effects of plasma treatment to nanofibers on initial cell adhesion and cell morphology. Colloids Surf. B Biointerfaces 113, 101–106. doi:10.1016/j.colsurfb.2013.08.031
Lotz, M. M., Burdsal, C. A., Erickson, H. P., and McClay, D. R. (1989). Cell adhesion to fibronectin and tenascin: Quantitative measurements of initial binding and subsequent strengthening response. J. Cell. Biol. 109 (4), 1795–1805. doi:10.1083/jcb.109.4.1795
Luchese, C. L., Benelli, P., Spada, J. C., and Tessaro, I. C. (2018). Impact of the starch source on the physicochemical properties and biodegradability of different starch-based films. J. Appl. Polym. Sci. 135 (33), 46564. doi:10.1002/app.46564
McClay, D. R., Wessel, G. M., and Marchase, R. B. (1981). Intercellular recognition: Quantitation of initial binding events. Proc. Natl. Acad. Sci. 78 (8), 4975–4979. doi:10.1073/pnas.78.8.4975
Mechanical properties, rheological behaviors, and phase morphologies of high-toughness PLA/PBAT blends by in-situ reactive compatibilization | Elsevier Enhanced Reader. doi:10.1016/j.compositesb.2019.107028
Meemusaw, M., and Magaraphan, R. (2016). Surface and bulk properties improvement of HDPE by a batch plasma treatment. J. Appl. Polym. Sci. 133 (7). doi:10.1002/app.43011
Morent, R., De Geyter, N., Leys, C., Gengembre, L., and Payen, E. (2008). Comparison between XPS- and FTIR-analysis of plasma-treated polypropylene film surfaces. Surf. Interface Anal. 40 (3–4), 597–600. doi:10.1002/sia.2619
Morent, R., De Geyter, N., Leys, C., Gengembre, L., and Payen, E. (2007). Study of the ageing behaviour of polymer films treated with a dielectric barrier discharge in air, helium and argon at medium pressure. Surf. Coat. Technol. 201 (18), 7847–7854. doi:10.1016/j.surfcoat.2007.03.018
Mortazavi, M., and Nosonovsky, M. (2012). A model for diffusion-driven hydrophobic recovery in plasma treated polymers. Appl. Surf. Sci. 258 (18), 6876–6883. doi:10.1016/j.apsusc.2012.03.122
Müller, R.-J., Kleeberg, I., and Deckwer, W.-D. (2001). Biodegradation of polyesters containing aromatic constituents. J. Biotechnol. 86 (2), 87–95. doi:10.1016/S0168-1656(00)00407-7
Nomadolo, N., Dada, O. E., Swanepoel, A., Mokhena, T., and Muniyasamy, S. (2022). A comparative study on the aerobic biodegradation of the biopolymer blends of poly(butylene succinate), poly(butylene adipate terephthalate) and poly(lactic acid). Polymers 14 (9), 1894. doi:10.3390/polym14091894
Novotna, Z., Reznickova, A., Rimpelova, S., Vesely, M., Kolska, Z., and Svorcik, V. (2015). Tailoring of PEEK bioactivity for improved cell interaction: Plasma treatment in action. RSC Adv. 5 (52), 41428–41436. doi:10.1039/C5RA03861H
Pathak, V. M., and Navneet, M. (2017). Review on the current status of polymer degradation: A microbial approach. Bioresour. Bioprocess. 4 (1), 15. doi:10.1186/s40643-017-0145-9
Ramkumar, M. C., Cools, P., Arunkumar, A., De Geyter, N., Morent, R., Kumar, V., et al. (2018). “Polymer coatings for biocompatibility and reduced nonspecific adsorption,” in Functionalised cardiovascular stents (USA: Elsevier), 155–198. doi:10.1016/B978-0-08-100496-8.00009-3
Ren, Y., Wang, C., and Qiu, Y. (2008). Aging of surface properties of ultra high modulus polyethylene fibers treated with He/O2 atmospheric pressure plasma jet. Surf. Coat. Technol. 202 (12), 2670–2676. doi:10.1016/j.surfcoat.2007.09.043
Ren, Y., Xu, L., Wang, C., Wang, X., Ding, Z., and Chen, Y. (2017). Effect of dielectric barrier discharge treatment on surface nanostructure and wettability of polylactic acid (PLA) nonwoven fabrics. Appl. Surf. Sci. 426, 612–621. doi:10.1016/j.apsusc.2017.07.211
Sander, M. (2019). Biodegradation of polymeric mulch films in agricultural soils: Concepts, knowledge gaps, and future research directions. Environ. Sci. Technol. 53 (5), 2304–2315. doi:10.1021/acs.est.8b05208
Serrano-Ruíz, H., Martín-Closas, L., and Pelacho, A. M. (2018). Application of an in vitro plant ecotoxicity test to unused biodegradable mulches. Polym. Degrad. Stab. 158, 102–110. doi:10.1016/j.polymdegradstab.2018.10.016
Sintim, H. Y., Bary, A. I., Hayes, D. G., Wadsworth, L. C., Anunciado, M. B., English, M. E., et al. (2020). In situ degradation of biodegradable plastic mulch films in compost and agricultural soils. Sci. Total Environ. 727, 138668. doi:10.1016/j.scitotenv.2020.138668
Slepička, P., Trostová, S., Slepičková Kasálková, N., Kolská, Z., Sajdl, P., and Švorčík, V. (2012). Surface modification of biopolymers by argon plasma and thermal treatment: Surface modification of biopolymers. Plasma process. Polym. 9 (2), 197–206. doi:10.1002/ppap.201100126
Soil Degradable, Soil degradable bioplastics for a sustainable modern agriculture; M. Malinconico, Ed.; green chemistry and sustainable technology; Springer Berlin Heidelberg: Berlin, Heidelberg, 2017. doi:10.1007/978-3-662-54130-2
Study of the biodegradation of PLA/PBAT films after biodegradation tests in soil and the aqueous medium. Biointerface Res. Appl. Chem. 2021, 12 (1), 833–846. doi:10.33263/BRIAC121.833846
Su, S., Duhme, M., and Kopitzky, R. (2020). Uncompatibilized PBAT/PLA blends: Manufacturability, miscibility and properties. Materials 13 (21), 4897. doi:10.3390/ma13214897
Sundriyal, P., Pandey, M., and Bhattacharya, S. (2020). Plasma-assisted surface alteration of industrial polymers for improved adhesive bonding. Int. J. Adhes. Adhes. 101, 102626. doi:10.1016/j.ijadhadh.2020.102626
Toro, M., Ramírez-Bahena, M.-H., Cuesta, M. J., Velázquez, E., and Peix, A. (2013). Pseudomonas guariconensis sp. Nov., isolated from rhizospheric soil. Int. J. Syst. Evol. Microbiol. 63 (Pt_12), 4413–4420. doi:10.1099/ijs.0.051193-0
Ungai-Salánki, R., Peter, B., Gerecsei, T., Orgovan, N., Horvath, R., and Szabó, B. (2019). A practical review on the measurement tools for cellular adhesion force. Adv. Colloid Interface Sci. 269, 309–333. doi:10.1016/j.cis.2019.05.005
Urbanek, A. K., Rymowicz, W., and Mirończuk, A. M. (2018). Degradation of plastics and plastic-degrading bacteria in cold marine habitats. Appl. Microbiol. Biotechnol. 102 (18), 7669–7678. doi:10.1007/s00253-018-9195-y
Vesel, A., Mozetic, M., Jaganjac, M., Milkovic, L., Cipak, A., and Zarkovic, N. (2011). Biocompatibility of oxygen-plasma-treated polystyrene substrates. Eur. Phys. J. Appl. Phys. 56 (2), 24024. doi:10.1051/epjap/2011110169
Wilkes, R. A., and Aristilde, L. (2017). Degradation and metabolism of synthetic plastics and associated products by Pseudomonas sp.: Capabilities and challenges. J. Appl. Microbiol. 123 (3), 582–593. doi:10.1111/jam.13472
Yousefi Rad, A., Ayhan, H., Kisa, Ü., and Pişkin, E. (1998). Adhesion of different bacterial strains to low-temperature plasma treated biomedical PVC catheter surfaces. J. Biomater. Sci. Polym. Ed. 9 (9), 915–929. doi:10.1163/156856298X-00244
Zettler, E. R., Mincer, T. J., and Amaral-Zettler, L. A. (2013). Life in the “plastisphere”: Microbial communities on plastic marine debris. Environ. Sci. Technol. 47 (13), 7137–7146. doi:10.1021/es401288x
Zhang, H., Flury, M., Miles, C., Liu, H., and DeVetter, L. (2020). Soil-biodegradable plastic mulches undergo minimal in-soil degradation in a perennial raspberry system after 18 months. Horticulturae 6 (3), 47. doi:10.3390/horticulturae-6030047
Keywords: plasma treatment, mulch films, poly (lactic acid) (PLA), poly(butylene adipate coterephthalate) (PBAT), soil degradation, composting
Citation: Bhattacharya S, Kansara HJ, Lodge J, Diaz CA and Lewis CL (2023) Plasma treatment process for accelerating the disintegration of a biodegradable mulch film in soil and compost. Front. Mater. 10:1232577. doi: 10.3389/fmats.2023.1232577
Received: 31 May 2023; Accepted: 10 July 2023;
Published: 27 July 2023.
Edited by:
Chi-Hui Tsou, Sichuan University of Science and Engineering, ChinaReviewed by:
Evangelia Vouvoudi, Aristotle University of Thessaloniki, Greece
Rossella Arrigo, Polytechnic University of Turin, Italy
Copyright © 2023 Bhattacharya, Kansara, Lodge, Diaz and Lewis. This is an open-access article distributed under the terms of the Creative Commons Attribution License (CC BY). The use, distribution or reproduction in other forums is permitted, provided the original author(s) and the copyright owner(s) are credited and that the original publication in this journal is cited, in accordance with accepted academic practice. No use, distribution or reproduction is permitted which does not comply with these terms.
*Correspondence: Christopher L. Lewis, Y2xsbWV0QHJpdC5lZHU=,
†ORCID: Christopher L. Lewis orcid.org/0000-0002-5728-9699;