- Department of Chemical Engineering and Materials, Universidad Complutense de Madrid, Madrid, Spain
Cellulose nanomaterials present unique properties of interest for their many applications. Their behavior is mainly related to the colloidal properties that determine their self-assembly and stability mechanisms as well as their interaction with the particles present in different matrices. This review shows the state of the art of nanocellulose from a colloidal material perspective, from the approach of how to control these properties and their influence on the final products, such as drug delivery, coatings, nanocomposites, or tissue engineering, emphasizing the role of colloidal behavior in determining their performance.
1 Introduction
Cellulose nanomaterials or directly named nanocellulose (NC), derived from renewable resources such as wood, plant-based cellulose as well as from wastes, both from forest and agro-industries, have emerged as a fascinating nanomaterial due to its remarkable properties and numerous applications in various fields (Blanco et al., 2018; Pennells et al., 2020). NC was first discovered in the late 20th century, the initial breakthrough occurred in the 1980s when researchers discovered that cellulose could be broken down into nanoscale dimensions (Turbak et al., 1983). However, it was not until the 1990s that the term “nanocellulose” was coined to describe these nano-sized cellulose particles (Klemm et al., 2011; Dufresne, 2013).
NC products exhibit unique properties, including high mechanical strength, large surface area, colloidal behavior, low density, and biodegradability, making an attractive set of materials for various industries (Salas et al., 2014; Rahman et al., 2023). The development of knowledge to tackle the challenges in their production, characterization and application has focused the attention of researchers in the last 3 years. According to Web of Science, between 2020 and 2022, 9,559 papers have been published on NC, cellulose nanocrystals (CNCs) and cellulose nanofibrils (CNFs). Among them, around 5,900 consider their properties, more than 1,300 are focused on their production, around 4,400 refers to the different application, more than 1,100 are directly related to the products obtained and 1,100 discuss characterization methodologies. Although 3,400 papers are published in the category of Polymer Science, only 21 are directly based on interfacial and colloidal properties of NC. Nevertheless, numerous authors have considered the interface and colloidal properties of NC products to improve the production processes or with the aim of optimizing their characteristics and facilitating their application in various fields (Zhu M. et al., 2021; Teo et al., 2022). Currently, there is a great fragmentation of knowledge on the colloidal behavior of NC products and therefore a need of a research integration and synthesis to provide an important step in the scientific process.
In this paper, we review the state of the art of NC from a colloidal material perspective, focusing on its colloidal characteristics and behavior which affect their self-assembly capacity, stabilization mechanisms, and interaction with other particles. Discussion provides an integrated, synthesized overview of the current state of knowledge on the factors influencing the colloidal stability of NC dispersions, the strategies employed to control these properties and their influence on the final applications of the NC dispersions, including a wide number of fields such as drug delivery, coatings, nanocomposites, or tissue engineering, emphasizing the role of colloidal behavior in determining their performance.
2 Colloidal behavior of nanocellulose
Colloidal particles are defined as microscopic particles with a dimension scale ranging from nanometers to micrometers. More broadly, can be defined as any substance, including films or fibers, with at least one dimension in this range and may exist as single materials or dispersions of one substance in a fluid phase (Lu and Weitz, 2013; Lee, 2018). Within the later, NC particles, typically in the form of CNC or CNF suspensions, exhibit colloidal behavior due to their nanoscale dimensions (Xu et al., 2020). The small size and large surface area-to-volume ratio of NC particles lead to their exceptional stability and reactivity (Shahzad et al., 2023). According to ISO 2017 20,477 standard, these particles, which mainly differ from each other in the presence of amorphous regions in CNFs, display a high aspect ratio in both cases (Figure 1). In addition, the introduction of repulsive charges that facilitates the isolation of individualized crystals or fibers provides them unique colloidal properties (Heise et al., 2022).
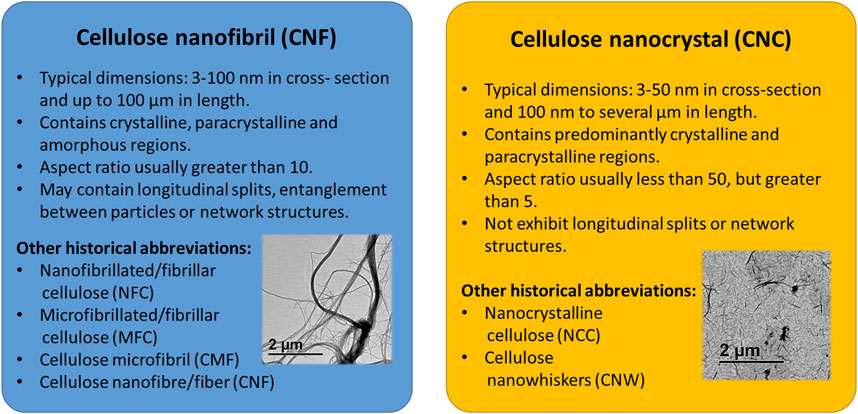
FIGURE 1. Differences between cellulose nanofibrils and cellulose nanocrystals (Adapted from ISO standard 20477).
NC is often produced in the form of colloidal suspensions, where individual NC particles are dispersed in a liquid medium, such as water (Solhi et al., 2023). High surface area of NC allows for more contact points with the matrix material in paper and composites, enhancing the reinforcing effect (Perdoch et al., 2022; Seydibeyoğlu et al., 2023). It facilitates improved stress transfer between the NC and the matrix, resulting in enhanced mechanical properties such as increased tensile strength, modulus, and toughness. Furthermore, a higher surface area promotes a greater interfacial area for effective bonding between the NC and the matrix material. This improved interfacial interaction enhances load transfer and dispersion of stress, leading to enhanced reinforcement in the composite system. The colloidal stability of NC suspensions ensures that these products remain well-dispersed (repulsive forces higher than attractive ones, as shown in Figure 2) avoiding aggregation and sedimentation over time (Nordenstrom et al., 2017). When this stability is altered (attractive forces > repulsive forces), NC particles aggregate or flocculate and their surface area and accessibility decrease, leading to reduced inter and intra interactions between themselves and with other materials reducing their reinforcement and barrier properties.
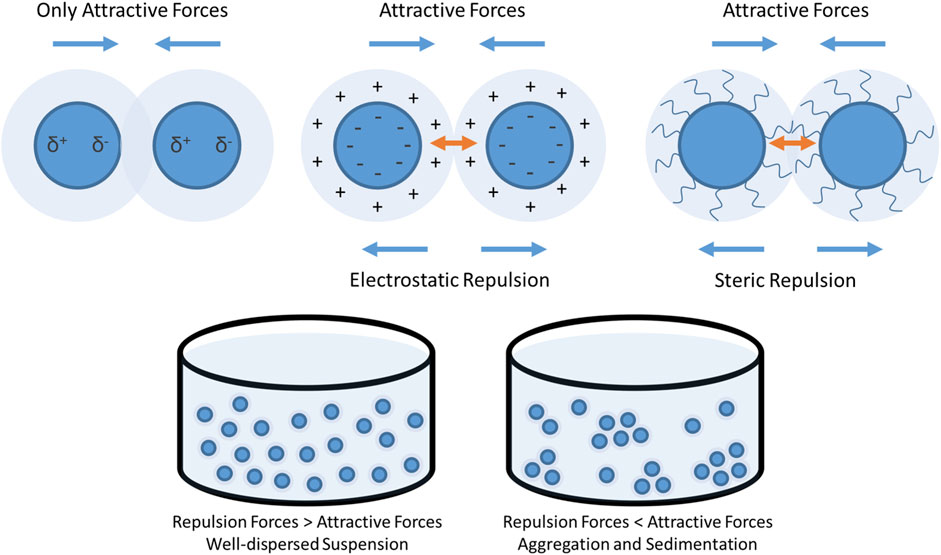
FIGURE 2. Stabilization mechanisms of nanoparticles: Force balancing of attractive forces (Van der Waals interactions and hydrogen bonds) with and without repulsion. Balances between attractive and repulsive forces [Adapted from Matter et al. (2020)].
The concentration of reinforcement particles reaches saturation due to two main factors: the frailty of the reinforcement constituent and the surface compliance between the matrix and the filler (Alagarsamy et al., 2023). The frailty of the reinforcement constituent refers to its limited stability. As more reinforcement particles are incorporated into the matrix, the overall structural integrity of the composite material increases. However, there is a limit to the number of NC particles that can be effectively dispersed and integrated without compromising the reinforcement’s mechanical properties. Once the concentration of particles surpasses this limit, the reinforcement constituent becomes more susceptible to breakage or deformation, reducing its effectiveness in enhancing the material’s strength. On the other hand, the surface compliance between the matrix and the reinforcement material also plays a crucial role in the saturation of reinforcement concentration. In composite materials, the matrix and the NC interact at their interface. Ideally, the NC should be uniformly distributed and strongly bonded to the matrix to maximize the transfer of stress and load between the two components. However, as the concentration of NC increases, it becomes more challenging to maintain this ideal interaction. Agglomeration or poor bonding between the matrix and NC may occur, leading to stress concentrations and weak points in the material. This limits the ability to further increase the concentration of reinforcement particles.
Surface area also affect the barrier properties of NC films or coatings that are attributed to the existence of dense networks with small pore sizes with tortuous path created by the interconnected NC fibers, which slows down the diffusion of gases and liquids (Ilyas et al., 2022; Aigaje et al., 2023). The accessibility of NC refers to its ability to interact with gases, liquids, or particles that attempt to pass through it. This accessibility influences the effective blocking of molecules or particles, contributing to improved barrier performance creating a barrier against water vapor transmission and other gases.
In summary, colloidal properties are crucial for the successful utilization of NC products. Stable NC dispersions facilitates the uniform and homogeneous mixing with other materials, leading to improved processability and product quality (Chu et al., 2020). Furthermore, stable dispersions also enable consistent and predictable behavior during processing, storage, and application of NC-based products. Colloidal stability plays a crucial role in maintaining the integrity and functionality of these products over extended periods. Stable dispersions of NC minimize the risk of phase separation, precipitation, or irreversible aggregation, ensuring the long-term performance and stability of the final products. This is key, for example, in biomedical applications, as unstable NC dispersions can lead to unpredictable behavior and potentially adverse effects on cells, tissues, or organisms. Stable and well-characterized NC suspensions are crucial for ensuring biocompatibility and minimizing any potential toxicity concerns (Joseph et al., 2020).
2.1 Stabilization mechanisms
NC particles behave as colloids dispersed in a medium, and the repulsion between them can be described by the concept of colloidal stability. Colloidal stability is based on the DLVO theory in which the equilibrium between repulsive and attractive forces are determined by several factors, including electrostatic repulsion, steric repulsion, and van der Waals forces (Figure 2). All these processes determine the stability of CNC dispersions and the 3D network stability of CNFs in which the importance of gravity and inertia decreases (Benselfelt et al., 2023). Therefore, they determine the final efficiency of the NC products in their different applications.
Electrostatic repulsion arises from the presence of charged groups in the NC. If all particles have the same charge, they repel each other due to electrostatic forces. This repulsion can be enhanced by increasing the charge density on the NC surface (de Souza et al., 2020) or by introducing ions with like charges into the surrounding medium, creating a double layer of ions around the colloidal particles that increased their zeta potential. Ionic strength of the medium has a significant effect on colloidal stability. Lower ionic strength can enhance colloidal stability. When the ionic strength is low, there are fewer ions present to shield the charged surface of colloidal particles. This allows for stronger electrostatic repulsion, preventing particle aggregation and maintaining colloidal dispersion. Conversely, high ionic strength leads to higher concentrations of ions in the surrounding medium, which can shield the charged surface of the colloidal particles, diminishing the repulsive forces that keep them dispersed. The reduced electrostatic repulsion allows for attractive forces, such as van der Waals interactions, to dominate, causing colloidal particles to aggregate or flocculate. This aggregation leads to the formation of larger and more settled particles, resulting in the destabilization and eventual precipitation or sedimentation of the colloidal system.
Steric repulsion, also known as steric hindrance, occurs when polymer chains or other surface-active molecules adsorb onto the colloid’s surface, creating a physical barrier that prevents particles from approaching each other closely (Van De Ven and Sheikhi, 2016; Tardy et al., 2017). This steric layer can hinder particle aggregation and maintain the NC colloidal stability (Raj et al., 2017). The strength of steric repulsion depends on factors such as the length, flexibility, and density of the polymer chains, as well as the surface coverage of the steric stabilizer. Higher polymer chain lengths, greater chain flexibility, and higher surface coverage generally enhance the steric repulsion and improve colloidal stability. NC particles can be also used as steric hindrance of other compounds, such as in the production of Pickering Emulsions in which NC play the role of stabilizer (Saffarionpour, 2020; Li et al., 2022). Steric repulsion is particularly effective in stabilizing colloidal systems against destabilizing forces, such as electrostatic attractions or van der Waals forces. Unlike other stabilization mechanisms, steric repulsion can operate independently of the electrolyte concentration or pH of the system, making it more robust and versatile.
Van der Waals forces are attractive forces that arise from temporary fluctuations in the electron distribution of particles and act at longer distances than electrostatic forces. While these forces are typically attractive, at very close distances, they can become repulsive due to overlapping electron clouds. This repulsion is known as the van der Waals repulsion and acts as a repulsive barrier preventing particles from coming into direct contact.
Hydrodynamic effects, risen from the relative motion between the particles and the surrounding fluid, as also key factors in the stabilization of suspensions (Gustafsson et al., 2018; Matter et al., 2020). Brownian motion is the random movement of particles in a fluid due to collisions with surrounding molecules. It arises from the thermal energy of the system. The continuous and erratic motion of particles and solvent molecules helps to counteract the attractive forces between particles, thereby preventing their aggregation and settling. Moreover, Brownian motion also aids in the stabilization of colloids by enhancing the rate of diffusion. The rapid and random movement of particles allows for efficient mixing and interaction between particles, leading to the equal distribution of particles throughout the medium. The random collisions with the molecules of the surrounding fluid generate a drag force, also known as the viscous drag or Stokes drag, which opposes the motion of the particles. The drag force depends on the size, shape, and velocity of the particles, as well as the viscosity of the fluid. The drag force tends to hinder the motion of colloidal particles, causing them to settle or aggregate over time if left undisturbed, leading to destabilization of the colloidal system. Hydrodynamic interactions between particles clearly affect their stability since the motion of particles induces fluid flows that can create attractive or repulsive forces. Therefore, hydrodynamic interactions can either promote particle aggregation or hinder it, depending on factors such as particle concentration and fluid flow conditions. Furthermore, hydrodynamic forces are involved in processes such as sedimentation and diffusion, which also alter the stability of NC systems. The rate of sedimentation depends on the particle size, density, and the viscosity of the fluid and favors destabilization while, one the other hand, diffusion driven by the random motion of particles due to thermal energy, can lead to the redistribution of particles in the system.
There are many factors that determine the stabilization of NC suspensions over time, and this is a key parameter for its application. However, it was only recently when researchers demonstrated that the dispersion of NC suspensions before its use is of critical importance for the successful application of these products (Balea et al., 2017; Campano et al., 2018). In the case of fibrillated NC low dispersion of fibers produce agglomerates with a reduced effect as reinforcement additives while high dispersion may break down and collapse the 3D network, reducing also the product efficiency (Sanchez-Salvador et al., 2022). The optimal openness of the 3D network depends on the final applications (Figure 3). This has been studied for the use of CNF as reinforcement of paper and for the application of coating formulations (Sanchez-Salvador et al., 2023).
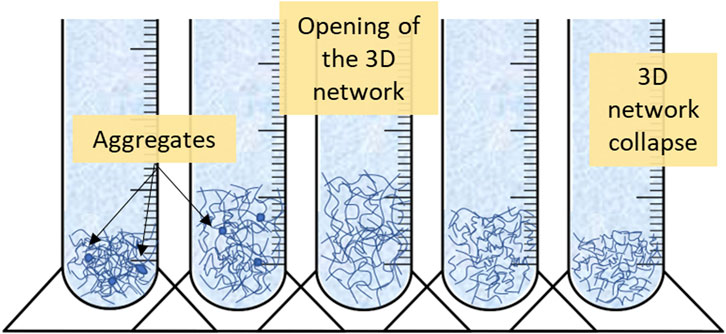
FIGURE 3. Representations of fibril network in the deposit of the graduated cylinders at increasing agitation speeds [Adapted from Sanchez-Salvador et al. (2022)].
2.2 Factors that influence nanocellulose stabilization
The stabilization of NC materials involves several factors, both intra and interrelated. These factors can influence the overall stability and performance of NC materials. Key intrarelationships are related to NC source (CNFs or CNCs), since it affects its intrinsic properties and stability; surface charge and surface functionalization can enhance stability by introducing chemical groups or coatings to mitigate aggregation and improve compatibility with specific applications; medium characteristics, as pH and ionic strength, by influencing surface charge, electrostatic interactions, and hydration forces; or dispersion. On the other hand, between the interrelations are important the solvent; temperature and dry methods in case of CNCs; dispersion or flocculation additives; and environmental factors, such as humidity and exposure to UV radiation. It is important to note that these factors are all connected, and their combined effects determine the overall stability of NC. Researchers and users must consider these factors collectively to optimize the stabilization of NC materials for various applications (Van De Ven and Sheikhi, 2016).
2.2.1 Surface Charge
The surface charge of NC particles affects their stability. NC can acquire a surface charge through various methods, such as chemical modification or pH adjustment (Table 1). The presence of charged groups on the surface can lead to electrostatic repulsion, enhancing stability by preventing particle aggregation. In general, NC presents hydroxyl groups on the surface that enable strong hydrogen bonding interactions between NC particles, leading to the formation of networks or hierarchical structures. However, it is also possible to cationize the products by direct cationization, etherification, esterification, adsorption, among others. In this way the compatibility and interaction of NC with various systems, such as polymers or biomolecules, is enhanced.
Cationization is one way of functionalized NC to enhance its properties and expand its range of application. It involves the direct chemical modification of anionic NC using cationic agents, such as quaternary ammonium salts, polyethyleneimine, or other polymers. The cationic agents interact with the hydroxyl groups on the cellulose surface increasing the positive charges, by a non-covalent kind of cationization following a reversible adsorption-desorption process (Aguado et al., 2022). The rate of adsorption and desorption of cations can depend on factors such as concentration, pH, temperature, and the nature of the cation and NC surface. In most cases the kinetics follow pseudo-second order kinetics, indicating that the intra-particle diffusion through the fiber is the rate-controlling step (Ranjbar et al., 2020). However, specific kinetic parameters vary depending on the experimental conditions, the specific NC material, and the cationic polymer studied.
Etherification involves the introduction of ether groups onto NC through the reaction between cellulose hydroxyl groups and cationic reagents, such as epoxides, alkyl halides or alkyl sulfates. The reaction typically occurs under alkaline conditions to facilitate the substitution of hydroxyl groups with ether linkages. The alkylating agent replaces the hydrogen atom of the hydroxyl group, resulting in the formation of an ether bond. The degree of etherification can be controlled by adjusting the reaction conditions, such as reaction time, temperature, and the concentration of alkylating agent (Gomri et al., 2022; Noremylia et al., 2022). In esterification, NC is reacted with cationic reagents, such as fatty acids or anhydrides, to form cationic esters. The reaction occurs between the hydroxyl groups of cellulose and the carboxyl groups of the cationic reagents (Noremylia et al., 2022).
2.2.2 Electrolyte concentration
The presence of electrolytes in the solution influence colloidal stability. High concentrations of electrolytes can screen the electrostatic repulsion between NC particles, leading to particle aggregation (Blanco et al., 2001). The type and concentration of electrolytes must be considered to maintain stability. The critical aggregation concentration follows the Schulze–Hardy law, i.e., the critical aggregation concentration decreased with increasing counterion valence. For the CNCs and monovalent ions, the critical concentration follows the trend Li+ > Na+ > K+ > Cs+ (Phan-Xuan et al., 2016). This is especially important when NC is used in closed water circuits such as in the production of paper and board since the high conductivity of the medium will reduce the effectiveness of the NC. Furthermore, in some cases it may destabilize the suspensions and break down the 3D network in the case of CNF.
2.2.3 Solvent and pH
The solvent and pH conditions can also affect the stability of NC dispersions. Different solvents can interact differently with the NC surface, affecting the colloidal stability. NC is well-dispersed in strong polar solvents due to the interaction between the surface hydroxyls and solvent molecules. The most used one is the water. On the other hand, the NC hydrophilicity makes difficult to disperse it in hydrophobic media, such as low polar and nonpolar organic solvents (Chu et al., 2020). Changes in pH can alter the surface charge of NC particles, affecting their stability and interactions with other particles or additives in a suspension (Aoudi et al., 2022). At specific pH values, the surface charge of NC particles can be neutralized or reversed, leading to flocculation or aggregation. At low pH values, the surface charge of the NC particles may become more positive due to protonation of the surface groups. This increased positive charge can reduce the electrostatic repulsion between nearby particles, leading to the formation of larger aggregates and flocs. On the other hand, at higher pH values, the surface charge of the NC particles can become more negative due to deprotonation of surface groups. This increased negative charge can enhance the electrostatic repulsion between particles, preventing flocculation and maintaining a stable dispersion.
The pH dependence of NC flocculation can be utilized for various applications. Adjusting the pH can control the dispersion or aggregation of NC, enabling the formation of desired structures or the separation of NC from a suspension. Additionally, pH-responsive additives can be used to enhance flocculation or stabilization properties under specific pH conditions. Therefore, understanding the pH conditions are crucial for optimizing NC flocculation processes and tailoring the properties of NC-based materials.
2.2.4 Addition of polymers
Polymer additives, such as surfactants or polymers, can be used to provide steric stabilization. These additives adsorb onto the NC surface, creating a protective layer that prevents particle aggregation by steric hindrance (Tardy et al., 2017). On the contrary, the addition of anionic/cationic polymers can produce the opposite effect, the flocculation of the NC particles, mainly governed by the charge density, forming aggregates with the polymer added (Raj et al., 2017; Balea et al., 2019b).
The effect of polyelectrolyte depends on its morphology, charge, molecular weight and on the surface charge of the NC. In general, the maximum surface coverage by a polyelectrolyte vary inversely to polymer charge density and is independent of polymer molecular weight and morphology. Properties of the soft colloid flocs (size, strength, and reformation) can be controlled by the polyelectrolyte charge density and concentration as well as by the shear forces. Different flocculation mechanisms are possible as neutralization, patching and bridging which form a self-supporting fibrous network of fibrillated cellulose (Figure 4). Some flocculants present different mechanism as a function of their molecular weight and charge like in the case of poly (diallyldimethylammonium chloride (pDADMAC). These mechanisms may change over time and depends on shear conditions. Reflocculation is possible when soft flocs are formed as in the case of neutralization and patching while only partial flocculation occur when bridging is the primary flocculation mechanism. Polysalts as polyaluminum chloride flocculates by compressing the colloidal electrical double layer, with small and dense flocs that do not form a 3D network. Polymers of high charge along their molecular structure attach themselves to the negatively charged particles, forming “patches” on the particle surfaces, as in the case of polyethylenimine (PEI). If 50% of the particle surface is covered the flocculation with other particles will be optimal for neutralization. Flocs are compact and soft easy to break down by shear forces but with the possibility of reflocculating when the shear forces decrease. Polymers of high molecular weight, like polyacrylamides (PAM), adsorb onto the surfaces of particles and extend into the surrounding solution, forming long loops and chains. These chains go beyond the Debye length or distance where the electrostatic interactions between charged particles are significant. They interact with other particles, linking them together by bridging which facilitating the formation of large and hard flocs, difficult to break down but with lower reflocculation capacity. With time these polymers may reconform with shorter and flatter loops (Cadotte et al., 2007; Raj et al., 2017).
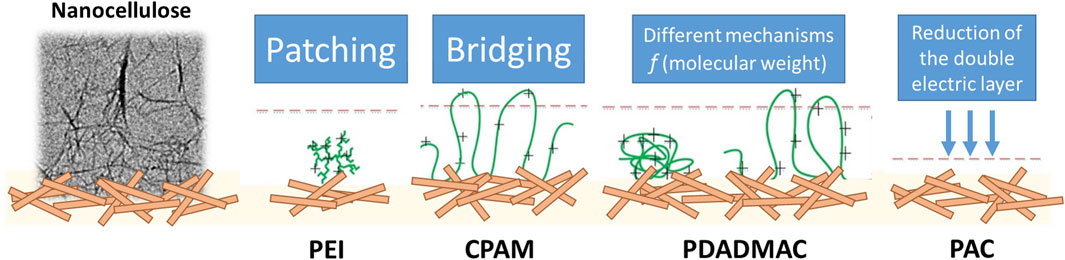
FIGURE 4. Main flocculation mechanisms of different polyelectrolytes (Adapted from (Raj et al., 2017)).
2.2.5 Mechanical shear
Mechanical shear can have both positive and negative effects on NC stability, depending on the specific circumstances. On the positive side, moderate mechanical shear can improve the dispersion and homogeneity of NC in a liquid medium, such as water or a polymer matrix. This can enhance the stability of NC suspensions or composites by preventing the formation of aggregates or sediments. Shear forces can break down large NC aggregates into smaller aggregates and individual particles, leading to improved rheological properties and increased stability. However, excessive, or prolonged mechanical shear can have detrimental effects on NC stability. Intense shear forces can cause the breakage or degradation of NC fibers, resulting in a reduction in their length and overall properties as shown by Sanchez-Salvador et al. (2022). This can lead to decreased stability, loss of mechanical strength, and changes in the structural integrity of NC-based materials. It is crucial to carefully control and optimize the shear conditions to balance the dispersion and stability of NC. By understanding the specific requirements of the NC material and application, it is possible to define appropriate shear conditions to maximize the benefits of mechanical shear while minimizing potential negative impacts on nanoparticles and on stability.
2.2.6 Temperature
Changes in temperature can impact the stability of NC dispersions. Low temperatures can decrease the mobility of particles, reducing the likelihood of aggregation and enhancing stability. Conversely, high temperatures increase the kinetic energy of particles, resulting in more vigorous Brownian motion which enhances collisions and aggregation. In some cases, increasing temperature can enhance the solubility of certain components, leading to changes in the colloid’s properties. This effect is particularly relevant in systems involving temperature-sensitive phase separation or aggregation, such as certain polymer-based composites. Temperature also influences the viscosity of both the dispersing medium and the colloidal system. Generally, temperature increases lead to a decrease in viscosity, which can affect the flow behavior and stability of the NC suspensions. Changes in viscosity can impact processes such as sedimentation, diffusion, and coagulation. Generally, NC exhibits increased stability at lower temperatures. High temperatures can lead to various degradation mechanisms, such as thermal decomposition and chemical reactions, which can negatively impact the structure and properties of NC. These degradation processes can result in a loss of mechanical strength, changes in particle size and morphology, and alterations in the surface chemistry of NC. Temperature may also induce phase transitions in defibrillation processes to produce NC gels. It is important to consider the temperature conditions during storage, processing, and application of NC to ensure its stability and preserve its desired characteristics.
2.3 Self-assembly and aggregation behavior
NC self-assembly refers to the spontaneous organization of NC particles into larger structures through non-covalent interactions. This self-assembly and aggregation behavior is due to its unique structural and physicochemical properties associated to its rod-like or fibrillar shape, high aspect ratio, and the presence of hydroxyl groups on its surface (Smalyukh, 2021). The process is driven by various forces, including van der Waals forces, hydrogen bonding, electrostatic interactions, and hydrophobic interactions. These forces cause the NC particles to align and arrange themselves in specific orientations, leading to the formation of hierarchical structures. The presence of hydroxyl groups enables strong hydrogen bonding interactions between particles, leading to the formation of the 3D networks. The self-assembly process can be influenced by factors such as surface modifications, concentration, pH, temperature, and the presence of additives (Wang et al., 2019). The combination of NC and polyelectrolytes enables the formation of dense films with excellent mechanical properties and with tailored optical properties (Wågberg and Erlandsson, 2021). CNCs and CNFscan be effectively formed Layer-by-Layer (LbL) structures together with cationic polyelectrolytes (Karabulut and Wågberg, 2011). These LbL structures exhibit a highly precise and well-defined architecture, and the resulting layers possess distinct structural colors when exposed to diffusely reflected white light, thus enabling to create different structural colors depending on the number of layers (Wgberg et al., 2008). Moreover, the incorporation of NC and PEI in LbL films provides an exceptional approach to produce mechanically durable coatings. For example, thin films of CNF/PEI had a Young’s modulus of ≈4 GPa at 64% RH, 12 GPa at 40% RH and 6 GPa at 30% RH (Kan and Cranston, 2013).
NC aggregation refers to the clustering or association of these NC particles to form aggregates. Aggregation can occur through reversible or irreversible mechanisms depending on the specific conditions. Reversible aggregation can be induced by changes in environmental factors such as pH, ionic strength, or temperature. It often leads to the formation of reversible networks, gels, or liquid crystalline phases. Irreversible aggregation, on the other hand, involves the formation of permanent structures or irreversible precipitation, which can limit the material’s functionality. An instance of a challenge is the irreversible aggregation that occurs during the drying process of NC, commonly referred to as hornification (Benselfelt et al., 2023). This phenomenon poses difficulties in the efficient transportation of NC as it requires them to be in a dispersed state containing over 90% water. Despite centuries of discussion, no definitive explanation for the mechanism of hornification has been established. Then, it is crucial to invest more efforts in comprehending hornification and finding preventive measures as it would greatly benefit the pulp and NC industries. In the case of CNFs prepared using the TEMPO/NaBr/NaClO reaction, an additional factor contributing to irreversible aggregation during drying is the formation of interfibrillar hemiacetals/hemiketals. These compounds arise from the reaction between aldehydes/ketones and hydroxyl groups and further promote the aggregation process. To mitigate this effect, the content of aldehydes and ketones can be decreased by employing NaBH4 reduction which minimizes the formation of interfibrillar bonds. Hot water (80 °C) in alkaline conditions can break the interfibrillar bonds. Additionally, oxidation of aldehydes using NaClO2 provides a slight improvement in redispersibility, but irreversible aggregation still takes place due to the presence of remaining ketones (Benselfelt et al., 2023).
In the case of functionalized cellulose, the presence of different charged groups on the NC surface can lead to electrostatic repulsion or to attraction between particles, affecting their aggregation behavior. The addition of charged groups to the surfaces of CNFs offers several advantages. It allows for the creation of stable dispersions at the colloidal level and improves the ability of the CNFs to disperse again after drying. The positive effect of charges on redispersibility can be attributed to two factors: the osmotic effect, which increases the force for swelling and aids in dispersion, and the steric effect caused by the charged groups, which prevents aggregation. Generally, the higher the surface charge density, the better the CNFs can regain their original properties after redispersion (Nordenstrom et al., 2021). On the other hand, the introduction of sterically stabilizing component by covalent grafting on the surface (Wen et al., 2017; Kaldéus et al., 2018) can form steric hindrance and increase the interface compatibility and the redispersability. However, the use of covalent grafting is often hindered by their difficulty of implementing on a large scale production and the irreversible changes to the surface properties of CNFs (Nordenstrom et al., 2021). Furthermore, the interaction between NC and other components in a system, such as polymers or other nanoparticles, can also influence aggregation. Therefore, the strategy of using redispersing agents such as polyethylene glycol, glycerol, cetyltrimethylammonium bromide, polyvinyl alcohol, maltodextrin, gum arabic or lignin, to circumvent the irreversible CNF association has been deeply reported (Vigneshwaran et al., 2011; Campano et al., 2018; Benselfelt et al., 2023).
The resulting final aggregates can exhibit different sizes, shapes, and structures depending on the specific conditions and properties of the NC material. Thus, the self-assembly and aggregation behavior of NC have significant implications for various applications. These properties can be harnessed to create materials with tailored structures and functionalities, such as films, coatings, gels, and composites. The ability of NC to form stable networks and gels has been exploited in areas like tissue engineering, drug delivery, and food science. Additionally, understanding and controlling the self-assembly and aggregation behavior of NC can contribute to the development of sustainable and renewable materials with improved mechanical, barrier, and optical properties.
3 Characterization of nanocellulose colloids
NC colloids are characterized by their unique properties and behavior at the nanoscale. Therefore, characterizing the colloidal properties of NC is essential for understanding its behavior and optimizing its applications. Techniques such as light scattering, electron microscopy, zeta potential measurements, and rheological analysis are commonly employed to determine particle size, size distribution, surface charge, and rheological properties of NC suspensions. These characterization techniques provide valuable insights into the stability and reactivity of NC colloids. Some key aspects that can be measured to characterize NC are the following.
3.1 Size, morphology and concentration
NC colloids typically have a rod-like or fibrillar shape, with dimensions ranging from a few nanometers to micrometers in length and a few nanometers in width. The size and morphology can be determined using advanced microscopic techniques such as transmission electron microscopy (TEM), atomic force microscopy (AFM) or scanning electron microscopy (SEM). Microscopic techniques are time-consuming and require the preparation of suitable samples on specialized supports or grids, which involves a drying step. Unfortunately, during the drying process, NC particles can aggregate, leading to larger particle sizes. Therefore, scattering techniques (neutron, X-ray, and light) can be used to determine the dimensions of NC, from nanoscale to submicroscale, in the dispersion state (Mao et al., 2017). Although small-angle neutron scattering (SANS), small-angle X-ray scattering (SAXS) have been used to determine statistically averaged dimensions of individual NC, light scattering techniques are more commonly employed for this purpose. This last type can be measured in terms of fluctuations in the intensity of the light scattering measurement, such as static or dynamic light scattering (SLS/DLS) or as a function of the scattering angle in the case of laser diffraction spectroscopy (LDS) (Bhattacharjee, 2016; Li et al., 2019). The technique of SLS requires a high level of sample cleanliness, as even slight contamination by foreign dust present in the solution can greatly affect the accuracy of measurements. This is because SLS measures the averaged intensity of scattered light, which scales approximately with the sixth power (∼r^6) of the particle diameter (assuming spherical particles). Therefore, it is crucial to ensure a dust-free sample before conducting SLS measurements, typically achieved through filtration. However, this cleaning method is not usually suitable for NC dispersions containing highly asymmetric particles. On the other hand, DLS is more feasible for analyzing less clean samples since it focuses solely on particle dynamics, where dust particles and nanoparticles exhibit significant differences (Mao et al., 2017). Nevertheless, the techniques based on light scattering provide the size information in terms of equivalent spherical diameter, assuming that the material is similar to spherical particles, so these techniques can be used to compared different samples, mainly in the fibrous particles with a greater deviation from sphericity (Gamelas et al., 2015).
The self-assembled and aggregated structures of NC is also determined by TEM and SEM to visualize the hierarchical structures at various length scales. X-ray diffraction (XRD) provides information about the crystallinity and orientation of NC assemblies. Furthermore, spectroscopic techniques such as infrared spectroscopy (FTIR) and nuclear magnetic resonance (NMR) spectroscopy aid in understanding the intermolecular interactions and chemical changes during self-assembly and aggregation processes (Asad et al., 2018).
Concentration of the NC particles is calculated by gravimetric analysis or thermogravimetric analysis (TGA), this traditional technique involves drying and weighing a known volume of NC suspension before and after evaporation of the solvent. The difference in weight provides the NC concentration (Jordan et al., 2019). Another simple technique is turbidity, that allow to quantify the light scattering or absorption caused by the suspended NC particles can provide a reliable measure of concentration (Foster et al., 2018). However, turbidity measurements are sensitive to particle size, and variations in NC particle size may affect the accuracy of concentration determination. It is essential to ensure consistent particle size distribution to obtain reliable turbidity results. Another technique is dynamic light scattering (DLS) which measures the intensity of scattered light caused by particle Brownian motion (Bhattacharjee, 2016). The diffusion coefficient is related to the size and concentration of the particles through the Stokes-Einstein equation. By comparing the measured diffusion coefficient with a calibration curve obtained using standard samples of known concentration, the concentration of NC can be accurately determined.
3.2 Chemical composition
The chemical composition of NC colloids can be analyzed to determine the purity and content of cellulose, hemicellulose, lignin, and other impurities. Carbon hydrogen nitrogen sulfur elemental analysis (CHNS EA) is used to characterize the chemical composition of NC in three main cases (Foster et al., 2018): 1) CNC produced from sulfuric acid hydrolysis because residual sulfur groups are easily identifiable; 2) functionalized NC with groups containing N or S and 3) to measure the degree of chemical leaching in polymers containing NC when the leachate contains C, H, N, S, or O. Other techniques such as Fourier transform infrared spectroscopy (FTIR), X-ray photoelectron spectroscopy (XPS), or solid-state nuclear magnetic resonance (NMR) spectroscopy can provide information about the chemical bonds and functional groups present in the colloid (Asad et al., 2018).
3.3 Surface charge
The surface charge of NC colloids plays a crucial role in their stability and interactions with other particles or materials (Hajian et al., 2017). The surface charge is commonly characterized by measuring the zeta potential. This technique determines the electric potential at the particle’s surface in a solution, providing insights into its stability and electrostatic interactions. By employing techniques such as electrophoretic mobility or laser Doppler velocimetry, or streaming current potential, zeta potential measurements enable the determination of the surface charge of NC (Raj et al., 2016).
Since NC has functional groups, such as carboxyl and hydroxyl groups, that dissociate and contribute to its surface charge. By varying the pH and monitoring the corresponding surface charge, the isoelectric point (IEP) of NC can be determined. The IEP represents the pH at which the NC surface has not net charge. On the other hand, conductometric titration involves the gradual addition of a charged titrant to a NC suspension while monitoring the change in its electrical conductivity. The point of zero charge (PZC) is determined when the conductivity remains constant, indicating that the NC surface has reached a neutral state. This technique provides valuable information about the NC’s surface charge behavior and its interactions with ions in solution (Wu et al., 2021).
The surface charge of NC also influences the rheological properties of the suspension, particularly at the percolation threshold which is identified as the concentration wherein there is no double yielding behavior (Mendoza et al., 2018a). At percolation threshold, increasing the surface charge of NC from 0.65 to at least 1 mmol per gram leads to higher viscosity due to stronger electrostatic interactions. However, at higher concentrations, the length of the NC and surface charge have minimal impact, and the gel’s dynamic rheological properties (G′ and G″) are primarily influenced by the NC concentration (Mendoza et al., 2018b). These findings suggest that NC gels can exhibit similar properties, regardless of the pulp source and with minimal surface charge, at fiber concentrations above the percolation threshold.
3.4 Mechanical properties
The mechanical properties of NC-based materials are highly dependent on their colloidal properties. The colloidal properties of NC, such as its surface area, aspect ratio, surface charge, and alignment, play a crucial role in determining its mechanical properties (Benselfelt et al., 2023). For instance, NC particles with high aspect ratios and high surface areas can lead to materials with improved mechanical properties such as higher strength and toughness. Similarly, high surface charge can affect the colloidal stability of the suspensions, which is essential for the formation of homogeneous NC-based composites. The degree of alignment and orientation of the NC particles is another important factor that can impact mechanical properties such as stiffness, elasticity, and tensile strength.
Mechanical characterization of NC has gained significant attention due to its potential applications in various fields such as materials science, biomedical engineering, and electronics. Mechanical properties can be evaluated using techniques like tensile testing or nanoindentation. Mechanical properties, including high strength, stiffness, and toughness (Fukuzumi et al., 2013). However, measuring these properties at the nanoscale presents numerous challenges associated to homogeneity of the suspensions, surface effects and potential sample deformation during measurements and environmental conditions. Macroscopic mechanical properties, such as tensile strength, elastic modulus, and strain at failure, can be determined by subjecting NC films or fibers to tensile tests. However, challenges arise due to sample preparation and alignment issues (Fukuzumi et al., 2013). The viscoelastic behavior of materials under dynamic loading can be measured by dynamic mechanical analysis (DMA), providing valuable information about their mechanical and thermal properties. By subjecting NC to a range of temperature and frequency variations, DMA enables the characterization of parameters such as storage modulus, loss modulus, and damping factor. These measurements aid in understanding NC’s response to temperature changes, its structural integrity, and its potential for applications requiring mechanical stability over a wide temperature range (Kajtna and Šebenik, 2017). Through DMA, Dominic et al. (2020) observed that a composite containing 5 wt% of rice husk-NC and 25 wt% carbon black exhibited a lower loss tangent (tan δ) at 60°C compared to a composite containing 30 wt% carbon black alone. This finding suggests that the presence of rice husk-NC contributes to reduce rolling resistance, a critical parameter in the context of tire applications.
On the other hand, the use of atomic force microscopy (AFM) is widely used to investigate the surface topography and mechanical properties of NC. Force-distance spectroscopy and nanoindentation techniques provide valuable information about Young’s modulus, adhesion, and stiffness (Lahiji et al., 2010).
3.5 Rheological properties
Rheology examines the flow behavior of NC suspensions under different shear conditions due to their unique structure and viscoelastic properties. NC suspensions display shear-thinning behavior, meaning that the resistance of the material to flow decreases as the shear rate increases. In addition, these suspensions also show viscoelastic behavior, which is a combination of viscous and elastic characteristics. The colloidal properties of NC, such as particle size, shape, concentration, and interactions with the solvent, can affect the rheological behavior of these suspensions. Depending on them, the suspensions can have different rheological properties. For example, the extensional viscosity of NC suspensions is significant and can be affected by the concentration of the nanoparticle and the degree of particle alignment. The shape of NC particles affects the yield strength and non-Newtonian character of the suspension flow, as rod-shaped particles show higher viscosity than spherical particles (Hubbe et al., 2017; Atakhanov et al., 2020). The behavior of NC suspensions in a flow depends on the shape, size, particle concentration, temperature, degree of interaction, and kinetic stability. The rheological study of the aqueous suspensions is of interest, since during flow of the suspension their initial structural organization changes and is destroyed (Hubbe et al., 2017). In addition, NC-based lubricants have been developed with unique rheological properties that make them effective for certain applications. Cationic surface modification of NC has been shown to affect its microstructure and rheological behavior, which has implications for its use in various applications. Overall, there is ongoing research into the rheological properties of NC and how they can be used to develop new materials and applications (Benselfelt et al., 2023).
Rheological characterization techniques such as shear rheology or oscillatory rheology can be employed to study their viscoelastic properties, including shear thinning behavior, elasticity, and gelation (Li et al., 2021). Rotational rheometers, which apply controlled shear stress or shear rate, are commonly employed for steady-state shear tests. These tests generate flow curves that provide information on viscosity, shear thinning behavior, and yield stress, enabling the determination of concentration and dispersion stability of colloidal suspensions (Moberg et al., 2017). Oscillatory measurements, utilizing dynamic oscillations of shear stress or strain, are useful for assessing the viscoelastic properties of NC suspensions. Storage modulus (G′) and loss modulus (G″) can be measured to determine the stability and strength of the particle network within the suspension (Pääkkö et al., 2007). Moberg et al. (2017) concluded that the rheological properties varied significantly between the suspensions depending on the dimensions of the cellulosic elements and their surface characteristics. In this context, the length (or the aspect ratio) of the particles plays a very important role together with the concentration of the sample. Increasing the concentration of NC led to an increase in viscosity and the formation of a gel structure, which is attributed to the formation of the colloidal network structure (Aulin et al., 2012). This points to the importance of a careful characterization of the dimensions and surface character of the elements when comparing such systems and using them in different situations. The interactions between the NC particles and the solvent can also affect the rheological behavior. For example, the addition of salt to a NC suspension can change the rheological properties due to changes in the electrostatic interactions between the particles.
3.6 Thermal properties
Thermal stability are essential characteristics of NC for some applications. The colloidal properties of NC can impact its thermal properties as well. For instance, the presence of hydroxyl groups on the surface of the particles can lead to strong intermolecular hydrogen bonding, which affects the thermal stability of NC composites. In addition, the orientation of NC particles can influence thermal conductivity as it is highly dependent on the degree of alignment of the particles. Several studies have reported improved thermal properties of NC-reinforced composites, particularly in terms of thermal stability, thermal conductivity, and heat storage capacity (Munier et al., 2020).
Differential scanning calorimetry (DSC) provides information about thermal behavior and stability. DSC is a widely used technique for measuring the thermal behavior of materials. In the context of NC, DSC allows the determination of important parameters such as glass transition temperature (Tg), melting temperature (Tm), and heat capacity (Gan et al., 2020). By subjecting NC samples to controlled heating and cooling cycles, DSC provides insights into the thermal transitions occurring within the material. The obtained data helps researchers understand the thermal stability, phase transitions, and energy storage capabilities of NC or thermogravimetric analysis TGA enables the measurement of weight changes in NC samples as a function of temperature. This technique is invaluable in determining thermal degradation properties such as onset temperature, degradation rate, and residue content. TGA can provide valuable information about NC’s thermal stability, decomposition behavior, and potential applications in high-temperature environments. Additionally, TGA coupled with evolved gas analysis (EGA) techniques allows the identification of gases released during thermal degradation, aiding in understanding the degradation mechanisms (Risoluti and Materazzi, 2019). Accurate determination of thermal conductivity is essential for assessing NC’s heat transfer properties. Several techniques, including the transient plane source (TPS) method and the laser flash method, are commonly employed for measuring thermal conductivity. These methods involve subjecting NC samples to controlled heat flow and measuring the resulting temperature changes. By quantifying the thermal conductivity, researchers can evaluate NC’s heat dissipation capabilities, making it useful for applications such as thermal insulation and electronic devices.
3.7 Dispersion stability and aggregation
Accurate measurement of NC stability and the occurrence of aggregation is crucial for understanding its colloidal behavior, optimizing manufacturing processes, and achieving desired material properties. Several widely used methods exist for evaluating dispersion stability and observing aggregation phenomena.
Turbidity is a valuable technique for assessing NC agglomeration and estimating the widths of NC materials. Higher turbidity values indicate a greater presence of aggregated particles in the suspensions (Shimizu et al., 2016). UV-Vis Spectroscopy utilizes the absorption of light by NC at specific wavelengths. Absorbance peak for NC suspensions shifted to longer wavelengths as the particle size increased, due to increased light scattering and reduced absorption (Goh et al., 2014). The effect of salt on the colloidal stability of NC suspensions leads to changes in the UV-Vis spectra due to particle aggregation (Lavoine et al., 2012). Recently, a multi-wavelength spectroscopic technique has been developed for quantifying the carboxyl group content in NC using methylene blue (MB) was used as a color indicator (Yan et al., 2021). DLS also provides information about particle size distribution and can be used to monitor changes in the dispersion stability but it has some limitations. DLS assumes that the particles are spherical and monodisperse, which is not true for NC. Non-spherical or aggregated particles can lead to erroneous size and concentration measurements. Additionally, high concentrations of NC can cause multiple scattering, affecting the accuracy of the results. Careful sample preparation, dilution, and data analysis techniques are necessary to mitigate these challenges (Foster et al., 2018).
On the other hand, light diffraction spectroscopy (LDS) is based on the relation that can be established between the scattering angle and the size of the particles. From the raw signal obtained one can extract information on the particle size distribution of the sample, based either on the Fraunhofer or on the Lorenz Mie theory, and on the fractal dimension of the aggregates. The fractal dimension supplies information about the density of the aggregates which is related to the colloidal destabilization mechanisms and, according to the Rayleigh–Gans–Debye theory, can be calculated from the negative slope of log–log plot of the scattered light intensity as a function of the wave number vector (Rasteiro et al., 2008).
Focused beam reflectance measurements (FBRM) have been also used to monitor NC aggregation. The FBRM devices operate by scanning a highly focused laser beam over particles in suspension at constant speed and measuring the time duration of the backscattered light from these particles. The chord length is the characteristic measurement of particle geometry which is obtained by multiplying the temporal duration of the reflection from each particle or floc by the velocity of the scanning laser (Blanco et al., 2002). By monitoring the number of counts and the chord length of the particles under different shear forces it is possible to determine the colloidal destabilization mechanism and the floc properties when NC is destabilized, and aggregates are larger than 0.5 µm.
In addition, shear birefringence technique is commonly employed to assess dispersion quality of CNCs (Nagarajan et al., 2021). This technique involves birefringence experiments using flat capillary tubes with an inner diameter of 10 mm which are used to equilibrate the CNCs suspensions, and the resulting images are polarized using an optical microscope. By observing the changes in cross-polarization of light passing through the suspensions, the optical effects can be quantified. In CNCs suspensions, individual nanocrystals possess a rigid rod-like structure, leading to a strong tendency to align parallel to a vector director (Habibi et al., 2010; Lane et al., 2022). This alignment of nanocrystals produces a macroscopic birefringence, which can be directly observed through a crossed polarizer. However, when the dispersion is poor due to agglomeration or a low number of particles present in the suspension, visible monochromatic domains are formed, and no birefringence is observed in such cases.
On the other hand, the use of electron microscopes, such as TEM, allows direct visualization and imaging of NC structures (da Silva et al., 2020). By comparing the area occupied by NC with the total area in an image, the concentration can be estimated. In the field of aggregate characterization, TEM is essential to assess dispersion stability and to ensure uniform properties in NC-based materials. It enables the visualization of both individual particles and aggregated structures. The high resolution of TEM makes it well-suited high for imaging NC. Furthermore, TEM enables the efficient screening of a large population of particles, with the flexibility to adjust the sampling as required (Campano et al., 2021). have devised a method to characterize the aggregation state of CNC suspensions by analyzing TEM micrographs. Utilizing this approach, particles captured in the images were automatically sorted into distinct groups based on their characteristics such as maximum Feret diameter (MFD), elongation, circularity, and area. This classification method demonstrated the highest inter-cluster distance, offering a valuable and descriptive representation of the CNC aggregates that allow to explain their colloidal behavior.
Monitoring viscosity, shear stress, and other rheological parameters helps to assess the stability of the dispersion, as aggregation can lead to changes in flow properties. Time-dependent rheological measurements, such as creep and stress relaxation tests, can be employed to evaluate the structural changes in NC suspensions over a specified period. An unstable dispersion may show an increase in viscosity due to particle aggregation or network formation, leading to reduced flowability (Iotti et al., 2011). Conversely, a stable dispersion will maintain its desired rheological properties without significant changes. Rheological analysis enables the determination of critical parameters, such as the gelation time, which indicates the onset of irreversible structural changes.
The measurement of the surface charge of NC using the zeta potential also allow to determine the stability of the colloidal particles. By measuring the electrophoretic mobility, which relates to zeta potential, one can assess the stability of the dispersion. For example, if zeta potential of NC ranges from −15 to 15 mV, there is a possibility of NC agglomeration, suggesting an insufficient charge on the particle surfaces. However, when the zeta is below −30 mV or above 30 mV, the NC suspension becomes more stable (Yang et al., 2017). In these cases, the higher magnitude of zeta potential ensures better electrostatic repulsion between the NC particles, leading to improved dispersion and reduced chances of agglomeration.
4 Interactions with other particles
The interaction of NC with other particles depends on factors such as NC concentration, surface chemistry, particle size, dispersion method, the nature of the surrounding medium and the processing conditions. These interactions play a crucial role in determining the properties and potential applications of NC-based materials. As there are many applications the potential application of NC with other particles is very wide, so we can highlight the interaction with paper products, cement fractions, surfactants, other nanoparticles and other polymers.
4.1 Nanocellulose and paper fractions
NC interacts with fibers, fines, fillers and anionic trash present in the pulp suspensions. The interaction with fibres and fines enhances the mechanical properties of the paper (Perdoch et al., 2022). The interaction with fines and fillers improves retention and reduces linting during paper printing, the interaction with colloidal material reduces the anionic trash and clean the process waters (Song et al., 2010; Balea et al., 2018). Nowadays, the main industrial application of NC is as bulk additive in papermaking to increase paper mechanical properties (Perdoch et al., 2022). The range of improvements is wide and depends on the NC structure. On the other hand, when NC is added to produce coating suspensions for papers, they can alter the rheological properties by increasing viscosity and providing shear-thinning behavior (Liu et al., 2017). This modification facilitates a better coating, improved runnability, and enhanced printability. Incorporating NC into paper also enhance its barrier properties against gases, liquids, and oils, offering potential applications in packaging materials (Dufresne, 2017; Zhang et al., 2021a). Although papermaking is the main industrial application of NC, nowadays there are still several challenges related to their high cost, production of homogeneous and stable products, optimal dispersion for the different uses and a better knowledge on the interaction mechanisms such as hydrogen bonding, adsorption, and bridging effects to optimize the integration of NC within the paper matrix (Balea et al., 2020; Das et al., 2020).
4.2 Nanocellulose and cement fractions
NC has shown promising interactions with various cement fractions. When incorporated into cementitious materials, NC can enhance their mechanical properties, improve workability, accelerated hydration, modify the rheology of the slurries, reduce the porosity, and offer sustainability benefits (Balea et al., 2019a; Guo et al., 2020; Szafraniec et al., 2022). These materials can be added to cement pastes, mortars, or concrete mixtures either during the mixing process or by pre-dispersion, acting as a multifunctional additive due to its unique properties, such as high aspect ratio, large surface area, and high tensile strength. The interaction between NC and cement fractions occurs through several mechanisms (Peters et al., 2010; Balea et al., 2021). Firstly, NC can act as a physical filler, reinforcing the cement matrix and increasing its strength and toughness. The NC fibers form a network within the cementitious material, reducing crack propagation and improving overall durability. Secondly, NC can influence the water-cement ratio and rheological properties of cementitious systems. It acts as a water retention agent, allowing for better control of the workability and setting time of cement mixtures. Nanocellulose also enhances the thixotropic behavior of cement pastes, leading to improved cohesion and reduced bleeding. Furthermore, nanocellulose can promote the hydration of cement, leading to accelerated strength development. It acts as a nucleation site for the formation of calcium silicate hydrate (C-S-H) gel, the primary binder in cementitious materials. This accelerated hydration can result in improved early-age strength and reduced curing time. In addition, NC can also enhance the thermal and acoustic properties of cement-based composites, making them more energy-efficient. Additionally, NC exhibits eco-friendly characteristics, contributing to the sustainability of cementitious materials reducing the carbon footprint of cement production (Balea et al., 2021). Continued research and development in this field hold great potential for the advancement of construction materials.
4.3 Nanocellulose and polymers
The interaction between NC and polymers has gained significant interest in recent years due to its potential applications in various fields such as materials science, biomedical engineering, or environmental sustainability due to NC can be incorporated into polymer matrices to form nanocomposites (Raj et al., 2016; Lasrado et al., 2020). NC and polymer interaction are typically produced by hydrogen bonding and van der Waals forces (Mishnaevsky Jr et al., 2019). NC fibers or particles can disperse within the polymer matrix, leading to enhanced mechanical properties, improved barrier properties, and increased thermal stability. Polymers used for flocculation purposes in NC systems can improve the dewatering and filtration efficiency of NC suspensions. Polymers, such as PAM, PEI, pDADMAC and biopolymers like chitosan, are commonly employed for flocculating NC suspensions (Raj et al., 2016; Balea et al., 2019b). These polymers interact with NC particles through different mechanism: charge neutralization, patching and polymer bridging mechanisms, as explain before, leading to the formation of different type of flocs: small-large, soft-hard, compact-spongous. The properties of the flocs will determine their stability and reflocculation capacity after being subjected to high shear forces like in a pump. Nanocellulose flocculated suspensions are used to favour the nanoparticle retention or to separate the nanoparticles after their use as in the case of adsorption.
Among the different types of polymers that can interact with nanocelluloses, proteins are a notable subset (Fritz et al., 2016). NC can interact with proteins through electrostatic interactions, hydrogen bonding, and hydrophobic interactions. These interactions can influence the stability, structure, and functionality of proteins. Nanocellulose can act as a carrier for protein delivery or as a scaffold for protein immobilization in various applications, such as biomedical devices or food systems. Although this is an emerging field of research it already has significant potential applications in various industries. Several key research needs in this area include: characterization of protein-nanocellulose interactions with standardized methods, such as spectroscopic techniques, surface analysis, and molecular simulations: Investigating the stability of protein-nanocellulose complexes is important for their successful application in various formulations as well as the potential for protein aggregation on nanocellulose surfaces is essential to ensure product safety and performance.
Further research is needed to deepen in the understanding of the fundamental interactions between NC and the different type of polymers at the molecular level. This includes studying the intermolecular forces, surface chemistry, pH, temperature, ionic strength, and the influence of the different polymer types and structures on the interaction behavior. In addition, the investigation of the compatibility and long-term stability of NC-polymer composites is crucial for their practical applications. Among them, for biological applications the evaluation of the potential cytotoxicity, biocompatibility, immune response, and long-term effects of these complexes are relevant for biological systems, including in vitro and in vivo models. Besides, the impact of NC on protein bioactivity and functionality is crucial, requiring the study of NC interaction with protein structure, folding, and enzymatic activity. Furthermore, the understanding of the NC influence on protein functionality is required, such as drug delivery, tissue engineering, and food science applications. In general, research also should focus on understanding the factors that affect the compatibility between NC and different polymers, as well as developing strategies to enhance the stability and prevent phase separation or degradation over time. Studying the reinforcement mechanisms within the composites, including load transfer, stress distribution, and deformation behavior, can help optimize the composite materials for specific applications. Exploring the potential for imparting additional functionalities to nanocellulose-polymer composites is an area of interest. Research should investigate techniques for incorporating functional additives, such as nanoparticles, dyes, or biomolecules, to create composites with tailored properties such as enhanced electrical conductivity, optical properties, or antimicrobial activity.
4.4 Nanocellulose and surfactants
Surfactants can be used to modify the surface properties of NC and enhance its dispersibility in water or organic solvents. The hydrophilicity of NC produces by the high content of hydroxyl groups can be reduced by adding surfactants with a hydrophilic end which bind to the NC and another hydrophobic (Xie and Liu, 2021). In this way surfactant molecules can adsorb onto the NC surface, forming a protective layer that prevents agglomeration or aggregation of the particles (Tardy et al., 2017). This enables the stable dispersion of NC in different media and facilitates its incorporation into various formulations. In this way, surfactants present different effects on the surface properties of NC, such as wettability, dispersibility, and stability. Some challenges in this field are a deep understanding of the interactions of surfactants at different concentrations with the NC surface, that can enable the development of tailored surface modifications for specific applications with different shear thinning behavior, and viscoelastic properties. The examination of the interfacial behavior between NC and surfactants at the air-water and oil-water interfaces can give light on the formation of surface films, emulsions, foams, and other interfacial structures, which can be utilized in areas such as food processing, cosmetics, and materials science.
4.5 Nanocellulose interactions with other nanoparticles
NC can interact with nanoparticles, such as metal nanoparticles or carbon nanotubes (Dias et al., 2020; Pagliaro et al., 2021). These interactions can be both physical and chemical in nature. Physical interactions include adsorption or coating of nanoparticles onto the NC surface, while chemical interactions may involve covalent bonding between the nanoparticles and NC. There are diverse potential applications for this combination of materials, such as in composites, coatings, films, electronics, energy storage, or biomedical devices (Pagliaro et al., 2021). Specific research is needed to understand their behavior and optimize their performance in each application, addressing unique challenges and requirements. The potential health and safety implications of nanocellulose and nanoparticles need thorough investigation. Researchers should assess their toxicity, environmental impact, and long-term effects on human health to ensure safe handling, disposal, and use in different applications.
5 Research needs of nanocellulose colloids
As it has been mentioned, several NC properties are critical for each application, so in most cases a balance between the different properties is required. Therefore, in this section we discuss the colloidal properties and research needs focusing on the main NC applications in several sectors (Figure 5).
5.1 Biomedical applications
NC-based colloidal systems have been explored for different biomedical applications such as drug delivery, tissue engineering scaffolds, wound healing materials, and bioimaging (Harish et al., 2022). This is a low market of high added value that represents an interesting niche market. The large surface area, surface charge and biocompatibility of NC make it an ideal candidate for these applications. The surface charge of NC affects its interactions with cells, proteins, and other biomolecules, and can influence properties such as colloidal stability, cellular uptake, and biocompatibility (Curvello et al., 2019). Controlling and optimizing the surface charge of nanocellulose can enhance its performance and suitability for various biomedical applications, including drug delivery, tissue engineering, and wound healing. Additionally, the colloidal stability of NC suspensions allows them to be used for controlled release of therapeutic agents while some parameters such as pH or ionic strength can be modified (Dutta et al., 2016; Curvello et al., 2019).
Further studies are needed to evaluate the biocompatibility of NC materials, including their interactions with living cells and tissues (Gumrah Dumanli, 2017; Rai and Dhar, 2022). This research should include in vitro and in vivo experiments to assess the cytotoxicity, immunogenicity, and long-term effects of nanocellulose on biological systems. Investigating the potential of NC as a carrier for controlled drug release is crucial. Research should focus on optimizing the loading and release kinetics of various therapeutic agents, such as drugs, proteins, and nucleic acids, using nanocellulose-based matrices or nanoparticles. Nanocellulose can be utilized to fabricate three-dimensional scaffolds for tissue regeneration. In addition, research also needs to optimize the mechanical properties, degradation rates, and cellular response of nanocellulose scaffolds, as well as to explore their potential in the support and proliferation of different cell types that can be seeded in the NC. Investigating the potential of NC in promoting wound healing is important. Research should focus on developing NC-based dressings or patches with enhanced antimicrobial properties, moisture retention capacity, and modulation of cellular responses to accelerate the healing process. Exploring the use of NC in diagnostic imaging techniques, such as contrast agents or nanoparticles for targeted imaging, is an emerging research area. Further investigation is needed to optimize the imaging capabilities, stability, and biocompatibility of nanocellulose-based contrast agents as well as a further development of NC functionalization.
5.2 Paper and board industry
NC colloids have the potential to revolutionize the paper industry by improving paper strength, transparency, and printability (Balea et al., 2020; Chugh et al., 2022). This market is of high interest because its high volume. The potential to increase barrier properties are of great importance to increase the potential of paper-based products to substitute plastics. Several parameters have influence on paper applications like particle size, surface area, crystallinity, chemical composition and dispersibility. However, the concentration may be considered as the key parameter. The concentration of NC in the paper or board formulation affects its mechanical strength, optical properties, and barrier properties (Das et al., 2020; Sanchez-Salvador et al., 2020). A higher NC concentration generally leads to improved strength and stiffness, increased opacity, and enhanced barrier properties, such as improved water resistance and gas barrier performance. However, excessively high concentrations can also pose processing problems and may result in decreased workability, dewatering difficulties or increased the viscosity of the formulation (Osong et al., 2016). Therefore, finding the optimal NC concentration is crucial for achieving the desired properties in NC-based paper and board products. Another important property is the dispersion degree before its use. Exploring ways to incorporate NC into paper to enhance its properties is still necessary. Starting from the two main possible alternatives to use the NC, in bulk and superficially as a coating, the modification of the NC surface or the developing of suitable surface treatments are the more used options to improve NC compatibility with paper fibers (Lengowski et al., 2019; Hashemzehi et al., 2022). The development of functional papers with enhanced barrier properties, lightweight packaging materials, biodegradable composites, and environmentally friendly printing substrates is still a research need (Hashemzehi et al., 2022; Mujtaba et al., 2022). An interesting novel topic of research is the synergic effect between the use of NC and hot-pressing. On the other hand, NC can interact with other type of fibers, for instance with textile fibers, imparting desirable properties, such as increased tensile strength, water absorption capacity, and dye adsorption efficiency (Spagnuolo et al., 2022).
5.3 Food industry
NC colloids can enhance food quality and safety by acting as stabilizers, emulsifiers, and thickeners. They can improve the stability and texture of food products, replace synthetic additives (Perumal et al., 2022). One of the most studied topics in this field have been the use of NC as Pickering emulsifier via the formation of 3D networks that envelopes the emulsion droplets inhibiting their movement (Martins et al., 2020; Teo et al., 2022). On the other hand, the eco-friendly nature of NC aligns well with the increasing demand for sustainable packaging solutions providing excellent barrier properties (Zhang et al., 2021c). One key parameter for NC in food and packaging applications is its particle size. Nanocellulose particles with a small and uniform size are desirable because they can improve the mechanical properties, transparency, and barrier properties of food packaging materials. Additionally, smaller particle sizes enhance the stability and dispersibility of nanocellulose in food systems, allowing for better control of the rheological properties, texture and organoleptic properties (Martins et al., 2020). In this application further studies are needed on the potential toxicity and any possible interactions with food components, investigating the compatibility of NC materials with different types of food products (Silva et al., 2020). Research should explore potential interactions between NC and various food components, such as acidity, moisture content, and oil content. Besides, a better understanding of the role and effectiveness of NC in preventing moisture, oxygen, and other gases from permeating the packaging material and affecting the food quality is still necessary, requiring further study of the potential of NC-based materials to extend the shelf life of food products by maintaining their freshness, preventing spoilage, and reducing microbial contamination is important (Ahankari et al., 2021). This may involve incorporating antimicrobial agents or developing active packaging systems (Moreirinha et al., 2020; Abdalkarim et al., 2021). Finally, consumer perceptions, acceptance, and willingness to adopt nanocellulose-based food packaging is crucial. Understanding consumer attitudes, concerns, and preferences can help drive market adoption and identify potential barriers to consumer acceptance.
5.4 Energy
NC colloids have shown promise in energy-related applications, including supercapacitors, batteries, and solar cells (Du et al., 2017). Their high surface area and electrical conductivity make them suitable for energy storage and conversion devices (Lokhande et al., 2022). High tensile strength and stiffness are required for reinforcing materials in energy-efficient composites. Thermal stability allows NC to withstand high temperatures without significant degradation. Low density contributes to lightweight materials and structures. Explore the potential of NC-based materials as efficient and cost-effective alternatives for energy generation and storage is essential to increase their performance and stability. However, the use of NC as a catalyst or support material for biofuel production needs to be further explored (El-Nahas et al., 2017). The potential of NC-based materials for energy harvesting applications, such as piezoelectric or triboelectric nanogenerators must be further explored as well as the use of NC to an efficiently conversion of mechanical or environmental energy into electrical energy (Zhang et al., 2021b). Investigate the compatibility and integration of NC-based energy materials with existing energy infrastructure and systems. Assess the feasibility of incorporating NC into current energy technologies and evaluate their performance in real-world conditions.
5.5 Environmental applications
NC materials, with a large surface area, enable an efficient adsorption and the removal of pollutants, making it valuable for environmental remediation and water purification processes (Mahfoudhi and Boufi, 2017; Shak et al., 2018). The mechanical strength of NC plays a crucial role in water applications such as filtration, membrane technology, and reinforcement of hydrogels. High mechanical strength allows NC-based materials to withstand water flow, pressure, and other mechanical stresses. NC exhibits excellent water retention properties, enabling it to retain water and maintain a stable water content in various applications, such as in personal care products or soil amendment for water retention in agriculture (Bauli et al., 2021; Barajas-Ledesma et al., 2022).
5.6 Coatings and films
NC colloids can serve as additives in coatings and films, imparting enhanced mechanical properties, barrier properties, and flame retardancy. They can be incorporated into paints or coatings to improve their performance and reduce the environmental impact of these materials (Panchal et al., 2018). The different properties that present the diverse NC materials can affect in the different properties of the end products. Some examples are the particle size that affects properties such as film transparency, mechanical strength, or the barrier performance, the aspect ratio which mainly affects the film strength, flexibility or reinforcement capability, the crystallinity that affects the mechanical properties, water absorption, or the barrier properties of films and coatings, the surface charge and chemistry functionalization that influences in the interactions with other particles, the dispersibility in solvents, or the adhesion to substrates, and also the influence in the rheological properties in coating production or during film processing, with modifications such as flow behavior or film thickness and uniformity control (Dufresne, 2019; Sharma et al., 2020; Pirozzi et al., 2021).
NC films and coatings are typically formed through solvent evaporation or self-assembly, in which the drying behavior affects in some properties such as film thickness, smoothness, and homogeneity (Wang et al., 2019). NC films can exhibit barrier properties against gases, such as oxygen or water vapor (Wu et al., 2022). The barrier performance depends on factors like film thickness, porosity, and crystallinity. NC can be combined with other materials, such as polymers or nanoparticles, to enhance specific properties or functionalities (Xu et al., 2021). Compatibility with other components is crucial for achieving desired film/coating performance.
5.7 Composites production for different sectors
The use of NC can serve in other multiple sectors and applications. NC colloids can serve in the production of composites and nanocomposites with other polymers mainly to enhance mechanical strength of them (Chakrabarty and Teramoto, 2018). Further research is required to optimize processing techniques for incorporating NC into composite materials. This includes investigating different methods for dispersing uniformly the NC materials within a matrix, such as melt blending, solution casting, or electrospinning. Exploring the potential of NC for imparting multifunctional properties to composites is an important research area, investigating its ability to enhance thermal, electrical, and barrier properties can open up new applications in areas such as packaging, electronics, and energy storage. Besides, NC can be added as an additive to reinforce fiber–cement composites. The use of NC in fiber cement production has been demonstrated to be efficient in improving mechanical properties, modifying the slurry’s rheology, reducing the porosity, and driving interactions with other components of the slurry (Balea et al., 2019a). In addition, the use of NC in energy-efficient materials for buildings and transportation is also in exploration, looking for the NC incorporation into composites, coatings, and insulation materials to enhance their thermal insulation properties and reduce energy consumption.
5.8 Others
Moreover all applications and sectors described, and due to the wide number of valuable properties of NC, these colloids can be applied in other sectors. Some examples are the use in cosmetics as a carrier of active ingredients or as a structuring agent of cosmetic formulations (Almeida et al., 2021), the use in textiles as an antimicrobial agent or fire-retardant additive (Spagnuolo et al., 2022) or to enhance oil recovery by the functionalization of the NC chains to make them more hydrophobic (Zhu et al., 2021a).
6 Conclusion and Future perspectives
When viewed from a colloidal material perspective, NC presents intriguing possibilities for various applications. Understanding the colloidal behavior, stabilization mechanisms, hydrodynamic forces and characterization techniques associated with NC colloids is crucial to unlock their potential. The unique properties and eco-friendly nature of NC make it an attractive material for industries seeking sustainable and high-performance solutions. The main lessons learnt in the last years have been that the industrial implementation is more difficult than expected due to the heterogeneity of these materials which highly affect their stability and performance on the final products.
Researchers and users must consider that stabilization of NC materials involves many factors, both intra and interrelated, that are all connected, and their combined effects determine the overall stability of a NC colloidal suspensions at a given time. To favor the use of NC products developing cost-effective and scalable methods to produce NC is a key research need. Investigating different sustainable production processes and optimizing extraction and purification processes can contribute to the large-scale production of NC for the different applications. Based on the specific key parameters identified for the different uses NC products fit-for-use must be produced for which colloidal properties are key. Research should focus on strategies to control the self-assembly and to achieve homogeneous products and uniform dispersion of NC within the different matrices, as well as on exploring innovative methods for manufacturing complex-shaped structures in which interactions between particles are critical to development sustainable and renewable materials with improved mechanical, barrier, and optical properties.
The sustainability aspects of NC production and its impact on the environment throughout its life cycle is also a key challenge. This includes assessing the environmental footprint, energy consumption, water usage, and waste management associated with NC production and its integration into paper products. The colloidal interactions when reactive and catalysts are reused are critical to further develop sustainable processes.
As NC move towards commercialization, it is essential to conduct comprehensive safety assessments and establish clear regulatory guidelines for the use of NC-based colloid systems in the different applications, especially for biomedical, food and packaging applications. Research should address long-term safety. Establishing standardized testing methods, quality control protocols, and regulatory frameworks for NC-based products can facilitate commercialization and ensure product safety and consistency.
Exploring synergistic combinations of NC with other biomaterials, such as polymers, hydrogels, or nanoparticles, can lead to improved properties and functionalities. Research should focus on understanding the mechanisms of NC integration into the matrix and on developing composite materials that leverage the unique characteristics of NC in combination with other materials for enhanced applications. Enhancing the mechanical strength and flexibility of NC-based materials is essential for their use as reinforcement agents. Research should focus on improving their resistance to tearing, puncturing, and other mechanical stresses encountered during transportation and handling. The continued research and development in this field will undoubtedly unlock further opportunities for NC-based colloidal systems. Future direction should consider.
• Multiscale Modeling: Integrating experimental measurements with computational modeling techniques to offer insights into the colloidal and mechanical behavior of NC. Multiscale simulations help bridge the gap between the nanoscale and macroscopic properties.
• In-situ Techniques: Developing in situ characterization techniques enables real-time monitoring of NC behavior under mechanical loading. This approach provides a deeper understanding of deformation mechanisms and dynamic property changes.
• Dispersibility and stability: Exploring surfactant-assisted dispersion methods to enhance the dispersibility and stability of NC suspensions. This research can involve optimizing surfactant concentrations, types, and processing conditions to achieve improved dispersion and long-term stability.
• Assessing the compatibility of NC and surfactants with other materials and evaluating their combined properties for specific applications. This research can explore the synergistic effects, such as improved mechanical strength, thermal stability, or barrier properties when NC is combined with surfactants, which can be valuable in fields like packaging, composites, and biomedicine.
Author contributions
ABl and CN contributed to conception and design of the review. ABl wrote the first draft of the manuscript. ABa, HX, and JS-S wrote sections of the manuscript. JS-S prepared figures and reference file. ABl and CN contributed to project management and adquisition. All authors contributed to the article and approved the submitted version.
Funding
The authors are grateful for the financial support by the Science and Innovation Ministry of Spain (project PID2020-113850RB-C21 “CON-FUTURO”) and the Community of Madrid (project S2018/EMT-4459 “RETOPROSOST2-CM”).
Conflict of interest
The authors declare that the research was conducted in the absence of any commercial or financial relationships that could be construed as a potential conflict of interest.
Publisher’s note
All claims expressed in this article are solely those of the authors and do not necessarily represent those of their affiliated organizations, or those of the publisher, the editors and the reviewers. Any product that may be evaluated in this article, or claim that may be made by its manufacturer, is not guaranteed or endorsed by the publisher.
References
Abdalkarim, S. Y. H., Chen, L.-M., Yu, H.-Y., Li, F., Chen, X., Zhou, Y., et al. (2021). Versatile nanocellulose-based nanohybrids: A promising-new class for active packaging applications. Int. J. Biol. Macromol. 182, 1915–1930. doi:10.1016/j.ijbiomac.2021.05.169
Aguado, R., Tarrés, Q., Mutjé, P., Pèlach, M. À., and Delgado-Aguilar, M. (2022). Non-covalently cationized nanocellulose from hemp: Kinetics, key properties, and paper strengthening. Industrial Crops Prod. 188, 115582. doi:10.1016/j.indcrop.2022.115582
Ahankari, S. S., Subhedar, A. R., Bhadauria, S. S., and Dufresne, A. (2021). Nanocellulose in food packaging: A review. Carbohydr. Polym. 255, 117479. doi:10.1016/j.carbpol.2020.117479
Aigaje, E., Riofrio, A., and Baykara, H. (2023). Processing, properties, modifications, and environmental impact of nanocellulose/biopolymer composites: A review. Polymers 15, 1219. doi:10.3390/polym15051219
Alagarsamy, M., Barkunan, S., Jayapal, N., Murugan, A., Muralikrishnan, P., and Ramulu, P. J. (2023). Reinforcement of nanocellulose as green agent in the electronic applications associated with the composites of polymer matrix. Int. J. Polym. Sci. 2023, 1–9. doi:10.1155/2023/9645190
Almeida, T., Silvestre, A. J., Vilela, C., and Freire, C. S. (2021). Bacterial nanocellulose toward green cosmetics: Recent progresses and challenges. Int. J. Mol. Sci. 22, 2836. doi:10.3390/ijms22062836
Ang, S., Haritos, V., and Batchelor, W. (2020). Cellulose nanofibers from recycled and virgin wood pulp: A comparative study of fiber development. Carbohydr. Polym. 234, 115900. doi:10.1016/j.carbpol.2020.115900
Aoudi, B., Boluk, Y., and El-Din, M. G. (2022). Recent advances and future perspective on nanocellulose-based materials in diverse water treatment applications. Sci. Total Environ. 843, 156903. doi:10.1016/j.scitotenv.2022.156903
Araya-Chavarría, K., Rojas, R., Ramírez-Amador, K., Sulbarán-Rangel, B., Rojas, O., and Esquivel-Alfaro, M. (2022). Cellulose nanofibers as functional biomaterial from pineapple stubbles via TEMPO oxidation and mechanical process. Waste Biomass Valorization 13, 1749–1758. doi:10.1007/s12649-021-01619-3
Asad, M., Saba, N., Asiri, A. M., Jawaid, M., Indarti, E., and Wanrosli, W. (2018). Preparation and characterization of nanocomposite films from oil palm pulp nanocellulose/poly (Vinyl alcohol) by casting method. Carbohydr. Polym. 191, 103–111. doi:10.1016/j.carbpol.2018.03.015
Atakhanov, A., Kholmuminov, A., Mamadierov, B., Turdikulov, I. K., and Ashurov, N. S. (2020). Rheological behavior of nanocellulose aqueous suspensions. Polym. Sci. Ser. A 62, 213–217. doi:10.1134/s0965545x20030013
Aulin, C., Salazar-Alvarez, G., and Lindström, T. (2012). High strength, flexible and transparent nanofibrillated cellulose–nanoclay biohybrid films with tunable oxygen and water vapor permeability. Nanoscale 4, 6622–6628. doi:10.1039/c2nr31726e
Balea, A., Campano, C., Merayo, N., Blanco, A., and Negro, C. (2017). “Assessing the dispersion influence of cellulose nanofibers on papermaking applications,” AIChE Annual Meeting, Minneapolis, MN, November 2, 2017.
Balea, A., Fuente, E., Blanco, A., and Negro, C. (2019a). Nanocelluloses: Natural-based materials for fiber-reinforced cement composites. A critical review. Polymers 11, 518. doi:10.3390/polym11030518
Balea, A., Fuente, E., Monte, M. C., Blanco, A., and Negro, C. (2021). Recycled fibers for sustainable hybrid fiber cement based material: A review. Materials 14, 2408. doi:10.3390/ma14092408
Balea, A., Fuente, E., Monte, M. C., Merayo, N., Campano, C., Negro, C., et al. (2020). Industrial application of nanocelluloses in papermaking: A review of challenges, technical solutions, and market perspectives. Molecules 25, 526. doi:10.3390/molecules25030526
Balea, A., Merayo, N., Fuente, E., Negro, C., Delgado-Aguilar, M., Mutje, P., et al. (2018). Cellulose nanofibers from residues to improve linting and mechanical properties of recycled paper. Cellulose 25, 1339–1351. doi:10.1007/s10570-017-1618-x
Balea, A., Monte, M. C., Fuente, E., Sanchez-Salvador, J. L., Blanco, A., and Negro, C. (2019b). Cellulose nanofibers and chitosan to remove flexographic inks from wastewaters. Environ. Sci. Water Res. Technol. 5, 1558–1567. doi:10.1039/c9ew00434c
Barajas-Ledesma, R. M., Wong, V. N., Little, K., Patti, A. F., and Garnier, G. (2022). Carboxylated nanocellulose superabsorbent: Biodegradation and soil water retention properties. J. Appl. Polym. Sci. 139, 51495. doi:10.1002/app.51495
Bauli, C. R., Lima, G. F., De Souza, A. G., Ferreira, R. R., and Rosa, D. S. (2021). Eco-friendly carboxymethyl cellulose hydrogels filled with nanocellulose or nanoclays for agriculture applications as soil conditioning and nutrient carrier and their impact on cucumber growing. Colloids Surfaces A Physicochem. Eng. Aspects 623, 126771. doi:10.1016/j.colsurfa.2021.126771
Benselfelt, T., Kummer, N., Nordenström, M., Fall, A. B., Nyström, G., and Wågberg, L. (2023). The colloidal properties of nanocellulose. Germany: ChemSusChem.
Bhattacharjee, S. (2016). DLS and zeta potential–what they are and what they are not? J. Control. release 235, 337–351. doi:10.1016/j.jconrel.2016.06.017
Blanco, A., Fuente, E., Negro, C., and Tijero, J. (2002). Flocculation monitoring: Focused beam reflectance measurement as a measurement tool. Can. J. Chem. Eng. 80, 1–7. doi:10.1002/cjce.5450800403
Blanco, A., Monte, M. C., Campano, C., Balea, A., Merayo, N., and Negro, C. (2018). “Nanocellulose for industrial use: Cellulose nanofibers (CNF), cellulose nanocrystals (CNC), and bacterial cellulose (BC),” in Handbook of nanomaterials for industrial applications (USA: Elsevier), 74–126.
Blanco, A., Negro, C., and Tijero, J. (2001). Developments of flocculation in papermaking. Editor Pira International (Reino Unido: Leatherhead).
Cadotte, M., Tellier, M. E., Blanco, A., Fuente, E., Van De Ven, T. G., and Paris, J. (2007). Flocculation, retention and drainage in papermaking: A comparative study of polymeric additives. Can. J. Chem. Eng. 85, 240–248. doi:10.1002/cjce.5450850213
Campano, C., Lopez-Exposito, P., Gonzalez-Aguilera, L., Blanco, Á., and Negro, C. (2021). In-depth characterization of the aggregation state of cellulose nanocrystals through analysis of transmission electron microscopy images. Carbohydr. Polym. 254, 117271. doi:10.1016/j.carbpol.2020.117271
Campano, C., Merayo, N., Balea, A., Tarrés, Q., Delgado-Aguilar, M., Mutjé, P., et al. (2018). Mechanical and chemical dispersion of nanocelluloses to improve their reinforcing effect on recycled paper. Cellulose 25, 269–280. doi:10.1007/s10570-017-1552-y
Chakrabarty, A., and Teramoto, Y. (2018). Recent advances in nanocellulose composites with polymers: A guide for choosing partners and how to incorporate them. Polymers 10, 517. doi:10.3390/polym10050517
Chu, Y., Sun, Y., Wu, W., and Xiao, H. (2020). Dispersion properties of nanocellulose: A review. Carbohydr. Polym. 250, 116892. doi:10.1016/j.carbpol.2020.116892
Chugh, M., Chandak, T., Jha, S., and Rawtani, D. (2022). “Nanocellulose in paper and wood industry,” in Nanocellulose materials (USA: Elsevier), 247–264.
Curvello, R., Raghuwanshi, V. S., and Garnier, G. (2019). Engineering nanocellulose hydrogels for biomedical applications. Adv. Colloid Interface Sci. 267, 47–61. doi:10.1016/j.cis.2019.03.002
Da Silva, L. C., Cassago, A., Battirola, L. C., Gonçalves, M. D. C., and Portugal, R. V. (2020). Specimen preparation optimization for size and morphology characterization of nanocellulose by TEM. Cellulose 27, 5435–5444. doi:10.1007/s10570-020-03116-7
Das, A. K., Islam, M. N., Ashaduzzaman, M., and Nazhad, M. M. (2020). Nanocellulose: Its applications, consequences and challenges in papermaking. J. Packag. Technol. Res. 4, 253–260. doi:10.1007/s41783-020-00097-7
De Souza, A. G., De Lima, G. F., Colombo, R., and Rosa, D. S. (2020). A new approach for the use of anionic surfactants: Nanocellulose modification and development of biodegradable nanocomposites. Cellulose 27, 5707–5728. doi:10.1007/s10570-020-03160-3
Dias, O. a. T., Konar, S., Leão, A. L., Yang, W., Tjong, J., and Sain, M. (2020). Current state of applications of nanocellulose in flexible energy and electronic devices. Front. Chem. 8, 420. doi:10.3389/fchem.2020.00420
Dominic, M., Joseph, R., Begum, P. S., Kanoth, B. P., Chandra, J., and Thomas, S. (2020). Green tire technology: Effect of rice husk derived nanocellulose (RHNC) in replacing carbon black (CB) in natural rubber (NR) compounding. Carbohydr. Polym. 230, 115620. doi:10.1016/j.carbpol.2019.115620
Du, X., Zhang, Z., Liu, W., and Deng, Y. (2017). Nanocellulose-based conductive materials and their emerging applications in energy devices-A review. Nano Energy 35, 299–320. doi:10.1016/j.nanoen.2017.04.001
Dufresne, A. (2017). Cellulose nanomaterial reinforced polymer nanocomposites. Curr. Opin. Colloid & Interface Sci. 29, 1–8. doi:10.1016/j.cocis.2017.01.004
Dufresne, A. (2019). Nanocellulose processing properties and potential applications. Curr. For. Rep. 5, 76–89. doi:10.1007/s40725-019-00088-1
Dufresne, A. (2013). Nanocellulose: A new ageless bionanomaterial. Mater. Today 16, 220–227. doi:10.1016/j.mattod.2013.06.004
Dutta, S., Samanta, P., and Dhara, D. (2016). Temperature, pH and redox responsive cellulose based hydrogels for protein delivery. Int. J. Biol. Macromol. 87, 92–100. doi:10.1016/j.ijbiomac.2016.02.042
El-Nahas, A. M., Salaheldin, T. A., Zaki, T., El-Maghrabi, H. H., Marie, A. M., Morsy, S. M., et al. (2017). Functionalized cellulose-magnetite nanocomposite catalysts for efficient biodiesel production. Chem. Eng. J. 322, 167–180. doi:10.1016/j.cej.2017.04.031
Foster, E. J., Moon, R. J., Agarwal, U. P., Bortner, M. J., Bras, J., Camarero-Espinosa, S., et al. (2018). Current characterization methods for cellulose nanomaterials. Chem. Soc. Rev. 47, 2609–2679. doi:10.1039/c6cs00895j
Fritz, C., Jeuck, B., Salas, C., Gonzalez, R., Jameel, H., and Rojas, O. J. (2016). Nanocellulose and proteins: Exploiting their interactions for production, immobilization, and synthesis of biocompatible materials. Cellul. Chem. Prop. Fibers, Nanocelluloses Adv. Mater., 207–224. doi:10.1007/12_2015_322
Fukuzumi, H., Saito, T., and Isogai, A. (2013). Influence of TEMPO-oxidized cellulose nanofibril length on film properties. Carbohydr. Polym. 93, 172–177. doi:10.1016/j.carbpol.2012.04.069
Gamelas, J. A., Pedrosa, J., Lourenço, A. F., Mutjé, P., González, I., Chinga-Carrasco, G., et al. (2015). On the morphology of cellulose nanofibrils obtained by TEMPO-mediated oxidation and mechanical treatment. Micron 72, 28–33. doi:10.1016/j.micron.2015.02.003
Gan, P., Sam, S., Abdullah, M. F. B., and Omar, M. F. (2020). Thermal properties of nanocellulose-reinforced composites: A review. J. Appl. Polym. Sci. 137, 48544. doi:10.1002/app.48544
Goh, E., Xu, X., and Mccormick, P. (2014). Effect of particle size on the UV absorbance of zinc oxide nanoparticles. Scr. Mater. 78, 49–52. doi:10.1016/j.scriptamat.2014.01.033
Gomri, C., Cretin, M., and Semsarilar, M. (2022). Recent progress on chemical modification of cellulose nanocrystal (CNC) and its application in nanocomposite films and membranes-A comprehensive review. Carbohydr. Polym. 294, 119790. doi:10.1016/j.carbpol.2022.119790
Gu, H., Gao, X., Zhang, H., Chen, K., and Peng, L. (2020). Fabrication and characterization of cellulose nanoparticles from maize stalk pith via ultrasonic-mediated cationic etherification. Ultrason. sonochemistry 66, 104932. doi:10.1016/j.ultsonch.2019.104932
Gumrah Dumanli, A. (2017). Nanocellulose and its composites for biomedical applications. Curr. Med. Chem. 24, 512–528. doi:10.2174/0929867323666161014124008
Guo, A., Sun, Z., Sathitsuksanoh, N., and Feng, H. (2020). A review on the application of nanocellulose in cementitious materials. Nanomaterials10, 2476. doi:10.3390/nano10122476
Gustafsson, O., Gustafsson, S., Manukyan, L., and Mihranyan, A. (2018). Significance of Brownian motion for nanoparticle and virus capture in nanocellulose-based filter paper. Membranes 8, 90. doi:10.3390/membranes8040090
Habibi, Y., Lucia, L. A., and Rojas, O. J. (2010). Cellulose nanocrystals: Chemistry, self-assembly, and applications. Chem. Rev. 110, 3479–3500. doi:10.1021/cr900339w
Hajian, A., Lindstrom, S. B., Pettersson, T., Hamedi, M. M., and WaGberg, L. (2017). Understanding the dispersive action of nanocellulose for carbon nanomaterials. Nano Lett. 17, 1439–1447. doi:10.1021/acs.nanolett.6b04405
Harish, V., Tewari, D., Gaur, M., Yadav, A. B., Swaroop, S., Bechelany, M., et al. (2022). Review on nanoparticles and nanostructured materials: Bioimaging, biosensing, drug delivery, tissue engineering, antimicrobial, and agro-food applications. Nanomaterials 12, 457. doi:10.3390/nano12030457
Hashemzehi, M., Mesic, B., Sjöstrand, B., and Naqvi, M. (2022). A comprehensive review of nanocellulose modification and applications in papermaking and packaging: Challenges, technical solutions, and perspectives. BioResources 17, 3718–3780. doi:10.15376/biores.17.2.hashemzehi
Haunreiter, K. J., Dichiara, A. B., and Gustafson, R. (2022). Nanocellulose by ammonium persulfate oxidation: An alternative to TEMPO-mediated oxidation. ACS Sustain. Chem. Eng. 10, 3882–3891. doi:10.1021/acssuschemeng.1c07814
Heise, K., Koso, T., King, A. W., Nypelö, T., Penttilä, P., Tardy, B. L., et al. (2022). Spatioselective surface chemistry for the production of functional and chemically anisotropic nanocellulose colloids. J. Mater. Chem. A 10, 23413–23432. doi:10.1039/d2ta05277f
Hubbe, M. A., Tayeb, P., Joyce, M., Tyagi, P., Kehoe, M., Dimic-Misic, K., et al. (2017). Rheology of nanocellulose-rich aqueous suspensions: A review. BioResources 12, 9556–9661. doi:10.15376/biores.12.4.hubbe
Ilyas, R., Azmi, A., Nurazzi, N., Atiqah, A., Atikah, M., Ibrahim, R., et al. (2022). Oxygen permeability properties of nanocellulose reinforced biopolymer nanocomposites. Mater. Today Proc. 52, 2414–2419. doi:10.1016/j.matpr.2021.10.420
Iotti, M., Gregersen, Ø. W., Moe, S., and Lenes, M. (2011). Rheological studies of microfibrillar cellulose water dispersions. J. Polym. Environ. 19, 137–145. doi:10.1007/s10924-010-0248-2
Iso (2017). ISO-TS 20477: 2017 (e): Nanotechnologies: Standard terms and their definition for cellulose nanomaterial. China: ISO.
Jordan, J. H., Easson, M. W., Dien, B., Thompson, S., and Condon, B. D. (2019). Extraction and characterization of nanocellulose crystals from cotton gin motes and cotton gin waste. Cellulose 26, 5959–5979. doi:10.1007/s10570-019-02533-7
Joseph, B., Sagarika, V., Sabu, C., Kalarikkal, N., and Thomas, S. (2020). Cellulose nanocomposites: Fabrication and biomedical applications. J. Bioresour. Bioprod. 5, 223–237. doi:10.1016/j.jobab.2020.10.001
Kajtna, J., and Šebenik, U. (2017). Novel acrylic/nanocellulose microsphere with improved adhesive properties. Int. J. Adhesion Adhesives 74, 100–106. doi:10.1016/j.ijadhadh.2016.11.013
Kaldéus, T., Nordenström, M., Carlmark, A., Wågberg, L., and Malmström, E. (2018). Insights into the EDC-mediated PEGylation of cellulose nanofibrils and their colloidal stability. Carbohydr. Polym. 181, 871–878. doi:10.1016/j.carbpol.2017.11.065
Kan, K. H., and Cranston, E. D. (2013). Mechanical testing of thin film nanocellulose composites using buckling mechanics. TAPPI J. 12, 9–17. doi:10.32964/tj12.4.9
Karabulut, E., and Wågberg, L. (2011). Design and characterization of cellulose nanofibril-based freestanding films prepared by layer-by-layer deposition technique. Soft Matter 7, 3467–3474. doi:10.1039/c0sm01355b
Klemm, D., Kramer, F., Moritz, S., Lindström, T., Ankerfors, M., Gray, D., et al. (2011). Nanocelluloses: A new family of nature-based materials. Angew. Chem. Int. Ed. 50, 5438–5466. doi:10.1002/anie.201001273
Lahiji, R. R., Xu, X., Reifenberger, R., Raman, A., Rudie, A., and Moon, R. J. (2010). Atomic force microscopy characterization of cellulose nanocrystals. Langmuir 26, 4480–4488. doi:10.1021/la903111j
Lane, C., Rode, D., and Rösgen, T. (2022). Birefringent properties of aqueous cellulose nanocrystal suspensions. Cellulose 29, 6093–6107. doi:10.1007/s10570-022-04646-y
Lasrado, D., Ahankari, S., and Kar, K. (2020). Nanocellulose-based polymer composites for energy applications—A review. J. Appl. Polym. Sci. 137, 48959. doi:10.1002/app.48959
Lavoine, N., Desloges, I., Dufresne, A., and Bras, J. (2012). Microfibrillated cellulose–its barrier properties and applications in cellulosic materials: A review. Carbohydr. Polym. 90, 735–764. doi:10.1016/j.carbpol.2012.05.026
Lee, E. (2018). Theory of electrophoresis and diffusiophoresis of highly charged colloidal particles. Germany: Academic Press.
Lengowski, E. C., Bonfatti Júnior, E. A., Kumode, M. M. N., Carneiro, M. E., and Satyanarayana, K. G. (2019). Nanocellulose in the paper making. Sustain. Polym. Compos. nanocomposites, 1027–1066. doi:10.1007/978-3-030-05399-4_36
Li, H., Li, J., Bodycomb, J., and Patience, G. S. (2019). Experimental methods in chemical engineering: Particle size distribution by laser diffraction—PSD. Can. J. Chem. Eng. 97, 1974–1981. doi:10.1002/cjce.23480
Li, M. C., Wu, Q., Moon, R. J., Hubbe, M. A., and Bortner, M. J. (2021). Rheological aspects of cellulose nanomaterials: Governing factors and emerging applications. Adv. Mater. 33, 2006052. doi:10.1002/adma.202006052
Li, W., Jiao, B., Li, S., Faisal, S., Shi, A., Fu, W., et al. (2022). Recent advances on pickering emulsions stabilized by diverse edible particles: Stability mechanism and applications. Front. Nutr. 738, 864943. doi:10.3389/fnut.2022.864943
Liu, C., Du, H., Dong, L., Wang, X., Zhang, Y., Yu, G., et al. (2017). Properties of nanocelluloses and their application as rheology modifier in paper coating. Industrial Eng. Chem. Res. 56, 8264–8273. doi:10.1021/acs.iecr.7b01804
Lokhande, P., Singh, P. P., Vo, D.-V. N., Kumar, D., Balasubramanian, K., Mubayi, A., et al. (2022). Bacterial nanocellulose: Green polymer materials for high performance energy storage applications. J. Environ. Chem. Eng. 10, 108176. doi:10.1016/j.jece.2022.108176
Lu, P. J., and Weitz, D. A. (2013). Colloidal particles: Crystals, glasses, and gels. Annu. Rev. Condens. Matter Phys. 4, 217–233. doi:10.1146/annurev-conmatphys-030212-184213
Mahfoudhi, N., and Boufi, S. (2017). Nanocellulose as a novel nanostructured adsorbent for environmental remediation: A review. Cellulose 24, 1171–1197. doi:10.1007/s10570-017-1194-0
Mao, Y., Liu, K., Zhan, C., Geng, L., Chu, B., and Hsiao, B. S. (2017). Characterization of nanocellulose using small-angle neutron, X-ray, and dynamic light scattering techniques. J. Phys. Chem. B 121, 1340–1351. doi:10.1021/acs.jpcb.6b11425
Martins, D., Estevinho, B., Rocha, F., Dourado, F., and Gama, M. (2020). A dry and fully dispersible bacterial cellulose formulation as a stabilizer for oil-in-water emulsions. Carbohydr. Polym. 230, 115657. doi:10.1016/j.carbpol.2019.115657
Mascheroni, E., Rampazzo, R., Ortenzi, M. A., Piva, G., Bonetti, S., and Piergiovanni, L. (2016). Comparison of cellulose nanocrystals obtained by sulfuric acid hydrolysis and ammonium persulfate, to be used as coating on flexible food-packaging materials. Cellulose 23, 779–793. doi:10.1007/s10570-015-0853-2
Matter, F., Luna, A. L., and Niederberger, M. (2020). From colloidal dispersions to aerogels: How to master nanoparticle gelation. Nano Today 30, 100827. doi:10.1016/j.nantod.2019.100827
Mendoza, L., Batchelor, W., Tabor, R. F., and Garnier, G. (2018a). Gelation mechanism of cellulose nanofibre gels: A colloids and interfacial perspective. J. colloid interface Sci. 509, 39–46. doi:10.1016/j.jcis.2017.08.101
Mendoza, L., Gunawardhana, T., Batchelor, W., and Garnier, G. (2018b). Effects of fibre dimension and charge density on nanocellulose gels. J. colloid interface Sci. 525, 119–125. doi:10.1016/j.jcis.2018.04.077
Mishnaevsky, L., Mikkelsen, L. P., Gaduan, A. N., Lee, K.-Y., and Madsen, B. (2019). Nanocellulose reinforced polymer composites: Computational analysis of structure-mechanical properties relationships. Compos. Struct. 224, 111024. doi:10.1016/j.compstruct.2019.111024
Moberg, T., Sahlin, K., Yao, K., Geng, S., Westman, G., Zhou, Q., et al. (2017). Rheological properties of nanocellulose suspensions: Effects of fibril/particle dimensions and surface characteristics. Cellulose 24, 2499–2510. doi:10.1007/s10570-017-1283-0
Moreirinha, C., Vilela, C., Silva, N. H., Pinto, R. J., Almeida, A., Rocha, M. a. M., et al. (2020). Antioxidant and antimicrobial films based on brewers spent grain arabinoxylans, nanocellulose and feruloylated compounds for active packaging. Food Hydrocoll. 108, 105836. doi:10.1016/j.foodhyd.2020.105836
Mujtaba, M., Lipponen, J., Ojanen, M., Puttonen, S., and Vaittinen, H. (2022). Trends and challenges in the development of bio-based barrier coating materials for paper/cardboard food packaging; a review. Sci. Total Environ. 851, 158328. doi:10.1016/j.scitotenv.2022.158328
Munier, P., Apostolopoulou-Kalkavoura, V., Persson, M., and Bergström, L. (2020). Strong silica-nanocellulose anisotropic composite foams combine low thermal conductivity and low moisture uptake. Cellulose 27, 10825–10836. doi:10.1007/s10570-019-02912-0
Nagarajan, K., Ramanujam, N., Sanjay, M., Siengchin, S., Surya Rajan, B., Sathick Basha, K., et al. (2021). A comprehensive review on cellulose nanocrystals and cellulose nanofibers: Pretreatment, preparation, and characterization. Polym. Compos. 42, 1588–1630. doi:10.1002/pc.25929
Niu, F., Li, M., Huang, Q., Zhang, X., Pan, W., Yang, J., et al. (2017). The characteristic and dispersion stability of nanocellulose produced by mixed acid hydrolysis and ultrasonic assistance. Carbohydr. Polym. 165, 197–204. doi:10.1016/j.carbpol.2017.02.048
Nordenstrom, M., Kaldéus, T., Erlandsson, J., Pettersson, T., Malmstrom, E., and Wågberg, L. (2021). Redispersion strategies for dried cellulose nanofibrils. ACS Sustain. Chem. Eng. 9, 11003–11010. doi:10.1021/acssuschemeng.1c02122
NordenstroM, M., Fall, A., NystroM, G., and WaGberg, L. (2017). Formation of colloidal nanocellulose glasses and gels. Langmuir 33, 9772–9780. doi:10.1021/acs.langmuir.7b01832
Noremylia, M., Hassan, M. Z., and Ismail, Z. (2022). Recent advancement in isolation, processing, characterization and applications of emerging nanocellulose: A review. Int. J. Biol. Macromol. 206, 954–976. doi:10.1016/j.ijbiomac.2022.03.064
Osong, S. H., Norgren, S., and Engstrand, P. (2016). Processing of wood-based microfibrillated cellulose and nanofibrillated cellulose, and applications relating to papermaking: A review. Cellulose 23, 93–123. doi:10.1007/s10570-015-0798-5
Pääkkö, M., Ankerfors, M., Kosonen, H., Nykänen, A., Ahola, S., Österberg, M., et al. (2007). Enzymatic hydrolysis combined with mechanical shearing and high-pressure homogenization for nanoscale cellulose fibrils and strong gels. Biomacromolecules 8, 1934–1941. doi:10.1021/bm061215p
Pagliaro, M., Ciriminna, R., Yusuf, M., Eskandarinezhad, S., Wani, I. A., Ghahremani, M., et al. (2021). Application of nanocellulose composites in the environmental engineering: A review. J. Compos. Compd. 3, 114–128. doi:10.52547/jcc.3.2.5
Panchal, P., Ogunsona, E., and Mekonnen, T. (2018). Trends in advanced functional material applications of nanocellulose. Processes 7, 10. doi:10.3390/pr7010010
Pedrosa, J. F., Rasteiro, M. G., Neto, C. P., and Ferreira, P. J. (2022). Effect of cationization pretreatment on the properties of cationic Eucalyptus micro/nanofibrillated cellulose. Int. J. Biol. Macromol. 201, 468–479. doi:10.1016/j.ijbiomac.2022.01.068
Pennells, J., Godwin, I. D., Amiralian, N., and Martin, D. J. (2020). Trends in the production of cellulose nanofibers from non-wood sources. Cellulose 27, 575–593. doi:10.1007/s10570-019-02828-9
Perdoch, W., Cao, Z., Florczak, P., Markiewicz, R., Jarek, M., Olejnik, K., et al. (2022). Influence of nanocellulose structure on paper reinforcement. Molecules 27, 4696. doi:10.3390/molecules27154696
Perumal, A. B., Nambiar, R. B., Moses, J., and Anandharamakrishnan, C. (2022). Nanocellulose: Recent trends and applications in the food industry. Food Hydrocoll. 127, 107484. doi:10.1016/j.foodhyd.2022.107484
Peters, S. J., Rushing, T. S., Landis, E. N., and Cummins, T. K. (2010). Nanocellulose and microcellulose fibers for concrete. Transp. Res. Rec. 2142, 25–28. doi:10.3141/2142-04
Phan-Xuan, T., Thuresson, A., Skepö, M., Labrador, A., Bordes, R., and Matic, A. (2016). Aggregation behavior of aqueous cellulose nanocrystals: The effect of inorganic salts. Cellulose 23, 3653–3663. doi:10.1007/s10570-016-1080-1
Pirozzi, A., Ferrari, G., and Donsì, F. (2021). The use of nanocellulose in edible coatings for the preservation of perishable fruits and vegetables. Coatings 11, 990. doi:10.3390/coatings11080990
Rahman, A., Wang, W., Govindaraj, D., Kang, S., and Vikesland, P. J. (2023). Recent advances in environmental science and engineering applications of cellulose nanocomposites. Crit. Rev. Environ. Sci. Technol. 53, 650–675. doi:10.1080/10643389.2022.2082204
Rai, R., and Dhar, P. (2022). Biomedical engineering aspects of nanocellulose: A review, Nanotechnology.
Raj, P., Batchelor, W., Blanco, A., De La Fuente, E., Negro, C., and Garnier, G. (2016). Effect of polyelectrolyte morphology and adsorption on the mechanism of nanocellulose flocculation. J. colloid interface Sci. 481, 158–167. doi:10.1016/j.jcis.2016.07.048
Raj, P., Blanco, A., De La Fuente, E., Batchelor, W., Negro, C., and Garnier, G. (2017). Microfibrilated cellulose as a model for soft colloid flocculation with polyelectrolytes. Colloids Surfaces A Physicochem. Eng. Aspects 516, 325–335. doi:10.1016/j.colsurfa.2016.12.055
Ranjbar, D., Raeiszadeh, M., Lewis, L., Maclachlan, M. J., and Hatzikiriakos, S. G. (2020). Adsorptive removal of Congo red by surfactant modified cellulose nanocrystals: A kinetic, equilibrium, and mechanistic investigation. Cellulose 27, 3211–3232. doi:10.1007/s10570-020-03021-z
Rasteiro, M., Garcia, F., Ferreira, P., Blanco, A., Negro, C., and Antunes, E. (2008). Evaluation of flocs resistance and reflocculation capacity using the LDS technique. Powder Technol. 183, 231–238. doi:10.1016/j.powtec.2007.07.024
Risoluti, R., and Materazzi, S. (2019). Mass spectrometry for evolved gas analysis: An update. Appl. Spectrosc. Rev. 54, 87–116. doi:10.1080/05704928.2018.1452252
Rozenberga, L., Vikele, L., Vecbiskena, L., Sable, I., Laka, M., and Grinfelds, U. (2016). “Preparation of nanocellulose using ammonium persulfate and method’s comparison with other techniques,” in Key engineering materials (USA: Trans Tech Publ), 21–25.
Saffarionpour, S. (2020). Nanocellulose for stabilization of Pickering emulsions and delivery of nutraceuticals and its interfacial adsorption mechanism. Food Bioprocess Technol. 13, 1292–1328. doi:10.1007/s11947-020-02481-2
Salas, C., Nypelö, T., Rodriguez-Abreu, C., Carrillo, C., and Rojas, O. J. (2014). Nanocellulose properties and applications in colloids and interfaces. Curr. Opin. Colloid & Interface Sci. 19, 383–396. doi:10.1016/j.cocis.2014.10.003
Sanchez-Salvador, J. L., Balea, A., Monte, M. C., Negro, C., Miller, M., Olson, J., et al. (2020). Comparison of mechanical and chemical nanocellulose as additives to reinforce recycled cardboard. Sci. Rep. 10, 3778–3814. doi:10.1038/s41598-020-60507-3
Sanchez-Salvador, J. L., Balea, A., Negro, C., Monte, M. C., and Blanco, A. (2022). Gel point as measurement of dispersion degree of nano-cellulose suspensions and its application in papermaking. Nanomaterials 12, 790. doi:10.3390/nano12050790
Sanchez-Salvador, J. L., Rasteiro, M. G., Balea, A., Sharma, M., Pedrosa, J. F., Negro, C., et al. (2023). Chitosan grafted/cross-linked with biodegradable polymers: A review. Sent Int. J. Biol. Macromol. 178, 325–343. doi:10.1016/j.ijbiomac.2021.02.200
Seydibeyoğlu, M. Ö., Dogru, A., Wang, J., Rencheck, M., Han, Y., Wang, L., et al. (2023). Review on hybrid reinforced polymer matrix composites with nanocellulose, nanomaterials, and other fibers. Polymers 15, 984. doi:10.3390/polym15040984
Shahzad, A., Ullah, M. W., Ali, J., Aziz, K., Javed, M. A., Shi, Z., et al. (2023). The versatility of nanocellulose, modification strategies, and its current progress in wastewater treatment and environmental remediation. Sci. Total Environ. 858, 159937. doi:10.1016/j.scitotenv.2022.159937
Shak, K. P. Y., Pang, Y. L., and Mah, S. K. (2018). Nanocellulose: Recent advances and its prospects in environmental remediation. Beilstein J. Nanotechnol. 9, 2479–2498. doi:10.3762/bjnano.9.232
Sharma, P. R., Sharma, S. K., Lindström, T., and Hsiao, B. S. (2020). Nanocellulose-enabled membranes for water purification: Perspectives. Adv. Sustain. Syst. 4, 1900114. doi:10.1002/adsu.201900114
Shimizu, M., Saito, T., Nishiyama, Y., Iwamoto, S., Yano, H., Isogai, A., et al. (2016). Fast and robust nanocellulose width estimation using turbidimetry. Macromol. Rapid Commun. 37, 1581–1586. doi:10.1002/marc.201600357
Shu, F., Guo, Y., Huang, L., Zhou, M., Zhang, G., Yu, H., et al. (2022). Production of lignin-containing nanocellulose from poplar using ternary deep eutectic solvents pretreatment. Industrial Crops Prod. 177, 114404. doi:10.1016/j.indcrop.2021.114404
Silva, F. A., Dourado, F., Gama, M., and Poças, F. (2020). Nanocellulose bio-based composites for food packaging. Nanomaterials 10, 2041. doi:10.3390/nano10102041
Smalyukh, I. I. (2021). Thermal management by engineering the alignment of nanocellulose. Adv. Mater. 33, 2001228. doi:10.1002/adma.202001228
Solhi, L., Guccini, V., Heise, K., Solala, I., Niinivaara, E., Xu, W., et al. (2023). Understanding nanocellulose–water interactions: Turning a detriment into an asset. Chem. Rev. 123, 1925–2015. doi:10.1021/acs.chemrev.2c00611
Song, H., Ankerfors, M., Hoc, M., and Lindström, T. (2010). Reduction of the linting and dusting propensity of newspaper using starch and microfibrillated cellulose. Nordic Pulp Pap. Res. J. 25, 519–521g. doi:10.3183/npprj-2010-25-04-p519-528
Spagnuolo, L., D'orsi, R., and Operamolla, A. (2022). Nanocellulose for paper and textile coating: The importance of surface chemistry. ChemPlusChem 87, e202200204. doi:10.1002/cplu.202200204
Szafraniec, M., Grabias-Blicharz, E., Barnat-Hunek, D., and Landis, E. N. (2022). A critical review on modification methods of cement composites with nanocellulose and reaction conditions during nanocellulose production. Materials 15, 7706. doi:10.3390/ma15217706
Tardy, B. L., Yokota, S., Ago, M., Xiang, W., Kondo, T., Bordes, R., et al. (2017). Nanocellulose–surfactant interactions. Curr. Opin. Colloid & Interface Sci. 29, 57–67. doi:10.1016/j.cocis.2017.02.004
Teo, S. H., Chee, C. Y., Fahmi, M. Z., Wibawa Sakti, S. C., and Lee, H. V. (2022). Review of functional aspects of nanocellulose-based pickering emulsifier for non-toxic application and its colloid stabilization mechanism. Molecules 27, 7170. doi:10.3390/molecules27217170
Tibolla, H., Pelissari, F. M., and Menegalli, F. C. (2014). Cellulose nanofibers produced from banana peel by chemical and enzymatic treatment. LWT-Food Sci. Technol. 59, 1311–1318. doi:10.1016/j.lwt.2014.04.011
Turbak, A. F., Snyder, F. W., and Sandberg, K. R. (1983). “Microfibrillated cellulose, a new cellulose product: properties, uses, and commercial potential,”Cellulose Conference, Syracuse, NY, May 24, 1982.
Van De Ven, T. G., and Sheikhi, A. (2016). Hairy cellulose nanocrystalloids: A novel class of nanocellulose. Nanoscale 8, 15101–15114. doi:10.1039/c6nr01570k
Vigneshwaran, N., Ammayappan, L., and Huang, Q. (2011). Effect of Gum Arabic on distribution behavior of nanocellulose fillers in starch film. Appl. Nanosci. 1, 137–142. doi:10.1007/s13204-011-0020-5
Wgberg, L., Decher, G., Norgren, M., Lindström, T., Ankerfors, M., and Axns, K. (2008). The build-up of polyelectrolyte multilayers of microfibrillated cellulose and cationic polyelectrolytes. Langmuir 24, 784–795. doi:10.1021/la702481v
Wågberg, L., and Erlandsson, J. (2021). The use of layer-by-layer self-assembly and nanocellulose to prepare advanced functional materials. Adv. Mater. 33, 2001474. doi:10.1002/adma.202001474
Wakabayashi, M., Fujisawa, S., Saito, T., and Isogai, A. (2020). Nanocellulose film properties tunable by controlling degree of fibrillation of TEMPO-oxidized cellulose. Front. Chem. 8, 37. doi:10.3389/fchem.2020.00037
Wang, Q., Yao, Q., Liu, J., Sun, J., Zhu, Q., and Chen, H. (2019). Processing nanocellulose to bulk materials: A review. Cellulose 26, 7585–7617. doi:10.1007/s10570-019-02642-3
Wen, Y., Wei, B., Cheng, D., An, X., and Ni, Y. (2017). Stability enhancement of nanofibrillated cellulose in electrolytes through grafting of 2-acrylamido-2-methylpropane sulfonic acid. Cellulose 24, 731–738. doi:10.1007/s10570-016-1182-9
Wu, T., Kummer, N., Kevin, J., Campioni, S., Zeng, Z., Siqueira, G., et al. (2021). Nanocellulose-lysozyme colloidal gels via electrostatic complexation. Carbohydr. Polym. 251, 117021. doi:10.1016/j.carbpol.2020.117021
Wu, Y., Liang, Y., Mei, C., Cai, L., Nadda, A., Van Le, Q., et al. (2022). Advanced nanocellulose-based gas barrier materials: Present status and prospects. Chemosphere 286, 131891. doi:10.1016/j.chemosphere.2021.131891
Xie, J., and Liu, S. (2021). A review of hydrophobic nanocellulose and its applications. Pap. Biomaterials 6, 35–42.
Xu, H., Sanchez-Salvador, J. L., Balea, A., Blanco, A., and Negro, C. (2022). Optimization of reagent consumption in TEMPO-mediated oxidation of Eucalyptus cellulose to obtain cellulose nanofibers. Cellulose 29, 6611–6627. doi:10.1007/s10570-022-04672-w
Xu, J.-T., and Chen, X.-Q. (2019). Preparation and characterization of spherical cellulose nanocrystals with high purity by the composite enzymolysis of pulp fibers. Bioresour. Technol. 291, 121842. doi:10.1016/j.biortech.2019.121842
Xu, T., Du, H., Liu, H., Liu, W., Zhang, X., Si, C., et al. (2021). Advanced nanocellulose-based composites for flexible functional energy storage devices. Adv. Mater. 33, 2101368. doi:10.1002/adma.202101368
Xu, Y., Atrens, A., and Stokes, J. R. (2020). A review of nanocrystalline cellulose suspensions: Rheology, liquid crystal ordering and colloidal phase behaviour. Adv. colloid interface Sci. 275, 102076. doi:10.1016/j.cis.2019.102076
Yan, N., Chai, X.-S., and Runge, T. (2021). A simple multi-wavelength spectroscopic method for the determination of carboxyl group content in nanocellulose. Cellulose 28, 2805–2811. doi:10.1007/s10570-021-03731-y
Yang, X., Han, F., Xu, C., Jiang, S., Huang, L., Liu, L., et al. (2017). Effects of preparation methods on the morphology and properties of nanocellulose (NC) extracted from corn husk. Industrial Crops Prod. 109, 241–247. doi:10.1016/j.indcrop.2017.08.032
Zhang, K., Ismail, M. Y., and Liimatainen, H. (2021a). Water-resistant nanopaper with tunable water barrier and mechanical properties from assembled complexes of oppositely charged cellulosic nanomaterials. Food Hydrocoll. 120, 106983. doi:10.1016/j.foodhyd.2021.106983
Zhang, M., Du, H., Liu, K., Nie, S., Xu, T., Zhang, X., et al. (2021b). Fabrication and applications of cellulose-based nanogenerators. Adv. Compos. Hybrid Mater. 4, 865–884. doi:10.1007/s42114-021-00312-2
Zhang, W., Zhang, Y., Cao, J., and Jiang, W. (2021c). Improving the performance of edible food packaging films by using nanocellulose as an additive. Int. J. Biol. Macromol. 166, 288–296. doi:10.1016/j.ijbiomac.2020.10.185
Zhu, J., Xie, S., Yang, Z., Li, X., Chen, J., Zhang, X., et al. (2021a). A review of recent advances and prospects on nanocellulose properties and its applications in oil and gas production. J. Nat. Gas Sci. Eng. 96, 104253. doi:10.1016/j.jngse.2021.104253
Keywords: nanocellulose self-assembly, suspension stabilization, colloids, cellulose nanofibrils, cellulose nanocrystals, electrostatic stabilization, steric repulsion
Citation: Sanchez-Salvador JL, Xu H, Balea A, Negro C and Blanco A (2023) Nanocellulose from a colloidal material perspective. Front. Mater. 10:1231404. doi: 10.3389/fmats.2023.1231404
Received: 30 May 2023; Accepted: 10 July 2023;
Published: 25 July 2023.
Edited by:
Zhiyong Gao, Central South University, ChinaReviewed by:
Liang Liu, Nanjing Forestry University, ChinaWenfei Wei, Chinese Academy of Sciences (CAS), China
Copyright © 2023 Sanchez-Salvador, Xu, Balea, Negro and Blanco. This is an open-access article distributed under the terms of the Creative Commons Attribution License (CC BY). The use, distribution or reproduction in other forums is permitted, provided the original author(s) and the copyright owner(s) are credited and that the original publication in this journal is cited, in accordance with accepted academic practice. No use, distribution or reproduction is permitted which does not comply with these terms.
*Correspondence: Angeles Blanco, YWJsYW5jb0B1Y20uZXM=