- 1Chemistry Department, Faculty of Science, Kuwait University, Kuwait City, Kuwait
- 2Engineering Department, Faculty of Marine Technology and Natural Sciences, Klaipeda University, Klaipeda, Lithuania
- 3Nicholas Copernicus University, Faculty of Chemistry, Torun, Poland
As a key kind of evolving carbonaceous adsorbent, nitrogen-enriched activated carbon has drawn a lot of focus due to its better physiochemical ability to eliminate an extensive range of wastewaters contaminants under severe conditions. Its environment-friendly character is one more reason behind this focus. Nitrogen also has immense effect on activated carbon structures’ pollutants adsorption capability; therefore, it is an area of interest. Reports concerning the reaction pathway of C-N (carbon-nitrogen) bond creation on AC surface are limited. Determining such mechanisms is challenging but critical to understand bond characteristics after carbonization. Moreover, it is vital to ascertain real-time kinetics concerning adsorption phenomena in liquid phase. Such a latest trend indicates that regulated nitrogen uses for carbonaceous substances having a biomass-based origin can provide the desired morphological characteristics produced through interconnections, production of enclosed holes, enhanced surface area, better adsorption ability, and many other benefits in contrast to conventional carbon-based substances. This review points out the developments in the main processes to introduce nitrogen atoms into the carbon matrix by utilizing different N-comprising chemical compounds. The nitrogen enrichment processes, reaction mechanisms and effects of nitrogen incorporation on the plant biomass-derived activated carbons (NEACs) are presented in brief. On the basis of their established physicochemical attributes, the adsorption performances of different biomass-derived NEACs have also been dealt with. More significantly, the review covers the technical issues in the present phase, topical trends, research gaps, economic viability along with a technical alignment recommendation to address the prevailing disadvantages.
Introduction
Generally, plant biomass-based carbon materials carry a specific appeal for academics and scientists. The activated carbon (AC) produced through plant-biomass carbonization demonstrates better chemical attributes, ample resources, low cost, biocompatibility, and matured porous structures over conventional petroleum-based and coal-based carbon materials. Simultaneously, addition of nitrogen atom within the carbon structure offers ample functional groups, sturdier adsorption ability and greater chemical solidity (Shi et al., 2012; Pei et al., 2018). The N-enriched ACs (NEACs) might possess enhanced properties like better-formed surfaces and pore morphologies, which could offer a reaction medium transfer channel and enhance the usage domain of carbon-based substances (Wang et al., 2012; Li et al., 2019; Bumajdad and Khan, 2021). Hence, a comprehensive and integrated study concerning AC production using several plant species and development of N-atom insertion into AC skeleton and associated applications concerning the elimination of many wastewater pollutants through adsorption is an interesting scientific domain. Several studies have shown that nitrogen enrichment has a significant impact on the surface attributes like surface basic sites, surface polarity, electrical conductivity, and electron donor. As far as adsorption is concerned, nitrogen doping is helpful in enhancing the hydrophilicity that improves the diffusion of the hydrophilic adsorbents within the aqueous media (Lorenc-Grabowska et al., 2013; Lupul et al., 2015; El-Shafey et al., 2016). Additionally, nitrogen doping may cause the development of surface charges that enhance electrostatic interactions between the adsorbate and sorbent; consequently, sorption ability is enhanced.
Of late, many studies (Jiang et al., 2011; Lim et al., 2012; Sakaushi and Antonietti, 2015; Deng et al., 2016) have shown that NEACs have demonstrated improved capability for the sequestration of extensively ranged contaminants from aquatic medium. Increased adsorption efficacy was associated with the electron releasing influence by the nitrogen-based functional groups introduced into ACs (Lorenc-Grabowska et al., 2013). The N-species treatment enhance the development of nitrogen-based functional groups on the porous surface of the carbon substrate; moreover, it influences the effective use of heteroatom synergistic characteristics and may results in fast adsorption of assimilated materials, thereby improving cycling stability. Hence, a precise understanding concerning nitrogen enrichment processes, reaction processes, and essential reaction factors influencing adsorption are critical for developing efficacious adsorbent materials, experimental design, enhancing process scale, and decision-making.
The process of thermochemical manufacturing of ACs from plant biomasses and their N-fortification has been schematically demonstrated in Figure 1. Generally, plants biomasses are heated up in a passive environment for evading oxidation. This process comprises the use of highly pure nitrogen (N2) gas for purging the reaction chamber in order to eliminate oxygen, air, and other emanating gases that might cause biomass oxidation leading to ash formation instead of the required ACs. The procedure also makes sure that biomass experiences devolatilization and drying concurrently which causes the creation of a superior heating value and better porous ACs. Chemical enrichment, usually called doping, with foreign atoms is an effectual technique for attaining the above-stated characteristics of carbon-based material. Several dopants, other than nitrogen, have been introduced into the carbon matrix, including phosphorous, boron, sulphur, and oxygen. Of the various available alternatives, nitrogen doping of carbon materials has drawn the attention of academics engaged in the adsorption methods because of its good ability to improve the significant attributes for optimal adsorption in the liquid stage (Wood et al., 2014; Hussain et al., 2018). Apart from the heteroatom outcome, the formed ACs’ properties like specific surface area and porosity are determined by plant species, nature, and plant parts like root, trunk, leaves, stem, etc. Furthermore, heating properties such as duration, rate, and temperature also affect AC characteristics.
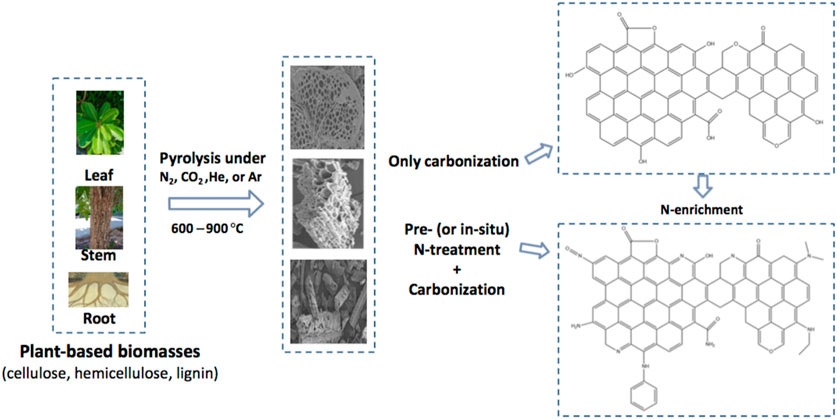
FIGURE 1. Schematic pathway of production and nitrogen enrichment of activated carbons (NEACs) form plant biomasses.
However, scaling-up adsorption by NEACs remain restricted because of the self-aggregated substrate-free characteristics that cause recycling and isolation challenges. Nevertheless, some research works have noted that, nitrogen-comprising adsorbents are not quite effective because of inferior nitrogen enrichment and intricacy of the operating processes that reduce their competitiveness. Hence, in order to enhance NEAC adsorption efficacy, extensive research has been conducted concerning N-development on carbon substrates to address the specified challenges and exploit the remarkable characteristics like greater surface area, appropriate surface characteristics, and appropriate pore dimensions.
Liquid phase-specific adsorption is a complicated process, and the underlying aspects are not understood as much. A vital aspect specific to the use of N-enriched ACs for treating an aqueous environment is that organic and inorganic material must be concurrently removed in order to purify water (Yang et al., 2019; Zuo et al., 2019). Hence, identifying better processes for increasing nitrogen content in adsorbents for increasing pollution elimination efficacy is desirable but difficult. Even with up to date progresses, the cost-efficient and larger scale production of NEACs is still very difficult-to develop, although low-cost functionalized ACs production has been a remarkable research domain in the field of material science and engineering. The superior material characters, like, larger specific surface area, fine particle size distribution, unique electrochemical properties, greater energy density and chemical stability are the key techno-economical points to receive enormous investments in the development of industrial-scale production plant for NEACs production (Jiang et al., 2013; Zhang S. et al., 2019). To date, a detailed assessment concerning the innovations in nitrogen-enriched biomass-based activated carbon (NEAC) and adsorption characteristics remains to be conducted. This review presents three critical technical roadblocks, (i) the N-enrichment procedures onto the biomass-derived AC along with the reaction mechanism (ii) characteristic transformations due to N-enrichment and (iii) efficacy of NEACs in eliminating pollutants from wastewater along with latest research initiatives to develop cost-efficient process development. Lastly, the review suggests future recommendations and research gaps concerning this domain.
Methodologies and reaction mechanism of nitrogen enrichment of activated carbon
Improvement of quality ACs having high nitrogen content is among the latest research trends specific to the adsorption field (Ilnicka and Lukaszewicz, 2015; Ilnicka et al., 2018). Considering the extensive use of AC-based substances for several environmental and experimental uses, it is vital to ascertain the comprehensive reaction phenomena that lead to the creation of nitrogenous functional groups on AC surface and their effects concerning aqueous phase adsorption. Nevertheless, there is information scarcity concerning the creation of N-functionalities through the use of valuable nitrogen-based reagents. Information concerning this domain is expected to significantly enhance biomass resource usage efficiency. Hence, a comprehensive assessment concerning N-enrichment processes for plant-based ACs is required (Chen et al., 2018).
The literature comprises several ways to enrich porous carbon with nitrogen. The commonly-used heat-treatment techniques may be divided into three highly used processes: (i) a two-step technique using nitrogen functionalisation and subsequent chemical activation (pre N-enrichment); (ii) a two-step technique comprising chemical activation and subsequent nitrogen functionalisation (post N-enrichment); and (iii) a single-stage technique comprising subjecting the biomass to a nitrogen donor and chemical activator to direct heat treatment (also called in-situ N-enrichment) (Liu Y. et al., 2019). The first method, which comprising pre-nitrogen enrichment, can leads to eliminating nitrogen functional groups due to the chemical etching that should follow the activation steps (Chen et al., 2018); thus, this method is not a rational option for increasing the amount of nitrogen content. In contrast, the post nitrogen enrichment-based (technique ii) causes activation to produce a porous morphology that facilitates deep penetration of the nitrogenous substance, thereby leading to effective doping of N. Nevertheless, the free radicals specific to the nitrogenous substances have a reaction affinity for surface functional groups having oxygen; therefore, there is a typical need for a reasonable degree of surface oxidation (Laheäär et al., 2014). The third technique, i.e., in-situ preparation, comprises chemical activation leading to interactions with the nitrogenous substances; consequently, modification phenomena lead to presently unknown effects. Figure 2 summaries typical chemical- and heat-based N-enrichment processes.
Nitrogen enrichment by means of the so-called ‘doping’ approach has been extensively recommended since past decade. Doping is described generally as the procedure of deliberate adding of tiny impurities to a material to adjust its attributes. The quality of the impurity (dopant) describes the attributes of the material post accomplishment of the doping procedure. Explicitly, the chemistry concerning this process is the heteroatom nitrogen dopants enhancing capacitive characteristics by augmenting carbon-surface charge density; additionally, basicity and wettability of carbon substrates are also enhanced (Shin et al., 2011) because of the enhancement of the electron density of the N-based dopant. It provides adsorbents with distinct molecular properties and several functional groups that cause a change in efficiency (Chen et al., 2012; Zhao, Y. et al., 2012; Pan et al., 2012; Nahar et al., 2013). Moreover, most studies assessing this subject use isolated phases, namely, carbonizing biomass at initial stage using appropriate activators, and nitrogen atom insertion through nitrogen doping. It is established that oxygen from biomass influences doping and activation (Houshmand et al., 2011). Therefore, it is appropriate to merge the three steps. Consequently, there is a need for further research specific to two areas: degree of reactivity of nitrogen-providing reagents and pore forming agents, for which pore tailoring and nitrogen dopant processes can be affected. Secondly, since different activating chemicals cause unique interactions when carbonized with plant-based biomasses, this must be another research target.
The acid/base characteristics of AC materials are subject, to a large degree, not only to the raw materials or their porosity and the structure of their surfaces but also to the heteroatoms incorporated in their structures. The most significant and extensively introduced heteroatoms on the surface of AC are nitrogen and oxygen. The acidic nature of porous carbon surfaces is closely associated with their oxygen-based surface groups which are chiefly present on the external surface or at the boundary of the basal plane and is contributory to the chemical character of the carbon. Conversely, the basicity of AC is related to: (i) resonating-electrons of aromatic rings of carbon that attract protons, and (ii) basic functionalities of surface that are able to bind with protons. Nitrogen functionalities usually provide basic characteristics, which can increase the interactions between the acid molecules and carbon surfaces by introducing dipole-dipole, covalent bonding, hydrogen bonding, etc. (Wenzhong et al., 2008; Shen and Fan, 2013). Different kinds of chemical compounds have been recommended to be used in the production of ordered micro-to meso-porous nitrogen-rich carbons; for instance, compounds having amine groups (ethylamine, ethylmethylamine, propylamine, trimethylamine, etc.), quaternary ammonium salts and surfactants, N-substituted amides, etc. Such appropriate N-doping materials undergo a reaction with plant biomasses and lead to a notable fraction of nitrogen in the ACs (Qu et al., 2015; Shi et al., 2015; Watanabe et al., 2015). Physiochemically created NEACs generally possess microporous carbon and sufficient surface area (Pietrzak et al., 2006; Zhao et al., 2014). The subsections that follow will address the evolution of the nitrogen enrichment techniques, reaction mechanisms along with the changes in the chemistry of the surface of the ACs.
Nitrogen enrichment through amine
Amines are compounds consisting of hydrocarbons (which are in the form of aryl or alkyl, R) with nitrogen-containing group (R2NH, RNH2, or R3N). Nitrogen functional groups can be generated on the carbon surfaces via acid-base reactions or polar and electrostatic interactions. Their amount is greatly enhanced when the carbon surface polarity increases, and acidic groups are present. Thus, preferring plant-based biomass (comparatively reactive material) for N-processing by amines has been recommended for several years, because it can be oxidized easily to a great extent. Subjecting the oxidized biomass to amines treatment should result in variations in the quantity of amine groups preserved on the surface and usually in variations in the chemistry of oxygen- and nitrogen-containing surface groups. Such groups are supposed to affect the rate of adsorption of several pollutants (Salame and Bandosz, 2003). To understand the effects of the amine modifications on the behavior of carbon as pollutant adsorbents, the surface of carbons before and after amination need to be analyzed in detail.
Several impregnation methods on the adsorbents have been reported in the literature. Of which, the wet impregnation method is regarded to be the easiest way for mounting amines onto the solid surfaces. In this method, for solid N-precursor, a solvent is used to mix it with the support material (the biomass). The diluted solution is then evaporated to get rid of the excess solvent. Minimized volatilization behaviour as well as higher amine capacity can be obtained through impregnation with intermediate molecular weight amines, including methyl diethanolamine (MDEA), diethanolamine (DEA) or tetraethylenepentamine (TEPA) (Heydari-Gorji et al., 2011; Builes et al., 2013). It has been reported that various amines that range from large amino polymers to simple monoamines can be employed for impregnation of amines to yield NEACs, as schematically represented in Figure 3.
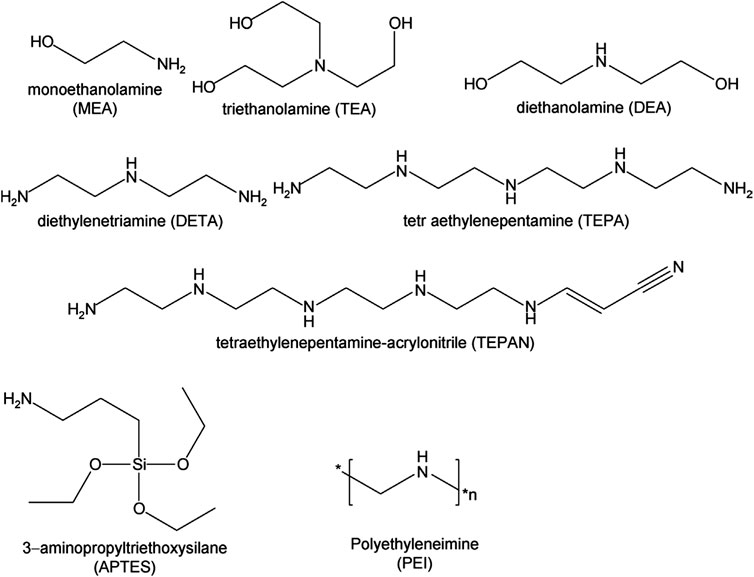
FIGURE 3. Some common amine sources used in solid adsorbents. Reproduced with permission from Elsevier publication (Ünveren et al., 2017).
Another common technique involves introducing the amine groups on the activated carbon’s surface via gas amination. Gas amination, that is, AC reacting with gaseous ammonia (NH3(g)), is carried out to surge the nitrogen content of AC and introduce nitrogen-containing groups that are chemically bonded with the carbon matrix. In general, such nitrogenous groups strengthen AC’s basic behaviour and hydrophobic characteristics (Biniak et al., 1997; Radkevich et al., 2008). Amination could be performed over a broad range of temperatures (200°C–900°C). The difference between high-temperature amination and low-temperature amination results in different modifications to the surface chemistry of the ACs as well as the AC’s textural properties. Particularly, low-temperature amination usually generates groups like amines, amides, lactams, nitriles and imides (Jansen and van Bekkum, 1994; Mangun et al., 2001), while high-temperature amination results in creation of groups like pyrrole, quaternary ammonium, pyridines and a possible formation of oxides of nitrogen (Szymański et al., 2004). This advocates the fact that amination carried out at high temperatures leads to increase in AC’s thermal stability as nitrogen atoms get incorporated into carbon rings (Biniak et al., 1997). Most likely, high-temperature amination occurs through creation of radicals of NH, NH2 and H (Houshmand et al., 2011). When treating carbon materials with NH3(g) under high temperatures, decomposition of NH3 occurs in order to release free radicals such as •NH and •NH2, as well as atomic nitrogen and hydrogen. These free radicals tend to attack the carbon in order to yield nitrogen-containing functional groups. These radicals could also, etch carbon fragments, resulting in changes with regards to the porosity, and could also substitute oxygen-containing species on the carbon in order to facilitate the formation of surface groups like–CN, –NH2, quaternary nitrogen, and pyrrolic. Such differences that exist between low- and high-temperature amination maybe also influenced by the initial surface chemistry of the AC. Functional groups that are present on the surface of the AC could impact the kind as well as the degree of amination. When carboxyl groups are present on the surface and amination occurs at low temperatures, reaction of ammonia with carboxyl may also occur in order to generate amides, lactams, amine or imides, as presented in Figure 4.
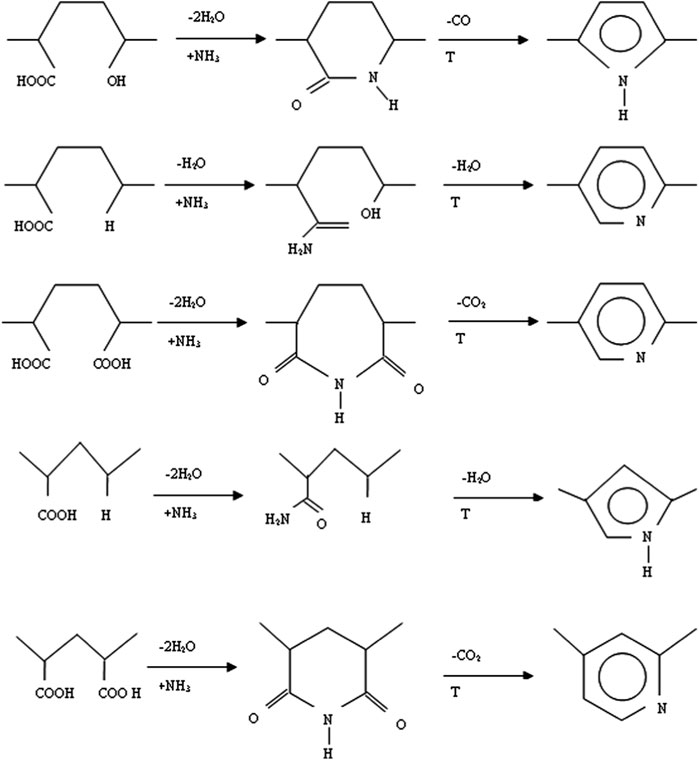
FIGURE 4. Various reactions of ammonia gas with carboxyl groups. Reproduced with permission from Elsevier publication (Jansen and van Bekkum, 1994).
High-temperature amination of pre-annealed and pre-oxidized ACs was compared by Biniak et al. (1997) and they found that pre-treating AC with nitrogen-enriching agents could result in pyrrole-like groups. As per Pevida et al. (2008), at temperatures more than 600°C, nitrogen gets incorporated into aromatic rings, while at lower temperature ranges, nitrogen forms more labile functionalities (e.g., amide-like functionalities) with easier thermal decomposition.
The key aspect relating to this analysis is to understand the alteration in chemistry after introduction of nitrogen onto the plant-derived ACs. As indicated elsewhere, surface acidity of carbons has a significant effect on the chemistry of the species containing nitrogen (El-Sayed and Bandosz, 2002). The plant biomass-based carbons generally contain additional oxygen-containing groups. Considering the basicity nature of amine molecules, amides, amines and carboxylate groups are likely to be the products because of surface reactions (Weast and Astle, 1982). The ligand strength of the AC’s surface is enhanced by the inherent functional amine (-NH2) groups that are attached to the AC, thus improving the sorbent material’s affinity towards transition-adsorbate cations. Furthermore, amine gets strongly adsorbed through hydrogen bonding on the oxygen-containing groups that are present on the AC surface (carbonyl, hydroxyl, quinone, ether, etc.) (Deliyanni and Bandosz, 2011). As per certain research groups, the most commonly employed reagent to introduce nitrogen into ACs is ammonia, NH3, which is employed alone or post carbon pre-oxidation with NH3–air gas mixtures (ammoxidation). To nitrogenate ACs, N,N-dimethylethanolamine, HCN, dicyanodiamine, N,N-dimethylpropanediamine, urea, polyaniline and melamine have also been employed (Shen and Fan, 2013; Luo et al., 2014; Sun et al., 2014; Zhang et al., 2016). Normally, the process includes a single nitrogenization stage or may involve two successive steps, in which oxidation of porous carbon is done first in liquid phase, which is then nitrogenated in liquid or gas phase. The nitrogenization treatment of the AC material can impact both its porous structure as well as surface chemical nature in a form, which to an extent, relies on its structure, the chemical agent employed, and the employed experimental technique. Ammoxidation (gas phase) and amination (aqueous phase) are frequently employed treatment methods to increase AC’s basicity (Daud and Houshamnd, 2010; Belhachemi and Addoun, 2011; Yang et al., 2014).
However, some nitrogen-treatment methods (e.g., ammoxidation) could also result in decrease in ACs’ porosity (Shen and Fan, 2013). Impregnation of amine/s could lead to development of amine-functional groups inside the microchannels, which could decrease the specific surface area when compared with the virgin biomass-ACs (Ali et al., 2018). Conversely, the literature mentions that ACs possessing high nitrogen functional group concentrations prepared via NH3(g) treatment demonstrated better adsorption abilities, particularly, for adsorption of transition metal ion species from aqueous solution when basic or neutral conditions are maintained (Jia et al., 2002). In this case, the controlling types of adsorption process occur because of the superior impact pertaining to developed chemical functional groups (Khalil et al., 2012; Zhang et al., 2014). The amounts of adsorbed transition metal ions could be seen to correlate with that of the nitrogen-rich carbons’. The enhanced adsorption of metal cations ‘M+’ has been suggested to be the result of coordination with nitrogen functional groups (Le Leuch and Bandosz, 2007). The hydrolysis of NEAC in the liquid medium promotes the formation of NH/:NH2-M complex into nitrogen-enriched carbon skeleton. When compared with pyrrolic groups, pyridinic groups are more basic, and thus it is suggested that these behave as ligands by forming surface species analogous to the coordination compounds as expressed in Figure 5.
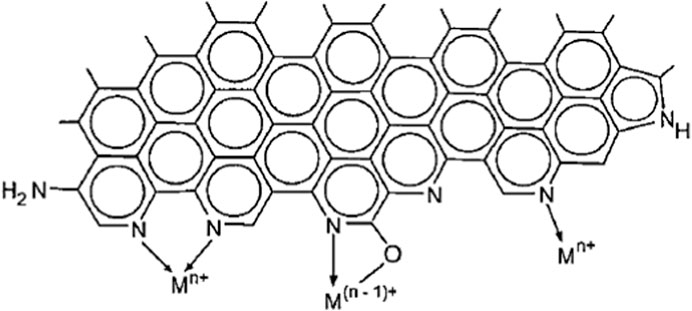
FIGURE 5. A schematic diagram of possible surface nitrogen functional groups in activated carbon for adsorption of transition metal ions. Reproduced with permission from ACS publication (Jia et al., 2002).
Also, it has been seen that at higher temperature (500°C–900°C), a reaction of •NH2, NH3 and •NH with carbonyl groups could occur via Maillard reaction (Xu et al., 2020), suppressing decarboxylation and decarbonylation as well as prohibiting the formation of CO2 and CO.
NH3 as well as its free radicals (•NH, •NH2, and •H) are heavily engaged in the AC surfaces under higher temperature, which also results in incorporating many nitrogen-containing functional groups to the surface of carbon. Researchers have noted interesting impacts of NH3 modification on customizing the micro- and mesopore structure with a reasonable specific surface area. The substitutional doping of heteroatoms like nitrogen leads to generation of active sites and then followed by a violent etching action to yield a high value of surface area. Chen et al. (2016) evaluated the impact of adding NH3(aq) as well as the activation agent KOH, on a plant biomass (bamboo). They identified that the nitrogen amount considerably increased to 10.4 wt%, demonstrating a strong interaction impact for combining chemical activation with NH3(aq) modification. Also, via the one step method, the strong interaction impact between chemical activation and modification of NH3(aq) has been found to yield larger pore volumes and pore diameters. Many oxygen-containing functional groups are generated at the pores due to chemical activation by the action of etching decomposition of the plant biomass. The interaction of NH3 and its free radicals (•NH, •NH2 and •H) with them and with the carbon walls, under thermal condition, release several gases thereby increases porosity and allows the formation of new pores (Zhang et al., 2016). When plant biomass-based ACs undergo hydrothermal treatment, several chemical reactions could occur in the presence of NH3(aq), thus leading to complex organic compounds formation (Mangun et al., 2001). As per a comparison study by a research group regarding the impact of NH3(aq) treatment of AC under CO2 activation, the textural characteristics and N contents inside the activated N-doped carbon can be controlled by changing the activation temperatures (Gu et al., 2017). The authors identified that there is a distinct increase in the pore volumes and specific surface areas from 0.27 to 1.384 cm3/g, and 551–2,813 m2/g, respectively, with rise in the activation temperature from 800°C to 1,000°C. However, an opposite trend was shown by the N contents which is a decrease from 4.53 to 2.38 atom%. It clearly shows that the carbon framework is activated via the doped nitrogen groups, which is helpful for activation during high temperatures, and thus applying ammonia treatment on the carbon may give larger surface area through increased porosity. In these activated samples, the concurrent presence of both mesopores and micropores could also contribute towards the adsorption of different ions/molecules (Xia et al., 2008; Mochizuki et al., 2022). It is worth mentioning here that, at elevated thermal treatment, the increase in overall volume of porosity might accompanied with a decrease in the extent of microporosity, render the NEAC a good adsorbent for large adsorbates but reduce its efficiency toward smaller ones (Gu et al., 2017; Mochizuki et al., 2022).
Based on these observations, researchers recommend that doping nitrogen by employing amine compounds is a key method to improve adsorptive performances for a majority of the adsorbates, particularly, to eliminate organic pollutants from liquid phase. Beside inorganic contaminants, N-rich ACs can be utilized for removal of different organic molecules. For example, Figure 6 demonstrates a plausible mechanism for some organic components adsorption (atrazine (ATZ), diuron, and quinoline (QUI), diclofenac, indole (IND) and dibenzothiophene (DBT)) onto the N-enriched carbon.
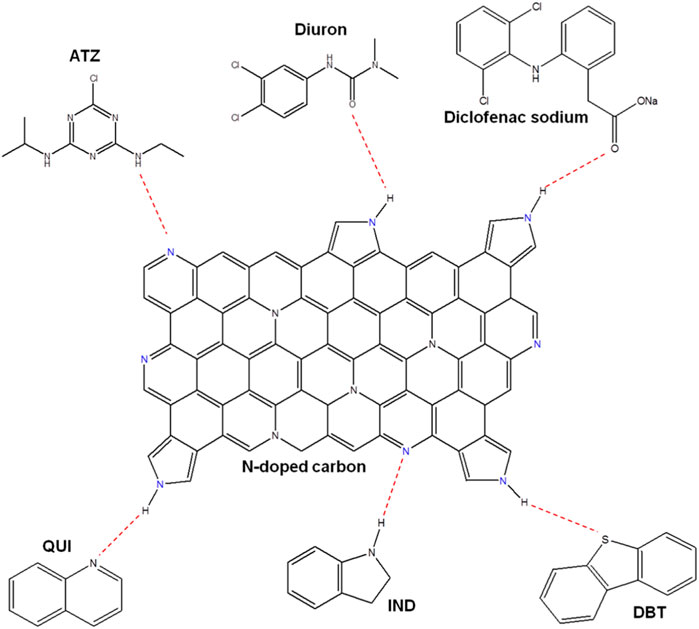
FIGURE 6. Plausible Adsorption Mechanism of the Six Adsorbates over nitrogen enriched activated carbon; nitrogen sites are highlighted in blue. Reproduced with permission from ACS publication (Ahmed et al., 2017).
As per the findings of earlier studies, certain interactions have been seen to occur between amines and activating agents which affect the characteristic of ACs; however, it is still not clear how such interactions occur.
Nitrogen enrichment through amide treatment and amidation
Introducing amide functionalities into the carbon skeleton may leads to formation of N-functional groups via exhaustion of less energy (functionalization at low temperatures, 200°C–400°C), which has positioned process to becomes favorable if compared with the direct introduction of amines functionalities (need high temperature). Multistep amidation/amination have been recommended by researchers (Mostazo-López et al., 2015), that is, post-modification of a porous activated carbon by incorporating N functional groups via organic reactions (can be seen in Figure 7). The modification approach comprises: (i) chemical oxidation by HNO3 in order to generate carboxylic acids (Figure 7; “KUA-COOH”) where KUA stand for “activated carbon”; (ii) amidation (formation amides and their cyclic derivatives such as of lactams, imides and may also produce pyridine, pyridine and pyrrole when CO-desorption occurs) via incorporating with acyl chloride followed by treatment with NH4NO3 (Figure 7; “KUA-CONH2”); (iii) via Hofmann rearrangement, converting amides into amine groups (amines, pyridine and pyrroles) (Figure 7; ‘KUA-NH2').
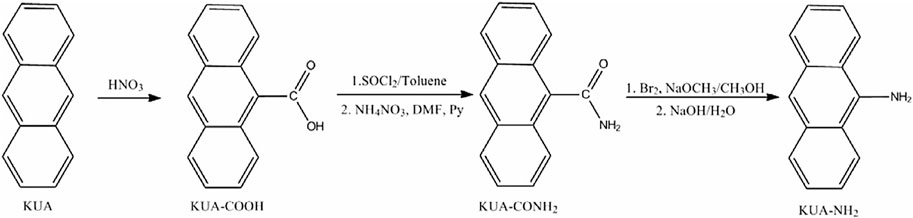
FIGURE 7. Preparation of NEACs through chemical oxidation (KUA-COOH) followed by amidation (KUA-CONH2) and amination (KUA-NH2). The used reagents are shown on the figure. Reproduced with permission from Elsevier publication (Mostazo-López et al., 2015).
Urea has been regarded as the most widespread and cost-effective amide that is chosen for performing the multiphase reactions with biomasses. Nitrogen processing through usage of urea is one of the most efficient methods resulting in nitrogen insertion into carbonaceous substances as, after effective completion of reaction, it may offer the generation of substantial quantity of stable nitrogen groups on the surface of carbon (Pietrzak et al., 2009). The ACs obtained in such a manner is characterised by a large quantity of nitrogen functional groups, especially of the types of pyridinic, pyrrolic, and quaternary nitrogen groups (Adib et al., 2000; Vidic and Siler, 2001; Bumajdad and Hasila, 2023). It is noteworthy that when heteroatoms such as nitrogen and oxygen are present, it determines the basicity and acidity of the surface of ACs, respectively (Gregg, 1934; Radovic, 2004). During reaction, the presence of groups with oxygen not only interacts with urea molecules but also facilitates the surface reactions as displayed in the methods presented in Figure 8.
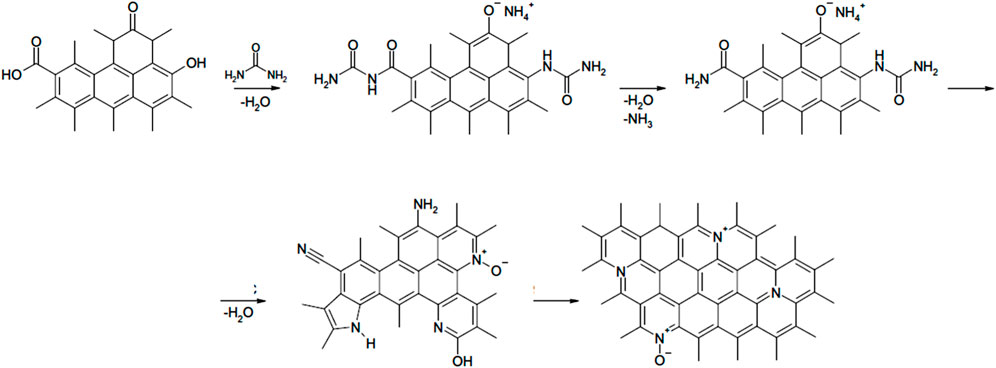
FIGURE 8. Possible chemical reactions of urea with surface functional groups and thermal transformations. Reproduced with permission from Elsevier publication (Rao et al., 2019b).
Various studies have shown that the developed nitrogenous group onto the AC, which is the result of urea treatment, play a key role with regards to adsorption performance in the aqueous media (Rao et al., 2019b). In order to determine the thermochemical reaction factors, it is imperative to understand the mechanism of nitrogen functional groups formation as well as stabilisation of active carbons during urea treatment.
The temperature alteration (thermal treatment in an inert atmosphere) as well as the oxygen containing groups are considered to play a key role in attracting urea molecule onto the surface of carbon atom. The first step involves reaction of oxygen-containing groups of carbon with urea, which then get hydrolysed. In the next step, amination occurs in the location where nitrogen groups get formed onto the active carbon. Hydrolysis is initiated with the thermal treatment, while at lower temperature, partial decomposition occurs with the formation of active nitrogen groups. Additional active nitrogen atoms are generated onto the carbon surface with increment in temperature (at 950°C) (Seredych et al., 2009). Pels et al. (1995) studied the evolution of N-functionalities as a result of thermal treatment. They observed a decreasing ratio for pyrrolic to pyridinic nitrogen with rise in temperature or prolonged heating (pyrrolic converted to pyridinic and quaternary nitrogen). This is because pyrrolic structure is less thermally stable than pyridinic and quaternary structures. Another important observation is the similarity of the present N-functionalities if subjected to severe thermal treatment regardless of the carbon precursor origin. It is interesting to note that the above-mentioned type of nitrogen functionalities can exist at post treatment with urea at higher temperature (950°C), and with much higher content. This could be due to the differences of the chemistries of the urea molecule under different thermal treatments as well as the mechanisms and kinetics of the reactions (Schaber et al., 2004). The complete reaction dynamics related to the development of urea-treated nitrogen functional groups as well as stability onto the AC is yet to be explained comprehensively.
Beside the surface area and porosity, the presence of acidic groups onto the AC may affect the adsorption of nonpolar as well as polar organic compounds from aqueous solution (Pendleton et al., 2002). This is because of the preferential adsorption of water on carbon surfaces that include oxygen groups. Adsorbing by water could occur through hydrogen bonds present on oxygen-containing groups, followed by additional clustering of water molecules on these sites (Boehm, 1994; El-Sayed and Bandosz, 2005). The water clusters that result could also block pollutant access to hydrophobic sites, chiefly micropores, which leads to decrease in the interaction energy between the adsorbent surface and the adsorbate molecule. On carbon modified with urea, a similar behaviour could be observed (El-Sayed and Bandosz, 2003). The strength of hydrogen bonding (that exists between water and the surface sites), the chemistry of such active sites, and their position on the surface all of which play an important role in determining the level of competition between water and polar adsorbate toward the adsorption sites. Due to their capability to bond strongly with the carbon surface oxygen, different kinds of amines could compete with water more efficiently than other adsorbates (El-Sayed and Bandosz, 2003).
Nitrogen enrichment using nitric acid
Treating ACs with HNO3 was acknowledged as a technique to inclusion of more oxygen-surface groups on the ACs surface via oxidation reactions. The tentative oxidation reaction mechanisms can be explained like Figure 9.
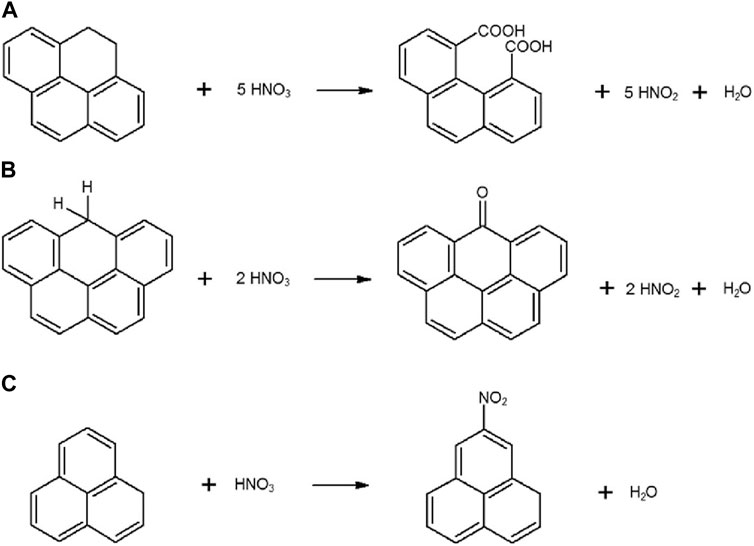
FIGURE 9. Oxidation methods for the enrichment of nitrogen functional groups of ACs with nitric acid. Reproduced with permission from Elsevier (Ternero-Hidalgo et al., 2016).
During HNO3 treatment, the aliphatic chains are altered, in this stage the aromatic rings remain same due to their better stability. It can be noticed from Figure 9A that the dicarboxylic group formed when aliphatic chain contains several carbon atoms, whereas ketone groups formation also took place when aliphatic chain contains just one carbon atom. This method comprises the formation of extremely reactive nitronium ions (NO+2) generated during the autoprotolysis reaction of HNO3, which resemble the self-ionization of water (Figure 9B and 9c). In these reactions, NO+2 present in lesser amount, since it is required to progress their formation in the presence of catalyst (Chingombe et al., 2005; Giraldo et al., 2020). Consequently, some researchers, have mixed sulfuric acid solutions with nitric acid in different ratios to introduce more nitrogen for the purpose of achieving better efficiency of nitration (Tanada et al., 1999; Abe et al., 2000; Yantasee et al., 2004). Better aromatic nitration is considered as the convenient environment for larger quantity of nitrogen grafting into the ACs matrix. Ternero-Hidalgo et al. (2016) proved that greater amount of nitrogen functional groups can be grafted onto the ACs surface if HNO3 be used as a nitrogen enrichment agent, with special conditions, etched by H3PO4. They claimed that the existence of “phosphorus species” played the vital role to bond N-species onto ACs surface. However, phosphorus species may exist in two forms in the ACs matrix, where in “P-containing carbons” the functional groups persist as nitro groups, while in “P-free carbons” N species remain in the configuration of lower oxidation states (Ternero-Hidalgo et al., 2016; Cordero-Lanzac et al., 2018). Beside some improvements in terms of nitrogen enrichment in the ACs structures by using HNO3, there are some drawbacks in using this technique. Improved ‘specific surface area’ is a vital material property for NEACs which can be severely affected by the oxidation with HNO3 (Arango et al., 2018; Baby et al., 2021).
Role of surfactants in nitrogen addition onto the ACs
Because of their aggregation behaviour near the interface, it has been reported that surfactants may induce different characteristics (better wettability, lower surface tension, etc.) on the AC surface. For AC functionalization, control properties, or regeneration, both anionic and cationic surfactants have been used (Ahn, et al., 2009; Choi et al., 2009; Wang et al., 2022). Surfactants are organic molecules that possess amphiphilic characteristics with both nonpolar region (hydrophobic tail) and polar region (hydrophilic head). Beside their surface activity, surfactants can self-aggregates in aqueous system to form micelles/vesicles and liquid crystals. Since AC is regarded to be highly hydrophobic, the surfactant molecules’ hydrophobic portions, under the control of enthalpic and entropic favourability, are pushed to get associated with AC surface. For associating with water molecules, hydrophilic head group is regarded to be favourable, while hydrophobic tail is regarded to be thermodynamically unfavourable and try to avoid it via surface adsorption or aggregation. Indeed, for quaternary ammonium-based surfactant (contains nitrogen in the head groups), a high adsorption capacity of anion pollutants (e.g., anionic Cr(VI) or the bromate ions) is expected due to the presence of the quaternary ammonium positive charge at the interface providing that the surfactant enhances the dispersibility of the ACs but not affecting much their porosity (Choi et al., 2009). Not only that, but quaternary ammonium surfactant was also found to reduce the pH sensitivity of the adsorption of some anionic onto the surface of AC (Chen et al., 2012; Farooq et al., 2012).
Not only in the pure form, the effect of quaternary surfactants, in the presence of non-ionic surfactants, on the ACs characteristics were also tested and found to be synergetic. For example, it was found that the use of a mixture of the cationic quaternary ammonium and the non-ionic Tween 80 surfactants enhanced the surface activity (higher specific capacitance) (Ntakirutimana et al., 2019).
Beside using nitrogen-containing surfactants to enhance the ACs characteristics, some researcher used it as precursor that undergo inert thermal treatment in the presence of AC precursor aiming to increase the N contents and introducing N-functionality though, the obtained N-yield is not promising at all. For example, Emamy et al. (2021) used the double-tailed dimethyldidodecylammonium bromide (DDAB) as N-promotor for ACs fabrication from chitosan. The obtained specific surface area were 306 m2/g and the nitrogen content 5.1% (N% content even less than that obtained from pure chitosan in the absence of DDAB). It is worth mentioning here that due to is super hydrophobicity, DDAB has very limited monomer solubility in water (it tends to aggregate into vesicles at very low concentration) and this might be one of the reasons behind such poor effect.
Reaction pathways of biomass N-Enriched ACs
The possible reaction pathways of biomass N-enriched ACs could be summarised as follow: when thermal treatment is ongoing, depolymerization of hemicellulose and cellulose into hydroxyl-acetone, furans, acetic acid, levoglucose, furfurals and other O-containing species occurs via ring-open, cyclization reactions and dehydration, along with release of CO, H2O, H2, CH4 and CO2. Lignin generally decomposes and forms 2-methoxy phenol, 2-methoxy-4-vinyl phenol, 4-vinyl phenol, 6-demethoxy-4-propenyl phenol and 2, 6-demethoxyphenol, 2 via ß–O-4 linkage breaking reactions, as well as free radicals combining reactions, by releasing H2, H2O and CH4. Meanwhile, high quantities of O-containing functional groups (−C=O, −COO, −OH and C−O−C) are present in the solid char product. When biomass N-enrichment occurred thermally through NH3 atmosphere, it reacted with the biomass to produce •NH2 and •NH, which then reacted with O-containing compounds to form N-containing compounds (like amide) through Maillard reactions. Certain amines can be transformed into N-heterocyclics (pyrroles, pyridines) through condensation, cyclization and dehydration reactions. Certain O-heterocyclics (furfurals and furans) also transformed to N-heterocyclics (that is, pyrroles) through substitution reactions. Phenols (-OH groups) might directly bond with NH3, •NH2, and •NH to create anilines through dehydration. These reactions are usually accompanied with discharging high amounts of CO2 and H2O. Moreover, NH3 cracking produced high amounts of •H, which might promote the hydrogenation and hydrodeoxygenation reactions, and form additional small molecular phenols (like phenol, p-cresol, 2-methyl phenol, 4-vinyl phenol, 4-ethyl phenol), by moving methoxy side chains and addition reactions of bigger molecular phenols, which results into release of N2, H2, and H2O. Furthermore, O-containing groups in consumed biomasses may undergoes reaction with NH3, •NH2, and •NH, and created large amounts of N-containing groups (pyridinic-N, pyridone- N-oxide, pyrrolic-N, and quaternary-N) through Maillard reactions. First, O-containing groups get into reaction with–NH2 to produce pyrrolic-N and pyridinic-N, and then some pyridinic-N are converted into quaternary-N through polymerization.
Material characters changes due to the presence of nitrogen
The use of carbon-based adsorbents relies on their physiochemical properties, such as their surface area per Gram of the adsorbent, shape/extent of their porosity, nature, extent, location of their functional groups, and their morphologies. Thus, a thorough surface physiochemical review of ACs must involve the characterisations of the physical constitution. The following paragraphs will highlight the materials’ properties changes when N-enrichment technique applied on plant-biomass derived ACs.
The process of thermal carbonization produces a consistent structure, with a simple and semi-developed pore structure (Osman et al., 2016), which must be widened and extended properly by chemical or physical activation. Baur et al. (2018) functionalized the pores of activated carbon fibre with amine (diethylenetriamine, DETA) via wet impregnation. After drying it was found that although the SBET decreases as a result of the reduction in porosity, the pore width was constant. The performance of the diethylenetriamine loading into the ACs was excellent and enhanced the removal of formaldehyde as much 100-fold if compared with the unloaded ACs.
So, the N-enrichment of ACs not only would affect its surface chemical property, but also its porous nature, in a form and to an extent that depended on the starting AC, the used N-reagent, and the employed experimental technique. Development of the surface area and microporosity inside the carbon structure has often been reported when plant biomasses thermally treated with NH3(g) (Stöhr et al., 1991). For example, the increase in surface area distributed in pores larger than 1 nm (determined by DFT model assuming slit-shape pores geometry) was as high as 151% for a wood-derived commercial AC that was subjected to high temperature NH3(g) treatment after oxidization, compared to the oxidized only predecessor (Dastgheib et al., 2004). There have been some reports of the porous structure remaining unchanged (Jansen and van Bekkum, 1994; Abe et al., 2000) or of a decrease in the microporosity and surface area (Xie, F. et al., 2000; Przepiórski, 2006) but such reduction might not be due to only the amination since the process accompanied with high temperature thermal treatment.
In case of NH3(g), the structure of pore remained virtually unaffected (Jansen and van Bekkum, 1994), and the surface region became greatly developed (He et al., 2010) or reduced/improved depending on the employed ammoxidation stages (Nowicki et al., 2009). With nitrogenating compounds other than NH3(g) (like amides and amines), reductions in the surface area and volume of micropores were also observed but such reductions might be also due to the employed thermal treatment (Seredych et al., 2008). Numbers of plants that have been taken under consideration by the researchers for producing NEACs, by applying wide varieties of nitrogen enrichment agents at different thermal conditions, are summarized in Table 1. Beside selecting nitrogen enrichment agents, the carbonization temperature, reaction retention time and employed experimental procedure also played a vital role to avail anticipated surface area. Researchers conducted their experiments at temperature ranged from 450°C to 1,110°C and achieved the SBET as small as 159.2 m2/g and as high as 2,995 m2/g as per Table 1.
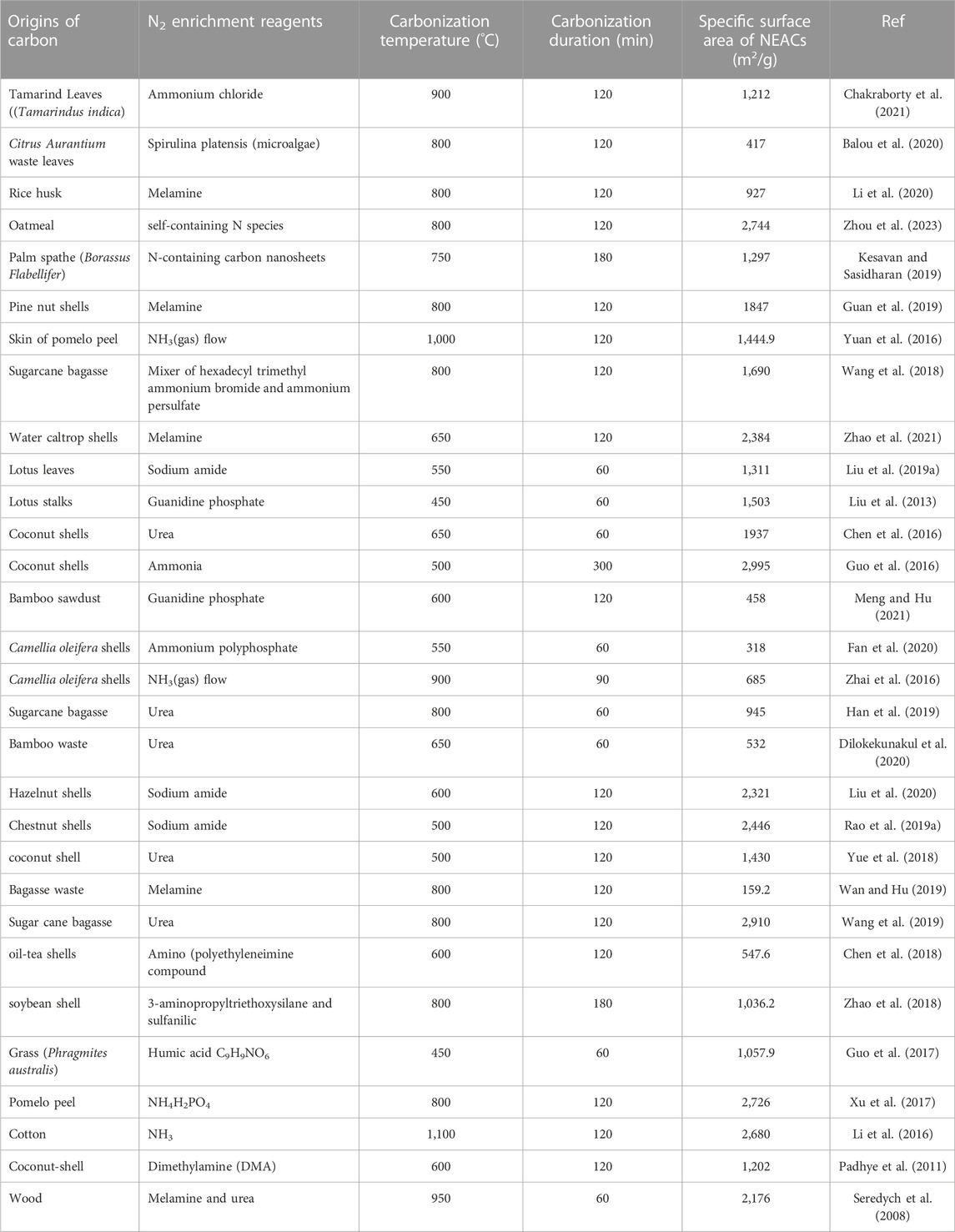
TABLE 1. Carbon source, nitrogen enrichments agents, thermal conditions, carbonization duration and achieved surface area of plant-derived NEACs.
Because the chemical functional groups formed on the surface of the NEACs plays a very significant role in the liquid phase adsorption, the intensity and nature of such functionalities are the primary factor to categorise the NEACs as prospective adsorbents. The FTIR technique is regarded as one of the most effective qualitative bulk characterisation methods for identifying the inorganic or organic functional groups developed on the ACs. Hence it has been used to identify the variety of functional groups onto the NEACs (Rehman et al., 2019).
A comparison study is always regarded as an optimum approach when two different types of materials properties needed to be differentiated. In this regard, Dilokekunakul et al. (2020) prepared nitrogen-enriched AC from bamboo waste and compare its performance toward CO2 adsorption with that of a pristine ACs. The unmodified carbonaceous materials (AC) were carbonized at 600°C and activated with CO2 gas at 900°C while the modified ACs were further treated with KOH and urea (N-reagent) with thermal treatment in air at 650°C (AC-UK). The oxidation treatment was also carried out without KOH and urea (AC-Air). The findings associated with this nitrogen enriched plant-derived ACs and none-NEAC, are displayed in Figure 10. A type I isotherm along with an H4 hysteresis loop was found to be demonstrated by all samples (NEACs and non-nitrogen enriched, see Figure 10A) which signify that microporous volume of all samples is greater than mesoporous volume. As observed in Figure 10B, pore size distributions attained via N2 sorptiometry indicate that there is a distinct fluctuation in the micropore volume of all the prepared samples. For NEAC (AC-UK), the surface area was smaller than the other two samples and the incremental micropore volume was seen average up to 1.47 nm (smaller than that of the AC and AC-Air). This means that the drop in surface area for AC-UK is due to less accumulative pore volume. For the FTIR spectra shown in Figure 10C, at 1,036 and 472 cm−1 bands, the FTIR peaks signify C–O stretching, while C–C stretching vibrations could be observed in every kind of ACs. Additional peaks at 3,467 and 1,638 cm−1 are demonstrated for the AC-UK, which could be due to N–H/O–H stretching as well as C=C stretching, respectively, which were minimal in AC and AC-Air. In spite of the lower SBET, at low pressure (<10 kPa), the CO2 adsorption ability at 0°C and 25°C were found to be the best for the AC enriched with nitrogen, i.e., sample AC-UK). When increasing the pressure above 20 kPa, it was found that the CO2 adsorption for AC-UK at 0°C is the lowest. This behaviour was referred, by the authors, to combined factors, namely, the nature and extent of N-functionalities, as depicted in Figure 10D, and pore size distribution (see Figure 10C). This chemical phenomena could lead to development of enhanced porous structure into the AC skeleton to improve adsorption (Dilokekunakul et al., 2020).
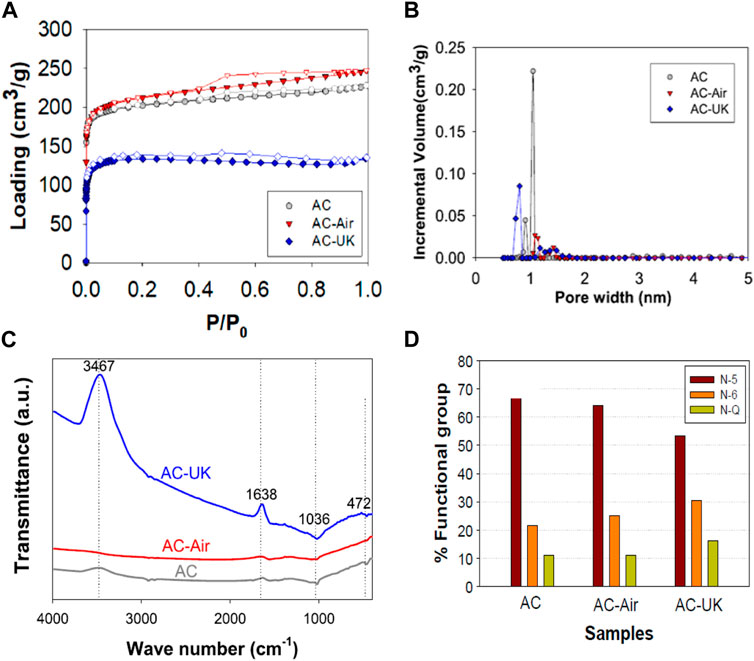
FIGURE 10. Comparative study on the indicated N-ACs samples using nitrogen sorptiometry (A, B), FT-IR (C), and XPS percentage composition of N1s signals (D). With the permission from Elsevier (Dilokekunakul et al., 2020).
X-ray photoelectron spectroscopy (XPS), also referred to as electron spectroscopy for chemical analysis (ESCA), is broadly employed to study carbon-based materials’ surface structures. It allows analysing the first 10 or 20 atomic layers and provides information pertaining to both elemental concentration as well as composition. Due to the sensitivity of the technique to the atom environment, XPS can be used to study the identity of functional groups presents on the surface. XPS spectra are extensively employed to study the NEACs’ surface structures as these offers both qualitative information regarding the types of surface ions/atoms presents in the materials as well as the relative surface amount (in atomic%) pertaining to each type. With regards to N-enriched AC samples, one interest would be the nitrogen core-electron (Nls) binding energy region (393–405 eV). The Nls spectrum can be defined as a composite curve that can be deconvoluted to several individual curves that signify the relative amounts and types of the functional states wherein the carbon exists. As described earlier, the treating the ACs and/or their precursors with N-reagents may considerably increase the content of nitrogen in the biomass-derived ACs. At the same time such treatment may reduce the oxygen content on the surface because of the preferential reaction between oxygen-containing functional groups and N-compounds (Hulicova-Jurcakova et al., 2009a). Figures 11A, B and display a typical XPS spectrum pertaining to the nitrogen-doped ACs. Deconvolution of the N 1s spectra can be result in four main peaks: pyridinic-N (N-6, 398.7 eV), pyrrolic/pyridine-N (N-5, 400.3 eV), quaternary (graphitic)-N (N-Q, 401.8 eV), and pyridine-N-oxide (N-X, 403.0 eV) (Sun et al., 2014). Some researchers assign the N 1s peak around 400 eV for pyrrolic/pyridone-N (Xu, B. et al., 2012; Hulicova-Jurcakova et al., 2009a; Hulicova-Jurcakova et al., 2009b). Overall, pyridinic-N and pyrrolic/pyridone-N are regarded to be more dominant, which is advantageous since these two functional groups help in conferring pseudo-capacitive behaviour pertaining to adsorbent (Xu, B. et al., 2012; Hulicova-Jurcakova et al., 2009a; Hulicova-Jurcakova et al., 2009b). These functional groups could be attributed to reaction of the N-agents and their decompositions (e.g., NH3, cyanic acid, etc.) with carboxyls and hydroxyls, while quaternary-N could result due to transformation of pyridinic-N into a graphitic substrate (Zhao et al., 2015). Interestingly, researchers identified that adding pore forming agents could surge the presence of certain N-functional groups such as pyridone-N-oxide and pyrrolic/pyridone-N, while reducing the quaternary-N content, and had no evident influence on the pyridinic-N amount (Chen et al., 2016).
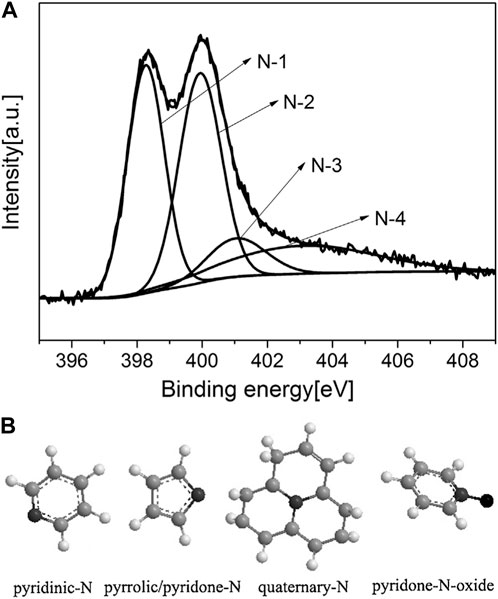
FIGURE 11. Typical XPS spectra of NEACs and chemical structure of four nitrogen-containing functional groups (A) depicts the XPS spectra; (B) for molecular form of groups. With the permission from Elsevier (Chen et al., 2016).
Pollutant’s adsorption mechanism and removal performance
In aqueous phase adsorption of organic molecules, surface chemistry and pore structure play significant roles and need to be investigated thoroughly (Moreno-Castilla, 2004). As mentioned earlier, recent research trends have demonstrated the tendency to use NEACs to eliminate wide-ranged pollutants from wastewater because of its greater adsorption capacity (Rao et al., 2008). Adsorption pertaining to an adsorbate by adsorbents can be defined in two aspects: adsorption capacity and affinity. Adsorption capacity may be limited by the available intermolecular space belong to a sorbent available for adsorption for a given adsorbate, while adsorption affinity relies on the level of strength possessed by attractive forces that exist between adsorbent and adsorbate. Several studies suggest that the mechanism of adsorption from solution can be determined not only by the physical characteristics associated with ACs structure (pore size distribution, specific surface area, microchannels, etc.), but also carbons’ surface and intra-porous chemical characteristics (Wright, 1967; Dil et al., 2017; Mazaheri et al., 2017; Rehman et al., 2019).
Adsorption by NEACs can 1) impact the mobility as well as fate of both general organic matters and xenobiotic pollutants (e.g., amino acids, humic acid and proteins, dyes, heavy metals, hydrophobic); 2) modify the surface properties relating to adsorbents via surface chemistry alteration by various treatments to eliminate or incorporate functional groups; and 3) be crucial for potential adsorbent applications pertaining to water treatment technologies (Yue et al., 2009; Rivera-Utrilla et al., 2011). It is imperative to mention that a key role is played by the types of the chemical functional groups with regards to the adsorption of targeted pollutants. Acid functional groups improve the removal process for cationic species, while basic functional groups help sequester anionic species from aqueous solutions (Rivera-Utrilla et al., 2011). As an example, in this review we discuss the adsorption of the hexavalent chromium ions, in order to explain the adsorption mechanism. Figure 12 presents a schematic pertaining to Cr(VI) adsorption onto the NEAC.
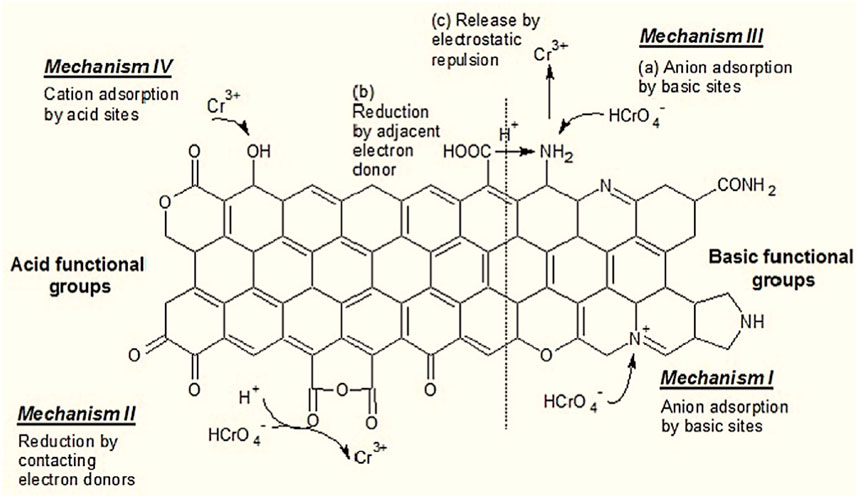
FIGURE 12. Adsorption mechanisms of hexavalent chromium on the activated carbon surface. Reproduced and adopted from Elsevier after permission (Valentín-Reyes et al., 2019).
The reaction can occur via four different mechanisms based on the interaction between various functional groups of AC (Figure 12) and the chromium species (hexavalent and trivalent Cr) during the adsorption process. The first mechanism (I) is associated with the anionic adsorption of Cr(VI) on to basic functional groups. The second mechanism (II) refers to the reduction of Cr(VI) to Cr(III) due to contact with certain functional groups that behave as electron donors. This second mechanism is related to these adsorbents that possess more acid functional groups when compared with basic sites, for example, the oxidized ACs. A three steps surface interaction is established by the third mechanism (III): (a) through anion adsorption, Cr(VI) is adsorbed on basic sites; however, (b) via an adjacent electron donor, it could be reduced to Cr(III) and then (c) via electrostatic repulsion, Cr(III) is released to the solution. Lastly, the fourth mechanism (IV) includes adsorption of Cr(III) (cation exchange) on acid sites, which is a coupled reduction-adsorption mechanism; thus, the fourth mechanism follows the mechanisms II and III. This adsorption mechanism is regarded to be optimum for adsorbents that have more acid sites available during adsorption processes (e.g., oxidized ACs); nevertheless, most adsorbents include acid functional groups that enable the chances of a combined reduction-adsorption interaction. In this case, the occurrence of adsorption mechanisms could be because of: (a) polar adsorption pertaining to the adsorbate via the adsorbent’s (hydrophilic shell) functional groups, (b) adsorbate surface accumulation, (c) initially with functional groups versus surface/polar charges or (d) π−π attraction occurring between the layers of the adsorbent (hydrophilic core) and the cyclic adsorbate. Essentially, the surface of NEACs provide adsorption spots enriched with high density of N-functional groups, thus multilayer of molecules of NEACs, comprised of piled chemilayers, have a much higher likelihood of entering aquatic surroundings because of the difficult and complex process of separating single-layer. It has been reported that N-functional groups perform adsorption via hydrogen bonds, π−π interactions and Lewis acid−base (Lian et al., 2016; Niu et al., 2022) which may enhance the adsorption of organic contaminants to be higher than that of the non-modified ACs.
Because of the nature of mixed polarized organic contaminant molecules in water, scholars considered NEACs for improved adsorption to eliminate organic toxins released from hospitals wastewater such as the toxins reported in (Emmanuel et al., 2005; Kist et al., 2013). Reviewing the literature revealed that there was a scarcity of research papers that explained the adsorption characteristics and reaction process during elimination of mixed polarized organic pollutant molecules from wastewater (Lloyd et al., 2015). Some scholars just put their attention on adsorption kinetics to eliminate pollutants from wastewater by the use of NEACs. For instance, Tanada et al. (1999) altered commercial ACs with amino groups for effective removal of formaldehydes from wastewater produced in a hospital. They considered that the adsorption follows monolayer adsorption. Lloyd et al. (2015) tried acid-amine modification of sawdust-based mesoporous AC to eliminate mixed polarized glutaraldehyde (GA) molecules from wastewater of the hospital and found strong enhancement of the adsorption due to the acid-amine modification. During the solution stage, GA monomers of hydroxyl functionality are predominating. Thus, for pH < 7 (NEACs carries net positive charge), adsorption interactions could happen through complexation that involve covalent carboxyl-hydroxyl bonding condensation as per Figure 13A. In case of basic condition (i.e., pH > 7), the surface of NEAC assumed net negative charge because of deprotonation of surface acidic and basic groups. During the solution stage, GA polymers have mixed hydroxyl, aldehyde, and carboxyl functionalities. Thus, in basic condition, it may be conjectured that particular adsorption interactions could happen through covalent bonding reactions like carboxyl-hydroxyl shown in Figure 13B; carboxyl-aldehyde shown in Figure 13C; hydroxyl-aldehyde shown in Figure 13D; and amine-aldehyde (Schiff bases formation) shown in Figure 13E. Furthermore, physical electrostatic pull could take place through amine-hydroxyl as shown in Figure 13F and amine-carboxyl (shown in Figure 13G). Therefore, the high GA adsorption on NEAC, in comparison with non-nitrogen modified AC, could be attributed to the anchored amine groups as well as the high acidic surface groups on the non-nitrogen modified AC.
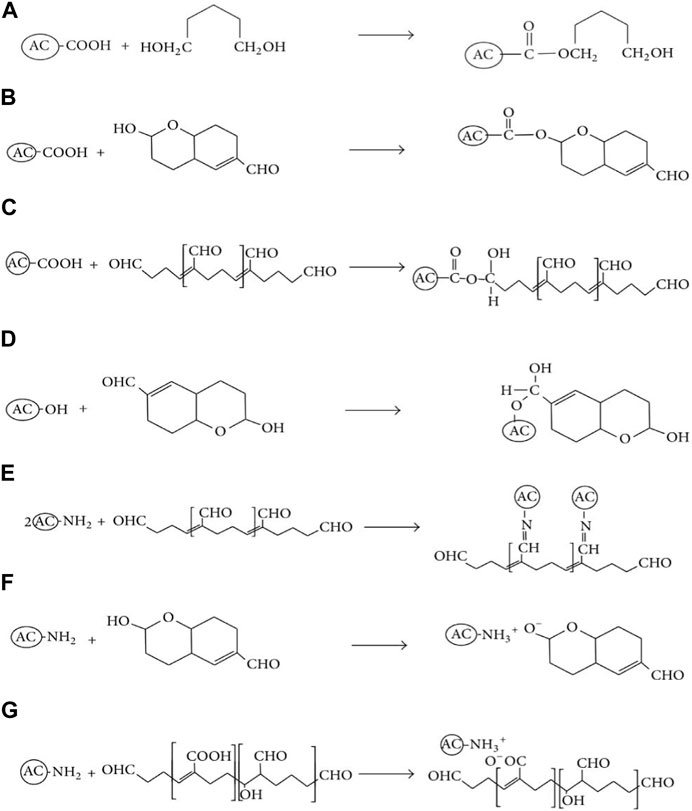
FIGURE 13. Reaction mechanism of mixed polarized GA monomeric and polymeric pollutant removal from wastewater. (A): carboxyl-hydroxyl condensation; (B): carboxyl-hydroxyl covalent bonding; (C): carboxyl-aldehyde covalent bonding; (D): hydroxy-aldehyde covalent bonding; (E): amine-aldehyde covalent bonding; (F): amine-hydroxyl electrostatic attraction; and (G): amine-carboxyl electrostattic attraction. Reproduced and adopted from Hindawi after permission (Lloyd et al., 2015).
When the aldehyde is of small size, like acetaldehyde, it was found that its adsorption on the polar heterogeneous surface of NEAC, controlled largely by the size and extent of small micropores with some contribution of the larger pores (El-Sayed and Bandosz, 2002).
Notwithstanding a wide difference in polarity, charge, size, among other relevant characteristics affecting the process of adsorption, for anions contaminants, the adsorption usually improved on NEACs compared to virgin ACs (recent published example is shown in Figure 14). Detailed explanation can be seen in the referred article.
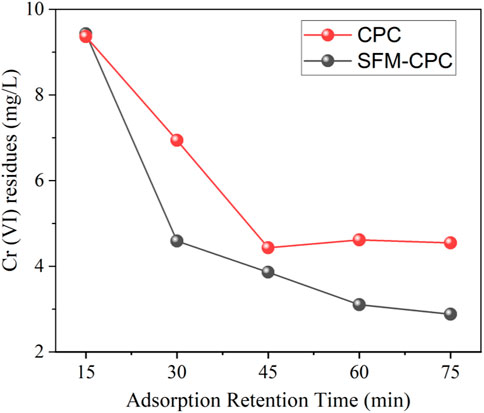
FIGURE 14. Adsorption performance comparison of plant derived AC and the nitrogen-enriched NEAC sample. Initial concentration was 30 mg/L and the pH = 4 (The maximum relative standard deviation is ±0.7% of the reported value. Adopted from Elsevier with permission (Bumajdad and Khan, 2021).
In concise, the effectiveness to eliminate pollutant substances from waters by process of adsorption was related to: the carbon surface’s acid–base character (Xie, R. et al., 2017), and also with the permeable carbon structure, i.e., its surface area (Dastgheib et al., 2004), volume of micropore (Przepiórski, 2006; Wang, J. et al., 2021), and pore size distribution (Chingombe et al., 2006). Due to such complexity, after amination of ACs, adsorption of pollutants might improve significantly, like, cyanide increased around five times (Monser and Adhoum, 2002) and Mercury adsorption enhanced more than two times (Zhu et al., 2009), but also it might decrease as well as in the case benzoic acid adsorption after AC amination (Abe et al., 2000). Table 2 summarise some of the practical applications of NRAC in removal of water contaminants.
COST-EFFECTIVE nitrogen-doped carbon materials
Cost is a vital aspect for technoeconomical evaluation of the sorbent materials. Plant biomasses have been on the top of the list as a ‘low-cost’ feedstock in the preparation of porous carbons (Babel et al., 2012; Kalyani and Anitha, 2013). Up to now, natural resources, for example, agricultural residues, plant biomasses, and other industrial bio-residues, have been considered as cheap precursors for ACs production and applied as wide-ranged industrial applications, especially, as adsorbents and electrodes manufacturing (Mohamed et al., 2010; Paul et al., 2019).
As per reaction engineering concept, the development of a cost-effective carbon material is not only depends on the source material but also on the design of process, where energy consumption and usages of number of chemical/s involved (Bumajdad and Khan, 2021; Zhang, Z. et al., 2019b). Hence, the development of renewable and green strategies may not only save energy resources for NEACs preparation, but also develop carbon structure with ample hetero atoms doping and improved microchannels in lower cost. Among the available doping strategies, the nitrogen doping is beneficial among other metallic and non-metallic doping as it can stimulate electronic transmission, possesses better stability and reasonable capacitances (Luo et al., 2022). Additionally, the developed porous network configuration and better surface area can provide lodgings more electrolytic ions onto the pore surface and channels of NEACs, and consequently elevate electrolyte diffusion and internal electron transfer during physiochemical reactions. Generated micropores in classified porous grid can intensely increase absorption/desorption efficiency, elude ion-sifting effects and hence increase the surface capacitance (Borghei et al., 2018). The immense necessity for research initiatives to propose a sustainable financial analysis in adsorbents production, regeneration and applications in water treatment was understood since 1990s (Bailey et al., 1999), but up to now, this is considered as one of the major technoeconomical gap in the literature (Ahmed et al., 2016). Just few articles (e.g., (Dickinson et al., 2015; Jirka and Tomlinson, 2015)) conducted cost analysis on biochar adsorbents production in certain scale; although the necessity of inclusive cost benefit analysis mentioned to be fully analyzed. An extensive variety of low-cost sorbents production techniques examined where numbers of reactants, precursors and reaction parameters had been justified and finally their effects on the adsorption performance mentioned. However, the information of the material-balance (raw materials chemicals), material costing and energy consumption not been mentioned until now, although it is highly important and indispensable for proposing any financial and cost-benefit analysis (Ahmed et al., 2016). Thus, analogies of the development of functionalized NEACs are challenging due to inconsistencies in data. Theoretical models based on financial parameters covering from raw materials collection costs to the achieving “break-even point (BEP)” for carbon based adsorbent production in larger scale has not also been carried out. Very few researchers have so far been able to accumulate published technical articles from literature containing the necessary information on the process cost analysis related to adsorbents modification and production methodologies. They have just considered the lab-scale researches, hence, neither mentioned any data on the production cost of closed-loop manufacturing of adsorbent nor predicted commercial scale costing (Bailey et al., 1999; Jirka and Tomlinson, 2013).
Drawbacks, possible scopes of research and recommendations
N-enriched activated carbons produced by thermochemical treatment of plant biomasses was reviewed in this work with focus on reaction mechanisms, the factors affecting reactions, improved materials’ characters, and pollutants removal efficiency, etc. Based on that, overall recommendations concerning the shortcomings on NEAC production and academic gaps are suggested.
i) Reaction Engineering
As specified previously, the nature of reactions existing between N-enriching substances and plant-based biomass is complex. The literature indicates that the present research trends seldom address real-time models for such reactions; moreover, there is no simulation-specific data regardless of its significance in regulating N-enrichment quantity and output quality. Computer simulations are critical for establishing the correlation between atomic-scale molecular characteristics and physically measurable characteristics; this is specifically pronounced typically to the surface science domain and, explicitly, carbon surfaces. N-substances and unmodified/modified biomass exhibit numerous organic reactions, of which several reactions are due to carbon-based surfaces. It is exceptionally difficult to assess the effects of particular functional groups using empirical techniques. Molecular simulation can address all such issues and can be used to assess adsorption data pertaining to extrinsic molecules having random functional groups and AC of different pore distributions at all pressure and temperature values. Researchers (An et al., 2019) formulated a slit-sheet AC framework to assess AC adsorption effectiveness using the solution of the kinetic expression using the Grand Canonical Monte Carlo (GCMC) simulation technique, whose schematics are depicted in Figure 15.
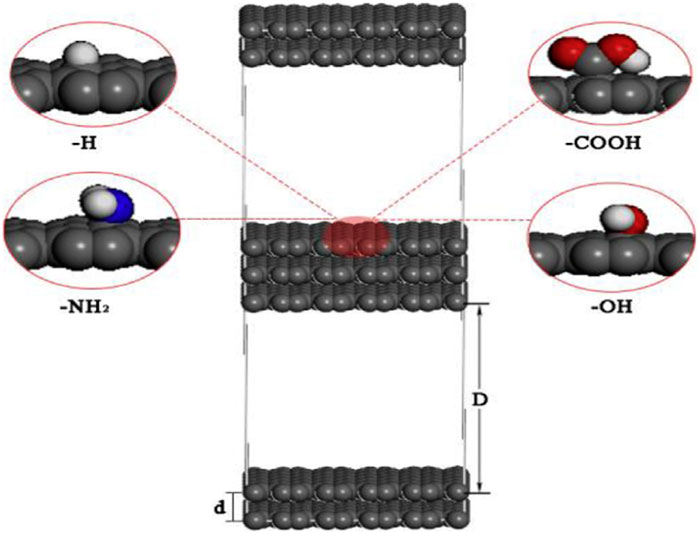
FIGURE 15. Molecular pore model of activated carbon after adsorption. Adopted from Elsevier with permission (An et al., 2019).
As indicated in Figure 15, they assessed four functional groups, namely, hydrogen (−H), amine (−NH2), hydroxyl (−OH), and carboxyl (−COOH), for evaluating the influence of functional groups on adsorption characteristics of the substance. Functional groups were contrasted by using predetermined densities. It should be noted that the simulation computed molecular structure created post adsorption; it could also establish that nitrogen functional groups were present.
Recommendation
To develop a precise modeling platform several factors, like-N-compound break down rate, diffusion, absorption and adsorption kinetics of N-molecule onto the carbon skeleton, necessary equation arrangement and solver section are very imperative.
ii) Carbonization Time
Carbonization of plant-based biomasses is reported to require between one to 5 hours of high-temperature heat treatment (Table 1). The extended carbonization process is more energy-intensive and the technique for AC production using plant-based biomass may be questionable. The challenge lies in formulating a substance that addresses an environmental concern, but it leads to another problem, which, in this case, the use of large amount of energy in the preparation procedure. Furthermore, because quiescent reactors are the presently-used AC production systems, reducing the temperature to room temperature level after the carbonization required an extended period of 6 h (Rivera-Utrilla et al., 2011). Fluidized bed reactors are optimal for such situations because fluidization has been established to enhance gas-solid contact surface; biomass molecules can interact better and accelerate the reaction, thereby providing benefits for continuous production and optimal heat transfer. Although plant biomasses pyrolysis has been carried out in diverse kinds of fluidized beds, such as bubbled fluidized bed (Heidari et al., 2014; Sellin et al., 2016), spouted bed (Amutio et al., 2011) and circulating fluidized bed (Duanguppama et al., 2016), this need to be the role rather than the exception.
Recommendation
The literature indicates that nitrogen enrichment and carbonization are concurrent processes that comprise multiphasic reactions (gas-solid-liquid). Such reactions require consistent mass and heat transfer requirements; hence, in order to ensure high output quality, there is a need for precise control. Fluidised Bed Reactors (FBRs) have been studied by researchers for the last 20 years to perform multiphasic reactions (Khan et al., 2014; Khan et al., 2016a; Khan et al., 2016b). It is established in the existing literature that an extended activation period was used for preparing ACs. Hence, there is a likelihood that apparatus effectiveness may be affected, and process application limited (Diebold and Bridgwater, 1997). This material engineering issue can be addressed by facilitating carbonization within fluidized bed reactors. Hence, there are several possibilities for using fluidization for enhanced N-enrichment and carbonization of plant biomass. Figure 16 depicts the suggested changes to the carbonisation framework that allows a 24-time reduction in carbonization time.
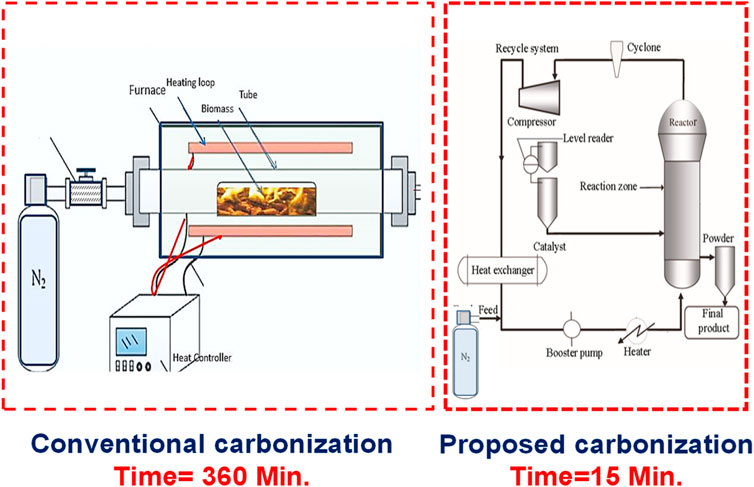
FIGURE 16. Conventional and Recommend engineering system for rapid carbonization of biomass derived ACs. Adopted from Elsevier with permission (Khan et al., 2014; Khan et al., 2016b; Gao et al., 2018).
iii) Scale-up and Real Wastewater Treatment
There has been a lack of research concerning pilot-scale NEAC production to date. Up to now, limited studies have addressed scaling-up the absorption process by NEACs, which is critical for estimating and confirming the required significant process factors and commercial use variables. One pilot-scale research study has been published concerning the efficacy of the formulated AC in eliminating wastewater micropollutants (Mailler et al., 2016). There is a lack of research concerning the use of NEACs for real wastewater processing as well. Most of the experiments that have been conducted to date have used lab-produced wastewater.
Recommendation
Hence, there is an immense research need to enhance NEACs production, design large-scale systems, and use them for practical wastewater management.
Conclusion
The present work is comprehensive review of reaction dynamics concerning nitrogen enrichment of plant-based biomass used for producing nitrogen-enriched activated carbon (NEAC). It has been observed that several amides and amines, were utilized so far for producing plant-biomass based NEACs. The amide family is the single most efficacious nitrogen-based substances offering significant N-functional groups for the carbon substrate. Amines and N-based surfactants are not as efficacious. It was indicated that imides, amides, amines, and nitriles functionalities are dominant at low temperatures (<600°C). Concurrently, thermally strong aromatic rings like pyridine or pyrrole imitating functions were more prevalent at higher temperatures (>600°C). The cause of thermal strength of amide functional groups and manageable changes from higher to lighter molecular weights, N-based groups provided distinct molecular characteristics corresponding to NEAC surfaces. Root, stem, bark, and leaves from live and dumped plants were used for AC and NEAC production. Among the plant parts used for biomass creation, leaves were found to have relatively more nitrogen content after carbonization; it may be attributed to the chlorophyll content present inside leaves. The significant changes pertaining to material characteristics concerning functional groups and the increase in nitrogen presence have been presented and contrasted using material characteristics-based outcomes. NEACs exhibited enhanced presence of C-N, N-H, N-O, N-CHn, while a majority of the ACs prepared without nitrogen-enhancing agents indicated the presence of only C-H, C-O and C-C bonds. NEAC wastewater pollutant elimination efficacy has been contrasted and summarized. Outcomes from the comparative assessment indicated that the use of NEACs allow more efficient wastewater pollution elimination if compared to pure ACs. A major research gap of coherent information of cost benefit studies and cost divergences analysis have been observed. Extensive research on the financial forecasting on the cost-effective chemical process development is severely necessary for clear understanding of economically viable adsorption processes and to explain the technology. Decision-makers and scholars pursuing additional research in this field are expected to benefit from the recommendations and indicated gaps.
Author contributions
AB was the principal investigator of the research project, he proposed the idea, supervised the experimental work, analyzed the results, and helped in writing the review manuscript. MK was the main researcher on this project, and he drafted the review. JL was the co-investigator of the research project where he helped in drafting the proposal, supervised some of the experimental work, and he revised this review. All authors contributed to the article and approved the submitted version.
Funding
This review is an outcome of the project PN1724SC03 which was funded by the Kuwait Foundation for the Advancement of Sciences, KFAS.
Acknowledgments
The authors also acknowledge the support of the Research Administration of Kuwait University (Grant Numbers GS 01/01, GS 01/03, GS 01/05, GE01/07, GS 03/01, GS02/01 and GS 02/08).
Conflict of interest
The authors declare that the research was conducted in the absence of any commercial or financial relationships that could be construed as a potential conflict of interest.
Publisher’s note
All claims expressed in this article are solely those of the authors and do not necessarily represent those of their affiliated organizations, or those of the publisher, the editors and the reviewers. Any product that may be evaluated in this article, or claim that may be made by its manufacturer, is not guaranteed or endorsed by the publisher.
References
Abe, M., Kawashima, K., Kozawa, K., Sakai, H., and Kaneko, K. (2000). Amination of activated carbon and adsorption characteristics of its aminated surface. Langmuir 16, 5059–5063. doi:10.1021/la990976t
Adib, F., Bagreev, A., and Bandosz, T. J. (2000). Adsorption/oxidation of hydrogen sulfide on nitrogen-containing activated carbons. Langmuir 16, 1980–1986. doi:10.1021/la990926o
Ahmed, I., Panja, T., Khan, N. A., Sarker, M., Yu, J.-S., and Jhung, S. H. (2017). Nitrogen-doped porous carbons from ionic liquids@MOF: Remarkable adsorbents for both aqueous and nonaqueous media. ACS Appl. Mater. Interfaces 9, 10276–10285. doi:10.1021/acsami.7b00859
Ahmed, M. B., Zhou, J. L., Ngo, H. H., and Guo, W. (2016). Insight into biochar properties and its cost analysis. Biomass Bioenergy 84, 76–86. doi:10.1016/j.biombioe.2015.11.002
Ahn, C. K., Park, D., Woo, S. H., and Park, J. M. (2009). Removal of cationic heavy metal from aqueous solution by activated carbon impregnated with anionic surfactants. J. Hazaradous Mater. 164, 1130–1136. doi:10.1016/j.jhazmat.2008.09.036
Alam, M. Z., Khan, M. J. H., Kabbashi, N. A., and Sayem, S. A. (2018). Development of an effective biosorbent by fungal immobilization technique for removal of dyes. Waste Biomass Valorization 9, 681–690. doi:10.1007/s12649-016-9821-9
Ali, S. N. F., El-Shafey, E. I., Al-Busafi, S., and Al-Lawati, H. A. J. (2019). Adsorption of chlorpheniramine and ibuprofen on surface functionalized activated carbons from deionized water and spiked hospital wastewater. J. Environ. Chem. Eng. 7, 102860. doi:10.1016/j.jece.2018.102860
Ali, U. F. M., Azmi, N. H., Isa, K. M., Aroua, M. K., Shien, T. R., and Khamidun, M. H. (2018). Optimization study on preparation of amine functionalized sea mango (cerbera odollam) activated carbon for Carbon Dioxide (CO2) adsorption. Combust. Sci. Technol. 190, 1259–1282. doi:10.1080/00102202.2018.1448393
Amutio, M., Lopez, G., Aguado, R., Artetxe, M., Bilbao, J., and Olazar, M. (2011). Effect of vacuum on lignocellulosic biomass flash pyrolysis in a conical spouted bed reactor. Energy and Fuels 25, 3950–3960. doi:10.1021/ef200712h
An, Y., Fu, Q., Zhang, D., Wang, Y., and Tang, Z. (2019). Performance evaluation of activated carbon with different pore sizes and functional groups for VOC adsorption by molecular simulation. Chemosphere 227, 9–16. doi:10.1016/j.chemosphere.2019.04.011
Arango, D. I., Zapata-Benabithe, Z., Arenas, E. C., and Perez-Osorno, J. C. (2018). Influence of surface modification with nitric acid on electrochemical performance of agroindustrial waste-based activated carbon. J. Mater. Sci. Mater. Electron. 29, 15557–15569. doi:10.1007/s10854-018-9132-y
Babel, K., Janasiak, D., and Jurewicz, K. (2012). Electrochemical hydrogen storage in activated carbons with different pore structures derived from certain lignocellulose materials. Carbon 50, 5017–5026. doi:10.1016/j.carbon.2012.06.030
Baby, R., Hussein, M. Z., Zainal, Z., and Abdullah, A. H. (2021). Functionalized activated carbon derived from palm kernel shells for the treatment of simulated heavy metal-contaminated water. Nanomaterials 11, 3133. doi:10.3390/nano11113133
Bailey, S. E., Olin, T. J., Bricka, R. M., and Adrian, D. D. (1999). A review of potentially low-cost sorbents for heavy metals. Water Res. 33, 2469–2479. doi:10.1016/s0043-1354(98)00475-8
Balou, S., Babak, S. E., and Priye, A. (2020). Synergistic effect of nitrogen doping and ultra-microporosity on the performance of biomass and microalgae-derived activated carbons for CO2 capture. ACS Appl. Mater. Interfaces 12, 42711–42722. doi:10.1021/acsami.0c10218
Baur, G., Spring, J., and Kiwi-Minsker, L. (2018). Amine functionalized activated carbon fibers as effective structured adsorbents for formaldehyde removal. Adsorption 24, 725–732. doi:10.1007/s10450-018-9974-x
Belhachemi, M., and Addoun, F. (2011). Effect of heat treatment on the surface properties of activated carbons. E-Journal Chem. 8, 992–999. doi:10.1155/2011/649254
Biniak, S., Szymański, G., Siedlewski, J., and Świątkowski, A. (1997). The characterization of activated carbons with oxygen and nitrogen surface groups. Carbon 35, 1799–1810. doi:10.1016/s0008-6223(97)00096-1
Boehm, H. (1994). Some aspects of the surface chemistry of carbon blacks and other carbons. Carbon 32, 759–769. doi:10.1016/0008-6223(94)90031-0
Boeykens, S. P., Redondo, N., Obeso, R. A., Caracciolo, N., and Vázquez, C. (2019). Chromium and Lead adsorption by avocado seed biomass study through the use of Total Reflection X-Ray Fluorescence analysis. Appl. Radiat. Isotopes 153, 108809. doi:10.1016/j.apradiso.2019.108809
Borghei, M., Lehtonen, J., Liu, L., and Rojas, O. J. (2018). Advanced biomass-derived electrocatalysts for the oxygen reduction reaction. Adv. Mater. 30, 1703691. doi:10.1002/adma.201703691
Builes, S., and Vega, L. F. (2013). Effect of immobilized amines on the sorption properties of solid materials: Impregnation versus grafting. Langmuir 29, 199–206. doi:10.1021/la3038507
Bumajdad, A., and Hasila, P. (2023). Surface modification of date palm activated carbonaceous materials for heavy metal removal and CO2 adsorption. Arabian J. Chem. 16, 104403. doi:10.1016/j.arabjc.2022.104403
Bumajdad, A., and Khan, M. J. H. (2021). The reuse of disposable COVID-19 surgical masks as a nitrogen-enrichment agent and structure promotor for a wild plant-derived sorbent. J. Industrial Eng. Chem. 102, 163–176. doi:10.1016/j.jiec.2021.07.003
Chakraborty, R., Maji, P. K., Verma, C., Nayak, A. K., Singha, S. S., and Pradhan, M. (2021). Inherent oxygen-and nitrogen-doped porous carbon derived from biomass of tamarind leaf for high-performance supercapacitor application. Energy Technol. 9, 2000734. doi:10.1002/ente.202000734
Chen, J., Yang, J., Hu, G., Hu, X., Li, Z., Shen, S., et al. (2016). Enhanced CO2 capture capacity of nitrogen-doped biomass-derived porous carbons. ACS Sustain. Chem. Eng. 4, 1439–1445. doi:10.1021/acssuschemeng.5b01425
Chen, S., Bi, J., Zhao, Y., Yang, L., Zhang, C., Ma, Y., et al. (2012). Nitrogen-doped carbon nanocages as efficient metal-free electrocatalysts for oxygen reduction reaction. Adv. Mater. 24, 5593–5597. doi:10.1002/adma.201202424
Chen, S., Wang, J., Wu, Z., Deng, Q., Tu, W., Dai, G., et al. (2018). Enhanced Cr(VI) removal by polyethylenimine- and phosphorus-codoped hierarchical porous carbons. J. Colloid Interface Sci. 523, 110–120. doi:10.1016/j.jcis.2018.03.057
Chen, W.-F., Zhang, Z.-Y., Li, Q., and Wang, H.-Y. (2012). Adsorption of bromate and competition from oxyanions on cationic surfactant-modified granular activated carbon (GAC). Chem. Eng. J. 203, 319–325. doi:10.1016/j.cej.2012.07.047
Chen, W., Chen, Y., Yang, H., Li, K., Chen, X., and Chen, H. (2018). Investigation on biomass nitrogen-enriched pyrolysis: Influence of temperature. Bioresour. Technol. 249, 247–253. doi:10.1016/j.biortech.2017.10.022
Chen, W., Yang, H., Chen, Y., Chen, X., Fang, Y., and Chen, H. (2016). Biomass pyrolysis for nitrogen-containing liquid chemicals and nitrogen-doped carbon materials. J. Anal. Appl. Pyrolysis 120, 186–193. doi:10.1016/j.jaap.2016.05.004
Chingombe, P., Saha, B., and Wakeman, R. J. (2006). Sorption of atrazine on conventional and surface modified activated carbons. J. Colloid Interface Sci. 302, 408–416. doi:10.1016/j.jcis.2006.06.065
Chingombe, P., Saha, B., and Wakeman, R. J. (2005). Surface modification and characterisation of a coal-based activated carbon. Carbon 43, 3132–3143. doi:10.1016/j.carbon.2005.06.021
Choi, H.-D., Jung, W.-S., Cho, J.-M., Ryu, B.-G., Yang, J.-S., and Baek, K. (2009). Adsorption of Cr(VI) onto cationic surfactant-modified activated carbon. J. Hazard. Mater. 166, 642–646. doi:10.1016/j.jhazmat.2008.11.076
Cordero-Lanzac, T., Rosas, J. M., García-Mateos, F. J., Ternero-Hidalgo, J. J., Palomo, J., Rodríguez-Mirasol, J., et al. (2018). Role of different nitrogen functionalities on the electrochemical performance of activated carbons. Carbon 126, 65–76. doi:10.1016/j.carbon.2017.09.092
Dastgheib, S. A., Karanfil, T., and Cheng, W. (2004). Tailoring activated carbons for enhanced removal of natural organic matter from natural waters. Carbon 42, 547–557. doi:10.1016/j.carbon.2003.12.062
Daud, W. M. A. W., and Houshamnd, A. H. (2010). Textural characteristics, surface chemistry and oxidation of activated carbon. J. Nat. Gas Chem. 19, 267–279. doi:10.1016/s1003-9953(09)60066-9
de Andrade, J. R., Vieira, M. G. A., Da Silva, M. G. C., and Wang, S. (2020). Oxidative degradation of pharmaceutical losartan potassium with N-doped hierarchical porous carbon and peroxymonosulfate. Chem. Eng. J. 382, 122971. doi:10.1016/j.cej.2019.122971
Deliyanni, E., and Bandosz, T. J. (2011). Effect of carbon surface modification with dimethylamine on reactive adsorption of NOx. Langmuir 27, 1837–1843. doi:10.1021/la1042537
Deng, Y., Xie, Y., Zou, K., and Ji, X. (2016). Review on recent advances in nitrogen-doped carbons: Preparations and applications in supercapacitors. J. Mater. Chem. A 4, 1144–1173. doi:10.1039/c5ta08620e
Dickinson, D., Balduccio, L., Buysse, J., Ronsse, F., Van Huylenbroeck, G., and Prins, W. (2015). Cost-benefit analysis of using biochar to improve cereals agriculture. GCB Bioenergy 7, 850–864. doi:10.1111/gcbb.12180
Diebold, J. P., and Bridgwater, A. V. (1997). “Overview of fast pyrolysis of biomass for the production of liquid fuels,” in Developments in thermochemical biomass conversion Editors A. V. Bridgwater, and D. G. B. Boocock (Dordrecht: Springer Netherlands).
Dil, E. A., Ghaedi, M., Ghezelbash, G. R., Asfaram, A., and Purkait, M. K. (2017). Highly efficient simultaneous biosorption of Hg2+, Pb2+ and Cu2+ by Live yeast Yarrowia lipolytica 70562 following response surface methodology optimization: Kinetic and isotherm study. J. industrial Eng. Chem. 48, 162–172. doi:10.1016/j.jiec.2016.12.035
Dilokekunakul, W., Teerachawanwong, P., Klomkliang, N., Supasitmongkol, S., and Chaemchuen, S. (2020). Effects of nitrogen and oxygen functional groups and pore width of activated carbon on carbon dioxide capture: Temperature dependence. Chem. Eng. J. 389, 124413. doi:10.1016/j.cej.2020.124413
Duanguppama, K., Suwapaet, N., and Pattiya, A. (2016). Fast pyrolysis of contaminated sawdust in a circulating fluidised bed reactor. J. Anal. Appl. Pyrolysis 118, 63–74. doi:10.1016/j.jaap.2015.12.025
El-Sayed, Y., and Bandosz, T. J. (2002). Acetaldehyde adsorption on nitrogen-containing activated carbons. Langmuir 18, 3213–3218. doi:10.1021/la0116948
El-Sayed, Y., and Bandosz, T. J. (2003). Effect of increased basicity of activated carbon surface on valeric acid adsorption from aqueous solution activated carbon. Phys. Chem. Chem. Phys. 5, 4892–4898. doi:10.1039/b306983b
El-Sayed, Y., and Bandosz, T. J. (2005). Role of Surface Oxygen Groups in Incorporation of nitrogen to activated carbons via ethylmethylamine adsorption. Langmuir 21, 1282–1289. doi:10.1021/la0483966
El-Shafey, E. I., Ali, S. N. F., Al-Busafi, S., and Al-Lawati, H. A. J. (2016). Preparation and characterization of surface functionalized activated carbons from date palm leaflets and application for methylene blue removal. J. Environ. Chem. Eng. 4, 2713–2724. doi:10.1016/j.jece.2016.05.015
Emamy, F. H., Bumajdad, A., and Lukaszewicz, J. P. (2021). Adsorption of hexavalent chromium and divalent lead ions on the nitrogen-enriched chitosan-based activated carbon. Nanomaterials 11, 1907. doi:10.3390/nano11081907
Emmanuel, E., Hanna, K., Bazin, C., Keck, G., Clément, B., and Perrodin, Y. (2005). Fate of glutaraldehyde in hospital wastewater and combined effects of glutaraldehyde and surfactants on aquatic organisms. Environ. Int. 31, 399–406. doi:10.1016/j.envint.2004.08.011
Espinosa, J. C., Manickam-Periyaraman, P., Bernat-Quesada, F., Sivanesan, S., Álvaro, M., García, H., et al. (2019). Engineering of activated carbon surface to enhance the catalytic activity of supported cobalt oxide nanoparticles in peroxymonosulfate activation. Appl. Catal. B Environ. 249, 42–53. doi:10.1016/j.apcatb.2019.02.043
Fan, Y., Wang, H., Deng, L., Wang, Y., Kang, D., Li, C., et al. (2020). Enhanced adsorption of Pb(II) by nitrogen and phosphorus co-doped biochar derived from Camellia oleifera shells. Environ. Res. 191, 110030. doi:10.1016/j.envres.2020.110030
Farooq, W., Hong, H.-J., Kim, E. J., and Yang, J.-W. (2012). Removal of Bromate (BrO−3) from water using cationic surfactant-modified powdered activated carbon (SM-PAC). Sep. Sci. Technol. 47, 1906–1912. doi:10.1080/01496395.2012.664232
Gao, X., Wu, L., Li, Z., Xu, Q., Tian, W., and Wang, R. (2018). Preparation and characterization of high surface area activated carbon from pine wood sawdust by fast activation with H3PO4 in a spouted bed. J. Material Cycles Waste Manag. 20, 925–936. doi:10.1007/s10163-017-0653-x
Ghani, Z. A., Yusoff, M. S., Zaman, N. Q., Zamri, M. F. M. A., and Andas, J. (2017). Optimization of preparation conditions for activated carbon from banana pseudo-stem using response surface methodology on removal of color and COD from landfill leachate. Waste Manag. 62, 177–187. doi:10.1016/j.wasman.2017.02.026
Giraldo, L., Vargas, D. P., and Moreno-Piraján, J. C. (2020). Study of CO2 adsorption on chemically modified activated carbon with nitric acid and ammonium aqueous. Front. Chem. 8, 543452. doi:10.3389/fchem.2020.543452
Gregg, S. J. (1934). The adsorption of gases by solids. London, United Kingdom: Methuen and co. ltd.
Gu, D., Ma, R., Zhou, Y., Wang, F., Yan, K., Liu, Q., et al. (2017). Synthesis of nitrogen-doped porous carbon spheres with improved porosity toward the electrocatalytic oxygen reduction. ACS Sustain. Chem. Eng. 5, 11105–11116. doi:10.1021/acssuschemeng.7b03046
Guan, L., Pan, L., Peng, T., Gao, C., Zhao, W., Yang, Z., et al. (2019). Synthesis of biomass-derived nitrogen-doped porous carbon nanosheets for high-performance supercapacitors. ACS Sustain. Chem. Eng. 7, 8405–8412. doi:10.1021/acssuschemeng.9b00050
Guo, L., Yang, J., Hu, G., Hu, X., Wang, L., Dong, Y., et al. (2016). Role of hydrogen peroxide preoxidizing on CO2 Adsorption of nitrogen-doped carbons produced from coconut shell. ACS Sustain. Chem. Eng. 4, 2806–2813. doi:10.1021/acssuschemeng.6b00327
Guo, Z., Zhang, J., Kang, Y., and Liu, H. (2017). Rapid and efficient removal of Pb(II) from aqueous solutions using biomass-derived activated carbon with humic acid in-situ modification. Ecotoxicol. Environ. Saf. 145, 442–448. doi:10.1016/j.ecoenv.2017.07.061
Han, J., Zhang, L., Zhao, B., Qin, L., Wang, Y., and Xing, F. (2019). The N-doped activated carbon derived from sugarcane bagasse for CO2 adsorption. Industrial Crops Prod. 128, 290–297. doi:10.1016/j.indcrop.2018.11.028
He, Q., Hu, Z., Jiang, Y., Chang, X., Tu, Z., and Zhang, L. (2010). Preconcentration of Cu(II), Fe(III) and Pb(II) with 2-((2-aminoethylamino)methyl)phenol-functionalized activated carbon followed by ICP-OES determination. J. Hazard. Mater. 175, 710–714. doi:10.1016/j.jhazmat.2009.10.067
He, S., Chen, Q., Chen, G., Shi, G., Ruan, C., Feng, M., et al. (2022). N-doped activated carbon for high-efficiency ofloxacin adsorption. Microporous Mesoporous Mater. 335, 111848. doi:10.1016/j.micromeso.2022.111848
Heidari, A., Stahl, R., Younesi, H., Rashidi, A., Troeger, N., and Ghoreyshi, A. A. (2014). Effect of process conditions on product yield and composition of fast pyrolysis of Eucalyptus grandis in fluidized bed reactor. J. Industrial Eng. Chem. 20, 2594–2602. doi:10.1016/j.jiec.2013.10.046
Heydari-Gorji, A., Belmabkhout, Y., and Sayari, A. (2011). Polyethylenimine-impregnated mesoporous silica: Effect of amine loading and surface alkyl chains on CO2 adsorption. Langmuir 27, 12411–12416. doi:10.1021/la202972t
Hou, Z., Tao, Y., Bai, T., Liang, Y., Huang, S., and Cai, J. (2021). Efficient Rhodamine B removal by N-doped hierarchical carbons obtained from KOH activation and urea-oxidation of glucose hydrochar. J. Environ. Chem. Eng. 9, 105757. doi:10.1016/j.jece.2021.105757
Houshmand, A., Wan Daud, W. M. A., and Shafeeyan, M. S. (2011). Exploring potential methods for anchoring amine groups on the surface of activated carbon for CO2 adsorption. Sep. Sci. Technol. 46, 1098–1112. doi:10.1080/01496395.2010.546383
Hulicova-Jurcakova, D., Kodama, M., Shiraishi, S., Hatori, H., Zhu, Z. H., and Lu, G. Q. (2009a). Nitrogen-enriched nonporous carbon electrodes with extraordinary supercapacitance. Adv. Funct. Mater. 19, 1800–1809. doi:10.1002/adfm.200801100
Hulicova-Jurcakova, D., Seredych, M., Lu, G. Q., and Bandosz, T. J. (2009b). Combined effect of nitrogen- and oxygen-containing functional groups of microporous activated carbon on its electrochemical performance in supercapacitors. Adv. Funct. Mater. 19, 438–447. doi:10.1002/adfm.200801236
Hussain, I., Li, Y., Qi, J., Li, J., and Wang, L. (2018). Nitrogen-enriched carbon sheet for methyl blue dye adsorption. J. Environ. Manag. 215, 123–131. doi:10.1016/j.jenvman.2018.03.051
Ilnicka, A., Lukaszewicz, J. P., Shimanoe, K., and Yuasa, M. (2018). Urea treatment of nitrogen-doped carbon leads to enhanced performance for the oxygen reduction reaction. J. Mater. Res. 33, 1612–1624. doi:10.1557/jmr.2018.116
Ilnicka, A., and Lukaszewicz, J. P. (2015). Synthesis of N-rich microporous carbon materials from chitosan by alkali activation using Na2CO3. Mater. Sci. Eng. B 201, 66–71. doi:10.1016/j.mseb.2015.08.002
Jansen, R. J. J., and Van Bekkum, H. (1994). Amination and ammoxidation of activated carbons. Carbon 32, 1507–1516. doi:10.1016/0008-6223(94)90146-5
Jia, Y. F., Xiao, B., and Thomas, K. M. (2002). Adsorption of metal ions on nitrogen surface functional groups in activated carbons. Langmuir 18, 470–478. doi:10.1021/la011161z
Jiang, H.-L., Liu, B., Lan, Y.-Q., Kuratani, K., Akita, T., Shioyama, H., et al. (2011). From metal–organic framework to nanoporous carbon: Toward a very high surface area and hydrogen uptake. J. Am. Chem. Soc. 133, 11854–11857. doi:10.1021/ja203184k
Jiang, H., Lee, P. S., and Li, C. (2013). 3D carbon based nanostructures for advanced supercapacitors. Energy and Environ. Sci. 6, 41–53. doi:10.1039/c2ee23284g
Jin, Z., Wang, B., Ma, L., Fu, P., Xie, L., Jiang, X., et al. (2020). Air pre-oxidation induced high yield N-doped porous biochar for improving toluene adsorption. Chem. Eng. J. 385, 123843. doi:10.1016/j.cej.2019.123843
Jirka, S., and Tomlinson, T. (2015). State of the biochar industry 2014. A survey of commercial activity in the biochar sector. United States: International Biochar Initiative.
Jirka, S., and Tomlinson, T. (2013). State of the biochar industry—a survey of commercial activity in the biochar field. United States: International Biochar Initiative.
Kalyani, P., and Anitha, A. (2013). Biomass carbon and its prospects in electrochemical energy systems. Int. J. Hydrogen Energy 38, 4034–4045. doi:10.1016/j.ijhydene.2013.01.048
Karnjanakom, S., and Maneechakr, P. (2019). Adsorption behaviors and capacities of Cr(VI) onto environmentally activated carbon modified by cationic (HDTMA and DDAB) surfactants. J. Mol. Struct. 1186, 80–90. doi:10.1016/j.molstruc.2019.03.022
Kesavan, T., and Sasidharan, M. (2019). Palm spathe derived N-doped carbon nanosheets as a high performance electrode for Li-ion batteries and supercapacitors. ACS Sustain. Chem. Eng. 7, 9b01261–12169. doi:10.1021/acssuschemeng.9b01261
Khalil, S. H., Aroua, M. K., and Daud, W. M. A. W. (2012). Study on the improvement of the capacity of amine-impregnated commercial activated carbon beds for CO2 adsorbing. Chem. Eng. J. 183, 15–20. doi:10.1016/j.cej.2011.12.011
Khan, M. J. H., Hussain, M. A., Mansourpour, Z., Mostoufi, N., Ghasem, N. M., and Abdullah, E. C. (2014). CFD simulation of fluidized bed reactors for polyolefin production – a review. J. Industrial Eng. Chem. 20, 3919–3946. doi:10.1016/j.jiec.2014.01.044
Khan, M. J. H., Hussain, M. A., and Mujtaba, I. M. (2016a). Developed hybrid model for propylene polymerisation at optimum reaction conditions. Polymers 8, 47. doi:10.3390/polym8020047
Khan, M. J. H., Hussain, M. A., and Mujtaba, I. M. (2016b). Multiphasic reaction modeling for polypropylene production in a pilot-scale catalytic reactor. Polymers 8, 220. doi:10.3390/polym8060220
Kist, L. T., Rosa, E. C., Machado, Ê. L., Camargo, M. E., and Moro, C. C. (2013). Glutaraldehyde degradation in hospital wastewater by photoozonation. Environ. Technol. 34, 2579–2586. doi:10.1080/09593330.2013.781200
Laheäär, A., Delpeux-Ouldriane, S., Lust, E., and Béguin, F. (2014). Ammonia treatment of activated carbon powders for supercapacitor electrode application. J. Electrochem. Soc. 161, A568–A575. doi:10.1149/2.051404jes
Le Leuch, L. M., and Bandosz, T. J. (2007). The role of water and surface acidity on the reactive adsorption of ammonia on modified activated carbons. Carbon 45, 568–578. doi:10.1016/j.carbon.2006.10.016
Lesaoana, M., Mlaba, R. P. V., Mtunzi, F. M., Klink, M. J., Ejidike, P., and Pakade, V. E. (2019). Influence of inorganic acid modification on Cr(VI) adsorption performance and the physicochemical properties of activated carbon. South Afr. J. Chem. Eng. 28, 8–18. doi:10.1016/j.sajce.2019.01.001
Li, G.-X., Hou, P.-X., Zhao, S.-Y., Liu, C., and Cheng, H.-M. (2016). A flexible cotton-derived carbon sponge for high-performance capacitive deionization. Carbon 101, 1–8. doi:10.1016/j.carbon.2015.12.095
Li, J., Michalkiewicz, B., Min, J., Ma, C., Chen, X., Gong, J., et al. (2019). Selective preparation of biomass-derived porous carbon with controllable pore sizes toward highly efficient CO2 capture. Chem. Eng. J. 360, 250–259. doi:10.1016/j.cej.2018.11.204
Li, Q., Hou, Y., Wang, J., Liu, Y., Xiang, N., and Huang, Z. (2020). Superiority of raw biomass and potassium hydroxide in preparation of ultrahigh nitrogen doping of carbon for NH3-SCR reaction. ACS Sustain. Chem. Eng. 8, 11308–11316. doi:10.1021/acssuschemeng.0c03193
Lian, F., Cui, G., Liu, Z., Duo, L., Zhang, G., and Xing, B. (2016). One-step synthesis of a novel N-doped microporous biochar derived from crop straws with high dye adsorption capacity. J. Environ. Manag. 176, 61–68. doi:10.1016/j.jenvman.2016.03.043
Liang, H., Sun, R., Song, B., Sun, Q., Peng, P., and She, D. (2020). Preparation of nitrogen-doped porous carbon material by a hydrothermal-activation two-step method and its high-efficiency adsorption of Cr(VI). J. Hazard. Mater. 387, 121987. doi:10.1016/j.jhazmat.2019.121987
Lim, S., Suh, K., Kim, Y., Yoon, M., Park, H., Dybtsev, D. N., et al. (2012). Porous carbon materials with a controllable surface area synthesized from metal–organic frameworks. Chem. Commun. 48, 7447–7449. doi:10.1039/c2cc33439a
Liu, H., Gao, Q., Dai, P., Zhang, J., Zhang, C., and Bao, N. (2013). Preparation and characterization of activated carbon from lotus stalk with guanidine phosphate activation: Sorption of Cd(II). J. Anal. Appl. Pyrolysis 102, 7–15. doi:10.1016/j.jaap.2013.04.010
Liu, S., Ma, R., Hu, X., Wang, L., Wang, X., Radosz, M., et al. (2020). CO2 Adsorption on hazelnut-shell-derived nitrogen-doped porous carbons synthesized by single-step sodium amide activation. Industrial Eng. Chem. Res. 59, 7046–7053. doi:10.1021/acs.iecr.9b02127
Liu, S., Yang, P., Wang, L., Li, Y., Wu, Z., Ma, R., et al. (2019a). Nitrogen-doped porous carbons from lotus leaf for CO2 capture and supercapacitor electrodes. Energy and Fuels 33, 6568–6576. doi:10.1021/acs.energyfuels.9b00886
Liu, Y., Dai, G., Zhu, L., and Wang, S. (2019b). Green conversion of microalgae into high-performance sponge-like nitrogen-enriched carbon. ChemElectroChem 6, 646–652. doi:10.1002/celc.201801272
Lloyd, M., Maurice, O. S., Aoyi, O., and Leswifi, T. Y. (2015). Sorption characteristics of mixed molecules of glutaraldehyde from water on mesoporous acid-amine modified low-cost activated carbon: Mechanism, isotherm, and kinetics. J. Chem. 2015, 1–13. doi:10.1155/2015/757256
Lorenc-Grabowska, E., Gryglewicz, G., and Diez, M. A. (2013). Kinetics and equilibrium study of phenol adsorption on nitrogen-enriched activated carbons. Fuel 114, 235–243. doi:10.1016/j.fuel.2012.11.056
Luo, L., Lan, Y., Zhang, Q., Deng, J., Luo, L., Zeng, Q., et al. (2022). A review on biomass-derived activated carbon as electrode materials for energy storage supercapacitors. J. Energy Storage 55, 105839. doi:10.1016/j.est.2022.105839
Luo, M., Wang, L., Li, H., Bu, Y., Zhao, Y., and Cai, J. (2023). Hierarchical porous biochar from kelp: Insight into self-template effect and highly efficient removal of methylene blue from water. Bioresour. Technol. 372, 128676. doi:10.1016/j.biortech.2023.128676
Luo, W., Wang, B., Heron, C. G., Allen, M. J., Morre, J., Maier, C. S., et al. (2014). Pyrolysis of cellulose under ammonia leads to nitrogen-doped nanoporous carbon generated through methane formation. Nano Lett. 14, 2225–2229. doi:10.1021/nl500859p
Lupul, I., Yperman, J., Carleer, R., and Gryglewicz, G. (2015). Adsorption of atrazine on hemp stem-based activated carbons with different surface chemistry. Adsorption 21, 489–498. doi:10.1007/s10450-015-9689-1
Mailler, R., Gasperi, J., Coquet, Y., Buleté, A., Vulliet, E., Deshayes, S., et al. (2016). Removal of a wide range of emerging pollutants from wastewater treatment plant discharges by micro-grain activated carbon in fluidized bed as tertiary treatment at large pilot scale. Sci. Total Environ. 542, 983–996. doi:10.1016/j.scitotenv.2015.10.153
Mangun, C. L., Benak, K. R., Economy, J., and Foster, K. L. (2001). Surface chemistry, pore sizes and adsorption properties of activated carbon fibers and precursors treated with ammonia. Carbon 39, 1809–1820. doi:10.1016/s0008-6223(00)00319-5
Mazaheri, H., Ghaedi, M., Ahmadi Azqhandi, M. H., and Asfaram, A. (2017). Application of machine/statistical learning, artificial intelligence and statistical experimental design for the modeling and optimization of methylene blue and Cd(ii) removal from a binary aqueous solution by natural walnut carbon. Phys. Chem. Chem. Phys. 19, 11299–11317. doi:10.1039/c6cp08437k
Mei, S., Gu, J., Ma, T., Li, X., Hu, Y., Li, W., et al. (2019). N-doped activated carbon from used dyeing wastewater adsorbent as a metal-free catalyst for acetylene hydrochlorination. Chem. Eng. J. 371, 118–129. doi:10.1016/j.cej.2019.04.008
Meng, X., and Hu, R. (2021). Nitrogen/phosphorus enriched biochar with enhanced porosity activated by guanidine phosphate for efficient passivation of Pb(II), Cu(II) and Cd(II). J. Mol. Liq. 323, 115071. doi:10.1016/j.molliq.2020.115071
Mochizuki, Y., Bud, J., Byambajav, E., and Tsubouchi, N. (2022). Influence of ammonia treatment on the CO2 adsorption of activated carbon. J. Environ. Chem. Eng. 10, 107273. doi:10.1016/j.jece.2022.107273
Mohamed, A. R., Mohammadi, M., and Darzi, G. N. (2010). Preparation of carbon molecular sieve from lignocellulosic biomass: A review. Renew. Sustain. Energy Rev. 14, 1591–1599. doi:10.1016/j.rser.2010.01.024
Monser, L., and Adhoum, N. (2002). Modified activated carbon for the removal of copper, zinc, chromium and cyanide from wastewater. Sep. Purif. Technol. 26, 137–146. doi:10.1016/s1383-5866(01)00155-1
Moreno-Castilla, C. (2004). Adsorption of organic molecules from aqueous solutions on carbon materials. Carbon 42, 83–94. doi:10.1016/j.carbon.2003.09.022
Mostazo-López, M. J., Ruiz-Rosas, R., Morallón, E., and Cazorla-Amorós, D. (2015). Generation of nitrogen functionalities on activated carbons by amidation reactions and Hofmann rearrangement: Chemical and electrochemical characterization. Carbon 91, 252–265. doi:10.1016/j.carbon.2015.04.089
Nahar, S., Khan, M. A., Khan, R. A., Abdullah, E. C. B., Khan, M. J. H., Islam, R., et al. (2013). An approach to utilize crust leather scrapes, dumped into the land, for the production of environmental friendly leather composite. Eng. J. 17, 17–24. doi:10.4186/ej.2013.17.3.17
Nguyen, D. H., Tran, H. N., Chao, H.-P., and Lin, C.-C. (2019). Effect of nitric acid oxidation on the surface of hydrochars to sorb methylene blue: An adsorption mechanism comparison. Adsorpt. Sci. Technol. 37, 607–622. doi:10.1177/0263617419867519
Niu, X., Liu, C., Li, L., Han, X., Chang, C., Li, P., et al. (2022). High specific surface area N-doped activated carbon from hydrothermal carbonization of shaddock peel for the removal of norfloxacin from aqueous solution. Water Sci. Technol. 85, 2964–2979. doi:10.2166/wst.2022.163
Nowicki, P., Pietrzak, R., and Wachowska, H. (2009). Influence of the precursor metamorphism degree on preparation of nitrogen-enriched activated carbons by ammoxidation and chemical activation of coals. Energy and Fuels 23, 2205–2212. doi:10.1021/ef801094c
Ntakirutimana, S., Tan, W., and Wang, Y. (2019). Enhanced surface activity of activated carbon by surfactants synergism. RSC Adv. 9, 26519–26531. doi:10.1039/c9ra04521j
Oh, W.-D., Veksha, A., Chen, X., Adnan, R., Lim, J.-W., Leong, K.-H., et al. (2019). Catalytically active nitrogen-doped porous carbon derived from biowastes for organics removal via peroxymonosulfate activation. Chem. Eng. J. 374, 947–957. doi:10.1016/j.cej.2019.06.001
Osman, N. B., Shamsuddin, N., and Uemura, Y. (2016). Activated carbon of oil palm empty fruit bunch (EFB); core and shaggy. Procedia Eng. 148, 758–764. doi:10.1016/j.proeng.2016.06.610
Padhye, L. P., Hertzberg, B., Yushin, G., and Huang, C.-H. (2011). N-nitrosamines formation from secondary amines by nitrogen fixation on the surface of activated carbon. Environ. Sci. Technol. 45, 8368–8376. doi:10.1021/es201696e
Pan, C., Qiu, L., Peng, Y., and Yan, F. (2012). Facile synthesis of nitrogen-doped carbon–Pt nanoparticle hybrids via carbonization of poly([Bvim] [Br]-co-acrylonitrile) for electrocatalytic oxidation of methanol. J. Mater. Chem. 22, 13578–13584. doi:10.1039/c2jm31973j
Paul, R., Du, F., Dai, L., Ding, Y., Wang, Z. L., Wei, F., et al. (2019). 3D heteroatom-doped carbon nanomaterials as multifunctional metal-free catalysts for integrated energy devices. Adv. Mater. 31, 1805598. doi:10.1002/adma.201805598
Pei, H., Wang, J., Yang, Q., Yang, W., Hu, N., Suo, Y., et al. (2018). Interfacial growth of nitrogen-doped carbon with multi-functional groups on the MoS2 skeleton for efficient Pb(II) removal. Sci. Total Environ. 631-632, 912–920. doi:10.1016/j.scitotenv.2018.02.324
Pels, J. R., Kapteijn, F., Moulijn, J. A., Zhu, Q., and Thomas, K. M. (1995). Evolution of nitrogen functionalities in carbonaceous materials during pyrolysis. Carbon 33, 1641–1653. doi:10.1016/0008-6223(95)00154-6
Pendleton, P., Wu, S. H., and Badalyan, A. (2002). Activated carbon oxygen content influence on water and surfactant adsorption. J. Colloid Interface Sci. 246, 235–240. doi:10.1006/jcis.2001.8052
Pevida, C., Plaza, M. G., Arias, B., Fermoso, J., Rubiera, F., and Pis, J. J. (2008). Surface modification of activated carbons for CO2 capture. Appl. Surf. Sci. 254, 7165–7172. doi:10.1016/j.apsusc.2008.05.239
Pietrzak, R., Nowicki, P., and Wachowska, H. (2009). The influence of oxidation with nitric acid on the preparation and properties of active carbon enriched in nitrogen. Appl. Surf. Sci. 255, 3586–3593. doi:10.1016/j.apsusc.2008.10.002
Pietrzak, R., Wachowska, H., and Nowicki, P. (2006). Preparation of nitrogen-enriched activated carbons from Brown coal. Energy and Fuels 20, 1275–1280. doi:10.1021/ef0504164
Przepiórski, J. (2006). Enhanced adsorption of phenol from water by ammonia-treated activated carbon. J. Hazard. Mater. 135, 453–456. doi:10.1016/j.jhazmat.2005.12.004
Qu, Y., Zhang, Z., Zhang, X., Ren, G., Lai, Y., Liu, Y., et al. (2015). Highly ordered nitrogen-rich mesoporous carbon derived from biomass waste for high-performance lithium–sulfur batteries. Carbon 84, 399–408. doi:10.1016/j.carbon.2014.12.001
Radkevich, V. Z., Senko, T. L., Wilson, K., Grishenko, L. M., Zaderko, A. N., and Diyuk, V. Y. (2008). The influence of surface functionalization of activated carbon on palladium dispersion and catalytic activity in hydrogen oxidation. Appl. Catal. A General 335, 241–251. doi:10.1016/j.apcata.2007.11.029
Rao, L., Liu, S., Wang, L., Ma, C., Wu, J., An, L., et al. (2019a). N-doped porous carbons from low-temperature and single-step sodium amide activation of carbonized water chestnut shell with excellent CO2 capture performance. Chem. Eng. J. 359, 428–435. doi:10.1016/j.cej.2018.11.065
Rao, L., Ma, R., Liu, S., Wang, L., Wu, Z., Yang, J., et al. (2019b). Nitrogen enriched porous carbons from d-glucose with excellent CO2 capture performance. Chem. Eng. J. 362, 794–801. doi:10.1016/j.cej.2019.01.093
Rao, M. M., Rao, G. P. C., Seshaiah, K., Choudary, N. V., and Wang, M. C. (2008). Activated carbon from Ceiba pentandra hulls, an agricultural waste, as an adsorbent in the removal of lead and zinc from aqueous solutions. Waste Manag. 28, 849–858. doi:10.1016/j.wasman.2007.01.017
Rehman, A., Park, M., and Park, S.-J. (2019). Current progress on the surface chemical modification of carbonaceous materials. Coatings 9, 103. doi:10.3390/coatings9020103
Rivera-Utrilla, J., Sánchez-Polo, M., Gómez-Serrano, V., Álvarez, P. M., Alvim-Ferraz, M. C. M., and Dias, J. M. (2011). Activated carbon modifications to enhance its water treatment applications. An overview. J. Hazard. Mater. 187, 1–23. doi:10.1016/j.jhazmat.2011.01.033
Sakaushi, K., and Antonietti, M. (2015). Carbon- and nitrogen-based porous solids: A recently emerging class of materials. Bull. Chem. Soc. Jpn. 88, 386–398. doi:10.1246/bcsj.20140317
Salame, I. I., and Bandosz, T. J. (2003). Role of surface chemistry in adsorption of phenol on activated carbons. J. Colloid Interface Sci. 264, 307–312. doi:10.1016/s0021-9797(03)00420-x
Schaber, P. M., Colson, J., Higgins, S., Thielen, D., Anspach, B., and Brauer, J. (2004). Thermal decomposition (pyrolysis) of urea in an open reaction vessel. Thermochim. Acta 424, 131–142. doi:10.1016/j.tca.2004.05.018
Sellin, N., Krohl, D. R., Marangoni, C., and Souza, O. (2016). Oxidative fast pyrolysis of banana leaves in fluidized bed reactor. Renew. Energy 96, 56–64. doi:10.1016/j.renene.2016.04.032
Seredych, M., Hulicova-Jurcakova, D., Lu, G. Q., and Bandosz, T. J. (2008). Surface functional groups of carbons and the effects of their chemical character, density and accessibility to ions on electrochemical performance. Carbon 46, 1475–1488. doi:10.1016/j.carbon.2008.06.027
Seredych, M., Van Der Merwe, M., and Bandosz, T. J. (2009). Effects of surface chemistry on the reactive adsorption of hydrogen cyanide on activated carbons. Carbon 47, 2456–2465. doi:10.1016/j.carbon.2009.04.037
Shen, W., and Fan, W. (2013). Nitrogen-containing porous carbons: Synthesis and application. J. Mater. Chem. A 1, 999–1013. doi:10.1039/c2ta00028h
Shi, Q., Zhang, R., Lv, Y., Deng, Y., Elzatahrya, A. A., and Zhao, D. (2015). Nitrogen-doped ordered mesoporous carbons based on cyanamide as the dopant for supercapacitor. Carbon 84, 335–346. doi:10.1016/j.carbon.2014.12.013
Shi, Y., Chen, J., Chen, J., Macleod, R. A., and Malac, M. (2012). Preparation and evaluation of hydrotreating catalysts based on activated carbon derived from oil sand petroleum coke. Appl. Catal. A General 441-442, 99–107. doi:10.1016/j.apcata.2012.07.014
Shin, K. Y., Hong, J. Y., and Jang, J. (2011). Heavy metal ion adsorption behavior in nitrogen-doped magnetic carbon nanoparticles: Isotherms and kinetic study. J. Hazard Mater 190, 36–44. doi:10.1016/j.jhazmat.2010.12.102
Stöhr, B., Boehm, H. P., and Schlögl, R. (1991). Enhancement of the catalytic activity of activated carbons in oxidation reactions by thermal treatment with ammonia or hydrogen cyanide and observation of a superoxide species as a possible intermediate. Carbon 29, 707–720. doi:10.1016/0008-6223(91)90006-5
Sun, L., Tian, C., Fu, Y., Yang, Y., Yin, J., Wang, L., et al. (2014). Nitrogen-doped porous graphitic carbon as an excellent electrode material for advanced supercapacitors. Chem. – A Eur. J. 20, 564–574. doi:10.1002/chem.201303345
Szymański, G. S., Grzybek, T., and Papp, H. (2004). Influence of nitrogen surface functionalities on the catalytic activity of activated carbon in low temperature SCR of NOx with NH3. Catal. Today 90, 51–59. doi:10.1016/j.cattod.2004.04.008
Tanada, S., Kawasaki, N., Nakamura, T., Araki, M., and Isomura, M. (1999). Removal of formaldehyde by activated carbons containing amino groups. J. Colloid Interface Sci. 214, 106–108. doi:10.1006/jcis.1999.6176
Ternero-Hidalgo, J. J., Rosas, J. M., Palomo, J., Valero-Romero, M. J., Rodríguez-Mirasol, J., and Cordero, T. (2016). Functionalization of activated carbons by HNO3 treatment: Influence of phosphorus surface groups. Carbon 101, 409–419. doi:10.1016/j.carbon.2016.02.015
Ünveren, E. E., Monkul, B. Ö., Sarıoğlan, Ş., Karademir, N., and Alper, E. (2017). Solid amine sorbents for CO2 capture by chemical adsorption: A review. Petroleum 3, 37–50. doi:10.1016/j.petlm.2016.11.001
Valentín-Reyes, J., García-Reyes, R. B., García-González, A., Soto-Regalado, E., and Cerino-Córdova, F. (2019). Adsorption mechanisms of hexavalent chromium from aqueous solutions on modified activated carbons. J. Environ. Manag. 236, 815–822. doi:10.1016/j.jenvman.2019.02.014
Vidic, R. D., and Siler, D. P. (2001). Vapor-phase elemental mercury adsorption by activated carbon impregnated with chloride and chelating agents. Carbon 39, 3–14. doi:10.1016/s0008-6223(00)00081-6
Wan, H., and Hu, X. (2019). Nitrogen doped biomass-derived porous carbon as anode materials of lithium ion batteries. Solid State Ionics 341, 115030. doi:10.1016/j.ssi.2019.115030
Wang, B., Wang, Y., Peng, Y., Wang, X., Wang, N., Wang, J., et al. (2018). Nitrogen-doped biomass-based hierarchical porous carbon with large mesoporous volume for application in energy storage. Chem. Eng. J. 348, 850–859. doi:10.1016/j.cej.2018.05.061
Wang, J., Wang, Y., Liang, Y., Zhou, J., Liu, L., Huang, S., et al. (2021). Nitrogen-doped carbons from in-situ glucose-coated ZIF-8 as efficient adsorbents for Rhodamine B removal from wastewater. Microporous Mesoporous Mater. 310, 110662. doi:10.1016/j.micromeso.2020.110662
Wang, J., Xue, C., Wu, Z., Li, W., Lv, Y., Asiri, A. M., et al. (2012). Hollow micro-mesoporous carbon polyhedra produced by selective removal of skeletal scaffolds. Carbon 50, 2546–2555. doi:10.1016/j.carbon.2012.02.003
Wang, S., Zou, K., Qian, Y., Deng, Y., Zhang, L., and Chen, G. (2019). Insight to the synergistic effect of N-doping level and pore structure on improving the electrochemical performance of sulfur/N-doped porous carbon cathode for Li-S batteries. Carbon 144, 745–755. doi:10.1016/j.carbon.2018.12.113
Wang, T.-H., Chen, C.-C., Xu, R.-X., Chen, C.-W., and Dong, C.-D. (2022). Upgrading waste activated carbon by equipping micro-/mesopore-dominant microstructures from the perspective of circular economy. Processes 10, 1631. doi:10.3390/pr10081631
Watanabe, H., Asano, S., Fujita, S.-I., Yoshida, H., and Arai, M. (2015). Nitrogen-doped, metal-free activated carbon catalysts for aerobic oxidation of alcohols. ACS Catal. 5, 2886–2894. doi:10.1021/acscatal.5b00375
Weast, R. C., and Astle, M. J. (1982). CRC handbook of chemistry and physics: A ready-reference book of chemical and physical data. Boca Raton, Fla: CRC Press.
Wenzhong, S., Zhijie, L., and Yihong, L. (2008). Surface chemical functional groups modification of porous carbon. Recent Pat. Chem. Eng. Discontin. 1, 27–40. doi:10.2174/2211334710801010027
Wood, K. N., O'hayre, R., and Pylypenko, S. (2014). Recent progress on nitrogen/carbon structures designed for use in energy and sustainability applications. Energy and Environ. Sci. 7, 1212–1249. doi:10.1039/c3ee44078h
Wright, E. H. M. (1967). The effect of surface complexes on carbons on the nature of the adsorbed phase in the adsorption of monocarboxylic acids and their methyl esters from solution. J. Colloid Interface Sci. 24, 180–184. doi:10.1016/0021-9797(67)90216-0
Xia, K., Gao, Q., Song, S., Wu, C., Jiang, J., Hu, J., et al. (2008). CO2 activation of ordered porous carbon CMK-1 for hydrogen storage. Int. J. Hydrogen Energy 33, 116–123. doi:10.1016/j.ijhydene.2007.08.019
Xie, F., Phillips, J., Silva, I. F., Palma, M. C., and Menéndez, J. A. (2000). Microcalorimetric study of acid sites on ammonia- and acid-pretreated activated carbon. Carbon 38, 691–700. doi:10.1016/s0008-6223(99)00156-6
Xie, R., Jin, Y., Chen, Y., and Jiang, W. (2017). The importance of surface functional groups in the adsorption of copper onto walnut shell derived activated carbon. Water Sci. Technol. 76, 3022–3034. doi:10.2166/wst.2017.471
Xu, B., Hou, S., Cao, G., Wu, F., and Yang, Y. (2012). Sustainable nitrogen-doped porous carbon with high surface areas prepared from gelatin for supercapacitors. J. Mater. Chem. 22, 19088–19093. doi:10.1039/c2jm32759g
Xu, D., Tong, Y., Yan, T., Shi, L., and Zhang, D. (2017). N,P-codoped meso-/microporous carbon derived from biomass materials via a dual-activation strategy as high-performance electrodes for deionization capacitors. ACS Sustain. Chem. Eng. 5, 5810–5819. doi:10.1021/acssuschemeng.7b00551
Xu, L., Shi, C., He, Z., Zhang, H., Chen, M., Fang, Z., et al. (2020). Recent advances of producing biobased N-containing compounds via thermo-chemical conversion with ammonia process. Energy fuels. 34 (9), 10441–10458. doi:10.1021/acs.energyfuels.0c01993
Xu, L., Wu, C., Liu, P., Bai, X., Du, X., Jin, P., et al. (2020). Peroxymonosulfate activation by nitrogen-doped biochar from sawdust for the efficient degradation of organic pollutants. Chem. Eng. J. 387, 124065. doi:10.1016/j.cej.2020.124065
Yang, G., Chen, H., Qin, H., and Feng, Y. (2014). Amination of activated carbon for enhancing phenol adsorption: Effect of nitrogen-containing functional groups. Appl. Surf. Sci. 293, 299–305. doi:10.1016/j.apsusc.2013.12.155
Yang, X., Wan, Y., Zheng, Y., He, F., Yu, Z., Huang, J., et al. (2019). Surface functional groups of carbon-based adsorbents and their roles in the removal of heavy metals from aqueous solutions: A critical review. Chem. Eng. J. 366, 608–621. doi:10.1016/j.cej.2019.02.119
Yantasee, W., Lin, Y., Fryxell, G. E., Alford, K. L., Busche, B. J., and Johnson, C. D. (2004). Selective removal of copper(II) from aqueous solutions using fine-grained activated carbon functionalized with amine. Industrial Eng. Chem. Res. 43, 2759–2764. doi:10.1021/ie030182g
Yuan, W., Feng, Y., Xie, A., Zhang, X., Huang, F., Li, S., et al. (2016). Nitrogen-doped nanoporous carbon derived from waste pomelo peel as a metal-free electrocatalyst for the oxygen reduction reaction. Nanoscale 8, 8704–8711. doi:10.1039/c6nr00764c
Yue, L., Xia, Q., Wang, L., Wang, L., Dacosta, H., Yang, J., et al. (2018). CO2 adsorption at nitrogen-doped carbons prepared by K2CO3 activation of urea-modified coconut shell. J. Colloid Interface Sci. 511, 259–267. doi:10.1016/j.jcis.2017.09.040
Yue, Z., Bender, S. E., Wang, J., and Economy, J. (2009). Removal of chromium Cr(VI) by low-cost chemically activated carbon materials from water. J. Hazard. Mater. 166, 74–78. doi:10.1016/j.jhazmat.2008.10.125
Zhai, Y., Xu, B., Zhu, Y., Qing, R., Peng, C., Wang, T., et al. (2016). Nitrogen-doped porous carbon from Camellia oleifera shells with enhanced electrochemical performance. Mater. Sci. Eng. C 61, 449–456. doi:10.1016/j.msec.2015.12.079
Zhang, C., Song, W., Ma, Q., Xie, L., Zhang, X., and Guo, H. (2016). Enhancement of CO2 capture on biomass-based carbon from black locust by KOH activation and ammonia modification. Energy and Fuels 30, 4181–4190. doi:10.1021/acs.energyfuels.5b02764
Zhang, S., Shi, X., Chen, X., Zhang, D., Liu, X., Zhang, Z., et al. (2019a). Large-scale and low-cost motivation of nitrogen-doped commercial activated carbon for high-energy-density supercapacitor. ACS Appl. Energy Mater. 2, 4234–4243. doi:10.1021/acsaem.9b00481
Zhang, X., Zhang, S., Yang, H., Feng, Y., Chen, Y., Wang, X., et al. (2014). Nitrogen enriched biochar modified by high temperature CO2–ammonia treatment: Characterization and adsorption of CO2. Chem. Eng. J. 257, 20–27. doi:10.1016/j.cej.2014.07.024
Zhang, Z., Yang, S., Li, H., Zan, Y., Li, X., Zhu, Y., et al. (2019b). Sustainable carbonaceous materials derived from biomass as metal-free electrocatalysts. Adv. Mater. 31, 1805718. doi:10.1002/adma.201805718
Zhao, C., Liu, G., Sun, N., Zhang, X., Wang, G., Zhang, Y., et al. (2018). Biomass-derived N-doped porous carbon as electrode materials for Zn-air battery powered capacitive deionization. Chem. Eng. J. 334, 1270–1280. doi:10.1016/j.cej.2017.11.069
Zhao, H.-B., Wang, W.-D., Lü, Q.-F., Lin, T.-T., Lin, Q., and Yang, H. (2015). Preparation and application of porous nitrogen-doped graphene obtained by co-pyrolysis of lignosulfonate and graphene oxide. Bioresour. Technol. 176, 106–111. doi:10.1016/j.biortech.2014.11.035
Zhao, X., Zhao, H., Zhang, T., Yan, X., Yuan, Y., Zhang, H., et al. (2014). One-step synthesis of nitrogen-doped microporous carbon materials as metal-free electrocatalysts for oxygen reduction reaction. J. Mater. Chem. A 2, 11666–11671. doi:10.1039/c4ta00846d
Zhao, Y., Hu, C., Hu, Y., Cheng, H., Shi, G., and Qu, L. (2012). A versatile, ultralight, nitrogen-doped graphene framework. Angew. Chem. Int. Ed. 51, 11371–11375. doi:10.1002/anie.201206554
Zhao, Z., Ma, C., Chen, F., Xu, G., Pang, R., Qian, X., et al. (2021). Water caltrop shell-derived nitrogen-doped porous carbons with high CO2 adsorption capacity. Biomass Bioenergy 145, 105969. doi:10.1016/j.biombioe.2021.105969
Zhou, Z., Bu, Y., Long, X., and Cai, J. (2023). N-Containing biochar from oatmeal: Hydrothermal synthesis and used as highly efficient adsorbent for Cr(VI) adsorptive-reduction removal. Biomass Convers. Biorefinery 2023, 1–10. doi:10.1007/s13399-023-03955-x
Zhu, J., Deng, B., Yang, J., and Gang, D. (2009). Modifying activated carbon with hybrid ligands for enhancing aqueous mercury removal. Carbon 47, 2014–2025. doi:10.1016/j.carbon.2009.03.047
Keywords: plant derived adsorbent, nitrogen doped activated carbon, adsorption efficiency, liquid-phase adsorption, water treatment
Citation: Bumajdad A, Khan MJH and Lukaszewicz JP (2023) Nitrogen-enriched activated carbon derived from plant biomasses: a review on reaction mechanism and applications in wastewater treatment. Front. Mater. 10:1218028. doi: 10.3389/fmats.2023.1218028
Received: 06 May 2023; Accepted: 21 July 2023;
Published: 04 August 2023.
Edited by:
Santosh K. Yadav, Drexel University, United StatesReviewed by:
María José Valero Romero, University of Malaga, SpainJinjun Cai, Xiangtan University, China
Copyright © 2023 Bumajdad, Khan and Lukaszewicz. This is an open-access article distributed under the terms of the Creative Commons Attribution License (CC BY). The use, distribution or reproduction in other forums is permitted, provided the original author(s) and the copyright owner(s) are credited and that the original publication in this journal is cited, in accordance with accepted academic practice. No use, distribution or reproduction is permitted which does not comply with these terms.
*Correspondence: Ali Bumajdad, YS5idW1hamRhZEBrdS5lZHUua3c=