- 1Key Laboratory of Light Field Manipulation and Information Acquisition, Ministry of Industry and Information Technology and School of Physical Science and Technology, Northwestern Polytechnical University, Xi’an, China
- 2Aviation Key Lab of Science and Technology on High Performance Electromagnetic Windows, AVIC Research Institute for Special Structures of Aeronautical Composites, Tsinan, China
Dielectric metamaterials with low ohmic losses and resonating in the local magnetic mode are preferable for enhancing material non-linearity. Here, we propose and experimentally demonstrate broadband extraordinary electromagnetic transmission (EET) behavior, which is induced by the coupling of magnetic modes of two ceramic cuboids. It is shown that extraordinary electromagnetic transmission behavior through a perforated metal sheet with a subwavelength aperture can be achieved by exciting the first-order magnetic mode Mie resonant coupling of these cuboids. Our findings indicate that the transmission bandwidth and amplitude are dependent on the strength of coupling between the two ceramic cuboids. Additionally, we utilized non-linear effects within the dielectric cuboids to achieve tunable extraordinary electromagnetic transmission behavior. Our results are promising for developing non-linearly tunable microwave devices such as filters and modulators of their strong light-matter interactions.
1 Introduction
Metamaterials, and their two-dimensional counterpart metasurfaces, are artificial media made up of subwavelength-sized resonant elements with tremendous potential for modulating wave beams (Pendry, 2000; Shelby et al., 2001; Smith et al., 2004; Chen et al., 2010; Fan et al., 2011; Liu et al., 2011; Soukoulis and Wegener, 2011; Chen et al., 2012; Jahani and Jacob, 2016; Yang et al., 2021a; Liu et al., 2021; He and Song, 2022; Yang et al., 2023a; Yang et al., 2023b; Li and Song, 2023; Nie et al., 2023). These micro and nano elements can be designed to have optical properties that do not exist in nature, such as negative refraction (Smith et al., 2004), electromagnetic clock (Schurig et al., 2006), electromagnetically induced transparency (Yang et al., 2021a), and metalens (Chen et al., 2012). Recently, researchers have combined metamaterials with the structure of single apertures to enhance transmission efficiency for light. This reduces the size of the structure while increasing its transmission efficiency (Guo and Zhou, 2014; Guo et al., 2016).
Similar research has been conducted in Terahertz frequencies (Navarro-Cía et al., 2018; Freer et al., 2020) and optical bands (Jeong et al., 2015; Qin et al., 2018). Extraordinary optical transmission (EOT) has attracted significant attention owing to its unique physical mechanism and potential applications in photodetectors and non-linear optics (Ebbesen et al., 1998; Martín-Moreno et al., 2001; García de Abajo, 2007; Genet and Ebbesen, 2007; Aydin et al., 2009; Chen et al., 2013). Dielectric metamaterials can achieve higher transmission efficiency than conventional EOT apertures (Hajian et al., 2017a). In the early stages, it was mainly based on periodic array structures to enhance the transmission efficiency of light through subwavelength diaphragms. In 2009, Aydin et al. (2009) achieved strongly localized electromagnetic field coupling and transmission enhancement by applying a single SRR at the near field of the aperture, which initiated the research on extraordinary optical transparency in terahertz band (Chen et al., 2013). Later, this idea was also employed in microwaves to realize high-efficiency extraordinary electromagnetic transmission (EET).
Epsilon-near-zero metamaterials can also achieve efficient EOT/EET behavior (Kurs et al., 2007; Hajian et al., 2017b). However, metamaterials operating in a single resonant mode can only achieve EOT/EET behavior near their resonant frequency. Obtaining EOT/EET behavior within a certain bandwidth range requires introducing more resonant modes and coupling. Andre Kurs et al. proposed the use of strongly coupled self-resonant coils and experimentally demonstrated effective non-radiative power transmission over a distance of up to 8 times the coil radius in Science in 2007 (Zhang et al., 2012; Jonah and Georgakopoulos, 2013). Researchers also found that the first-order Mie resonance of two magnetic mode resonance units will split into two modes: in-phase resonance and reverse resonance (Hao et al., 2008). However, research on broadband EET/EOT is relatively sparse, which is due to the sharp resonance of meta-atoms.
In this work, we have demonstrated the EET behavior of a perforated metal sheet with subwavelength apertures by utilizing near-field coupling of the first-order magnetic mode Mie resonance between two identical ceramic cuboids. The bandwidth of EET was extended by combining the splitting of resonant modes and coupling behavior between ceramic cuboids and metal sheets. Dielectric materials were chosen as resonators to enhance transmission efficiency. By introducing perforated metal sheets to suppress coupling between ceramic cuboids, we increased transmittance in the original transmission dips, achieving broadband transmission instead of double-frequency point transmission. Modulation of transmission amplitude and the frequency band is achieved by varying spacing between two ceramic cuboids to change coupling strength and mode resonance. Further modulation is possible based on non-linear effects in dielectric material for tunable EET frequency. These tunable non-linear EET metamaterials can play an important role in designing new sensor devices and filter devices due to their wider operating frequency range, greater functionality, and lower costs (Hao et al., 2008; Ju et al., 2011; He et al., 2019; Bao and Cui, 2020; Yang et al., 2021b; Zhu et al., 2022).
2 Results and discussion
Figure 1A shows the schematic diagram of double ceramic cuboids placed on a Teflon substrate. The Teflon substrate is with a dimension of 22.86 × 10.16 × 1 mm3, and the two ceramic cuboids are made of low-loss material calcium titanate (CaTiO3) doped with 1wt% ZrO2, having a relative permittivity of approximately 121 (Zhao et al., 2008; Zhao et al., 2009; Luo et al., 2015). The CaTiO3 ceramic was synthesized through the conventional solid-state method. All the raw powders of CaTiO3 and ZrO2 were mixed by ball milling in deionized water for 30 h. After dried, the powders were pestled in agate mortar and sieved through 50 or 60-mesh sieve. Then, the PVA solution (polyvinyl alcohol, 5 wt%) was added to the sieved powders as organic binder. The cylinders were formed by uniaxial compression at the pressure of 4 MPa with a customized mould. Finally, the cylinders were sintered at the temperature ranging from 1,250°C to 1,400°C (Luo et al., 2015). Each ceramic cuboid has dimensions of 3.5 × 3.5 × 1 mm3. We employed a finite-difference time-domain (FDTD) method to calculate the transmission spectra of the EET metamaterial. In the simulations, electric boundary conditions were set along the x- and y-directions to simulate a standard waveguide of WR90, and perfect match layers (PMLs) were set along the z-direction. The transmission and reflection spectra were calculated for two ceramic cuboids in the WR90 waveguide, and the results are shown in Figure 1B. For the transmission spectrum, two resonance dips appear at 8.776, and 9 GHz with a transmittance of 0.132, and 0.038 respectively, and a transmission peak appears at 8.852 GHz with a transmittance of 0.758. A typical first-order magnetic mode Mie resonance may be seen in the circular distribution of the magnetic field in the ceramic block as shown in the insert magnetic field distribution with a frequency of 8.776 GHz, which confirms that the ceramic cuboid is a magnetic dipole resonance.
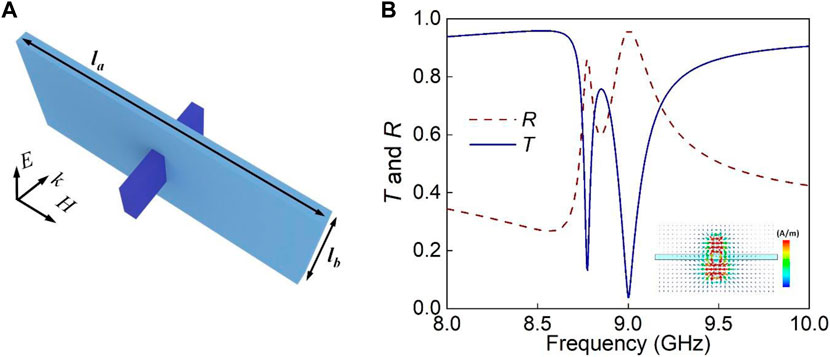
FIGURE 1. (A) Schematic diagram of our design with two ceramic cuboids on opposite sides of the Teflon substrate and (B) its reflection and transmission spectra in waveguide WR90, the insert shows the magnetic field distribution with a frequency of 8.776 GHz.
Figure 2A shows that when two ceramic cuboids are placed on opposite sides of a small hole, there is a distinct peak in dual-frequency transmission, where the small hole diameter is 6 mm. The red dotted line in Figure 2B shows the transmittance when only the perforated metal sheet is inserted in the waveguide. Simulated results reveal two transmission peaks at frequencies of 9.02 and 9.14 GHz, while experimental findings show transmission maxima at 8.94 and 9.095 GHz with respective transmittances of 0.6 and 0.742. Note that the experimental EET results have slightly lower frequency and peak values than those from the simulation. This may be attributed to the uniformity doping of the samples during manufacture and small offset in the position of the samples during the experimental operation. However, the general features of the measured spectra agree well with the numerical simulations. Unlike coupling just one ceramic cuboid and a metal aperture (Cai et al., 2022), double ceramic cuboids’ EET behavior has no specific frequency restriction but instead has a specified bandwidth. The mechanism behind this enhancement is examined together with local field distribution to achieve amplitude and frequency band modulation of EET behavior by varying coupling mode and strength between the two ceramic cuboids.
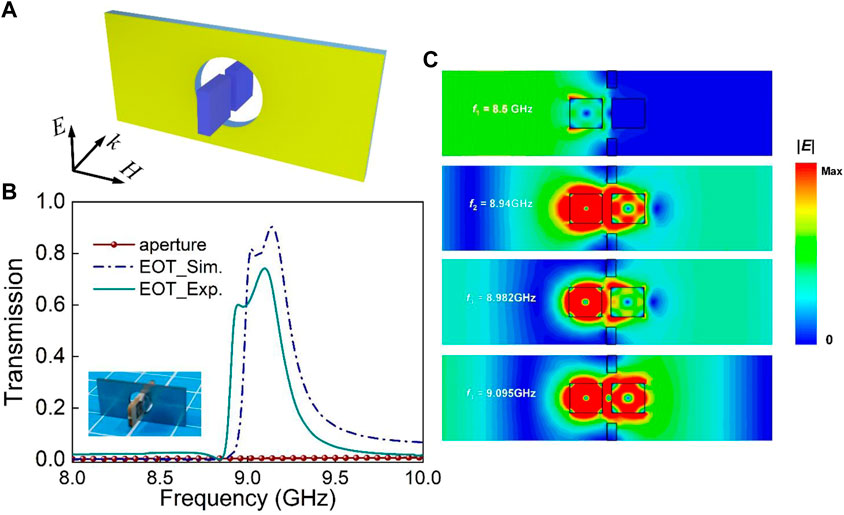
FIGURE 2. (A) Schematic diagram of the EET metamaterial with double cuboids placed in the center of a perforated metal sheet. (B) Transmission spectra of the metamaterial with only aperture and with double ceramic cuboids. (C) Calculated electric field distribution of EET metamaterial with double ceramic cuboids placed in the center of an aperture inside a waveguide at frequencies of 8.5, 8.94, 8.982, and 9.095 GHz.
Figure 2C displays the calculated local electric field distribution for four frequency points: 8.5, 8.94, 8.982, and 9.095 GHz. The reference pair is set at a frequency of 8.5 GHz since almost no energy can pass through the subwavelength aperture at 8.5 GHz. At frequencies 8.94 and 9.095 GHz, the resonant phase of both ceramic cuboids was similar, allowing energy to couple from one cuboid to another with improved transmission efficiency when interacting with the perforated metal sheet. At 8.982 GHz, the resonant phase of both ceramic cuboids was opposite but still allowed successful coupling and normal energy transmission as if there were only ceramic cuboids in front. In general, the mutual coupling of magnetic modes between ceramic cuboids can make the energy transfer from one ceramic cuboid to another, and the perforated metal sheet can effectively inhibit the energy transfer back. It can also be understood that when the coupling energy passes through the metal sheet again, the phase change of the electric field occurs so that the originally reflected electromagnetic wave is reflected port in 2 again. Therefore, the coupling behavior between two ceramic cuboids is the key to realizing EET behavior. Can we change the coupling strength between two ceramic cuboids or change the coupling mode between ceramics to modulate the realized EET behavior? Based on this idea, we continue the work in the following parts.
For wireless energy transmission using the magnetic mode, distance is the most critical factor affecting efficiency. Therefore, the relationship between the spacing (g) between two ceramic cuboids and coupling efficiency has been investigated. Figure 3 shows that there are two transmission peaks when g = 0.5 mm and g = 1 mm due to a mode generated by the coupling of two ceramic cuboids. This results in broadband transmission as we can observe this mode when the spacing is close. However, increasing the spacing to g = 1.5 mm suppresses this resonance due to a perforated metal plate, resulting in only one transmission peak at a frequency corresponding to the first-order Mie resonance of each ceramic cuboid (f = 9.045 GHz with a transmission rate of 0.62). Further increasing the spacing between ceramic cuboids leads to f = 9.07 GHz and a lower transmission rate of 0.15 for g = 2.5 mm. By changing the coupling between these two ceramic cuboids, we can modulate both the bandwidth and amplitude of transmissions from broadband (g = 0.5 mm, double transmission peak, 8.91–9.12 GHz) to a single band (9.045 GHz), and from an amplitude range of 0.835 to 0.15 by changing spacings from 0.5 to 2.5 mm, respectively.
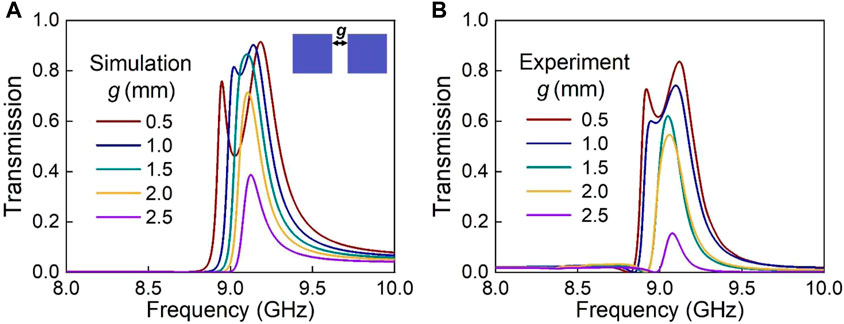
FIGURE 3. (A) Simulated and (B) measured transmission spectra with different spacing parameter g from 0.5 to 2.5 mm.
The transmission amplitude spectra of the sample with different in output power are measured through a Vector Network Analyzer (VNA) combined with a power amplifier. By adjusting the power of the incident electromagnetic field, we may control the transmission frequency by taking use of the thermal expansion effect. Figure 4 shows measured transmission spectra with various electromagnetic wave incident power P, and the insert shows the waveguide WR90 where the sample was located during the experiment. With P increasing from 5 to 30 dBm, the EET operating frequency shows a red shift of 60 MHz. Thanks to the Mie resonance inside the ceramic cuboid, the accompanying strong local field raises the internal temperature changes its dielectric constant, which is responsible for the red shift of the resonant frequency (Guo et al., 2016). In other words, as incident power increases, the temperature in the cuboid will increase accordingly, and the real and imaginary part of the permittivity of CaTiO3 will also increase, which leads to red shift and larger energy dissipation of the EET effect. The effect could be interpreted as a third-order Kerr non-linearity with the relative permittivity ceramic following the expression (Makarov et al., 2017):
where
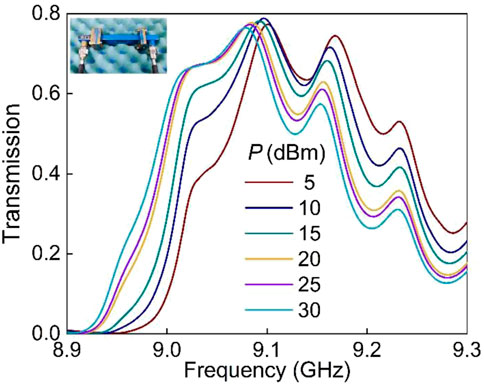
FIGURE 4. Measured transmission spectra with different input power levels P from 5 to 30 dBm, the insert shows the waveguide WR90 where the sample was located during the experiment.
The transmission of metamaterial is dependent on the power of the incident wave, which allows for a mechanism to achieve non-linearly tunable electromagnetic metamaterial (Fan et al., 2021). The measured results indicate that our metamaterials can be employed for a frequency-agile device with high transmittance.
3 Conclusion
In summary, we have theoretically and experimentally demonstrated the EET behavior by using the first-order magnetic mode Mie resonant coupling of two ceramic cuboids. Initially, we analyze the coupling behavior of two identical titanate (CaTiO3) doped with 1wt% ZrO2 cuboids at their resonant frequency. Subsequently, we position these cuboids on either side of a perforated metal sheet to leverage their local field coupling for achieving EET with a bandwidth exceeding 100 MHz. We then employ the spacing between these ceramic cuboids to modulate the strength of magnetic mode resonance coupling while controlling transmission amplitude and bandwidth. Finally, by exploiting non-linear effects in ceramic materials, we achieve EET frequency control above 60 MHz via incident power modulation. This study enhances our comprehension of light-matter interactions and provides valuable insights into research on tunable electromagnetic anomaly transmission microwave devices.
Data availability statement
The raw data supporting the conclusion of this article will be made available by the authors, without undue reservation.
Author contributions
YF and FZ conceived the idea and guided the research. WC designed the metasurface, characterized the samples, and wrote the manuscript. RY, SC, ZY, YZ, QF, LX, WZ, and QZ contributed to the final version of the manuscript. All authors contributed to the article and approved the submitted version.
Funding
The authors would like to acknowledge financial support from the National Key R&D Program of China (Grant Nos. 2022YFB3806002 and 2022YFB3806000), the Natural Science Foundation of China (NSFC) (Grants Nos. 12074314 and 61771402), and the Science and Technology New Star Program of Shaanxi Province (No. 2023KJXX-148).
Conflict of interest
The authors declare that the research was conducted in the absence of any commercial or financial relationships that could be construed as a potential conflict of interest.
Publisher’s note
All claims expressed in this article are solely those of the authors and do not necessarily represent those of their affiliated organizations, or those of the publisher, the editors and the reviewers. Any product that may be evaluated in this article, or claim that may be made by its manufacturer, is not guaranteed or endorsed by the publisher.
References
Aydin, K., Cakmak, A. O., Sahin, L., Li, Z., Bilotti, F., Vegni, L., et al. (2009). Split-ring-resonator-coupled enhanced transmission through a single subwavelength aperture. Phys. Rev. Lett. 102, 013904. doi:10.1103/PhysRevLett.102.013904
Bao, L., and Cui, T. J. (2020). Tunable, reconfigurable, and programmable metamaterials. Microw. Opt. Technol. Lett. 62, 9–32. doi:10.1002/mop.32164
Cai, W., Fan, Y., Fu, Q., Yang, R., Zhu, W., Zhang, Y., et al. (2022). Nonlinearly tunable extraordinary optical transmission in a hybird metamaterial. J. Phys. D. 55, 195106. doi:10.1088/1361-6463/ac5082
Chen, H., Chan, C. T., and Sheng, P. (2010). Transformation optics and metamaterials. Nat. Mat. 9, 387–396. doi:10.1038/nmat2743
Chen, W. C., Landy, N. I., Kempa, K., and Padilla, W. J. (2013). A subwavelength extraordinary-optical-transmission channel in babinet metamaterials. Adv. Opt. Mat. 1, 221–226. doi:10.1002/adom.201200016
Chen, X., Huang, L., Mühlenbernd, H., Li, G., Bai, B., Tan, Q., et al. (2012). Dual-polarity plasmonic metalens for visible light. Nat. Commun. 3, 1198. doi:10.1038/ncomms2207
Ebbesen, T. W., Lezec, H. J., Ghaemi, H. F., Thio, T., and Wolff, P. A. (1998). Extraordinary optical transmission through sub-wavelength hole arrays. Nature 391, 667–669. doi:10.1038/35570
Fan, Y., Han, J., Wei, Z., Wu, C., Cao, Y., Yu, X., et al. (2011). Subwavelength electromagnetic diode: One-way response of cascading nonlinear meta-atoms. Appl. Phys. Lett. 98, 151903. doi:10.1063/1.3579241
Fan, Y., He, X., Zhang, F., Cai, W., Li, C., Fu, Q., et al. (2021). Fano-resonant hybrid metamaterial for enhanced nonlinear tunability and hysteresis behavior. Research 2021, 9754083. doi:10.34133/2021/9754083
Freer, S., Camacho, M., Kuznetsov, S. A., Boix, R. R., Beruete, M., and Navarro-Cía, M. (2020). Revealing the underlying mechanisms behind TE extraordinary THz transmission. Photonics Res. 8, 430. doi:10.1364/prj.8.000430
García de Abajo, F. J. (2007). Colloquium: Light scattering by particle and hole arrays. Rev. Mod. Phys. 79, 1267–1290. doi:10.1103/RevModPhys.79.1267
Genet, C., and Ebbesen, T. W. (2007). Light in tiny holes. Nature 445, 39–46. doi:10.1038/nature05350
Guo, Y., Liang, H., Hou, X., Lv, X., Li, L., Li, J., et al. (2016). Thermally tunable enhanced transmission of microwaves through a subwavelength aperture by a dielectric metamaterial resonator. Appl. Phys. Lett. 108, 051906. doi:10.1063/1.4941406
Guo, Y., and Zhou, J. (2014). Total broadband transmission of microwaves through a subwavelength aperture by localized E-field coupling of split-ring resonators. Opt. Express. 22, 27136. doi:10.1364/OE.22.027136
Hajian, H., Caglayan, H., and Ozbay, E. (2017). Long-range Tamm surface plasmons supported by graphene-dielectric metamaterials. J. Appl. Phys. 121, 033101. doi:10.1063/1.4973900
Hajian, H., Ozbay, E., and Caglayan, H. (2017). Beaming and enhanced transmission through a subwavelength aperture via epsilon-near-zero media. Sci. Rep. 7, 4741. doi:10.1038/s41598-017-04680-y
Hao, F., Sonnefraud, Y., Van Dorpe, P., Maier, S. A., Halas, N. J., and Nordlander, P. (2008). Symmetry breaking in plasmonic nanocavities: Subradiant LSPR sensing and a tunable fano resonance. Nano Lett. 8, 3983–3988. doi:10.1021/nl802509r
He, C., and Song, Z. (2022). Terahertz graphene metasurfaces for cross-polarized deflection, focusing, and orbital angular momentum. Opt. Express. 30, 25498. doi:10.1364/OE.462330
He, Q., Sun, S., and Zhou, L. (2019). Tunable/reconfigurable metasurfaces: Physics and applications. Research 2019, 1849272. doi:10.34133/2019/1849272
Jahani, S., and Jacob, Z. (2016). All-dielectric metamaterials. Nat. Nanotechnol. 11, 23–36. doi:10.1038/nnano.2015.304
Jeong, J., Joushaghani, A., Paradis, S., Alain, D., and Poon, J. K. S. (2015). Electrically controllable extraordinary optical transmission in gold gratings on vanadium dioxide. Opt. Lett. 40, 4408. doi:10.1364/ol.40.004408
Jonah, O., and Georgakopoulos, S. V. (2013). Wireless power transfer in concrete via strongly coupled magnetic resonance. IEEE Trans. Antennas Propag. 61, 1378–1384. doi:10.1109/TAP.2012.2227924
Ju, L., Geng, B., Horng, J., Girit, C., Martin, M., Hao, Z., et al. (2011). Graphene plasmonics for tunable terahertz metamaterials. Nat. Nanotechnol. 6, 630–634. doi:10.1038/nnano.2011.146
Kurs, A., Karalis, A., Moffatt, R., Joannopoulos, J. D., Fisher, P., and Soljačić, M. (2007). Wireless power transfer via strongly coupled magnetic resonances. Science 317, 83–86. doi:10.1126/science.1143254
Li, C., and Song, Z. (2023). Tailoring terahertz wavefront with state switching in VO2 Pancharatnam-Berry metasurfaces. Opt. Laser Technol. 157, 108764. doi:10.1016/j.optlastec.2022.108764
Liu, N., Hentschel, M., Weiss, T., Alivisatos, A. P., and Giessen, H. (2011). Three-dimensional plasmon rulers. Science 332, 1407–1410. doi:10.1126/science.1199958
Liu, W., Xu, J., and Song, Z. (2021). Bifunctional terahertz modulator for beam steering and broadband absorption based on a hybrid structure of graphene and vanadium dioxide. Opt. Express. 29, 23331. doi:10.1364/OE.433364
Luo, T., Li, B., Zhao, Q., and Zhou, J. (2015). Dielectric behavior of low microwave loss unit cell for all dielectric metamaterial. Int. J. Antenn Propag. 2015, 1–6. doi:10.1155/2015/291234
Makarov, S. V., Zalogina, A. S., Tajik, M., Zuev, D. A., Rybin, M. V., Kuchmizhak, A. A., et al. (2017). Light-Induced tuning and reconfiguration of nanophotonic structures. Laser Photonics Rev. 11, 1700108. doi:10.1002/lpor.201700108
Martín-Moreno, L., García-Vidal, F. J., Lezec, H. J., Pellerin, K. M., Thio, T., Pendry, J. B., et al. (2001). Theory of extraordinary optical transmission through subwavelength hole arrays. Phys. Rev. Lett. 86, 1114–1117. doi:10.1103/PhysRevLett.86.1114
Navarro-Cía, M., Pacheco-Peña, V., Kuznetsov, S. A., and Beruete, M. (2018). Extraordinary THz transmission with a small beam spot: The leaky wave mechanism. Adv. Opt. Mat. 6, 1701312. doi:10.1002/adom.201701312
Nie, R., He, C., Zhang, R., and Song, Z. (2023). Vanadium dioxide-based terahertz metasurfaces for manipulating wavefronts with switchable polarization. Opt. Laser Technol. 159, 109010. doi:10.1016/j.optlastec.2022.109010
Pendry, J. B. (2000). Negative refraction makes a perfect lens. Phys. Rev. Lett. 85, 3966–3969. doi:10.1103/PhysRevLett.85.3966
Qin, Y., He, J., Yang, F., Zhang, Z., Yuan, Z., and Wu, M. (2018). Extraordinary optical transmission properties of a novel Bi-layered plasmonic nanostructure array. Optik 174, 684–691. doi:10.1016/j.ijleo.2018.08.073
Schurig, D., Mock, J. J., Justice, B. J., Cummer, S. A., Pendry, J. B., Starr, A. F., et al. (2006). Metamaterial electromagnetic cloak at microwave frequencies. Science 314, 977–980. doi:10.1126/science.1133628
Shelby, R. A., Smith, D. R., and Schultz, S. (2001). Experimental verification of a negative index of refraction. Science 292, 77–79. doi:10.1126/science.1058847
Smith, D. R., Pendry, J. B., and Wiltshire, M. C. K. (2004). Metamaterials and negative refractive Index. Science 305, 788–792. doi:10.1126/science.1096796
Soukoulis, C. M., and Wegener, M. (2011). Past achievements and future challenges in the development of three-dimensional photonic metamaterials. Nat. Photonics. 5, 523–530. doi:10.1038/nphoton.2011.154
Yang, R., Fan, Y., Zhu, W., Hu, C., Chen, S., Wei, H., et al. (2023). Terahertz silicon metagratings: High-efficiency dispersive beam manipulation above diffraction cone. Laser Photonics Rev. 2023, 2200975. doi:10.1002/lpor.202200975
Yang, R., Lou, J., Zhang, F., Zhu, W., Xu, J., Cai, T., et al. (2021). Active control of terahertz toroidal excitations in a hybrid metasurface with an electrically biased silicon layer. Adv. Photonics Res. 2, 2100103. doi:10.1002/adpr.202100103
Yang, R., Xu, J., Shen, N.-H., Zhang, F., Fu, Q., Li, J., et al. (2021). Subwavelength optical localization with toroidal excitations in plasmonic and Mie metamaterials. InfoMat 3, 577–597. doi:10.1002/inf2.12174
Yang, R., Zhang, F., Li, Z., Fu, Q., and Fan, Y. (2023). Controllable electromagnetically induced transparency in an electrically tunable terahertz hybrid metasurface. Opt. Laser Technol. 163, 109380. doi:10.1016/j.optlastec.2023.109380
Zhang, F., Kang, L., Zhao, Q., Zhou, J., and Lippens, D. (2012). Magnetic and electric coupling effects of dielectric metamaterial. New J. Phys. 14, 033031. doi:10.1088/1367-2630/14/3/033031
Zhao, Q., Kang, L., Du, B., Zhao, H., Xie, Q., Huang, X., et al. (2008). Experimental demonstration of Isotropic negative permeability in a three-dimensional dielectric composite. Phys. Rev. Lett. 101, 027402. doi:10.1103/PhysRevLett.101.027402
Zhao, Q., Zhou, J., Zhang, F., and Lippens, D. (2009). Mie resonance-based dielectric metamaterials. Mat. Today. 12, 60–69. doi:10.1016/S1369-7021(09)70318-9
Keywords: extraordinary optical transmission, non-linear, tunable metamaterials, Mie resonance, dielectric metamaterials
Citation: Cai W, Xuan L, Fan Y, Yang R, Zhang W, Zhang Q, Chen S, Ye Z, Zhang Y, Fu Q and Zhang F (2023) Coupled magnetic Mie resonances induced extraordinary optical transmission and its non-linear tunability. Front. Mater. 10:1207156. doi: 10.3389/fmats.2023.1207156
Received: 17 April 2023; Accepted: 24 May 2023;
Published: 05 June 2023.
Edited by:
Yadong Xu, Soochow University, ChinaReviewed by:
Zhengyong Song, Xiamen University, ChinaKe Chen, Nanjing University, China
Fei Sun, Taiyuan University of Technology, China
Copyright © 2023 Cai, Xuan, Fan, Yang, Zhang, Zhang, Chen, Ye, Zhang, Fu and Zhang. This is an open-access article distributed under the terms of the Creative Commons Attribution License (CC BY). The use, distribution or reproduction in other forums is permitted, provided the original author(s) and the copyright owner(s) are credited and that the original publication in this journal is cited, in accordance with accepted academic practice. No use, distribution or reproduction is permitted which does not comply with these terms.
*Correspondence: Yuancheng Fan, cGh5ZmFuQG53cHUuZWR1LmNu; Fuli Zhang, ZnVsaS56aGFuZ0Bud3B1LmVkdS5jbg==