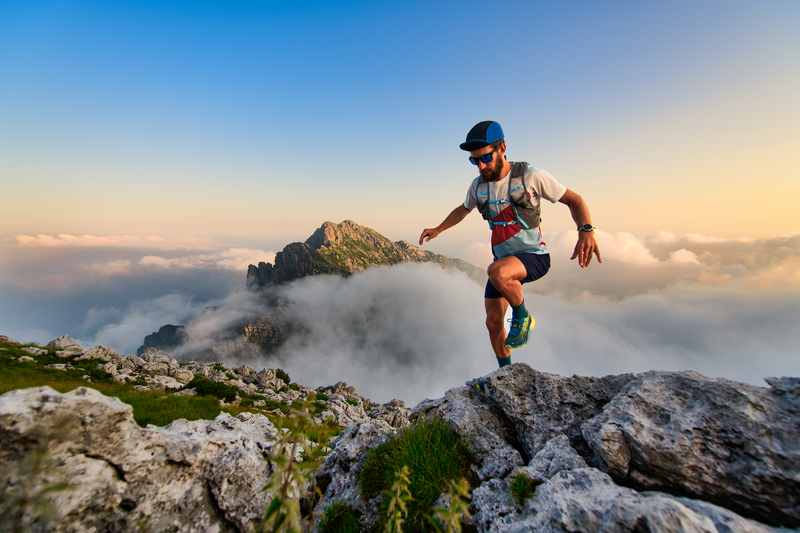
95% of researchers rate our articles as excellent or good
Learn more about the work of our research integrity team to safeguard the quality of each article we publish.
Find out more
REVIEW article
Front. Mater. , 31 July 2023
Sec. Biomaterials and Bio-Inspired Materials
Volume 10 - 2023 | https://doi.org/10.3389/fmats.2023.1204318
Tissue engineering represents a promising approach for impaired articular cartilage tissue regeneration. 3D printed hydrogels have become an emerging tissue engineering strategy because they closely mimic the physical and biochemical characteristics of the extracellular matrix. The formulation of hydrogel ink holds significant importance in attaining a precisely defined scaffold, which could exhibit excellent shape fidelity post-printing. Natural polysaccharide-based hydrogels are a highly promising class of scaffold biomaterials for articular cartilage regeneration in the field of material science and tissue engineering. These hydrogels are particularly advantageous due to their exceptional water absorption capacity, biodegradability, adjustable porosity, and biocompatibility, which closely resemble those of the natural extracellular matrix. This review aims to provide a comprehensive overview of the key characteristics, functions, and research progress in 3D printing technology for natural polysaccharide-based hydrogels. Specifically, this review categorizes the commonly used natural polysaccharide-based hydrogel materials in cartilage tissue engineering, and summarizes the classic literature in this area. In the end, we provide a comprehensive analysis of the challenges and potential applications of natural polysaccharide-based hydrogels in cartilage tissue engineering.
Cartilage, a remarkable viscoelastic connective tissue, undergoes formation during the embryonic phase of human development, preceding the initiation of bone formation (Camarero-Espinosa et al., 2016). In the realm of mammals, this resilient cartilaginous framework plays a crucial role as a blueprint for bone maturation, beginning in the embryonic stage and persisting throughout skeletal development in select regions of the body. Cartilage exhibits remarkable variations in its structural characteristics throughout its depth, showcasing divergent orientations of its constituents, unique compositions of the extracellular matrix (ECM), and intricate arrangements of chondrocytes. Moreover, chondrocytes residing in different zones exhibit distinct morphological features and selectively express markers that are inherently characteristic of each specific zone. The development of this stratified architecture occurs during maturation, arising from the interplay of externally applied and internally generated hydrostatic forces within the tissue. Consequently, the articular cartilage can be classified into distinct zones, namely: 1) the uppermost layer, often referred to as the superficial or tangential zone, 2) the intermediate or transitional zone, 3) the deep or radial zone, and 4) the calcified zone (Temenoff and Mikos, 2000; Williams et al., 2008). These zones have been extensively studied and characterized. Furthermore, articular cartilage exhibits a secondary microstructure that varies in relation to the radial distance from the chondrocytes. Hyaline cartilage is a crucial nonlinear, inhomogeneous, anisotropic, poro-viscoelastic connective tissue, which is usually referred to as articular cartilage. It plays a pivotal role as a friction-reducing and load-bearing cushion within synovial joints, facilitating smooth skeletal movements in mammal. Following the process of maturation, hyaline cartilage undergoes structural and compositional changes that establish it as a crucial component of articular joints, offering a remarkable interface with low friction while effectively supporting and transferring loads. The predominant constituents of hyaline cartilage include water (comprising approximately 70%–80% of its weight) that interacts synergistically with essential ECM components, such as proteoglycans, collagens, and other minor proteins and macromolecules. This intricate interplay of constituents contributes to the unique properties and functionality of hyaline cartilage.
The effective management of articular cartilage defects remains a significant and enduring clinical hurdle for orthopaedic surgeons. Articular cartilage is a highly specialized and structurally intricate tissue characterized by remarkable durability. However, owing to its avascular and aneural properties, it exhibits a limited intrinsic ability to self-repair (Setton et al., 1999; Jackson et al., 2001; Makris et al., 2015; Antich et al., 2020; Sang et al., 2023). Chronic joint pain and functional impairment are commonly observed in individuals who have experienced tissue damage due to either traumatic injury or degenerative pathology. Such conditions often give rise to a gradual deterioration of tissue, ultimately resulting in reduced joint function and mobility (Lories and Luyten, 2011). Failure to repair extensive focal chondral lesions and other cartilage injuries can result in the deterioration of the entire tissue, thus increasing an individual’s susceptibility to developing osteoarthritis (OA), a debilitating condition that is a major contributor to global disability (Hunter and Bierma-Zeinstra, 2019). Due to the simultaneous impact of population ageing, rising obesity rates, and an upsurge in joint injuries, the prevalence of this syndrome, which is already burdensome, is on the rise. Global estimates indicate that approximately 250 million individuals are currently affected by this condition. OA is a chronic condition that frequently manifests in individuals with untreated traumatic osteochondral lesions (Glyn-Jones et al., 2015). The manifestation of OA can be observed through various clinical indicators such as joint stiffness, pain, swelling, and restricted range of motion (Lespasio et al., 2017). Due to the limited availability of effective repair treatments, individuals with end-stage OA frequently undergo joint replacement procedures (Kloppenburg and Berenbaum, 2020). Thus, the timely management of articular cartilage lesions is imperative in order to mitigate or postpone the onset and progression of OA. The primary reparative approaches, namely microfracture, osteochondral transplantation, and autologous chondrocyte implantation, are associated with significant limitations, including the formation of fibrocartilage (Bae et al., 2006; Kreuz et al., 2006), limited donor sources, two operations required, etc. Consequently, it is imperative to expedite the development of a viable alternative approach to facilitate the regeneration of articular cartilage (Zhang et al., 2019).
Over the past few decades, substantial endeavors have been directed towards devising cartilage tissue engineering (CTE) methods as alternative therapeutic modalities to address the limitations of conventional clinical approaches. CTE techniques exhibit considerable promise for clinical implementation (Vinatier and Guicheux, 2016). CTE represents a highly encouraging avenue for restoring and rehabilitating articular cartilage, as it has the potential to stimulate tissue formation at the subchondral bone interface, thereby ameliorating the clinical manifestations of OA patients. Scaffolds constitute a fundamental constituent of CTE and exert a crucial impact on the regenerative potential of cartilage. Numerous scaffolding methodologies have been devised in the realm of tissue engineering (TE) (Ma et al., 1995; Mooney et al., 1996; Yang et al., 2002; Wu et al., 2006). The application of 3D bioprinting technology has emerged as a potent modality for fabricating scaffolds capable of providing cells with the necessary microenvironmental condition for TE (Murphy and Atala, 2014a; Patel et al., 2017). The hallmark of 3D bioprinting technologies is the generation of intricate structures through a process of layer-by-layer deposition, facilitated by computer-aided design (CAD). This method provides precise regulation over the shape and configuration of the scaffold, enabling the production of scaffolds with multiple layers, as well as the customized design of anatomically tailored implants (Lafuente-Merchan et al., 2022). The layer-by-layer deposition process facilitated by 3D bioprinting technology allows for the swift fabrication of scaffolds used in TE applications (Li et al., 2022). The material for deposition is commonly referred to as bio-ink, which comprises of cells and biomaterials. It has the potential to be supplemented with additional compounds such as medications, proteins, genetic matter, or growth factors (Ruiz-Alonso et al., 2021). These bio-inks have to meet certain requirements, such as biocompatility, biodegradability, printability, bioactivity and proper mechanical properties (Gungor-Ozkerim et al., 2018; Abdollahiyan et al., 2020). The mechanical properties of articular cartilage are mainly manifested in compressive properties, tensile and shear properties. The compressive aggregate modulus of articular cartilage in joint structures spans a range of 0.08–2 MPa, exhibiting depth-dependent variations within the tissue (Athanasiou et al., 1991; Schinagl et al., 1997). The Young’s modulus of articular cartilage displays zone-dependent variations within the tissue, with values ranging from 5 to 25 MPa. Notably, the superficial zone exhibits higher modulus values compared to the middle and deep zones (Kempson et al., 1968; Woo et al., 1979; Akizuki et al., 1986). Articular cartilage experiences shear stresses resulting from the translational and rotational movements of bones, relying predominantly on the solid phase of the tissue for support. Experimental measurements have determined the equilibrium shear modulus to range from 0.05 to 0.25 MPa. Moreover, calculations have indicated that the dynamic shear modulus varies from 0.1 to 4 MPa, while the loss angle is approximately 10° (Zhu et al., 1993; Wong and Sah, 2010).
In addition to the aforementioned requirements, it is essential for bioprinting ink demonstrate the capability to imitate the architecture and composition of the articular cartilage ECM to enable chondrogenic cell adhesion, migration, proliferation, and differentiation (Yang et al., 2017). Several investigations have demonstrated that hydrogel is regarded as a highly suitable material for addressing cartilage defects (Hunt et al., 2014; Xue et al., 2021). Hydrogels are polymer materials that exhibit water-swelling behavior and possess a 3D network structure. The formation of this structure is achieved through crosslinking reactions among hydrophilic polymers. Due to their ability to mimic the inner environment of the ECM, hydrogels have gained significant attention for their biomimetic properties (Grande et al., 1997; Chen et al., 2021). The excellent biocompatibility and biodegradability exhibited by hydrogels have made them a popular choice for the fabrication of in vitro tissues and organs (Correia et al., 2012; Gao et al., 2018). In addition, the hydrogel’s distinct properties of elevated moisture levels and porosity confer a significant advantage by creating a 3D crosslinking network that facilitates cellular retention, differentiation, migration, adhesion, and proliferation (Zhang et al., 2009). Hydrogels promote chondrocyte attachment similarly to the way cartilage ECM does, and unlike chondrocytes in other monolayer culture, chondrocytes embedded in hydrogels maintain their phenotype (Yamaoka et al., 2006; Aisenbrey and Bryant, 2016). The viscoelasticity of hydrogels allows transferring load effectively to chondrocytes, thereby ensuring their survival and controlling chondrogenic differentiation (Li J. et al., 2019a). Thus far, significant advancements have been made in the use of hydrogels and their composites for the repair of cartilage injuries, indicating promising prospects for potential clinical applications (Zhang et al., 2009).
Hydrogels can be classified as either natural or synthetic. Over the past several years, significant advancements have been made in the design of both types of hydrogels, with the specific goal of facilitating cartilage restoration. Such advancements involve the creation of 3D microenvironments that can effectively support the growth and proliferation of chondrocytes or stem cells (Bose et al., 2013). The natural hydrogel is primarily composed of proteins and polysaccharides derived from the ECM. This type of hydrogel exhibits properties that are similar to the ECM, including high water content, porosity, and softness (Gunatillake et al., 2003; Stoppel et al., 2015). The widespread usage of synthetic hydrogels in CTE can be attributed to several advantageous features they possess, which make them suitable for cartilage regeneration. These benefits include their ease of processing, high mechanical properties, and their ability to be controlled in terms of shape, porous structure, and degradation rate (Dang and Leong, 2006; Yao et al., 2015; Rao et al., 2018). Synthetic hydrogels possess certain properties that are unsuitable for the purpose of cartilage regeneration. These properties include inferior biocompatibility, low bioactivity, and the induction of aseptic inflammation due to the degradation products they release upon implantation into immunocompetent large animal models and human subjects. In light of recent research, synthetic hydrogels have been deemed suboptimal for the purpose of cartilage regeneration. In contrast, natural hydrogels are gaining favor as biomimetic scaffolds due to their exceptional biocompatibility, notable biological activity, minimal immunogenicity, and low cytotoxicity associated with their degradation byproducts (Diekjürgen and Grainger, 2017; Liu et al., 2017). Currently, natural hydrogel materials comprise polysaccharide-based hydrogels such as chitosan, hyaluronic acid, alginate, chondroitin sulfate and agarose, as well as protein-based hydrogels including gelatin, fibrin, elastin, silk fibroin, and collagen in addition to other materials derived from the extracellular matrix. Within the repertoire of biopolymers capable of constructing natural hydrogels, polysaccharides and their derivatives have gained increasing popularity for utilization in 3D bioprinting (Li N. et al., 2021a). Polysaccharides belong to the category of biopolymers that consist of monomeric units linked together via glycosidic bonds (Dai et al., 2019). Various polysaccharides, like chitosan, hyaluronic acid, alginate, chondroitin sulfate, and agarose have gained significant attention in the scientific community due to their wide availability, cost-effectiveness, and renewable nature. These polysaccharides have been extensively utilized in various applications, and their diverse physicochemical properties make them highly versatile and suitable for different uses (Teixeira et al., 2022). Polysaccharides are regarded as unique scaffold materials, owing to their beneficial characteristics such as biocompatibility, biodegradability, and customizable functionality, which position them among the most desirable choices for scaffold development. Moreover, these materials possess several appealing characteristics, such as facile derivatization/functionalization capabilities, a vast array of chemical structures with high diversity, and favorable rheological and mechanical properties (Oliveira and Reis, 2011). In addition, hydrogels based on natural polysaccharide-based hydrogels (NP-hydrogels) exhibit notable resemblances to the ECM of cartilage, thereby presenting them as a promising option for scaffold material in the context of CTE (Li P. et al., 2021b).
As previously discussed, 3D bioprinting offers a rapid means of manufacturing scaffolds used in CTE applications, utilizing a material known as bioprinting ink. Among the various options available in the realm of CTE, hydrogels have emerged as the most promising choice. Their injectability facilitates the delivery of stem cells, while their advantages in terms of minimally invasive surgery further bolster their appeal. As a result, hydrogels have found extensive application as bioprinting ink in the realm of 3D bioprinting. The procedure of 3D bioprinting can be typically classified into a tripartite process. Firstly, it involves acquiring relevant data pertaining to the characteristics of biological tissues or organs and creating 3D models through techniques such as CT and MRI. Subsequently, bioink must be prepared to facilitate tissue or organ repair. Lastly, 3D structures of natural tissues or organs are created through the application of a bio-printer (Heinrich et al., 2019). Categorized by their operational mechanisms, 3D bioprinting methodologies can be grouped into three types: extrusion-based, inkjet-based, and light-based 3D printing. Each of them has its own advantages and disadvantages (Table 1).
The application of 3D bioprinting technology enables the attainment of meticulous regulation over both the external shape and internal pore architecture of scaffolds (Jungst et al., 2016; Lai et al., 2019). Its capability to position cells and biomaterials with precision in a stratified manner enables the fabrication of constructs that possess controlled porosity, thus facilitating optimal diffusion of essential nutrients, oxygen, and metabolic waste products for the embedded cells (Murphy and Atala, 2014b; Turnbull et al., 2018). Furthermore, bioprinting methodologies enable the incorporation of elevated cell densities, which is unattainable by alternative techniques or necessitates the sequential inclusion of cells post-scaffold fabrication (Yang et al., 2022). Thus, 3D printing surpasses conventional techniques in the fields of TE. (Figure 1).
FIGURE 1. Diagram of articular cartilage regeneration using 3D printed hydrogel. Adapted with permission from Yang et al. (2022).
This article presents a comprehensive overview of the properties, roles, and recent advancements in NP-hydrogels and their composites for 3D printing of articular cartilage. The NP-hydrogel materials that are frequently utilized in CTE are systematically classified. We also present a detailed exposition of the benefits and limitations associated with each type of NP-hydrogel, with the aim of providing valuable guidance for the development of articular cartilage scaffolds. Finally, the article explores the existing obstacles and future prospects of NP-hydrogels in the context of CTE.
The principal NP-hydrogels employed in 3D printing for the regeneration of articular cartilage tissue comprise chitosan, hyaluronic acid, alginate, and chondroitin sulfate (Table 2).
Chitosan is a positively charged polysaccharide that is synthesized via alkaline N-deacetylation of chitin, a compound that is widely available in nature (Zhao et al., 2011). Sources of chitin encompass a broad range of materials, including crustacean shells, insect cuticles (particularly those derived from shrimps and crabs), and fungi cell walls (Martínez-Camacho et al., 2010). Chitosan is a natural linear polysaccharide composed of β-linked d-glucosamine residues, which may contain a variable number of randomly situated N-acetylglucosamine groups (Jeuken et al., 2016). Its structural similarity to glycosaminoglycans (GAGs) of the ECM has been noted, as well as its vital function in promoting cell-to-cell adhesion (Costa-Pinto et al., 2011; Levengood and Zhang, 2014; LogithKumar et al., 2016). Chitosan, owing to its chemical structural similarity with various GAGs such as those found in cartilage and meniscus, the predominant ECM molecules, imitates the native microenvironment for chondrocytes and meniscus cells, thus fostering chondrogenic activity and expression of cartilage-specific proteins (Chen and Cheng, 2009; Park et al., 2009; Neves et al., 2011). As a result, chitosan, with its advantageous characteristics of bioactivity, biocompatibility, and biodegradability, has emerged as a promising natural biomaterial scaffold for the repair of cartilage defects (Comblain et al., 2017). It has found wide application in TE, particularly for articular cartilage regeneration (Rodriguez-Vazquez et al., 2015; LogithKumar et al., 2016). Synovial mesenchymal stem cells (SMSCs), possessing exceptional chondrogenic potential and exhibiting a strong correlation with cartilage repair, are deemed optimal seed cells for articular cartilage tissue engineering. Li P. et al. (2021b) used chitosan hydrogel as a cell scaffold and improved the mechanical properties of the hydrogel by adding 3D printed PCL, while introducing the tetrahedral framework nucleic acid (TFNA) to improve the regenerative microenvironment, which can be absorbed into SMSCs and promote the proliferation and cartilage differentiation of SMSC. The scaffold synthesized demonstrates a Young’s modulus of up to 4.37 MPa, with the main contributor to its mechanical properties being PCL. Here, chitosan acts as a cationic polysaccharide that binds to DNA and recruits free TFNA by electrostatic interaction after intraarticular injection in vivo. The implementation of the entire system resulted in a deceleration of the long-term progression of osteoarthritis following the occurrence of articular cartilage defects, and developed a new strategy for cartilage regeneration. Chitosan-based cryogels are a promising scaffold for application in TE. Nonetheless, the crosslinked network generated in the hydrogel matrix can hinder the growth of ice crystals and impede the development of a cryogel with a macroporous structure at low temperatures. Consequently, the production of a cryogel scaffold through 3D printing has seldom been documented. Chen et al. (2022) used polyurethane nanoparticles as a crosslinker to react with chitosan, and then froze the product at −20°C to develop a 3D printable chitosan cryogel, which has injectability and shape-restoring properties that provide good mechanical integrity for the proliferation and cartilage formation differentiation of adult stem cells derived from human adipocy (hADSCs) (Figure 2). The synthesized bulk cryogel exhibits a compressive modulus of 5.8 kPa and a tensile strength of up to 295.6 kPa. The findings suggest that chitosan presents a new and efficient approach for articular cartilage repair. Nevertheless, the mechanical characteristics of chitosan are suboptimal (Shariatinia and Jalali, 2018; Kou et al., 2021), which constrains the application of chitosan hydrogel in the context of articular cartilage regeneration. Additionally, a related concern with respect to chitosan is the acidic milieu required for its dissolution, which may result in decreased cell viability (Gong et al., 2020). Notwithstanding, the chemical alteration of chitosan, facilitated by its numerous amine and hydroxyl groups, for instance carboxymethyl chitosan and hydroxybutyl chitosan, improves its water solubility. The mechanical characteristics of chitosan-based ink may be enhanced through several approaches, such as modifying the degree of deacetylation and molecular weight of chitosan, integrating synthetic polymers and bioceramics, and conducting post-treatment procedures on 3D printed chitosan constructs (Rajabi et al., 2021) These methods will improve the application value and significance of chitosan in articular cartilage tissue engineering.
FIGURE 2. Study design schematic diagram. Adapted with permission from Li P. et al. (2021b).
Hyaluronic Acid (HA) is a linear polysaccharide that exists naturally, comprising repetitive disaccharide units consisting of glucuronic acid and N-acetylglucosamine, and is extensively distributed in cartilage (Yue et al., 2015; Sun et al., 2018). It exhibits remarkable biocompatibility and biodegradability, while also inducing negligible immunogenicity (Mondal et al., 2016). HA, a constituent of the ECM, can engage with various chondrocyte surface receptors, leading to a beneficial impact on numerous cellular pathways, such as those responsible for chondrocyte proliferation, ECM secretion, and phenotype regulation (Chung and Burdick, 2009; Kim et al., 2011; Lebourg et al., 2013). In contrast to other polysaccharides, HA has the ability to regulate cartilage function and repair cartilage damage through various mechanisms. Prior research has established that HA has the potential to enhance the lubricity of cartilage boundaries, modulate inflammation at cartilage lesions, stimulate cell adhesion and proliferation, and improve cartilage ECM deposition and regeneration. These findings suggest that HA may be a promising avenue for application in the field of CTE (Ishida et al., 1997; Park et al., 2013; Lin W. F. et al., 2020a). HA-based hydrogels have been shown to have lubricating and buffering effects, which could restore the viscosity and elasticity of synovial fluid (Toh et al., 2010; Stellavato et al., 2019). More importantly, injectable HA-based hydrogels have shown promising results in repairing bone and cartilage defects of varying sizes via minimally invasive surgical procedures. This approach is advantageous due to the ability of the hydrogel to completely fill the defect site and provide a favorable environment for cell growth and tissue regeneration. Despite the attractive bioactive properties and high biocompatibility of HA, which have rendered it an appealing biomaterial in the field of CTE, its use in 3D extrusion-based bioprinting is limited due to its inadequate physical properties. One of the limitations of using HA in 3D bioprinting is the insufficient viscosity of its solutions, which can result in poor stability within the printing reservoir and hinder the homogeneous distribution of cells in the printed construct. The gelation properties of HA, which are crucial for preserving the 3D structure post-printing, are found to be insufficient. In order to achieve optimal mechanical properties for cartilage structures, Antich et al. (2020) have developed a unique bioink based on HA. By co-printing a combination of HA with alginate and polylactic acid (PLA), it has been observed that HA-based bioinks have the potential to enhance cell function through the upregulation of chondrogenic gene markers and the deposition of specific matrices, ultimately leading to the promotion of chondrogenesis. Compared to the standalone PLA scaffold, the addition of HA to the scaffold significantly enhances the compressive modulus. The authors suggest that this improvement in mechanical properties may be attributed to the colloid osmotic pressure and viscoelasticity properties of HA, which contribute to its load-bearing capability. HA-based hydrogels have been demonstrated to induce significant morphological differentiation of mesenchymal stem cells (MSCs) and exhibit a capacity for cartilage tissue reconstruction when utilized for MSCs delivery. In the context of implantation into injured joints, mesenchymal stem cells or chondrocytes delivered via hydrogels may encounter increased levels of reactive oxygen species (ROS) within the inflammatory microenvironment. This exposure has the potential to disrupt the cells’ phenotype and normal functions, ultimately impeding the efficacy of tissue regeneration. To mitigate the side effects induced by ROS during 3D bioprinting constructs and to promote cartilage tissue regeneration in the context of OA disease, Shi et al. (2022) have developed a multifunctional hydrogel, which is created through a dynamic covalent bond between phenylboronic acid grafted hyaluronic acid (HA-PBA) and poly(vinyl alcohol). In addition, a secondary crosslinking mechanism has been employed between the acrylate moiety on HA-PBA and the free thiol group from thiolated gelatin, resulting in enhanced stability of the hydrogel (Figure 3). The multifunctional hydrogel described above has been proposed as a viable bioink for the creation of 3D bioprinted constructs with anti-ROS properties, which could promote the regeneration of cartilage tissue within an elevated ROS and chronic inflammatory microenvironment. The use of bioinks containing high concentrations of polymeric materials is a common practice in 3D bioprinting to facilitate the fabrication of stable 3D cell-hydrogel constructs. However, this approach may result in limited cell bioactivity and an uneven distribution of newly synthesized ECM. Hauptstein et al. (2020) crosslinked thiolated HA and allyl modified polyglycidyl by ultraviolet light and added unmodified high molecular weight HA to accommodate PCL-enabled 3D bioprinting, and unexpectedly found that the distribution of cartilage ECM in low-polymer content bioink was greatly improved by supplementing high molecular weight HA. During the initial preparation day, the Young’s modulus of 3wt% gels was approximately 0.3 kPa, whereas the Young’s modulus of 10wt% gels measured 10.9 kPa. After 21 days of cartilage differentiation, the Young’s modulus of 10% gels increased to 28 kPa, while the 3 wt% gels exhibited significantly higher stiffness with values of 36.9 kPa for 3 wt% -hmHA and 45.4 kPa for 3 wt% +hmHA gels. According to the authors, this finding emphasizes the importance of a homogeneous distribution of the ECM in achieving higher construct stiffness. These structures combine PCL-enabled 3D printability with uniform ECM distribution and increased stiffness after cartilage differentiation and thus represent the promise of cartilage regeneration.
FIGURE 3. Diagrammatic representation of the production process for a dynamic hydrogel made from HA and gelatin that are covalently linked. (A) The synthesis strategy of the functional group HA-PBA-Ac. (B) Diagrammatic illustration depicting the creation of a gelatin-crosslinked dynamic HA hydrogel and (C) outline of the experimental design used in the bioprinting study. Adapted with permission from Shi et al. (2022).
Alginate is a hydrophilic polysaccharide of natural origin, characterized by a negative charge (Lee and Mooney, 2012). It is composed of linear, unbranched copolymers consisting of varying proportions of (1-4)-linked b-D-mannuronic acid M) and a-L-guluronic acid G) monomers, which are linked together through covalent bonds. The copolymer structure is defined by the arrangement of consecutive G sequences, consecutive M sequences, and alternating MG sequences, with the physical properties of alginate being influenced by the copolymer composition, sequence formula, and overall length of the linear chain (Augst et al., 2006; Bidarra et al., 2014). Alginate has gained considerable attention as a biocompatible material due to its high water content, good porosity, and adjustable viscosity. It is known to readily form hydrogels, which can be utilized as scaffolds for loading both cells and drugs (Maity and Das, 2021). Due to their biocompatibility and cost-effectiveness, natural alginate hydrogels have become a popular choice in the field of TE (Cao et al., 2023). Despite its advantages, the clinical utility of alginate can be limited by its suboptimal mechanical properties. Kosik-Koziol et al. (2017) developed a novel approach to enhance the mechanical properties of 3D-printed hydrogel constructs for CTE by incorporating short submicron polylactide (PLA) fibers into composite bioinks containing alginate. Incorporating PLA short fibers into alginate constructs resulted in a threefold increase in Young’s modulus compared to pristine alginate constructs(from 6.9 to 25.1 kPa). In addition, the incorporation of short sub-micron PLA fibers into alginate hydrogels not only enhanced mechanical properties, but also improved cell viability compared to hydrogels composed solely of alginate. The latter were observed to undergo partial leaching after 14 days of incubation. Remarkably, the chondrocytes retained their rounded morphology, implying that the fiber-reinforced hydrogel system represents a promising substrate for chondrocyte encapsulation and the mechanical support of tissue structures. Given the limitations of traditional approaches, growth factor therapy has emerged as an attractive alternative for promoting the regeneration of functional cartilage (Quintana et al., 2009). Currently, several approaches have been devised to regulate the delivery of growth factors in a temporal and spatial manner to modulate the differentiation of MSCs. Nevertheless, apprehensions regarding the initial burst releases and changes to the bioactivity of growth factors still persist (Freeman and Kelly, 2017; Chen et al., 2018; Caballero Aguilar et al., 2019; Gonzalez-Fernandez et al., 2019; Peak et al., 2019). Wang et al. (2021) have introduced a new class of bioink consisting of alginate sulfate functionalized, growth factor eluting, alginate-gelatin methacryloyl (GelMA) interpenetrating networks (Figure 4). This bioink is specifically designed to facilitate chondrogenesis of encapsulated MSCs, while providing suitable mechanical properties for the regeneration of articular cartilage. The authors have successfully synthesized dual crosslinked S-IPN constructs, exhibiting a remarkable compression modulus of approximately 32.48 kPa. This value is significantly higher than the sum of the individual components, suggesting a synergistic effect of dual crosslinking in enhancing construct stiffness. The incorporation of alginate sulfate into the GelMA interpenetrating network bioink facilitated effective and continuous delivery of growth factor, resulting in enhanced chondrogenesis and inhibition of hypertrophy of encapsulated MSCs both in vitro and in vivo. Alginate is a biocompatible and natural polymer that has been widely utilized in the 3D printing of bone and cartilage. Due to its non-animal derived origin, it is a favorable material for hydrogel ink in terms of biocompatibility. Although notable advances have been achieved in the realm of 3D bioprinting of alginate for orthopedic purposes, there are still a number of questions to be addressed, such as bad mechanical properties, short of long-term stability, the absence of functional moieties that can improve cell adhesion and proliferation, etc. (Murab et al., 2022)
FIGURE 4. Diagram illustrates the steps involved in preparing and printing the sulfated interpenetrate network bioink. (A) The process of creating a bioink for alginate/alginate sulfate-GelMA IPN involves the addition of the growth factor TGF-β3 to the mixture of alginate/alginate sulfate and GelMA solution, which is then combined with porcine MSCs after the growth factor has been bound to the alginate/alginate sulfate. (B) The picture shows 3D bioprinted structures. (C) A diagram illustrating the crosslinking procedures, wherein cylinders were printed and exposed to UV for 15 min and then subjected to ionic crosslinking in a calcium bath for an additional 15 min. The resulting constructs were either cultured in vitro for 6 weeks or implanted subcutaneously for 4 weeks. Adapted with permission from Wang et al. (2021).
The articular cartilage, being a vital component of the musculoskeletal system, is composed of various biomolecules, of which chondroitin sulfate plays a crucial role as the predominant GAGs. Chondroitin sulfate (CS) is a crucial element of the cartilage ECM, constituting over 80% of the GAGs present. The presence of CS imparts the articular cartilage with essential physiological functions that are imperative for the smooth functioning of the joint (Lin T. S. et al., 2020b). CS in the cartilage ECM plays a crucial role in providing mechanical support and imparting the necessary viscoelastic properties to the tissue. The composition of CS varies across different species and age groups. In the context of therapeutic intervention for joint-related pathologies, CS has been employed in combination with glucosamine to alleviate pain and facilitate the restoration of cartilage, thus addressing the underlying causes of joint dysfunction. This synergistic approach holds promise for ameliorating joint afflictions and enhancing overall joint health (Li et al., 2016). In its native state, CS primarily occurs as a component of aggrecan, a natural polymer that plays a crucial role in several biological processes that contribute to the maintenance of cartilage and its capacity to resist compressive forces. CS’s hydrophilic properties and abundance of negatively charged residues enable it to facilitate the retention of a significant volume of water within the ECM. Under compression, some of this water is released, only to be reabsorbed when the load is removed (Roughley and Mort, 2014). In addition to its mechanical role in providing resistance, the mechanism of water retention mediated by CS also facilitates the exchange of nutrients and waste products, thereby contributing to the proper function and performance of the chondrocytes that are embedded within the matrix. CS is implicated in a range of mechanical and biological processes associated with cartilage function, including resistance to compressive forces, as well as the absorption of water and nutrients. Moreover, CS exerts a regulatory effect on chondrocyte metabolism at the cellular level (Monfort et al., 2008; Aisenbrey and Bryant, 2019). It sustains the structural integrity of cartilage and promotes the restoration of joint function in arthritic conditions by virtue of several biological properties. These properties include the capacity to modulate inflammatory responses (Corradetti et al., 2016), preserve the stem cell niche (Dyck et al., 2015) and regulate enzymatic activities involved in cartilage homeostasis (Sage et al., 2013). In addition, CS is a significant constituent of the ECM in mineralized tissues. CS plays a pivotal role in regulating bone remodeling within the intrabony microenvironment by modulating the differentiation of osteoclasts and osteoblasts (Salbach et al., 2012). Thus, CS-based scaffolds have garnered significant attention in the field of CTE (Wang et al., 2007; Varghese et al., 2008; Sharma et al., 2013). To generate a biocompatible cell-carrying hydrogel with a modifiable structure. Li C. D. et al., 2019b) fabricated a shape-controllable bionic hydrogel composed of water-soluble HBC and oxidized CS (OCS). The Schiff base reaction was employed to covalently crosslink the materials, thereby improving their mechanical properties (Figure 5). In vitro and in vivo experiments demonstrate the viability of culturing hMSCs in the HBC/OCS hydrogel, with the cells retaining high levels of activity. As a result, this biomimetic hydrogel, which is both shape-controllable and cell-laden, holds great promise for utilization in the realm of articular cartilage tissue engineering. Costantini et al. (2016) employed GelMA, CS aminoethyl methacrylate (CS-AEMA), and methacrylic acid hyaluronic acid (HAMA) as the main components for the fabrication of a cartilage scaffold using 3D printing. In his study, a 3D biomimetic hydrogel scaffold was constructed for the purposes of CTE. The scaffold was designed to achieve high cell density, with a concentration exceeding 107cells/mL, as well as a high cell survival rate, which was observed to be greater than 85%–90%. Additionally, the scaffold exhibited a high printing resolution, reaching approximately 100 μm. Following a 3-week culture period, it was observed that the scaffold promoted the differentiation of BM-MSCs into chondrocytes. As for the mechanical performance of the scaffold, its compressive modulus can reach approximately 100.1 kPa. Notwithstanding the potential benefits of employing CS hydrogel systems in CTE, extant research indicates that such systems are encumbered by certain limitations that impede their successful implementation. Specifically, their degradation kinetics are deemed unsuitable, their mechanical properties are inadequate in mimicking native cartilage tissue, and they demonstrate a limited capacity for integration with the host tissue (Shin et al., 2021).
FIGURE 5. The process of fabricating hydrogel implants made of HBC/OCS materials. (A) Images depicting the injectability of pre-crosslinked hydrogels composed of 20HBC-3OCS and 40HBC-3OCS HBC/OCS. (B) printed sacrificial molds (C) Hydrogel implants with different shapes made of HBC/OCS. (D) Implants made of self-crosslinked HBC/OCS hydrogel with diverse shapes and constituents. Adapted with permission from Li C. D. et al. (2019b).
This article begins with a succinct overview of the merits and limitations of widely used 3D printing methods. Subsequently, it presents a comprehensive summary of hydrogels produced through 3D printing with natural polysaccharides, delineating their distinctive features. Moreover, select prototypical instances are singled out to generate curiosity and enhance readers’ cognizance regarding the superior potential of NP-hydrogels in the field of CTE. In the presented examples, we provide a comprehensive overview of the diverse cell types employed in cartilage tissue engineering. It is worth noting that among these, MSCs derived from bone marrow or umbilical cord have emerged as the most promising candidates for advancing CTE applications. The intrinsic lack of vascularization, innervation, and inadequate chondrocyte differentiation in natural cartilage renders the tissue incapable of proficient self-restoration. However, the advent of bioprinting techniques has revolutionized TE by enabling the construction of customized artificial tissues that replicate the physiological characteristics of native tissues with precision. In comparison to traditional methodologies, 3D bioprinting provides several benefits, including the ability to incorporate intended micro/nanostructures into scaffolds at the intended location, efficient fabrication at a high throughput, and the capacity for achieving exceptional spatiotemporal resolution. The selection of a suitable bioink material assumes a critical role in the precise fabrication of 3D printed scaffolds designed for orthopedic applications. In light of this, hydrogels derived from natural polysaccharides exhibit tunable chemical properties, desirable processability, satisfactory cellular biocompatibility, biodegradability, low cytotoxicity, and an inherent structural similarity to the ECM of native cartilage. As a result, they have garnered considerable attention in the development of scaffolds for CTE. Nonetheless, the mechanical attributes of such hydrogels fall short of matching the requisite properties of native cartilage, thereby constraining their clinical utilization. By blending natural and synthetic hydrogels, it is feasible to harness the superior mechanical characteristics of the latter alongside the desirable biocompatibility of the former, thereby achieving an optimal composite hydrogel for diverse biomedical applications. Hence, the integration of natural and synthetic biomaterials utilizing state-of-the-art fabrication methodologies continues to constitute a prominent avenue for the development of in vitro cartilage constructs. However, it should be acknowledged that achieving a complete restoration of cartilage to its native composition, architecture, mechanics, and biofunctionality remains a formidable obstacle. Despite considerable advancements in the field of 3D bioprinting of natural polysaccharides for articular cartilage applications, successful clinical implementation remains a significant challenge. Enhancing the rheological and mechanical characteristics of natural polysaccharide-based inks and scaffolds represents a crucial area of focus to address this issue. Further efforts are necessary to improve the translational potential of this technology for clinical use.
Conceptualization, JZ and YQ; writing, XW and XC; investigation, MK and RD; supervision, JZ and YQ; funding acquisition, RD and YQ. All authors contributed to the article and approved the submitted version.
The work was funded by the Rural and Social Development Branch, Changchun Science and Technology Bureau, grant number 21ZGY23 and Jilin Health Science and Technology Capability Improvement Project, grant number 2022C107.
The authors declare that the research was conducted in the absence of any commercial or financial relationships that could be construed as a potential conflict of interest.
All claims expressed in this article are solely those of the authors and do not necessarily represent those of their affiliated organizations, or those of the publisher, the editors and the reviewers. Any product that may be evaluated in this article, or claim that may be made by its manufacturer, is not guaranteed or endorsed by the publisher.
Abdollahiyan, P., Oroojalian, F., Mokhtarzadeh, A., and de la Guardia, M. (2020). Hydrogel-based 3D bioprinting for bone and cartilage tissue engineering. Biotechnol. J. 15 (12), e2000095. doi:10.1002/biot.202000095
Aisenbrey, E. A., and Bryant, S. J. (2019). The role of chondroitin sulfate in regulating hypertrophy during MSC chondrogenesis in a cartilage mimetic hydrogel under dynamic loading. Biomaterials 190, 51–62. doi:10.1016/j.biomaterials.2018.10.028
Aisenbrey, E., and Bryant, S. (2016). Mechanical loading inhibits hypertrophy in chondrogenically differentiating hMSCs within a biomimetic hydrogel. J. Mater. Chem. B 4 (20), 3562–3574. doi:10.1039/c6tb00006a
Akizuki, S., Mow, V. C., Muller, F., Pita, J. C., Howell, D. S., and Manicourt, D. H. (1986). Tensile properties of human knee joint cartilage: I. Influence of ionic conditions, weight bearing, and fibrillation on the tensile modulus. J. Orthop. Res. 4 (4), 379–392. doi:10.1002/jor.1100040401
Ali, M. A., Rajabi, M., and Sali, S. S. (2020). Additive manufacturing potential for medical devices and technology. Curr. Opin. Chem. Eng. 28, 127–133. doi:10.1016/j.coche.2020.05.001
Antich, C., de Vicente, J., Jimenez, G., Chocarro, C., Carrillo, E., Montanez, E., et al. (2020). Bio-inspired hydrogel composed of hyaluronic acid and alginate as a potential bioink for 3D bioprinting of articular cartilage engineering constructs. Acta Biomater. 106, 114–123. doi:10.1016/j.actbio.2020.01.046
Athanasiou, K. A., Rosenwasser, M. P., Buckwalter, J. A., Malinin, T. I., and Mow, V. C. (1991). Interspecies comparisons of in situ intrinsic mechanical properties of distal femoral cartilage. J. Orthop. Res. 9 (3), 330–340. doi:10.1002/jor.1100090304
Augst, A. D., Kong, H. J., and Mooney, D. J. (2006). Alginate hydrogels as biomaterials. Macromol. Biosci. 6 (8), 623–633. doi:10.1002/mabi.200600069
Bae, D. K., Yoon, K. H., and Song, S. J. (2006). Cartilage healing after microfracture in osteoarthritic knees. Arthroscopy 22 (4), 367–374. doi:10.1016/j.arthro.2006.01.015
Bidarra, S. J., Barrias, C. C., and Granja, P. L. (2014). Injectable alginate hydrogels for cell delivery in tissue engineering. Acta Biomater. 10 (4), 1646–1662. doi:10.1016/j.actbio.2013.12.006
Bose, S., Vahabzadeh, S., and Bandyopadhyay, A. (2013). Bone tissue engineering using 3D printing. Mater Today 16 (12), 496–504. doi:10.1016/j.mattod.2013.11.017
Caballero Aguilar, L. M., Silva, S. M., and Moulton, S. E. (2019). Growth factor delivery: Defining the next generation platforms for tissue engineering. J. Control Release 306, 40–58. doi:10.1016/j.jconrel.2019.05.028
Camarero-Espinosa, S., Rothen-Rutishauser, B., Foster, E. J., and Weder, C. (2016). Articular cartilage: From formation to tissue engineering. Biomater. Sci. 4 (5), 734–767. doi:10.1039/c6bm00068a
Cao, Y., Cong, H., Yu, B., and Shen, Y. (2023). A review on the synthesis and development of alginate hydrogels for wound therapy. J. Mater Chem. B 11, 2801–2829. doi:10.1039/d2tb02808e
Chen, J. P., and Cheng, T. H. (2009). Preparation and evaluation of thermo-reversible copolymer hydrogels containing chitosan and hyaluronic acid as injectable cell carriers. Polymer 50 (1), 107–116. doi:10.1016/j.polymer.2008.10.045
Chen, R., Yu, Y., Zhang, W., Pan, Y., Wang, J., Xiao, Y., et al. (2018). Tuning the bioactivity of bone morphogenetic protein-2 with surface immobilization strategies. Acta Biomater. 80, 108–120. doi:10.1016/j.actbio.2018.09.011
Chen, T. C., Wong, C. W., and Hsu, S. H. (2022). Three-dimensional printing of chitosan cryogel as injectable and shape recoverable scaffolds. Carbohydr. Polym. 285, 119228. doi:10.1016/j.carbpol.2022.119228
Chen, Y., Udduttula, A., Xie, X., Zhou, M., Sheng, W., Yu, F., et al. (2021). A novel photocrosslinked phosphate functionalized Chitosan-Sr5 (PO4) 2SiO4 composite hydrogels and in vitro biomineralization, osteogenesis, angiogenesis for bone regeneration application. Compos. Part B Eng. 222, 109057. doi:10.1016/j.compositesb.2021.109057
Cho, Y. H., Lee, I. H., and Cho, D. W. (2005). Laser scanning path generation considering photopolymer solidification in micro-stereolithography. Microsyst. Technol. 11 (2-3), 158–167. doi:10.1007/s00542-004-0468-2
Chohan, J. S., Singh, R., Boparai, K. S., Penna, R., and Fraternali, F. (2017). Dimensional accuracy analysis of coupled fused deposition modeling and vapour smoothing operations for biomedical applications. Compos Part B-Eng 117, 138–149. doi:10.1016/j.compositesb.2017.02.045
Chung, C., and Burdick, J. A. (2009). Influence of three-dimensional hyaluronic acid microenvironments on mesenchymal stem cell chondrogenesis. Tissue Eng. Part A 15 (2), 243–254. doi:10.1089/ten.tea.2008.0067
Comblain, F., Rocasalbas, G., Gauthier, S., and Henrotin, Y. (2017). Chitosan: A promising polymer for cartilage repair and viscosupplementation. Biomed. Mater Eng. 28 (s1), S209–S215. doi:10.3233/bme-171643
Corradetti, B., Taraballi, F., Minardi, S., Van Eps, J., Cabrera, F., Francis, L. W., et al. (2016). Chondroitin sulfate immobilized on a biomimetic scaffold modulates inflammation while driving chondrogenesis. Stem Cells Transl. Med. 5 (5), 670–682. doi:10.5966/sctm.2015-0233
Correia, C., Pereira, A. L., Duarte, A. R., Frias, A. M., Pedro, A. J., Oliveira, J. T., et al. (2012). Dynamic culturing of cartilage tissue: The significance of hydrostatic pressure. Tissue Eng. Pt A 18 (19-20), 1979–1991. doi:10.1089/ten.tea.2012.0083
Costa-Pinto, A. R., Reis, R. L., and Neves, N. M. (2011). Scaffolds based bone tissue engineering: The role of chitosan. Tissue Eng. Part B Rev. 17 (5), 331–347. doi:10.1089/ten.teb.2010.0704
Costantini, M., Idaszek, J., Szoke, K., Jaroszewicz, J., Dentini, M., Barbetta, A., et al. (2016). 3D bioprinting of BM-MSCs-loaded ECM biomimetic hydrogels for in vitro neocartilage formation. Biofabrication 8 (3), 035002. doi:10.1088/1758-5090/8/3/035002
Dababneh, A. B., and Ozbolat, I. T.Bioprinting Technology (2014). Bioprinting technology: A current state-of-the-art review. J. Manuf. Sci. E-T Asme 136 (6). doi:10.1115/1.4028512
Dai, L., Cheng, T., Duan, C., Zhao, W., Zhang, W., Zou, X., et al. (2019). 3D printing using plant-derived cellulose and its derivatives: A review. Carbohyd Polym. 203, 71–86. doi:10.1016/j.carbpol.2018.09.027
Dang, J. M., and Leong, K. W. (2006). Natural polymers for gene delivery and tissue engineering. Adv. drug Deliv. Rev. 58 (4), 487–499. doi:10.1016/j.addr.2006.03.001
Diekjürgen, D., and Grainger, D. W. (2017). Polysaccharide matrices used in 3D in vitro cell culture systems. Biomaterials 141, 96–115. doi:10.1016/j.biomaterials.2017.06.020
Dyck, S. M., Alizadeh, A., Santhosh, K. T., Proulx, E. H., Wu, C. L., and Karimi-Abdolrezaee, S. (2015). Chondroitin sulfate proteoglycans negatively modulate spinal cord neural precursor cells by signaling through LAR and RPTPσ and modulation of the rho/ROCK pathway. Stem Cells 33 (8), 2550–2563. doi:10.1002/stem.1979
Freeman, F. E., and Kelly, D. J. (2017). Tuning alginate bioink stiffness and composition for controlled growth factor delivery and to spatially direct MSC fate within bioprinted tissues. Sci. Rep-Uk 7, 17042. doi:10.1038/s41598-017-17286-1
Gao, F., Xu, Z., Liang, Q., Liu, B., Li, H., Wu, Y., et al. (2018). Direct 3D printing of high strength biohybrid gradient hydrogel scaffolds for efficient repair of osteochondral defect. Adv. Funct. Mater. 28 (13), 1706644. doi:10.1002/adfm.201706644
Glyn-Jones, S., Palmer, A. J., Agricola, R., Price, A. J., Vincent, T. L., Weinans, H., et al. (2015). Osteoarthritis. Lancet 386 (9991), 376–387. doi:10.1016/s0140-6736(14)60802-3
Gong, J., Schuurmans, C. C. L., Genderen, A. M. V., Cao, X., Li, W., Cheng, F., et al. (2020). Complexation-induced resolution enhancement of 3D-printed hydrogel constructs. Nat. Commun. 11 (1), 1267. doi:10.1038/s41467-020-14997-4
Gonzalez-Fernandez, T., Rathan, S., Hobbs, C., Pitacco, P., Freeman, F. E., Cunniffe, G. M., et al. (2019). Pore-forming bioinks to enable spatio-temporally defined gene delivery in bioprinted tissues. J. Control Release 301, 13–27. doi:10.1016/j.jconrel.2019.03.006
Grande, D. A., Halberstadt, C., Naughton, G., Schwartz, R., and Manji, R. (1997). Evaluation of matrix scaffolds for tissue engineering of articular cartilage grafts. J. Biomed. Mater Res. 34 (2), 211–220. doi:10.1002/(sici)1097-4636(199702)34:2<211::aid-jbm10>3.0.co;2-l
Gunatillake, P. A., Adhikari, R., and Gadegaard, N. (2003). Biodegradable synthetic polymers for tissue engineering. Eur. Cell Mater 5 (1), 1–16. doi:10.22203/ecm.v005a01
Gungor-Ozkerim, P. S., Inci, I., Zhang, Y. S., Khademhosseini, A., and Dokmeci, M. R. (2018). Bioinks for 3D bioprinting: An overview. Biomater. Sci. 6 (5), 915–946. doi:10.1039/c7bm00765e
Hauptstein, J., Bock, T., Bartolf-Kopp, M., Forster, L., Stahlhut, P., Nadernezhad, A., et al. (2020). Hyaluronic acid-based bioink composition enabling 3D bioprinting and improving quality of deposited cartilaginous extracellular matrix. Adv. Healthc. Mater 9 (15), e2000737. doi:10.1002/adhm.202000737
Heinrich, M. A., Liu, W., Jimenez, A., Yang, J., Akpek, A., Liu, X., et al. (2019). 3D bioprinting: From benches to translational applications. Small 15 (23), 1805510. doi:10.1002/smll.201805510
Hunt, J. A., Chen, R., van Veen, T., and Bryan, N. (2014). Hydrogels for tissue engineering and regenerative medicine. J. Mater Chem. B 2 (33), 5319–5338. doi:10.1039/c4tb00775a
Hunter, D. J., and Bierma-Zeinstra, S. (2019). Osteoarthritis. Lancet 393 (10182), 1745–1759. doi:10.1016/s0140-6736(19)30417-9
Ishida, O., Tanaka, Y., Morimoto, I., Takigawa, M., and Eto, S. (1997). Chondrocytes are regulated by cellular adhesion through CD44 and hyaluronic acid pathway. J. Bone Min. Res. 12 (10), 1657–1663. doi:10.1359/jbmr.1997.12.10.1657
Jackson, D. W., Scheer, M. J., and Simon, T. M. (2001). Cartilage substitutes: Overview of basic science and treatment options. J. Am. Acad. Orthop. Surg. 9 (1), 37–52. doi:10.5435/00124635-200101000-00005
Jeuken, R., Roth, A., Peters, R., Van Donkelaar, C., Thies, J., Van Rhijn, L., et al. (2016). Polymers in cartilage defect repair of the knee: Current status and future prospects. Polymers 8 (6), 219. doi:10.3390/polym8060219
Jungst, T., Smolan, W., Schacht, K., Scheibel, T., and Groll, J. r. (2016). Strategies and molecular design criteria for 3D printable hydrogels. Chem. Rev. 116 (3), 1496–1539. doi:10.1021/acs.chemrev.5b00303
Kempson, G. E., Freeman, M. A., and Swanson, S. A. (1968). Tensile properties of articular cartilage. Nature 220 (5172), 1127–1128. doi:10.1038/2201127b0
Kim, I. L., Mauck, R. L., and Burdick, J. A. (2011). Hydrogel design for cartilage tissue engineering: A case study with hyaluronic acid. Biomaterials 32 (34), 8771–8782. doi:10.1016/j.biomaterials.2011.08.073
Kim, J. D., Choi, J. S., Kim, B. S., Choi, Y. C., and Cho, Y. W. (2010). Piezoelectric inkjet printing of polymers: Stem cell patterning on polymer substrates. Polymer 51 (10), 2147–2154. doi:10.1016/j.polymer.2010.03.038
Kloppenburg, M., and Berenbaum, F. (2020). Osteoarthritis year in review 2019: Epidemiology and therapy. Osteoarthr. Cartil. 28 (3), 242–248. doi:10.1016/j.joca.2020.01.002
Kosik-Koziol, A., Costantini, M., Bolek, T., Szoke, K., Barbetta, A., Brinchmann, J., et al. (2017). PLA short sub-micron fiber reinforcement of 3D bioprinted alginate constructs for cartilage regeneration. Biofabrication 9 (4), 044105. doi:10.1088/1758-5090/aa90d7
Kou, S. G., Peters, L. M., and Mucalo, M. R. (2021). Chitosan: A review of sources and preparation methods. Int. J. Biol. Macromol. 169, 85–94. doi:10.1016/j.ijbiomac.2020.12.005
Kreuz, P. C., Steinwachs, M. R., Erggelet, C., Krause, S. J., Konrad, G., Uhl, M., et al. (2006). Results after microfracture of full-thickness chondral defects in different compartments in the knee. Osteoarthr. Cartil. 14 (11), 1119–1125. doi:10.1016/j.joca.2006.05.003
Lafuente-Merchan, M., Ruiz-Alonso, S., Garcia-Villen, F., Gallego, I., Galvez-Martin, P., Saenz-Del-Burgo, L., et al. (2022). Progress in 3D bioprinting technology for osteochondral regeneration. Pharmaceutics 14 (8), 1578. doi:10.3390/pharmaceutics14081578
Lai, Y., Li, Y., Cao, H., Long, J., Wang, X., Li, L., et al. (2019). Osteogenic magnesium incorporated into PLGA/TCP porous scaffold by 3D printing for repairing challenging bone defect. Biomaterials 197, 207–219. doi:10.1016/j.biomaterials.2019.01.013
Lebourg, M., Rochina, J. R., Sousa, T., Mano, J., and Ribelles, J. L. (2013). Different hyaluronic acid morphology modulates primary articular chondrocyte behavior in hyaluronic acid-coated polycaprolactone scaffolds. J. Biomed. Mater Res. A 101 (2), 518–527. doi:10.1002/jbm.a.34349
Lee, K. Y., and Mooney, D. J. (2012). Alginate: Properties and biomedical applications. Prog. Polym. Sci. 37 (1), 106–126. doi:10.1016/j.progpolymsci.2011.06.003
Lespasio, M. J., Piuzzi, N. S., Husni, M. E., Muschler, G. F., Guarino, A., and Mont, M. A. (2017). Knee osteoarthritis: A primer. Perm. J. 21, 16–183. doi:10.7812/tpp/16-183
Levengood, S. L., and Zhang, M. (2014). Chitosan-based scaffolds for bone tissue engineering. J. Mater Chem. B 2 (21), 3161–3184. doi:10.1039/c4tb00027g
Li, C. D., Wang, K., Zhou, X. J., Li, T., Xu, Y., Qiang, L., et al. (2019b). Controllable fabrication of hydroxybutyl chitosan/oxidized chondroitin sulfate hydrogels by 3D bioprinting technique for cartilage tissue engineering. Biomed. Mater 14 (2), 025006. doi:10.1088/1748-605x/aaf8ed
Li, J., Chen, G., Xu, X., Abdou, P., Jiang, Q., Shi, D., et al. (2019a). Advances of injectable hydrogel-based scaffolds for cartilage regeneration. Regen. Biomater. 6 (3), 129–140. doi:10.1093/rb/rbz022
Li, L., Li, Y., Feng, D. Y., Xu, L. H., Yin, F. X., Zang, H. C., et al. (2016). Preparation of low molecular weight chondroitin sulfates, screening of a high anti-complement capacity of low molecular weight chondroitin sulfate and its biological activity studies in attenuating osteoarthritis. Int. J. Mol. Sci. 17 (10), 1685. doi:10.3390/ijms17101685
Li, M., Sun, D., Zhang, J., Wang, Y., Wei, Q., and Wang, Y. (2022). Application and development of 3D bioprinting in cartilage tissue engineering. Biomater. Sci. 10 (19), 5430–5458. doi:10.1039/d2bm00709f
Li, N., Qiao, D., Zhao, S., Lin, Q., Zhang, B., and Xie, F. (2021a). 3D printing to innovate biopolymer materials for demanding applications: A review. Mater Today Chem. 20, 100459. doi:10.1016/j.mtchem.2021.100459
Li, P., Fu, L., Liao, Z., Peng, Y., Ning, C., Gao, C., et al. (2021b). Chitosan hydrogel/3D-printed poly (ε-caprolactone) hybrid scaffold containing synovial mesenchymal stem cells for cartilage regeneration based on tetrahedral framework nucleic acid recruitment. Biomaterials 278, 121131. doi:10.1016/j.biomaterials.2021.121131
Lin, T. S., Hsieh, C. H., Kuo, C., Juang, Y. P., Hsieh, Y. S. Y., Chiang, H. S., et al. (2020b). Sulfation pattern of chondroitin sulfate in human osteoarthritis cartilages reveals a lower level of chondroitin-4-sulfate. Carbohyd Polym. 229, 115496. doi:10.1016/j.carbpol.2019.115496
Lin, W. F., Liu, Z., Kampf, N., and Klein, J. (2020a). The role of hyaluronic acid in cartilage boundary lubrication. Cells-Basel 9 (7), 1606. doi:10.3390/cells9071606
Liu, M., Zeng, X., Ma, C., Yi, H., Ali, Z., Mou, X., et al. (2017). Injectable hydrogels for cartilage and bone tissue engineering. Bone Res. 5 (1), 17014–17020. doi:10.1038/boneres.2017.14
LogithKumar, R., KeshavNarayan, A., Dhivya, S., Chawla, A., Saravanan, S., and Selvamurugan, N. (2016). A review of chitosan and its derivatives in bone tissue engineering. Carbohydr. Polym. 151, 172–188. doi:10.1016/j.carbpol.2016.05.049
Lories, R. J., and Luyten, F. P. (2011). The bone-cartilage unit in osteoarthritis. Nat. Rev. Rheumatol. 7 (1), 43–49. doi:10.1038/nrrheum.2010.197
Ma, P. X., Schloo, B., Mooney, D., and Langer, R. (1995). Development of biomechanical properties and morphogenesis ofin vitro tissue engineered cartilage. J. Biomed. Mater. Res. 29 (12), 1587–1595. doi:10.1002/jbm.820291215
Maity, C., and Das, N. (2021). Alginate-based smart materials and their application: Recent advances and perspectives. Top. Curr. Chem. (Cham) 380 (1), 3. doi:10.1007/s41061-021-00360-8
Makris, E. A., Gomoll, A. H., Malizos, K. N., Hu, J. C., and Athanasiou, K. A. (2015). Repair and tissue engineering techniques for articular cartilage. Nat. Rev. Rheumatol. 11 (1), 21–34. doi:10.1038/nrrheum.2014.157
Martínez-Camacho, A. P., Cortez-Rocha, M. O., Ezquerra-Brauer, J. M., Graciano-Verdugo, A. Z., Rodriguez-Félix, F., Castillo-Ortega, M. M., et al. (2010). Chitosan composite films: Thermal, structural, mechanical and antifungal properties. Carbohyd Polym. 82 (2), 305–315. doi:10.1016/j.carbpol.2010.04.069
Mohamed, O. A., Masood, S. H., and Bhowmik, J. L. (2015). Optimization of fused deposition modeling process parameters: A review of current research and future prospects. Adv. Manuf. 3 (1), 42–53. doi:10.1007/s40436-014-0097-7
Mondal, S., Haridas, N., Letha, S. S., Vijith, V., Rajmohan, G., and Rosemary, M. J. (2016). Development of injectable high molecular weight hyaluronic acid hydrogels for cartilage regeneration. J. Macromol. Sci. A 53 (8), 507–514. doi:10.1080/10601325.2016.1189284
Monfort, J., Pelletier, J. P., Garcia-Giralt, N., and Martel-Pelletier, J. (2008). Biochemical basis of the effect of chondroitin sulphate on osteoarthritis articular tissues. Ann. Rheum. Dis. 67 (6), 735–740. doi:10.1136/ard.2006.068882
Mooney, D. J., Baldwin, D. F., Suh, N. P., Vacanti, J. P., and Langer, R. (1996). Novel approach to fabricate porous sponges of poly(D,L-lactic-co-glycolic acid) without the use of organic solvents. Biomaterials 17 (14), 1417–1422. doi:10.1016/0142-9612(96)87284-x
Murab, S., Gupta, A., Wlodarczyk-Biegun, M. K., Kumar, A., van Rijn, P., Whitlock, P., et al. (2022). Alginate based hydrogel inks for 3D bioprinting of engineered orthopedic tissues. Carbohyd Polym. 296, 119964. doi:10.1016/j.carbpol.2022.119964
Murphy, S. V., and Atala, A. (2014a). 3D bioprinting of tissues and organs. Nat. Biotechnol. 32 (8), 773–785. doi:10.1038/nbt.2958
Murphy, S. V., and Atala, A. (2014b). 3D bioprinting of tissues and organs. Nat. Biotechnol. 32 (8), 773–785. doi:10.1038/nbt.2958
Neves, S. C., Moreira Teixeira, L. S., Moroni, L., Reis, R. L., Van Blitterswijk, C. A., Alves, N. M., et al. (2011). Chitosan/Poly(ɛ-caprolactone) blend scaffolds for cartilage repair. Biomaterials 32 (4), 1068–1079. doi:10.1016/j.biomaterials.2010.09.073
Ngo, T. D., Kashani, A., Imbalzano, G., Nguyen, K. T. Q., and Hui, D. (2018). Additive manufacturing (3D printing): A review of materials, methods, applications and challenges. Compos Part B-Eng 143, 172–196. doi:10.1016/j.compositesb.2018.02.012
Nguyen, A. K., and Narayan, R. J. (2017). Two-photon polymerization for biological applications. Mater Today 20 (6), 314–322. doi:10.1016/j.mattod.2017.06.004
Oliveira, J., and Reis, R. (2011). Polysaccharide-based materials for cartilage tissue engineering applications. J. Tissue Eng. Regen. M. 5 (6), 421–436. doi:10.1002/term.335
Park, H., Choi, B., Hu, J., and Lee, M. (2013). Injectable chitosan hyaluronic acid hydrogels for cartilage tissue engineering. Acta Biomater. 9 (1), 4779–4786. doi:10.1016/j.actbio.2012.08.033
Park, K. M., Lee, S. Y., Joung, Y. K., Na, J. S., Lee, M. C., and Park, K. D. (2009). Thermosensitive chitosan-Pluronic hydrogel as an injectable cell delivery carrier for cartilage regeneration. Acta Biomater. 5 (6), 1956–1965. doi:10.1016/j.actbio.2009.01.040
Patel, D. K., Sakhaei, A. H., Layani, M., Zhang, B., Ge, Q., and Magdassi, S. (2017). Highly stretchable and UV curable elastomers for digital light processing based 3D printing. Adv. Mater 29 (15), 1606000. doi:10.1002/adma.201606000
Peak, C. W., Singh, K. A., Adlouni, M., Chen, J., and Gaharwar, A. K. (2019). Printing therapeutic proteins in 3D using nanoengineered bioink to control and direct cell migration. Adv. Healthc. Mater 8 (11), e1801553. doi:10.1002/adhm.201801553
Quan, H. Y., Zhang, T., Xu, H., Luo, S., Nie, J., and Zhu, X. Q. (2020). Photo-curing 3D printing technique and its challenges. Bioact. Mater. 5 (1), 110–115. doi:10.1016/j.bioactmat.2019.12.003
Quintana, L., zur Nieden, N. I., and Semino, C. E. (2009). Morphogenetic and regulatory mechanisms during developmental chondrogenesis: New paradigms for cartilage tissue engineering. Tissue Eng. Part B Rev. 15 (1), 29–41. doi:10.1089/ten.teb.2008.0329
Rahmati, S., Shirazi, S., and Baghayeri, H. (2009). Piezo-electric head application in a new 3D printing design. Rapid Prototyp. J. 15 (3), 187–191. doi:10.1108/13552540910960280
Rajabi, M., McConnell, M., Cabral, J., and Ali, M. A. (2021). Chitosan hydrogels in 3D printing for biomedical applications. Carbohydr. Polym. 260, 117768. doi:10.1016/j.carbpol.2021.117768
Rao, S. H., Harini, B., Shadamarshan, R. P. K., Balagangadharan, K., and Selvamurugan, N. (2018). Natural and synthetic polymers/bioceramics/bioactive compounds-mediated cell signalling in bone tissue engineering. Int. J. Biol. Macromol. 110, 88–96. doi:10.1016/j.ijbiomac.2017.09.029
Rodriguez-Vazquez, M., Vega-Ruiz, B., Ramos-Zuniga, R., Saldana-Koppel, D. A., and Quinones-Olvera, L. F. (2015). Chitosan and its potential use as a scaffold for tissue engineering in regenerative medicine. Biomed. Res. Int. 2015, 1–15. doi:10.1155/2015/821279
Roughley, P. J., and Mort, J. S. (2014). The role of aggrecan in normal and osteoarthritic cartilage. J. Exp. Orthop. 1 (1), 8. doi:10.1186/s40634-014-0008-7
Ruiz-Alonso, S., Lafuente-Merchan, M., Ciriza, J., Saenz-Del-Burgo, L., and Pedraz, J. L. (2021). Tendon tissue engineering: Cells, growth factors, scaffolds and production techniques. J. Control Release 333, 448–486. doi:10.1016/j.jconrel.2021.03.040
Sage, J., Mallevre, F., Barbarin-Costes, F., Samsonov, S. A., Gehrcke, J. P., Pisabarro, M. T., et al. (2013). Binding of chondroitin 4-sulfate to cathepsin S regulates its enzymatic activity. Biochemistry 52 (37), 6487–6498. doi:10.1021/bi400925g
Salbach, J., Rachner, T. D., Rauner, M., Hempel, U., Anderegg, U., Franz, S., et al. (2012). Regenerative potential of glycosaminoglycans for skin and bone. J. Mol. Med. 90 (6), 625–635. doi:10.1007/s00109-011-0843-2
Sang, S., Mao, X., Cao, Y., Liu, Z., Shen, Z., Li, M., et al. (2023). 3D bioprinting using synovium-derived MSC-laden photo-cross-linked ECM bioink for cartilage regeneration. ACS Appl. Mater Interfaces 15, 8895–8913. doi:10.1021/acsami.2c19058
Schinagl, R. M., Gurskis, D., Chen, A. C., and Sah, R. L. (1997). Depth-dependent confined compression modulus of full-thickness bovine articular cartilage. J. Orthop. Res. 15 (4), 499–506. doi:10.1002/jor.1100150404
Setton, L. A., Elliott, D. M., and Mow, V. C. (1999). Altered mechanics of cartilage with osteoarthritis: Human osteoarthritis and an experimental model of joint degeneration. Osteoarthr. Cartil. 7 (1), 2–14. doi:10.1053/joca.1998.0170
Shariatinia, Z., and Jalali, A. M. (2018). Chitosan-based hydrogels: Preparation, properties and applications. Int. J. Biol. Macromol. 115, 194–220. doi:10.1016/j.ijbiomac.2018.04.034
Sharma, B., Fermanian, S., Gibson, M., Unterman, S., Herzka, D. A., Cascio, B., et al. (2013). Human cartilage repair with a photoreactive adhesive-hydrogel composite. Sci. Transl. Med. 5 (167), 167ra6. doi:10.1126/scitranslmed.3004838
Shi, W., Fang, F., Kong, Y., Greer, S. E., Kuss, M., Liu, B., et al. (2022). Dynamic hyaluronic acid hydrogel with covalent linked gelatin as an anti-oxidative bioink for cartilage tissue engineering. Biofabrication 14 (1), 014107. doi:10.1088/1758-5090/ac42de
Shin, J., Kang, E. H., Choi, S., Jeon, E. J., Cho, J. H., Kang, D., et al. (2021). Tissue-Adhesive chondroitin sulfate hydrogel for cartilage reconstruction. Acs Biomater. Sci. Eng. 7 (9), 4230–4243. doi:10.1021/acsbiomaterials.0c01414
Shirazi, S. F., Gharehkhani, S., Mehrali, M., Yarmand, H., Metselaar, H. S., Adib Kadri, N., et al. (2015). A review on powder-based additive manufacturing for tissue engineering: Selective laser sintering and inkjet 3D printing. Sci. Technol. Adv. Mater 16 (3), 033502. doi:10.1088/1468-6996/16/3/033502
Stellavato, A., Vassallo, V., La Gatta, A., Pirozzi, A. V. A., De Rosa, M., Balato, G., et al. (2019). Novel hybrid gels made of high and low molecular weight hyaluronic acid induce proliferation and reduce inflammation in an osteoarthritis in vitro model based on human synoviocytes and chondrocytes. Biomed. Res. Int. 2019, 1–13. doi:10.1155/2019/4328219
Stoppel, W. L., Ghezzi, C. E., McNamara, S. L., Iii, L. D. B., and Kaplan, D. L. (2015). Clinical applications of naturally derived biopolymer-based scaffolds for regenerative medicine. Ann. Biomed. Eng. 43, 657–680. doi:10.1007/s10439-014-1206-2
Sun, M. Y., Sun, X. T., Wang, Z. Y., Guo, S. Y., Yu, G. J., and Yang, H. Z. (2018). Synthesis and properties of gelatin methacryloyl (GelMA) hydrogels and their recent applications in load-bearing tissue. Polymers 10 (11), 1290. doi:10.3390/polym10111290
Teixeira, M. C., Lameirinhas, N. S., Carvalho, J. P., Silvestre, A. J., Vilela, C., and Freire, C. S. (2022). A guide to polysaccharide-based hydrogel bioinks for 3D bioprinting applications. Int. J. Mol. Sci. 23 (12), 6564. doi:10.3390/ijms23126564
Temenoff, J. S., and Mikos, A. G. (2000). Review: Tissue engineering for regeneration of articular cartilage. Biomaterials 21 (5), 431–440. doi:10.1016/s0142-9612(99)00213-6
Toh, W. S., Lee, E. H., Guo, X. M., Chan, J. K. Y., Yeow, C. H., Choo, A. B., et al. (2010). Cartilage repair using hyaluronan hydrogel-encapsulated human embryonic stem cell-derived chondrogenic cells. Biomaterials 31 (27), 6968–6980. doi:10.1016/j.biomaterials.2010.05.064
Turnbull, G., Clarke, J., Picard, F., Riches, P., Jia, L., Han, F., et al. (2018). 3D bioactive composite scaffolds for bone tissue engineering. Bioact. Mater. 3 (3), 278–314. doi:10.1016/j.bioactmat.2017.10.001
Varghese, S., Hwang, N. S., Canver, A. C., Theprungsirikul, P., Lin, D. W., and Elisseeff, J. (2008). Chondroitin sulfate based niches for chondrogenic differentiation of mesenchymal stem cells. Matrix Biol. 27 (1), 12–21. doi:10.1016/j.matbio.2007.07.002
Vinatier, C., and Guicheux, J. (2016). Cartilage tissue engineering: From biomaterials and stem cells to osteoarthritis treatments. Ann. Phys. Rehabil. Med. 59 (3), 139–144. doi:10.1016/j.rehab.2016.03.002
Wang, B., Diaz-Payno, P. J., Browe, D. C., Freeman, F. E., Nulty, J., Burdis, R., et al. (2021). Affinity-bound growth factor within sulfated interpenetrating network bioinks for bioprinting cartilaginous tissues. Acta Biomater. 128, 130–142. doi:10.1016/j.actbio.2021.04.016
Wang, D. A., Varghese, S., Sharma, B., Strehin, I., Fermanian, S., Gorham, J., et al. (2007). Multifunctional chondroitin sulphate for cartilage tissue-biomaterial integration. Nat. Mater 6 (5), 385–392. doi:10.1038/nmat1890
Wang, J., Goyanes, A., Gaisford, S., and Basit, A. W. (2016). Stereolithographic (SLA) 3D printing of oral modified-release dosage forms. Int. J. Pharm. 503 (1-2), 207–212. doi:10.1016/j.ijpharm.2016.03.016
Williams, G. M., Klisch, S. M., and Sah, R. L. (2008). Bioengineering cartilage growth, maturation, and form. Pediatr. Res. 63 (5), 527–534. doi:10.1203/pdr.0b013e31816b4fe5
Wong, B. L., and Sah, R. L. (2010). Mechanical asymmetry during articulation of tibial and femoral cartilages: Local and overall compressive and shear deformation and properties. J. Biomech. 43 (9), 1689–1695. doi:10.1016/j.jbiomech.2010.02.035
Woo, S. L., Lubock, P., Gomez, M. A., Jemmott, G. F., Kuei, S. C., and Akeson, W. H. (1979). Large deformation nonhomogeneous and directional properties of articular cartilage in uniaxial tension. J. Biomech. 12 (6), 437–446. doi:10.1016/0021-9290(79)90028-9
Wu, L. F., Zhao, L. D., Jian, M., Mao, Y. X., Yu, M., and Guo, X. H. (2018). EHMP-DLP: Multi-projector DLP with energy homogenization for large-size 3D printing. Rapid Prototyp. J. 24 (9), 1500–1510. doi:10.1108/rpj-04-2017-0060
Wu, L., Jing, D., and Ding, J. (2006). A "room-temperature" injection molding/particulate leaching approach for fabrication of biodegradable three-dimensional porous scaffolds. Biomaterials 27 (2), 185–191. doi:10.1016/j.biomaterials.2005.05.105
Xu, T., Jin, J., Gregory, C., Hickman, J. J., and Boland, T. (2005). Inkjet printing of viable mammalian cells. Biomaterials 26 (1), 93–99. doi:10.1016/j.biomaterials.2004.04.011
Xue, X., Hu, Y., Deng, Y., and Su, J. (2021). Recent advances in design of functional biocompatible hydrogels for bone tissue engineering. Adv. Funct. Mater. 31 (19), 2009432. doi:10.1002/adfm.202009432
Yamaoka, H., Asato, H., Ogasawara, T., Nishizawa, S., Takahashi, T., Nakatsuka, T., et al. (2006). Cartilage tissue engineering using human auricular chondrocytes embedded in different hydrogel materials. J. Biomed. Mater. Res. Part A 78 (1), 1–11. doi:10.1002/jbm.a.30655
Yang, J., Zhang, Y. S., Yue, K., and Khademhosseini, A. (2017). Cell-laden hydrogels for osteochondral and cartilage tissue engineering. Acta Biomater. 57, 1–25. doi:10.1016/j.actbio.2017.01.036
Yang, X., Li, S., Ren, Y., Qiang, L., Liu, Y., Wang, J., et al. (2022). 3D printed hydrogel for articular cartilage regeneration. Compos. Part B Eng. 237, 109863. doi:10.1016/j.compositesb.2022.109863
Yang, Y., Magnay, J. L., Cooling, L., and El, H. A. (2002). Development of a 'mechano-active' scaffold for tissue engineering. Biomaterials 23 (10), 2119–2126. doi:10.1016/s0142-9612(01)00342-8
Yao, Q., Wei, B., Liu, N., Li, C., Guo, Y., Shamie, A. N., et al. (2015). Chondrogenic regeneration using bone marrow clots and a porous polycaprolactone-hydroxyapatite scaffold by three-dimensional printing. Tissue Eng. Pt A 21 (7-8), 1388–1397. doi:10.1089/ten.tea.2014.0280
Yue, K., Trujillo-de Santiago, G., Alvarez, M. M., Tamayol, A., Annabi, N., and Khademhosseini, A. (2015). Synthesis, properties, and biomedical applications of gelatin methacryloyl (GelMA) hydrogels. Biomaterials 73, 254–271. doi:10.1016/j.biomaterials.2015.08.045
Zhang, L., Hu, J., and Athanasiou, K. A. (2009). The role of tissue engineering in articular cartilage repair and regeneration. Crit. Reviews™ Biomed. Eng. 37 (1-2), 1–57. doi:10.1615/critrevbiomedeng.v37.i1-2.10
Zhang, Y. B., Yu, J. K., Ren, K. X., Zuo, J. L., Ding, J. X., and Chen, X. S. (2019). Thermosensitive hydrogels as scaffolds for cartilage tissue engineering. Biomacromolecules 20 (4), 1478–1492. doi:10.1021/acs.biomac.9b00043
Zhao, D., Huang, J., Hu, S., Mao, J., and Mei, L. (2011). Biochemical activities of N,O-carboxymethyl chitosan from squid cartilage. Carbohyd Polym. 85 (4), 832–837. doi:10.1016/j.carbpol.2011.04.007
Zhu, W., Ma, X., Gou, M., Mei, D., Zhang, K., and Chen, S. (2016). 3D printing of functional biomaterials for tissue engineering. Curr. Opin. Biotechnol. 40, 103–112. doi:10.1016/j.copbio.2016.03.014
Keywords: bioprinting, 3D printing, hydrogels, natural polysaccharide, articular cartilage
Citation: Wu X, Cheng X, Kang M, Dong R, Zhao J and Qu Y (2023) Natural polysaccharide-based hydrogel bioprinting for articular cartilage repair. Front. Mater. 10:1204318. doi: 10.3389/fmats.2023.1204318
Received: 12 April 2023; Accepted: 21 July 2023;
Published: 31 July 2023.
Edited by:
Arun Prabhu Rameshbabu, Harvard Medical School, United StatesReviewed by:
João C. Silva, University of Lisbon, PortugalCopyright © 2023 Wu, Cheng, Kang, Dong, Zhao and Qu. This is an open-access article distributed under the terms of the Creative Commons Attribution License (CC BY). The use, distribution or reproduction in other forums is permitted, provided the original author(s) and the copyright owner(s) are credited and that the original publication in this journal is cited, in accordance with accepted academic practice. No use, distribution or reproduction is permitted which does not comply with these terms.
*Correspondence: Jianwu Zhao, amlhbnd1QGpsdS5lZHUuY24=; Yang Qu, cXV5QGpsdS5lZHUuY24=
†These authors have contributed equally to this work and share first authorship
Disclaimer: All claims expressed in this article are solely those of the authors and do not necessarily represent those of their affiliated organizations, or those of the publisher, the editors and the reviewers. Any product that may be evaluated in this article or claim that may be made by its manufacturer is not guaranteed or endorsed by the publisher.
Research integrity at Frontiers
Learn more about the work of our research integrity team to safeguard the quality of each article we publish.