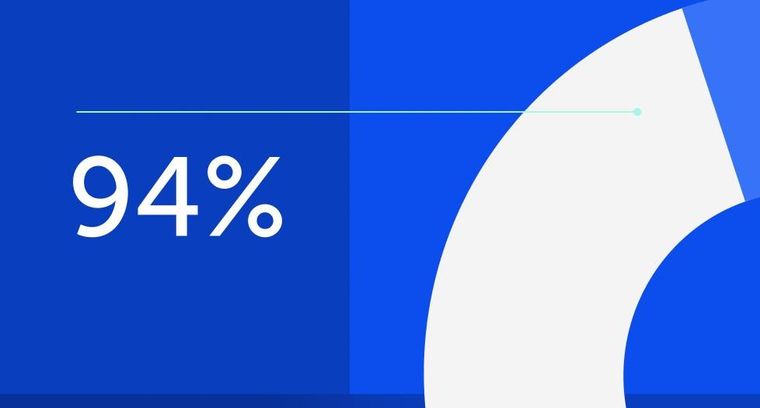
94% of researchers rate our articles as excellent or good
Learn more about the work of our research integrity team to safeguard the quality of each article we publish.
Find out more
ORIGINAL RESEARCH article
Front. Mater., 06 April 2023
Sec. Polymeric and Composite Materials
Volume 10 - 2023 | https://doi.org/10.3389/fmats.2023.1177093
This article is part of the Research TopicAdditive Manufacturing for PolymersView all 10 articles
Macro-sized porous single crystalline-like (PSC-like) TiO2 is endowed with unique structural advantages due to its structural consistency and porosity in a large area, which would significantly enhance its photoelectrochemical function. However, there are significant technical challenges in the growth of porous single crystalline-like monoliths. The consistency of structure dominates the structure so that the grain boundary is reduced to the minimum, which is in contradiction with the three-dimensional percolation structure. Here we report a lattice reconstruction strategy based on solid-solid transformation to grow porous single crystal-like anatase TiO2 dominated by (200) and (101) facets at 2 cm scale. In comparison with the traditional definition of porous single crystal, it has two different lattice orientations, but still has good photoelectrochemical properties. The band gap engineering introduces Ti3+ gap into the lattice to generate TinO2n−1 with Magneli phase, limiting the created active structure to the lattice with two-dimensional surface, which would open a new avenue to create highly active surfaces to capture photons and transport electrons stably. The PSC-like TinO2n−1 provides enhanced exciton lifetime (3–5 ns) as a photocatalytic catalyst and shows significant visible light absorption. The independent PSC-like TinO2n−1 delivers high photocurrent of 1.8–5.5 mA · cm−2 at room temperature and does not decay for 10 h.
The surface of transition metal oxides is usually used to carry the reaction of electron transfer and proton coupling, which is very important for the solar energy conversion process (Schrauben et al., 2012; Guo et al., 2019). Among photocatalysts, Ti-based semiconductor materials provide the high photocatalytic activity and play a key role in photocatalysis and solar energy conversion (Zhao et al., 2019; Chen et al., 2020). As an n-type photoanode, the band gap of titanium dioxide is about 3.2eV, and its energy conversion efficiency is often limited by the effective absorption of visible light and the rapid recombination of electrons and holes. In the process of modification of traditional nano-photocatalytic materials, nitrogen doping with unsaturated coordination structure is widely used to change the catalytic properties of materials (Bie et al., 2019; Meng et al., 2019; Xu et al., 2020). However, the metal-nitrogen group with immeasurable nitrogen coordination has structural disturbance in its catalytic activity, which is frequently accompanied by poor stability and dependability.
Anatase titanium dioxide is one of the transition metal oxide photocatalysts discussed in recent years because of its high photocatalytic activity due to its structural characteristics. As a typical traditional photocatalyst, its conduction band composed of valence electrons and empty orbitals is well understood. The Ti4+ and bridging O2- ions are staggered along the [101] axis, providing convenience for electronic structure adjustment (Li et al., 2006; Guo et al., 2016). Generally, titanium dioxide semiconductor with a broadband gap of ∼3.2eV is exposed to sunlight as the light collection device, and its absorption of visible light is limited, and the collection rate of light source at near-infrared wavelength is extremely low. Affected by its electronic structure, the conduction band of TiO2 receives the electrons excited from the valence band, and forms a high-energy and easily mobile electron-hole pair with the valence band structure with holes. Electron-hole pairs are usually used as medium to participate in the chemical reaction on the catalyst surface and reassemble to release heat and light energy. In addition, the electron-hole pair would also be dissociated again and transferred to the active site of the catalyst in the form of free electrons to form surface groups (Kou et al., 2017; Chen and Ardo, 2018). In this process, the band gap adjustment strategy and the sufficient reaction sites provided by the high specific surface are the keys to determine the photocatalytic performance (Li et al., 2022).
Different from the strategy of doping impurities into titanium dioxide lattice to form point defects, the TinO2n−1 with Magneli phase effectively enhances the absorption of visible light through the self-doping of Ti3+. The doping strategy with point defects is to achieve effective absorption of visible light by reducing the conduction band or increasing the valence band (Yu et al., 2013; Faraji et al., 2019). However, traditional black titanium dioxide prepared by hydrogenation often forms core-shell nanoparticles covered by Ti3+ disordered phase on the surface. The crystal structure that cannot be accurately confirmed and too many grain boundaries will greatly hinder the rapid transport of electrons. Here we adjust the electronic structure of the material to achieve enhanced absorption of visible and infrared light by introducing Ti3+ gap into PSC-like TinO2n−1 crystal to produce local defect structure (Cheng et al., 2019).
Titanium dioxide is usually used as the photoanode in the photochemical cell, and the direct catalytic splitting of water under light irradiation and external bias is one of the most active topics (Qian et al., 2019; Zhang et al., 2019; Bie et al., 2022). Generally, photogenic holes on the surface of titanium dioxide photoanode would activate water in the electrolyte to generate surface OH, while the electrons are transferred to the opposite electrode to reduce protons to hydrogen (Liu et al., 2019; Ros et al., 2020). The generation of OH is the key to the realization of photocatalytic water, which would provide opportunities for the participation of highly active hydroxyl radicals in heterogeneous catalysis, and shows great potential in basic and applied research (Shin et al., 2022).
In this work, the lattice reconstruction strategy based on the phase transformation is used to fabricate the PSC-like TinO2n−1 monoliths at 2 cm scale (Jin et al., 2020; Xiao and Xie, 2022). PSC-like TinO2n-1 monoliths has a high monocrystal-like property, and its dominant lattice structure maintains a high consistency with bulk single crystal. Its surface well-defined structure with clear and long-range order at atomic scale significantly reduces the scattering effect of electrons/holes caused by the interface between traditional grains, which will significantly enhance the functionality of charge separation and transmission required to inhibit charge recombination. Moreover, the PSC-like TinO2n-1 monoliths possess a large specific surface area, which makes them suitable as photoanodes in photoelectrochemical cells. This provides ample reaction sites for photocatalytic reactions and facilitates the collection of electrons/holes, resulting in ultra-high photocurrent and water decomposition performance.
The growth of precursor KTiOPO4 (KTP) single crystal is achieved by the topseed crystal method with molybdate as the cosolvent (Jacco et al., 1984). KTP single crystal with (110) faces is directionally cut and polished to 10 mm × 10 mm × 0.5 mm for growth of PSC-like anatase TiO2. The KTP single crystal is introduced into the vacuum tube furnace that can accurately control the pressure and temperature, and 100–200 sccm H2/Ar gas flow is introduced to control the pressure to stabilize to 67–333 mbar. KTP is maintained at 600°C–950°C for 10–30 h in a strong reducing atmosphere to generate PSC-like anatase TinO2n−1 monoliths of Magneli phase.
We detect the formation of the phase and determined the orientation of the crystal plane on the X-ray diffractometer (XRD, Mniflex 600). Then the porous morphology of the crystal material is observed by field emission scanning electron microscopy (FE-SEM, SU8010). TEM samples are prepared by using FIB (ZeissAuriga), selected area electron diffraction (SAED) tests are carried out at 200 kV accelerating voltage. The crystal structure is characterized on spherical aberration corrected high resolution transmission electron microscope (Cs-HRTEM) (FEITitan3G2 60–300) at 300 kV accelerating voltage. X-ray photoelectron spectroscopy (XPS, Escalab 250Xi) determines the chemical state of Ti in TinO2n−1. The electron paramagnetic resonance (EPR) spectrums are obtained on JES TE200 (JEOL) to evaluate the signals of Ti3+ and oxygen vacancies. The performance test of photoelectric decomposition water is carried out in 1M NaOH solution at 25°C.
In the photoelectrochemical test, we use the three-electrode test method. The electrochemical workstation (IM6, Zahner) is equipped with a two-chamber photocatalyst cell with Pt and saturated calomel electrode as the counter electrode and reference electrode, respectively. In addition, PSC-like TinO2n−1 monoliths are used as the working electrode, which is separated by an anion exchange membrane (Nafion 212). The photoelectric decomposition of water is tested in 1M sodium hydroxide solution at 25°C. The Nernst equation is that to convert all potentials to RHE reference scale:
The growth of porous single crystalline-like TiO2 is a dynamic equilibrium process between heat and force. We grow and treat (110) KTP (10 mm × 10 mm × 0.5 mm) to grow PSC-like anatase TiO2 monoliths with the identical dimension in a reducing atmosphere at 600–950°C and 67–333 mbar. It is well known that titanium dioxide is a typical photoelectric semiconductor with different crystal configurations such as rutile and anatase. The growth process of PSC-like rutile TiO2 often requires higher operating temperatures to drive the phase transition, which remains technically difficult. There is a strong dependence between the formation of PCS-like TinO2n−1 crystal rate and the crystal quality of KTP precursor. In the process of solid-solid phase transformation, the growth process of recrystallization is more sensitive to the surface roughness of the parent crystal. In Figure 1A, the sharp characteristic peak in the XRD pattern indicates that KTP with (110) facets has high single crystal quality. In inset image, the K and P atom escape channels along the KTP (110) facet are not completely unobstructed, which is one of the reasons for the appearance of competitive crystal planes in addition to the dominant crystal planes during the PSC-like TiO2 monolith growth process. As shown in Supplementary Figure S1, the Raman spectroscopy and SEM picture indicate the smooth uniform structure in KTP. We polish the KTP substrate to maintain its surface roughness below 0.24 nm as shown in Figure 1B; Figure 1C shows the flatness of KTP at the three-dimensional nanometer scale under the atomic force microscope. We used focused ion beam (FIB) to prepare the sample of Transmission Electron Microscope (TEM), that is (110) KTP slice with ∼50 nm thickness as shown in Figure 1D, which coupled with Cs-HRTEM to observe the lattice structure of mother crystal KTP in the field, as shown in Figure 1E. The uniform distribution of K, Ti, P, and O elements in the form of regular periodic arrays re-certified the surface well-defined structure of the single crystal to accommodate the order of solid-solid phase transformation to Ti-O coordination structure. The lattice spacing of 0.563 nm and 0.623 nm are certified as [110] and [200] facets of KTP, respectively, which is consistent with the structure of the selected area electron diffraction pattern (SAED), as shown in Figure 1F (Sorokina and Voronkova, 2007). Breaking the KTP single crystal structure as the starting step of the lattice reconstruction process, the long range ordered crystal structure of the single crystal is the key to the formation of the porous single crystalline TiO2 nucleus. In order to accelerate the growth of porous single crystalline-like monolith, the mother phase should be single crystalline state to enable stable solid-solid transformation (Li and Xie, 2023).
FIGURE 1. (A) XRD pattern of (110) KTP single crystal, the inset is crystal structure of KTP and lattice channel of K/P removal along (110) facet. (B) Surface roughness. (C) Surface 3D AFM image of KTP substrate. (D) TEM image of KTP slice. (E) Spherical aberration corrected Scanning Transmission Electron Microscope (Cs-HRTEM) image of the KTP view towards [110] plane. (F) SAED pattern of KTP.
Porous single crystal monoliths have the unique advantage in the construction of well-defined structures at surface interfaces by combining the consistency of the structure with the porous structure. Porous single crystalline-like monoliths retain a porous skeleton and the grain boundary region is minimized to ensure a high single crystal rate. In Supplementary Figure S2, The PSC-like anatase TiO2 is grown on the solid-solid phase transformation, and adjusted the single crystal rate by adjusting the annealing process to reduce the longitudinal temperature gradient. The higher the annealing rate, the faster the transverse and longitudinal surface stress changes, which means a lower single crystal rate. Figure 2A shows the XRD pattern of PSC-like anatase TiO2 dominated by (200) facet. The characteristic peaks of low exponent observed at 25.28° are consistent with the orientation [101] of anatase TiO2, indicating competitive growth between crystal facets, which is due to a high lattice mismatch of ∼23.58% between (110) KTP and (200) TiO2 (Jiang et al., 2011). Figure 2B and Figure 2C show the microstructure of PSC-TiO2 growing along the (110) KTP, which with pore size of ∼150 nm. The crystal scale of PSC-like TiO2 remains unchanged relative to KTP, while the directional removal of K/P as the target atom results in the reduction of the overall atomic density to form pores. We further confirmed the TiO2 transformation using EDS and elemental mapping, as shown in Supplementary Figure S3. Figure 2D shows the STEM image of PSC TiO2 monolith, confirming the 3D interconnected channel structure at ∼100–200 nm. It is noteworthy that the annealing process is one of the methods to adjust the dislocation density in PSC-like TiO2 monolith. The very small areas in PSC-like TiO2 monolith evolves from sub-grain boundary to grain boundary during the recrystallization process. Figure 2E shows the Cs-HRTEM image of the skeleton in PSC-like TiO2 framework. The introduction of a small amount Ti3+ causes the random dislocations in PSC Ti38O75 monolith, while a periodic dislocation such as Ti9O17 is shown in Supplementary Figure S4 with a large number of Ti3+ gaps. The chemical formula of TinO2n−1 is determined by the oxidation of graphite in a vacuum system. The SAED image of Fourier transform shown in the Figure 2F confirms that it is also a typical tetragonal single-crystalline structure.
FIGURE 2. (A) XRD pattern of PSC-like anatase TiO2. (B) The SEM image of PSC-like anatase TiO2 monolith. (C) Surface 3D AFM image of TiO2 with porous structure. (D) STEM image of the PSC-like TiO2, the inset image is TEM at the corresponding interface. (E) Cs-HRTEM image of PSC-like Ti38O75 viewed towards [-1–31] axis. (F) The illustration is the structure of anatase TiO2.
The peaks of Raman spectroscopy at 145, 197, 395, and 515 cm−1 are observed in Figure 3A, although slight Raman shift is observed, they are still consistent with the crystal structure of anatase phase (Es-Souni et al., 2008). We use X-ray photoelectron spectroscopy (XPS) to study the evolution of the Ti chemical state in the surface of PSC-like TiO2 under an Ar atmosphere. The surface Ti is mainly +4 in Ti38O75 as shown in Figure 3B, indicating that there is sufficient oxidation state in the lattice. The EPR spectrum in Figure 3C confirms the coexistence of Ti3+ and a small amount of oxygen vacancy on the surface of PSC Ti38O75 monolith, the signal index increases when the n valve are reduced to 9. The loss of oxygen means that the n value decreases in TinO2n-1, resulting in more Ti4+ being reduced to Ti3+ gap. Which would adjust the electronic and band gap structure of TiO2 to expand the visible-infrared light absorption, as shown in Figure 3D. The presence of the high concentration of Ti3+ interstitials in PSC-like Ti9O17 monolith with the magneli phase actually transforms it into a black electronic conductor. The transient absorption spectrum of PSC-like TinO2n−1 (n = 9 and 38) are further studied under the excitation wavelength of 523 nm. As shown in Figure 3E and Figure 3F, the ultra-long exciton lifetime (∼4.7 ns) in PSC-like TinO2n−1 (n = 9 and 38) monoliths is verified, which the lifetime is ∼5 times that of polycrystalline materials. This result indicates that the inhibition of structural coherence on charge recombination is significantly enhanced.
FIGURE 3. (A) Raman scattering spectra of PSC-like TinO2n-1. (B) XPS spectra of PSC-like TinO2n−1 (n = 9 and 38). (C) EPR spectra of Ti9O17 and Ti38O75. (D) Ultraviolet-visible absorbance spectra. (E and F) Decay profiles and fitting curve of femtosecond transient absorption spectra.
The precise adjustment of the electronic structure of TiO2 can achieve the maximum photocurrent response of light collection and increase the specific surface area of porous structure to enhance the photocatalytic performance. Here we use a three-electrode setup to study the photocurrent performance of PSC-like TiO2, as Figure 4A shown. In Figure 4B, we further used Linear Scanning Voltammetry to test the photocurrent-potential curve of PSC-like TinO2n−1 (n = 9 and 38) photoelectrode in 1M NaOH electrolyte solution. Under 10 times air mass (AM) 1.5G illumination of simulated sunlight, the photocurrent generated by PSC-like Ti9O17 photoanode could be as high as 5.5 mA · cm−2 at an external bias voltage of 1.6V. In addition, the initial potential of the PSC-like Ti9O17 electrode for water decomposition is ∼0.1 V in a 0.5M Na2SO4 electrolyte solution under illumination intensity of 10 AM 1.5 G. In Supplementary Figure S6, the dark current density is generally lower than 0.5 mA cm-2, which can be attributed to the synergy between the structural characteristics of PSC-like monolith and electronic transport. The current density is 5 times higher than that of the PSC-like Ti38O75 electrode and ∼8 times higher than that of the Non-porous polycrystal (Npc-TiO2) electrode. Which performance is well above that of nano-catalytic materials, demonstrating the superior activity and structural advantages of PSC-like Ti9O17 crystals in photoanode.
FIGURE 4. (A) The schematic diagram of photoelectrocatalysis cell. (B and C) Linear Scanning Voltammetry (LSV) curve of three electrode system using the P-SC TinO2n-1 photoanodes for water oxidation in the 1 M NaOH and 0.5M NaSO4, respectively. (TinO2n−1 as working electrode, Pt as counter electrode, Hg/Hg2Cl2 as reference electrode) (D) The photocurrent with enhanced light intensity up to 50×AM1.5 G sunlight using PSC-like Ti9O17 and non-porous polycrystalline (Npc) TiO2 electrodes. The external bias voltage is 1.23V. (E) Photocurrent durability test of the PSC-like Ti9O17 and Ti38O75 at 1.23V. (F) The crystal structure of Ti9O17 after stability test.
The photocurrent of PSC-like Ti9O17 increases linearly with the irradiation intensity, which photocurrent is as high as ∼ 20 mA cm-2 under the illumination intensity of 50 AM 1.5 G and the external bias voltage of 1.23 V. In addition, Npc-TiO2 is uniformly loaded on conductive glass of SnO2 doped with fluorine (FTO) as a photoanode system, which shows a saturated photocurrent density of ∼0.8 mA · cm−2 under the illumination intensity of 20 AM 1.5 G at 1.23V, indicating that its high-density grain boundary structure limits the conversion of energy. We further evaluated the durability of the PSC-like Ti9O17 photoelectrode by chronoamperometric measurement, as shown in Figure 4E, even after 10 h of operation, the photocurrent almost did not decay. It is noteworthy that the catalyst after operation still maintains uniform porous skeleton and well-defined lattice structure, as shown in Supplementary Figure S6 and Figure 4F, thus verifying the structural stability of PSC-like TiO2 as photoelectrode in the catalytic oxidation of water. In the Cs-HRTEM image of Figure 4E, the presence of residual electrolyte on the catalyst surface causes slight distortion in lattice clarity, but it would be ignored. The synergistic effect of porous microstructure, structural coherence and transport characteristics regulated by band gap engineering significantly enhanced the photocatalytic activity, resulting in an extremely high photoelectrochemical water oxidation performance.
In this work, we have grown PSC-like TiO2 monoliths dominated by (200) facets based on a lattice reconstruction strategy via solid-solid phase transformation, which has extremely high single-crystallinity and minimized grain boundary area while maintaining long-range ordered lattice structure and three-dimensional infiltration channels. It has two different lattice orientations, which is different with the porous single crystal, but still has good photoelectrochemical properties. We further introduce Ti3+ into the lattice by bandgap engineering to obtain a series of TinO2n−1 monolith with the Magneli phase, and tune the electronic structure to narrow the bandgap and enhance the absorption of visible light. We evaluated the PSC-like TiO2 as a photocathode for water oxidation at 25 °C in a three electrode system and obtained a high current with no obvious attenuation after 10 h of continuous operation. We have demonstrated that the synergistic effect of long-range ordered lattice structure and porous framework can effectively enhance photocatalytic activity and structural stability, providing a new avenue for the design of novel optoelectronic materials and the tuning of surface photoreactivity.
The original contributions presented in the study are included in the article/Supplementary Material, further inquiries can be directed to the corresponding authors.
KL wrote and revised the manuscript. FC performed the Crystal growth. YL, LL, and CW contributed to the results analysis. KX edited the manuscript. XL supervised the whole project.
This work was supported by the National Key Research and Development Program of China (2021YFA1501500), the Natural Science Foundation of Fujian Province (2020J05082, 2020J01113), Strategic Priority Research Program of Chinese Academy of Sciences (XDB2000000), Sichuan Science and Technology Program (2019JDJQ0011, 2020ZDZX0018, and 2022YFG0001), Youth Innovation Promotion Association, Chinese Academy of Sciences (2020371, 2023393), and West Light Foundation of the Chinese Academy of Sciences.
The authors declare that the research was conducted in the absence of any commercial or financial relationships that could be construed as a potential conflict of interest.
All claims expressed in this article are solely those of the authors and do not necessarily represent those of their affiliated organizations, or those of the publisher, the editors and the reviewers. Any product that may be evaluated in this article, or claim that may be made by its manufacturer, is not guaranteed or endorsed by the publisher.
The Supplementary Material for this article can be found online at: https://www.frontiersin.org/articles/10.3389/fmats.2023.1177093/full#supplementary-material
Bie, C., Wang, L., and Yu, J. (2022). Challenges for photocatalytic overall water splitting. Chem 8, 1567–1574. doi:10.1016/j.chempr.2022.04.013
Bie, C., Zhu, B., Xu, F., Zhang, L., and Yu, J. (2019). In situ grown monolayer N-doped graphene on CdS hollow spheres with seamless contact for photocatalytic CO2 reduction. Adv. Mater. 31, 1902868–1902876. doi:10.1002/adma.201902868
Chen, D., Cheng, Y., Zhou, N., Chen, P., Wang, Y., Li, K., et al. (2020). Photocatalytic degradation of organic pollutants using TiO2-based photocatalysts: A review. J. Clean. Prod. 268, 121725. doi:10.1016/j.jclepro.2020.121725
Chen, H. Y., and Ardo, S. (2018). Direct observation of sequential oxidations of a titania-bound molecular proxy catalyst generated through illumination of molecular sensitizers. Nat. Chem. 10, 17–23. doi:10.1038/NCHEM.2892
Cheng, F., Lin, G., Hu, X., Xi, S., and Xie, K. (2019). Porous single-crystalline titanium dioxide at 2 cm scale delivering enhanced photoelectrochemical performance. Nat. Commun. 10, 3618–3619. doi:10.1038/s41467-019-11623-w
Es-Souni, M., Kartopu, G., Habouti, S., Piorra, A., Es-Souni, M., Solterbeck, C. H., et al. (2008). Processing and thin film formation of TiO2-Pt nanocomposites. Phys. Status Solidi Appl. Mater. Sci. 205, 305–310. doi:10.1002/pssa.200723181
Faraji, M., Yousefi, M., Yousefzadeh, S., Zirak, M., Naseri, N., Jeon, T. H., et al. (2019). Two-dimensional materials in semiconductor photoelectrocatalytic systems for water splitting. Energy Environ. Sci. 12, 59–95. doi:10.1039/c8ee00886h
Guo, Q., Zhou, C., Ma, Z., Ren, Z., Fan, H., and Yang, X. (2016). Elementary photocatalytic chemistry on TiO2 surfaces. Chem. Soc. Rev. 45, 3701–3730. doi:10.1039/c5cs00448a
Guo, Q., Zhou, C., Ma, Z., and Yang, X. (2019). Fundamentals of TiO2 photocatalysis: Concepts, mechanisms, and challenges. Adv. Mater. 31, 1901997–1902026. doi:10.1002/adma.201901997
Jacco, J. C., Loiacono, G. M., Jaso, M., Mizell, G., and Greenberg, B. (1984). Flux growth and properties of KTiOPO4. J. Cryst. Growth 70, 484–488. doi:10.1016/0022-0248(84)90306-3
Jiang, H. B., Cuan, Q., Wen, C. Z., Xing, J., Wu, D., Gong, X.-Q., et al. (2011). Anatase TiO2 crystals with exposed high-index facets. Angew. Chem. 123, 3848–3852. doi:10.1002/ange.201007771
Jin, L., Cheng, F., Li, H., and Xie, K. (2020). Porous tantalum nitride single crystal at two-centimeter scale with enhanced photoelectrochemical performance. Angew. Chem. - Int. Ed. 59, 8891–8895. doi:10.1002/anie.202001204
Kou, J., Lu, C., Wang, J., Chen, Y., Xu, Z., and Varma, R. S. (2017). Selectivity enhancement in heterogeneous photocatalytic transformations. Chem. Rev. 117, 1445–1514. doi:10.1021/acs.chemrev.6b00396
Li, B., Zhao, J., Onda, K., Jordan, K. D., Yang, J., and Petek, H. (2006). Ultrafast interfacial proton-coupled electron transfer. Science 311, 1436–1440. doi:10.1126/science.1122190
Li, W. T., and Xie, K. (2023). Porous single crystals at the macroscale: From growth to application. Acc. Chem. Res. 56, 374–384. doi:10.1021/acs.accounts.2c00777
Li, Z., Wang, S., Wu, J., and Zhou, W. (2022). Recent progress in defective TiO2 photocatalysts for energy and environmental applications. Renew. Sustain. Energy Rev. 156, 111980. doi:10.1016/j.rser.2021.111980
Liu, N., Xu, M., Yang, Y., Zhang, S., Zhang, J., Wang, W., et al. (2019). Auδ--Ov-Ti3+ interfacial site: Catalytic active center toward low-temperature water gas shift reaction. ACS Catal. 9, 2707–2717. doi:10.1021/acscatal.8b04913
Meng, A., Zhang, L., Cheng, B., and Yu, J. (2019). Dual cocatalysts in TiO2 photocatalysis. Adv. Mater. 31, 1807660–1807731. doi:10.1002/adma.201807660
Qian, R., Zong, H., Schneider, J., Zhou, G., Zhao, T., Li, Y., et al. (2019). Charge carrier trapping, recombination and transfer during TiO2 photocatalysis: An overview. Catal. Today 335, 78–90. doi:10.1016/j.cattod.2018.10.053
Ros, C., Andreu, T., and Morante, J. R. (2020). Photoelectrochemical water splitting: A road from stable metal oxides to protected thin film solar cells. J. Mater. Chem. A 8, 10625–10669. doi:10.1039/d0ta02755c
Schrauben, J. N., Hayoun, R., Valdez, C. N., Braten, M., Fridley, L., and Mayer, J. M. (2012). Titanium and zinc oxide nanoparticles are proton-coupled electron transfer agents. Science 336, 1298–1301. doi:10.1126/science.1220234
Shin, H. J., Sohn, S. D., Kim, Y., Jung, S., Kang, J. S., Han, H., et al. (2022). C60 adsorbed on TiO2 drives dark generation of hydroxyl radicals. ACS Catal. 12, 5990–5996. doi:10.1021/acscatal.2c00755
Sorokina, N. I., and Voronkova, V. I. (2007). Structure and properties of crystals in the potassium titanyl phosphate family: A review. Crystallogr. Rep. 52, 80–93. doi:10.1134/S1063774507010099
Xiao, Y., and Xie, K. (2022). Active exsolved metal–oxide interfaces in porous single-crystalline ceria monoliths for efficient and durable CH4/CO2 reforming. Angew. Chem. - Int. Ed. 61, e202113079. doi:10.1002/anie.202113079
Xu, F., Meng, K., Cheng, B., Wang, S., Xu, J., and Yu, J. (2020). Unique S-scheme heterojunctions in self-assembled TiO2/CsPbBr3 hybrids for CO2 photoreduction. Nat. Commun. 11, 4613–4619. doi:10.1038/s41467-020-18350-7
Yu, X., Kim, B., and Kim, Y. K. (2013). Highly enhanced photoactivity of anatase TiO2 nanocrystals by controlled hydrogenation-induced surface defects. ACS Catal. 3, 2479–2486. doi:10.1021/cs4005776
Zhang, Y. C., Afzal, N., Pan, L., Zhang, X., and Zou, J. J. (2019). Structure-activity relationship of defective metal-based photocatalysts for water splitting: Experimental and theoretical perspectives. Adv. Sci. 6, 1900053. doi:10.1002/advs.201900053
Keywords: porous crystal, titanium dioxide, magneli phase, photoelectricity, catalysis
Citation: Liu K, Cheng F, Luo Y, Liu L, Wang C, Xie K and Luo X (2023) Porous single crystalline-like titanium dioxide monolith with enhanced photoelectrochemical performance. Front. Mater. 10:1177093. doi: 10.3389/fmats.2023.1177093
Received: 01 March 2023; Accepted: 22 March 2023;
Published: 06 April 2023.
Edited by:
Jingjun Wu, Zhejiang University, ChinaReviewed by:
Xiaozhen Zhang, Jingdezhen Ceramic Institute, ChinaCopyright © 2023 Liu, Cheng, Luo, Liu, Wang, Xie and Luo. This is an open-access article distributed under the terms of the Creative Commons Attribution License (CC BY). The use, distribution or reproduction in other forums is permitted, provided the original author(s) and the copyright owner(s) are credited and that the original publication in this journal is cited, in accordance with accepted academic practice. No use, distribution or reproduction is permitted which does not comply with these terms.
*Correspondence: Xiangang Luo, bHhnQGlvZS5hYy5jbg==; Kui Xie, a3hpZUBmamlyc20uYWMuY24=
Disclaimer: All claims expressed in this article are solely those of the authors and do not necessarily represent those of their affiliated organizations, or those of the publisher, the editors and the reviewers. Any product that may be evaluated in this article or claim that may be made by its manufacturer is not guaranteed or endorsed by the publisher.
Research integrity at Frontiers
Learn more about the work of our research integrity team to safeguard the quality of each article we publish.