- 1Harbin Institute of Technology, Harbin, China
- 2Key Lab of Structures Dynamic Behavior and Control of the Ministry of Education and Key Lab of Smart Prevention and Mitigation of Civil Engineering Disasters of the Ministry of Industry and Information Technology, Harbin Institute of Technology, Harbin, China
The transport properties of moisture and ionic chelators in the concrete affect not only the durability of the concrete, but also the effectiveness of the repair material in repairing cracks in the concrete. The transport of water and ionic chelators in the concrete was investigated by capillary absorption tests and ICP-OES (Inductively Coupled Plasma Optical Emission Spectrometer). It was found that the smaller the radius of the capillary pore within the concrete, the longer it takes to saturate it with water.The different pore sizes of capillaries take different times to reach saturation, which is one of the reasons for the “square root shift phenomenon” of capillary water absorption in concrete. The higher the initial concentration, the easier it is for the ion chelators to be transported inside the concrete within a certain transport depth range. However, after a certain range of transport depth, the initial concentration does not have a significant effect on the transport of the ion chelators. This is not the same as the transport pattern of nonchelated ions. Comparing the transport processes of chelated and non-chelated ions within the concrete, it was found that chelated ions form aggregation zones when transported within the concrete. The analysis suggests that the repair of pores and cracks within the concrete by the chelating agent promotes the creation of aggregation zones. Other factors such as the presence of chelated ions, the transport medium, changes in porosity, and wet and dry cycles can all influence the formation of chelated ion aggregates.
1 Introduction
Concrete is a non-homogeneous porous and brittle material with a large number of internal pores and crevices (Huang et al., 2021). In addition, concrete structures are highly susceptible to cracks of different sizes during construction and service. (Safiuddin et al., 2018; Kishiki et al., 2021). These cracks not only directly affect the strength of concrete, but also accelerate the rate of diffusion of various types of aggressive ions to the interior of concrete, seriously affecting the durability of reinforced concrete. (Ws et al., 2020; Zhou et al., 2020; Basu et al., 2021). In order to extend the service life of concrete structures, modifiers are usually added to the concrete in appropriate amounts prior to construction to give the concrete some self-healing ability (Yang et al., 2019; Liu et al., 2020; Wang et al., 2022b; Wang et al., 2022c). For cracks generated in concrete already in service, they are repaired more often by manual repair (Kramar et al., 2016; Dh et al., 2021). Crack repair technology based on ionic chelators is to add ionic chelators to the repair material and use its chelating effect to promote the formation of secondary hydration products at the designated repair interface, thereby enhancing the repair effect of cracks (Wang et al., 2022a; Zhang et al., 2022). Ionic chelators can form water-soluble chelates with calcium, magnesium and other metal ions in concrete. These chelates use water as a carrier to transfer to the concrete and then undergoes a series of chain reactions with other ions to produce water-insoluble crystalline substances to fill the internal pores of the concrete, making it more compact. This repair technique not only repairs cracks in the concrete, but also adds a degree of self-healing capacity to the concrete structure being repaired (Wang et al., 2020a; Wang et al., 2020b).
The quality of the concrete repair depends on the quality of the interface between the repair material and the concrete being repaired (Courard et al., 2015). Which depends on the development of cement hydration and microstructure at the repair interface. The moisture transfer during the repair process plays a crucial role in both cement hydration and porosity of the cementitious repair material (Lan et al., 2022). On the other hand, water as a carrier of ion chelators plays a vital role in the penetration process of chelating ions to the interior of concrete. Moisture transport within the concrete can be said to be the basis for the process of ion chelators transport to within the concrete (Zhang and Ye, 2018; Liu et al., 2022). There are three general modes of transport of water and water-soluble ions within cementitious materials, namely, permeation, diffusion and capillary absorption (Pel, 1991; Saeidpour and Wads, 2015). Capillary absorption is primarily a process whereby liquid phase water is drawn into the pore interior by capillary forces when the capillary pore is in a non-saturated state.
As early as the 1970s, Hall C (Hall, 1977; Hall and Kalimeris, 1982) and others began to study the flow equations of water in unsaturated porous media and their solution. Subsequently, capillary absorption experiments were carried out on porous materials such as bricks and rocks, and the “square root of time” law for capillary water transport was established in the experimental results. Based on extensive experiments, Hall C et al. (Hall, 1981) proposed an empirical theory for the front end of moisture transport, stating that the “square root of time” law for capillary water transport only applies to one-dimensional transport of moisture. The capillary absorption coefficient was introduced directly into cementitious materials in the early 1990s (Hall, 1993). Subsequently many scholars (Jamal et al., 2004; Razak et al., 2004; Choucha et al., 2018) have also conducted numerous experimental studies on the capillary absorption coefficient as one of the important parameters for evaluating the durability of cementitious materials. As late as the last century, carried out continuous tests for very long periods of time beyond the standard test times (Martys and Ferraris, 1997). It was found that even under one-dimensional transport conditions, the capillary water absorption process of cementitious materials does not fully comply with the “square root of time” law of capillary water transport. They attribute this shift to rehydration during transport and to the non-miscible transport of gas-liquid water and air during transport. Since then, scholars have begun to experimentally study the effects of heating conditions, initial humidity, nanomaterials and other factors on the capillary absorption coefficient (Espinosa and Franke, 2006; Oltulu and Sahin, 2011; Schiller et al., 2015). These studies invariably confirm that the capillary absorption coefficient of cementitious materials is not fixed during the capillary absorption process and is influenced by a number of factors (Walid et al., 2017). But they almost always focus on the description of various phenomena, with less micro-analysis and derivation (Caggiano et al., 2018).
As with capillary absorption, the internal transport of the ionic chelators in the concrete plays a crucial role in the effectiveness of the repair material containing the chelators. When there is no external environmental influence, the concentration of the various substances in the solution inside the concrete is in a state of equilibrium. This equilibrium is disturbed as soon as moisture, gases, ions, or other media enter the concrete. The process of breaking the equilibrium in turn affects the transport of moisture and ions within the concrete (Hemstad et al., 2020). Due to the complexity of its own material composition and the service environment in which it is located, the pore solution within the concrete is generally a multi-ion coexistence solution state, and there is an electrochemical coupling effect between dissimilar ions within the solution. Many scholars (Qing-feng et al., 2012; Weerdt et al., 2014; Urokovi et al., 2019) have used various assumptions to consider the interaction between ions in concrete pore solutions, but these assumptions do not directly reflect the true electrochemical state present in the pore solution during ion transport. Most of the existing literature also analyses the transport properties of constructed moisture and multiple ions through numerical simulations. Due to the complexity of the internal environment of concrete, the results of multiple ion transport are not the same as considering only single ion transport, and the principles of chelated ion transport differ significantly from non-chelated ion transport. The ion chelators can form chelates with the calcium and magnesium plasma in the pores, and the pH value in the solution will be affected in this process, and the change in pH value will in turn affect the stability of the chelate, which in turn affects the secondary hydration process of the concrete (Chen et al., 2018). Therefore, it is necessary to experimentally investigate the transport properties of chelating agents within concrete.
In fact, the transport properties of water and ions within the concrete directly affect the durability of the concrete structure (Zhang et al., 2011; Liu et al., 2018; Bai et al., 2019). Therefore, the study of the transport of water and ionic chelators within concrete is of great importance for the durability of concrete and the repair of concrete structures. The studies on moisture and chelated ion transport within concrete can provide significant value for theoretical studies and practical applications of concrete crack repair.
2 Experiments
2.1 Materials and methods
This experiment selected P.O 42.5 ordinary Portland cement produced by Jilin YaTai Cement. The selected cement performance meets the requirements of ASTM C1157-11. Its chemical composition is shown in Table 1 below.
The role of fly ash in cement-based materials has three primary effects: Form effect, activity effect, and micro-aggregate effect. The fly ash used in this experiment is Class I, and its chemical composition and physical properties are shown in Table 2 below.
2.2 Capillary water absorption coefficient
This experiment is based on existing research and investigates the internal moisture transport in concrete with different mix ratios using the isometric weighing method. The specimens were made into cubes of 100 mm in length according to the proportions in Table 3. The specimens were removed after 28 days and cut into 40 × 40 × 80 mm rectangular specimens for better comparison with the mortar and net slurry specimens in the subsequent tests.
Measurement of the capillary water absorption process in concrete, drawing on the method specified in ASTM C1585-2004. The specimens are cleaned and dried to a constant weight in a drying oven before the formal water absorption test begins. When the weight of the specimen no longer changes, the specimen is cooled to room temperature in the desiccator and then the sides of the specimen are sealed with epoxy resin. The capillary water absorption process is shown in Figure 1. The specimen is weighed on an electronic scale with an accuracy of 0.01 g. The specimen is placed in an absorbent container and water is added to the marked height and the time of absorption is recorded. After the water absorption time is reached, the test piece is removed and weighed, and the data is processed to obtain the relationship between water absorption and time. To make the results more accurate, eight capillary water absorption specimens were set up for each set of tests.
The actual measured water absorption of cementitious materials is influenced by a number of factors, in addition to the capillary water absorption of concrete, the humidity and temperature of the environment in which the cementitious material is located can affect its water absorption properties. The path of water loss and the rate of water loss in cementitious materials is also an important factor in their water absorption (A., 1985). In order to investigate the effect of the water loss process on the capillary water absorption process, this experiment was set up under the same conditions as the water loss test.
2.3 Experiments on the transport of ionic chelators inside concrete
Sodium hexametaphosphate (SHMP) was chosen to study the transport properties of ionic chelators in concrete. SHMP is a commonly used admixture in cementitious materials. SHMP acts as a retarder (Zhang et al., 2019), dispersant (Jia et al., 2016) and other agents in concrete (Bhattacherjee et al., 2022), and it is a chelating agent that forms chelates with cations in concrete. The chelating ions in EDTA-2Na were chosen as the influencing factor for the internal transport of SHMP in concrete. EDTA-2Na is a chemical often used in concrete to inhibit sulphate attack in cementitious materials, to enhance the strength of cementitious materials, and is also a frequently used chelating agent for calcium ions (Yan et al., 2020). As there were no other P-containing components in the concrete and additives, the P content was chosen to characterise the ion chelators transport process in the SHMP. The concrete specimens were soaked in different concentrations of SHMP and EDTA-2Na for 60 days before measurement. The concentrations of the ionic chelators immersion solutions are shown in Tables 4–6.
To investigate the difference in the transport properties of chelated and non-chelated ions in concrete, a set of NaCl solution immersion tests with the same molar concentration as the 5% single admixture SHMP solution were also set up.
After the specimen has been immersed for 60 d, the specimen is removed and the powder is drilled. Each specimen is drilled for powder at 10 selected locations (as shown in Figure 2).
The powder is drilled from the top to the bottom at each selected location in a sequence of different depth intervals. Depth intervals are set to 0–2 mm, 2–4 mm, 4–6 mm, 6–8 mm, 8–10 mm, 10–12 mm, 12–14 mm, and 14–16 mm in order. The powder was collected at the same depth and the P-element and organic carbon content of the powder was measured. Collecting powders from the same depth interval of the same specimen and measuring the P-element and organic carbon content of them.
3 Test results and analysis
3.1 Capillary water absorption test results
The results of the capillary water absorption in concrete are shown in Figure 3. From the test results, it can be seen that the actual water absorption of concrete is not proportional to the open square of the water absorption time, and that the amount of water absorbed in the same time varies for different strengths of concrete. In contrast, the water loss during concrete water loss shows a relatively good linear correlation with the water loss time, as shown in Figure 4.
C. Hall et al. (C et al., 1995; Lockington and Parlange, 2003) attribute this to the secondary hydration of the concrete during water transfer and water absorption. Taking the results of the C2 group test as an example, the changes in mass of the specimens before and after the capillary absorption test in this test are shown in Table 7. As can be seen from Table 7, the changes in specimen mass caused by secondary hydration during the experiment did not have a significant effect on the results of the capillary water absorption test.
Normally, only capillaries with pore sizes in the range of 50 nm-1 um can affect the permeability of concrete (Yu and Ye, 2013). In contrast, Rong’s tests showed that the number of capillary pores above 50 nm pore size remained constant during the later hydration process for concrete cured to 28 days of age (Rong et al., 2014). This indicates that for mature age concrete, the secondary hydration of the concrete at a later stage does not change the water absorption of the capillaries in the concrete. It can be concluded from this that the results of Figures 3, 4 are not only due to secondary hydration during water transport, but also to other reasons.
Moisture in the capillary tube internal transmission, due to the direction of the liquid surface tension tangent to the surface, and there is always a tendency to contract the surface of the liquid, making the liquid surface to generate additional pressure ΔP, from the Laplace equation we know:
where: θ is the contact angle, can be expressed as the angle between the liquid surface tension and the liquid-solid surface tension at any point of the intersection, r is the radius of the capillary pore, σ is the surface tension of the liquid.
It is assumed that the capillary walls do not react with water. The relationship between the length of the water intrusion path x in the capillary pore and the time t of water absorption at this point is (Benavente et al., 2002; Benavente et al., 2015):
where: υ is viscosity coefficient of water in capillary pore.
As can be seen from Eq. 2, The capillary depth of water absorption in porous materials is related to the pore size of the capillaries and the properties of the pore wall material itself. The pore size distribution of the capillary pores in cementitious materials is complex and the capillary absorption coefficient is not the same for different pore sizes.
Capillary pores in concrete can be equated to both horizontal and vertical situations. In the case of horizontal capillary pores, the original gas within the pore is compressed due to the sealing effect of the water or the pore itself. At this point the capillary suction in concrete is powered by the combined force of the additional pressure generated by the surface tension of the liquid on the surface of the liquid and the pressure generated by the compression of the gas within the pore, as shown in Figure 5.
When the pressure generated by the compressed gas and liquid surface tension in the liquid surface to produce additional pressure equal, can be seen as concrete capillary water absorption reached saturation. At this point, the water within the concrete is no longer transmitted through the capillary absorption, and the concrete at this time through the volume of water absorbed by the capillary and the volume of gas compressed within the pore space is equal. Set the volume of ΔV, according to the gas pressure formula can be seen when the concrete capillary water absorption saturation, the gas is compressed to produce a force equal to the additional pressure generated by the surface tension of the liquid on the surface of the liquid, it can be obtained:
where: ΔV is the volume of water absorbed by the pores when the concrete is saturated with water.
Substituting the gas pressure equation
where
Under the condition of constant pore cross-sectional area it can be introduced that:
where:
Substitute Eqs 1, 2 into Eq. 5 can be obtained capillary pore level when the concrete capillary water absorption reached saturation time t_0 and capillary pore size of the relationship between the equation is:
When the capillary pores are in the vertical state, the different states of connectivity of the capillary pores will directly affect the time of saturation of its water absorption. For non-closed pores, as there is no air compression in the forward direction of its water absorption, the power of capillary water absorption of concrete is provided by the combination of liquid surface tension and the gravity of water, as shown in Figure 6A. When the concrete is saturated with capillary water absorption, the combined force of the two is zero, which gives:
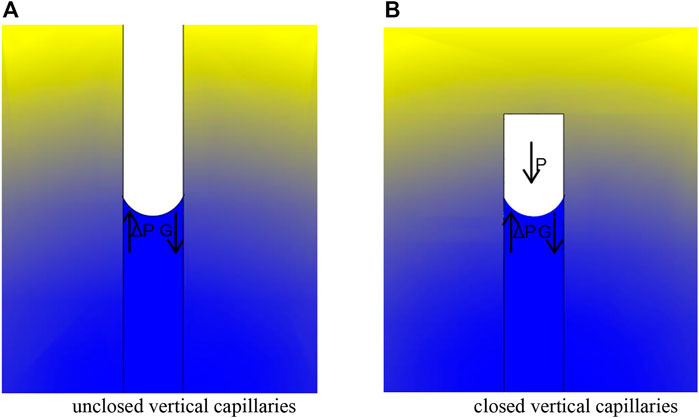
FIGURE 6. Internal forces on vertical capillaries. (A). Unclosed vertical capillaries (B). Closed vertical capillaries.
Substitute Eqs 1, 2into Eq. 7, a non-closed capillary pore vertical when the concrete capillary water absorption reached saturation time
When the pore is closed, the surface tension of the liquid, the pressure generated by the compression of the gas in the pore and the gravitational force of the water absorbed by the capillary pore provide the driving force for the capillary suction of concrete, as shown in Figure 6B.
When the combined force of these three forces is zero, the capillaries are saturated with water absorption that gives
Substituting Eqs 1, 2 into Eq. 9, we can obtain a non-closed capillary pore vertical when the concrete capillary water absorption reached saturation time t_0 and capillary pore size of the relationship between the equation is:
where:
Equations 6, 8, 10 all show that the capillary pores of different pore sizes not only differ in the amount of water they absorb, but also in the time it takes for the water to reach saturation. The larger the radius of the concrete capillary pore, the shorter the time it takes for the water to reach saturation, and the smaller its capillary absorption coefficient. This is in line with the test results in Figures 3, 4. This means that the rate of water absorption and the time to saturation vary depending on the pore size of the capillaries within the concrete.
The capillary absorption coefficient of the internal capillaries of cement-based materials is related to the pore size of the capillaries and the properties of the material itself. The internal capillary absorption coefficient of concrete can be seen as the superposition of the capillary absorption coefficients of all the capillaries involved in water absorption. At an early stage of the absorption process, almost all the capillaries are in a state of absorption. The capillary water absorption coefficient of concrete consists of the superposition of the capillary water absorption coefficients of all the individual capillaries. As all capillaries are not saturated with water, the water absorption coefficient is almost constant and the water absorption curve is approximately linear, with the amount of water absorbed being proportional to the square root of the water absorption time. When the capillary pores that have reached water absorption saturation are no longer involved in water absorption, the capillary absorption coefficient of this part of the capillary pore is no longer involved in the superposition of the capillary absorption coefficient of concrete. The number of capillary pores involved in capillary absorption gradually decreases in the order of pore size from large to small, resulting in a reduction in the capillary absorption coefficient of concrete. Macroscopically it shows a decrease in water absorption, a decrease in the rate of absorption, a decrease in the overall capillary absorption coefficient and a gradual smoothing of the absorption curve. During the process of water loss in concrete, the temperature, humidity, wind speed and kinematic viscosity of the air in the environment have a greater impact on the internal water transfer (Boukadida and Nasrallah, 2001). Compared to these factors, the influence of pore size on the process of water loss from capillaries is not significant, and the rate of water loss from capillaries with different pore sizes does not vary much (Yiotis et al., 2007; Qtp et al., 2019). As a result, the square root of water loss during the concrete water loss test shows a good linear correlation with the square root of water loss time.
3.2 Ionic chelators transport patterns
The effects of concentrations, other ionic chelating agents, transport media and wet and dry cycles on the internal transport of ionic chelating agents into concrete were investigated separately.
3.2.1 Concentrations
The concrete was immersed in different concentrations of chelating agent solutions and the experimental results of chelating agent transport within the concrete were as follows.
As can be seen from Figure 7, the concentration of ion chelators decreases broadly as the depth of penetration increases. The higher the concentration of the immersion solution over a range of depths, the higher the osmotic concentration at the same depth. This indicates that the concentration difference provides the impetus for the chelating agent to be transferred to the interior of the concrete. Unlike non-chelating ions, as the initial concentration of ion chelator increases, the concentration of ion chelator in the concrete decreases more rapidly with increasing depth. Once the chelator has been transported to a certain depth, the effect of the initial concentration on the transport of the ion chelator within the concrete gradually diminishes. This shows that the initial concentration can only influence the transport of ionic chelator in concrete within a certain depth range. Over a certain depth range, the initial concentration has almost no effect on the transport of the ion chelators. At the same molar concentration, the ionic chelating agent is weaker than the non-ionic chelating agent in terms of its ability to tran sport inside the concrete, as shown in Figure 8.
In addition, the ionic chelator “aggregates” in some areas during transport within the concrete. Intuitively, as the depth of penetration increases, the concentration of ion chelators increases and then decreases in some areas, forming a zone of ion chelators aggregation at different depths above both sides, as shown in Figure 9. Ionic chelating agents have a repairing effect on the internal pores of concrete (Wang et al.; Zhang et al., 2023) This repair ability allows the ion chelator to continuously reduce the porosity within the concrete during the transfer process. The decrease of concrete porosity will cause the ion chelator transport speed to decrease, which in turn will result in the build-up of ion chelator at a certain depth, forming an ion chelators aggregation zone. The experimental results in Figure 9 also shows that as the initial concentration of the immersion solution of the ion chelator increases, aggregates appear further in concrete. This means that the high concentration of ionic chelating agents may not be conducive to the repair of internal concrete damage. When the concentration of the ionic chelators solution in the soaked concrete was increased to 5%, the ionic chelators “aggregates” did not occur within the measurement range of the test. However, a comparison with the non-chelated ions transport process (Figure 8) shows that the degree of change in the transport rate of chelated ions is much less than that of non-chelated ions. As the initial concentration of ionic chelator is higher, the concentration of ionic chelator decreases faster with increasing depth within a certain depth range. This suggests that high concentrations of ionic chelator can still repair pores and microcracks within the concrete. However, the increase in ionic chelator concentration does not have a significant enhancement on its repairing ability.
The experimental results show that the transport pattern of chelating agent ions inside concrete is not exactly the same as the transport pattern of non-chelating ions. The initial concentration difference of the ion chelator is the driving force for the transport of the ion chelator into the concrete interior. When the depth of transport exceeds a certain range, the influence of the initial concentration on the transport process of the ion chelator inside the concrete diminishes. The ion chelator will form an aggregation zone when it is transported inside the concrete. The reason for this is that the ionic chelator has a repairing effect on the internal pores and cracks of the concrete. The ionic chelator repairs pores and micro-cracks within the concrete as it transfers within the concrete. The smaller pores in the repaired concrete reduce the rate of chelated ion transport on the inside of the repaired concrete, while the rate of chelated ion transport on the outside of this part of the concrete changes even less. The different degree of reduction in chelating ion transport velocity between the two sides results in localised aggregation in the concrete being repaired by the chelating agent.
3.2.2 Influence of other ionic chelators
When other ionic chelators interfere, the transport of chelators ions within the concrete is shown in Figure 10:
The test results show that the rate of transport of chelated ions into the concrete is reduced in the presence of other chelating agents. However, the presence of the other chelating agent promotes the formation of “aggregation” zones. As shown in Figure 11, the presence of another chelating agent resulted in more chelating ion “aggregation” zones in the experimental specimens. This indicates that the effect of different chelating ions on concrete repair is superimposable and that the chelate’s ability to repair concrete is enhanced by the addition of different chelating agents.
3.3.3 Transport media and wet/dry cycles
The results of the effect of different transport media on the ion chelators transport process are shown in Figure 12. From the experimental results it can be seen that the order of the magnitude of the permeability of SHMP in the three media of concrete, mortar and net slurry is: mortar > concrete > net slurry. This corresponds to the porosity of the three measured by the mercury pressure test. It shows that porosity is an important factor affecting the transport performance of ion chelators, the larger the porosity, the better the permeability of ion chelators. As in the previous test, the phenomenon of complex aggregation was also observed for all three, and the aggregation was particularly evident when mortar was used as the transport medium. This once again shows that the complex ion chelators penetrates into the cementitious material and repairs it, and the greater the porosity the more pronounced the repair process. As the ion chelators is transported into the concrete it repairs micro-damage within the concrete, the repair process changes the porosity of the concrete and causes areas of ion chelators “aggregation” within the concrete. The higher the porosity of the cementitious material, the more adequately the ionic chelators will repair the internal damage and the more obvious this “aggregation” phenomenon will be.
The experimental results of the effect of wet and dry cycles on the internal transport of ionic chelators in concrete are shown in Figure 13. As can be seen from Figure 13, when the penetration depth was less than 12 mm, the concentration in the wet/dry cycle group was greater than that in the comparison group at the same depth. At the same initial concentration, the wet/dry cycle increases the rate of transport of the ion chelators within the concrete, which is the same as the transport pattern of non-chelated ions. The aggregation of the ion chelators under dry/wet cycling conditions was not significant, indicating that the repairing effect of the ion chelators in the dry/wet cycling group of tests was not significant. Which means that the wet/dry cycle promotes the rate of ion chelators transport, but is not conducive to the repair of internal concrete pores and cracks by chelated ions.
4 Conclusion
Ionic chelating agents are one of the additives commonly used in cementitious materials. In this paper, the transport process of ion chelators inside concrete is experimentally investigated, taking into account that water is the carrier for the transport of chelated ions to the inside of concrete, and also exploring the process of capillary water absorption inside concrete. The following conclusions were drawn from the experiments .
1) The time for capillary pores of different pore sizes to reach water absorption saturation is not the same, the larger the pore size, the shorter the time required for capillary pores to reach water absorption saturation. The capillary absorption coefficient of concrete can be regarded as the superposition of the capillary absorption coefficients of all the capillary pores involved in capillary absorption within it. The large pore size capillaries are the first to reach water absorption saturation and then no longer participate in capillary absorption. Resulting in a gradual decrease in the capillary absorption coefficient of concrete. Macroscopically, the relationship between the capillary water absorption of concrete and the square root of time is no longer linear. The time taken for the different pore sizes within the concrete to reach water saturation is not synchronised, which is one of the reasons for the “square root shift” in the one-dimensional capillary water absorption of concrete.
2) The initial concentration difference is one of the driving forces behind the transport of chelated ions within the concrete. In the range of certain transport depths, the higher the initial concentration, the greater the rate of transport of chelated ions within the concrete. Outside of a certain depth of transport, the initial concentration of the ion chelators has a reduced effect on its transport within the concrete.
3) The transport patterns of chelated and non-chelated ions within concrete are not identical. When transported within concrete, chelated ions repair pores and micro-cracks within the concrete and reduce the local porosity of the concrete. Which can result in areas of chelated ion aggregation within the concrete. The increase in the initial concentration of the ion chelator does not have a significant effect on its ability to repair and therefore delays the appearance of the chelated ion aggregation zone. The porosity of the transport medium can also influence the transport of chelated ions within the concrete. The higher the porosity, the greater the rate of transport of the ion chelators within the concrete and the more obvious the repair of internal pores and microcracks in the concrete.
4) The addition of other ionic chelating agents reduces the rate of transport of chelated ions within the concrete, resulting in a lower concentration of specific chelated ions at the same depth. However, the addition of other chelating agents enhances the repair effect of the chelated ions on the concrete, resulting in more zones of ion chelating agent aggregation within the concrete.
5) The wet/dry cycle increased the concentration of chelated ions at the same depth, but reduced the number of ion chelator aggregation zones. It suggests that the wet and dry cycle increases the rate of ion chelator transport within the concrete, but is not conducive to the repair of internal concrete damage by chelating ions.
Data availability statement
The original contributions presented in the study are included in the article/supplementary material, further inquiries can be directed to the corresponding author.
Author contributions
Under the supervision of XG and RL performed sample preparation, data analysis, structure fabrication and manuscript writing. Both authors read and contributed to the manuscript.
Conflict of interest
The authors declare that the research was conducted in the absence of any commercial or financial relationships that could be construed as a potential conflict of interest.
Publisher’s note
All claims expressed in this article are solely those of the authors and do not necessarily represent those of their affiliated organizations, or those of the publisher, the editors and the reviewers. Any product that may be evaluated in this article, or claim that may be made by its manufacturer, is not guaranteed or endorsed by the publisher.
References
Bai, Y., Wang, Y., and XI, Y. (2019). Modeling the effect of temperature gradient on moisture and ionic transport in concrete. Cem. Concr. Compos. 106, 103454. doi:10.1016/j.cemconcomp.2019.103454
Basu, P., Thomas, B. S., Gupta, R. C., and Agrawal, V. (2021). Strength, permeation, freeze-thaw resistance, and microstructural properties of self-compacting concrete containing sandstone waste. J. Clean. Prod. 305, 127090. doi:10.1016/j.jclepro.2021.127090
Benavente, D., Lock, P., Ángeles GarcíA Del Cura, M., and OrdóñEZ, S. (2002). Predicting the capillary imbibition of porous rocks from microstructure. Transp. Porous Media 49, 59–76. doi:10.1023/a:1016047122877
Benavente, D., Pla, C., Cueto, N., Galvañ, S., MartíNEZ-MartíNEZ, J., GarcíA-Del-Cura, M. A., et al. (2015). Predicting water permeability in sedimentary rocks from capillary imbibition and pore structure. Eng. Geol. 195, 301–311. doi:10.1016/j.enggeo.2015.06.003
Bhattacherjee, S., Jain, S., and Santhanam, M. (2022). A method to increase the workability retention of concrete with limestone calcined clay based cementitious system using a dispersing agent containing sodium hexametaphosphate. Cem. Concr. Compos. 132, 104624. doi:10.1016/j.cemconcomp.2022.104624
Boukadida, N., and Nasrallah, S. B. (2001). Mass and heat transfer during water evaporation in laminar flow inside a rectangular channel — Validity of heat and mass transfer analogy. Int. J. Therm. Sci. 40, 67–81. doi:10.1016/s1290-0729(00)01181-9
Caggiano, A., Schicchi, D. S., Mankel, C., Ukrainczyk, N., and Koenders, E. (2018). A mesoscale approach for modeling capillary water absorption and transport phenomena in cementitious materials. Comput. Struct. 200, 1–10. doi:10.1016/j.compstruc.2018.01.013
Chen, H., Feng, P., Ye, S., and Wei, S. (2018). The coupling effect of calcium concentration and pH on early hydration of cement. Constr. Build. Mater. 185, 391–401. doi:10.1016/j.conbuildmat.2018.07.067
Choucha, N. N. S., Benyahia, N. N. A., Ghrici, N. N. M., and Mansour, N. N. M. S. (2018). Effect of natural pozzolan content on the properties of engineered cementitious composites as repair material. Front. Struct. Civ. Eng. 12, 261–269. doi:10.1007/s11709-017-0394-x
Courard, L., Bissonnette, B., and Garbacz, A. (2015). Fundamental approach for the concept of concrete repair compatibility, Proceedings of the International Conference on Concrete Repair, Rehabilitation and Retrofitting, October 2015, Leiden, Netherlands.
Dh, A., Cong, W. A., Bing, Y. A., Xh, A., Hx, A., Xw, A., et al. (2021). Investigation of composite silane emulsion modified by in-situ functionalized graphene oxide for cement-based materials. Constr. Build. Mater. 304, 124662. doi:10.1016/j.conbuildmat.2021.124662
Espinosa, R. M., and Franke, L. (2006). Inkbottle Pore-Method: Prediction of hygroscopic water content in hardened cement paste at variable climatic conditions. Cem. Concr. Res. 36, 1954–1968. doi:10.1016/j.cemconres.2006.06.011
Hall, C., and Kalimeris, A. N. (1982). Water movement in porous building materials—V. Absorption and shedding of rain by building surfaces. Build. Environ. 17, 257–262. doi:10.1016/0360-1323(82)90018-x
Hall, C. (1977). Water movement in porous building materials—I. Unsaturated flow theory and its applications. Build. Environ. 12, 117–125. doi:10.1016/0360-1323(77)90040-3
Hall, C. (1981). Water movement in porous building materials—IV. The initial surface absorption and the sorptivity. Build. Environ. 16, 201–207. doi:10.1016/0360-1323(81)90014-7
Hall, C., (1995). Water anomaly in capillary liquid absorption by cement-based materials. J. Mater. Sci. Lett. 14, 1178–1181.
Hemstad, P., Machner, A., and Weerdt, K. D. (2020). The effect of artificial leaching with HCl on chloride binding in ordinary Portland cement paste. Cem. Concr. Res. 130, 105976. doi:10.1016/j.cemconres.2020.105976
Huang, J., Li, W., Huang, D., Wang, L., Chen, E., Wu, C., et al. (2021). Fractal analysis on pore structure and hydration of magnesium oxysulfate cements by first principle, thermodynamic and microstructure-based methods. Fractal and Fractional. 5, 4, 164.
Jamal, K. H. A. T. I. B., and Roger & Clay, (2004). Absorption characteristics of metakaolin concrete. Cem. Concr. Res. 34, 19–29. doi:10.1016/s0008-8846(03)00188-1
Jia, Y., Wang, B., Wu, Z., Han, J., Zhang, T., Vandeperre, L. J., et al. (2016). Role of sodium hexametaphosphate in MgO/SiO2 cement pastes. Cem. Concr. Res. 89, 63–71. doi:10.1016/j.cemconres.2016.08.003
Kishiki, S., Yang, X., Ishida, T., Tatsumi, N., and Yamada, S. (2021). Experimental study of concrete breakout failure mechanism in an exposed column base with a foundation beam. Eng. Struct. 243, 112661. doi:10.1016/j.engstruct.2021.112661
Kramar, S., Šajna, A., and Ducman, V. (2016). Assessment of alkali activated mortars based on different precursors with regard to their suitability for concrete repair. Constr. Build. Mater. 124, 937–944. doi:10.1016/j.conbuildmat.2016.08.018
Lan, M., Zhou, J., Li, H., and Wang, Y. (2022). Numerical simulation on cement hydration and microstructure development in repair-substrate interface. Front. Mater. 9. doi:10.3389/fmats.2022.829743
Liu, B., Shi, J., Sun, M., He, Z., and Tan, J. (2020). Mechanical and permeability properties of polymer-modified concrete using hydrophobic agent. J. Build. Eng. 31, 101337. doi:10.1016/j.jobe.2020.101337
Liu, C., Baudet, B., and Zhang, M. (2022). Lattice Boltzmann modelling of ionic diffusivity in non-saturated limestone blended cement paste. Constr. Build. Mater. 316, 126060. doi:10.1016/j.conbuildmat.2021.126060
Liu, Q. F., Feng, G. L., Xia, J., Yang, J., and Li, L. Y. (2018). Ionic transport features in concrete composites containing various shaped aggregates: A numerical study. Compos. Struct. 183, 371–380. doi:10.1016/j.compstruct.2017.03.088
Lockington, D. A., and Parlange, J. Y. (2003). Anomalous water absorption in porous materials. J. Phys. D Appl. Phys. 36 (8), 760–767. doi:10.1088/0022-3727/36/6/320
Martys, N. S., and Ferraris, C. F. (1997). Capillary transport in mortars and concrete. Cem. Concr. Res. 27, 747–760. doi:10.1016/s0008-8846(97)00052-5
Oltulu, M., and Sahin, R. (2011). Single and combined effects of nano-SiO2, nano-Al2O3 and nano-Fe2O3 powders on compressive strength and capillary permeability of cement mortar containing silica fume. Mater. Sci. Eng. A 528, 7012–7019. doi:10.1016/j.msea.2011.05.054
Pel, L. L. (1991). Moisture transport in porous building materials. Sci. Technol. Eur. Cult. Herit. 41, 593–596.
Platten, A. K. (1985). A study of evaporation and drying in porous building materials. PhD thesis. Manchester, England: University of Manchester Institute of Science and Technology.
Qing-Fengliu, , Long-Yuan, , Easterbrook, D., and Yang, J. (2012). Multi-phase modelling of ionic transport in concrete when subjected to an externally applied electric field. Eng. Struct. 42, 201–213. doi:10.1016/j.engstruct.2012.04.021
Qtp, A., Nm, A., Ej, A., Dj, A., Gds, B., and Gyb, C. (2019). Insights and issues on the correlation between diffusion and microstructure of saturated cement pastes. Cem. Concr. Compos. 96, 106–117. doi:10.1016/j.cemconcomp.2018.11.018
Razak, H. A., Chai, H. K., and Wong, H. S. (2004). Near surface characteristics of concrete containing supplementary cementing materials. Cem. Concr. Compos. 26, 883–889. doi:10.1016/j.cemconcomp.2003.10.001
Rong, Z. D., Sun, W., Xiao, H. J., and Wang, W. (2014). Construction and building materials, Effect of silica fume and fly ash on hydration and microstructure evolution of cement based composites at low water-binder ratios, 51, 446-450.
Saeidpour, M., and Wads, L. (2015). Evidence for anomalous water vapor sorption kinetics in cement based materials. Cem. Concr. Res. 70, 60–66. doi:10.1016/j.cemconres.2014.10.014
Safiuddin, M., Kaish, A. B. M. A., Woon, C.-O., and Raman, S. N. (2018). Early-Age Cracking in Concrete: Causes, Consequences, Remedial Measures, and Recommendations, 8. Applied Sciences.
Schiller, P., Wahab, M., Bier, T., Waida, S., and M?Gel, H. J. (2015). Capillary forces and sorption hysteresis of cement pastes with small slit pores. Procedia Mater. Sci. 11, 649–654. doi:10.1016/j.mspro.2015.11.010
Urokovi, M., Matkovi-Alogovi, D., and Janotka, I. (2019). Mobility of trace elements in pore solutions of Portland cement pastes exposed to leaching. Croat. Chem. Acta 92, 103–114. doi:10.5562/cca3511
Walid, D. E. B. O. U. C. H. A., Nordine, L. E. K. L. O. U., Abdelhafid, K. H. E. L. I. D. J., Mohamed, N., and Oudjit, (2017). Natural pozzolana addition effect on compressive strength and capillary water absorption of Mortar. Energy Procedia 139, 689–695. doi:10.1016/j.egypro.2017.11.273
Wang, H., Yuan, M., Wu, J., Wan, P., and Liu, Q. (2022). Self-healing properties of asphalt concrete with calcium alginate capsules containing different healing agents. Mater. (Basel) 15, 5555. doi:10.3390/ma15165555
Wang, L., Yu, Z., Liu, B., Zhao, F., Tang, S., and Jin, M. (2022). Effects of fly ash dosage on shrinkage, crack resistance and fractal characteristics of face slab concrete. Fractal and Fractional, 6, 6.
Wang, L., Zeng, X., Li, Y., Yang, H., and Tang, S. (2022). Influences of MgO and PVA fiber on the abrasion and cracking resistance, pore structure and fractal features of hydraulic concrete. Fractal and Fractional, 6, 11, 674.
Wang, R., Yu, J., Gu, S., Han, X., He, P., Liu, Q., et al. (2020a). Effect of ion chelator on hydration process of Portland cement. Constr. Build. Mater. 259, 119727. doi:10.1016/j.conbuildmat.2020.119727
Wang, R., Yu, J., Gu, S., He, P., Han, X., and Liu, Q. Investigation of migration and self-healing ability of ion chelator in cement-based materials by a novel method. Constr. Build. Mater., 262.
Wang, R., Yu, J., Gu, S., He, P., Han, X., and Liu, Q. (2020b). Investigation of self-healing capability on surface and internal cracks of cement mortar with ion chelator. Constr. Build. Mater. 236, 117598. doi:10.1016/j.conbuildmat.2019.117598
Weerdt, K. D., OrsáKOVá, D., and Geiker, M. R. (2014). The impact of sulphate and magnesium on chloride binding in Portland cement paste. Cem. Concr. Res. 65, 30–40. doi:10.1016/j.cemconres.2014.07.007
Ws, A., Mn, A., and Bsa, B. (2020). Reinforcement corrosion and transport of water and chloride ions in shrinkage-compensating cement concretes. Cem. Concr. Res. 135, 106121. doi:10.1016/j.cemconres.2020.106121
Yan, X., Jiang, L., Guo, M., Chen, Y., Zhu, P., Jin, W., et al. (2020). Using EDTA-2Na to inhibit sulfate attack in slag cement mortar under steam curing. Constr. Build. Mater. 265, 120324. doi:10.1016/j.conbuildmat.2020.120324
Yang, Z. H. O. U., Jingshun, C. A. I., Dongshuai, H. O. U., Honglei, C. H. A. N. G., and Jiao & Yu, (2019). The inhibiting effect and mechanisms of smart polymers on the transport of fluids throughout nano-channels - ScienceDirect. Appl. Surf. Sci. 500, 144019.
Yiotis, A. G., Tsimpanogiannis, I. N., Stubos, A. K., and Yortsos, Y. C. (2007). Coupling between external and internal mass transfer during drying of a porous medium. Water Resour. Res. 43, n/a. doi:10.1029/2006wr005558
Yu, Z., and Ye, G. (2013). The pore structure of cement paste blended with fly ash. Constr. Build. Mater. 45, 30–35. doi:10.1016/j.conbuildmat.2013.04.012
Zhang, C., Guan, X., Lu, R., Li, J., and Li, Y. (2023). Effect of cementitious capillary crystalline waterproof material on the various transport properties of cracked cementitious composites. Constr. Build. Mater. 365. doi:10.1016/j.conbuildmat.2022.130138doi:130138
Zhang, C., Liu, R., Chen, M., Li, X., and Zhu, Z. (2022). Coupled effect of self-healing granules and permeable crystalline additive on early-age cracks repair in cement material. Mater. Lett. 323, 132560. doi:10.1016/j.matlet.2022.132560
Zhang, M., Ye, G., and Breugel, K. V. (2011). Microstructure-based modeling of water diffusivity in cement paste. Constr. Build. Mater. 25, 2046–2052. doi:10.1016/j.conbuildmat.2010.11.042
Zhang, Y., Liu, H., Liu, J., and Tong, R. (2019)., 12. Materials, 4190. doi:10.3390/ma12244190Effect of sodium hexametaphosphate and trisodium phosphate on dispersion of polycarboxylate superplasticizer
Zhang, Y., and Ye, G. (2018). A model for predicting the relative chloride diffusion coefficient in unsaturated cementitious materials. Cem. Concr. Res. 115, 133–144. doi:10.1016/j.cemconres.2018.10.013
Keywords: concrete crack repair, capillary absorption, ionic transport, ionic chelator, hydration products
Citation: Lu R and Guan X (2023) Transport properties of moisture and ionic chelators in concrete. Front. Mater. 10:1176873. doi: 10.3389/fmats.2023.1176873
Received: 01 March 2023; Accepted: 30 March 2023;
Published: 11 April 2023.
Edited by:
Muhan Wang, Qingdao University of Technology, ChinaReviewed by:
Zhiyong Liu, Southeast University, ChinaE. Chen, Chalmers University of Technology, Sweden
Copyright © 2023 Lu and Guan. This is an open-access article distributed under the terms of the Creative Commons Attribution License (CC BY). The use, distribution or reproduction in other forums is permitted, provided the original author(s) and the copyright owner(s) are credited and that the original publication in this journal is cited, in accordance with accepted academic practice. No use, distribution or reproduction is permitted which does not comply with these terms.
*Correspondence: Rongwei Lu, MTVCOTMzMDA3QGhpdC5lZHUuY24=