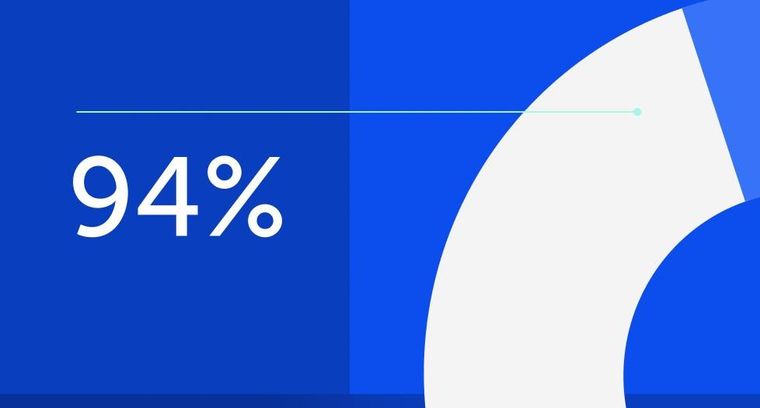
94% of researchers rate our articles as excellent or good
Learn more about the work of our research integrity team to safeguard the quality of each article we publish.
Find out more
REVIEW article
Front. Mater., 06 April 2023
Sec. Polymeric and Composite Materials
Volume 10 - 2023 | https://doi.org/10.3389/fmats.2023.1168616
This article is part of the Research TopicThe Role of Polymer Hydrogels in the Bio-Medical FieldView all 6 articles
Congenital and acquired tissular losses due to disease or trauma are a major world health problem. Regenerative therapy aims to fix damaged tissues by directing the natural capacity of a host organism to use biofunctionalized artificial tissue scaffolds. These three-dimensional (3D) scaffolds can be customized with cells and/or bioactive molecules to induce cellular homing and angiogenesis, essential to ensure successful tissue regeneration. Hydrogels (HGs) scaffolds are networks of hydrophilic homopolymers, copolymers, and/or macromers with chemical and biological activities that enhance their cell colonization. The use of HGs in regenerative medicine has shown to be advantageous since HGs can be prepared under clinical-grade conditions and tailored to the specific needs of the replaced tissue. They can be made to emulate native extracellular matrices (ECMs) including physical, mechanical, and chemical cues and resilience properties. These customized HGs can reproduce the natural hygroscopic capacity of the original tissue which improves cellular anchoring, nutrition, and waste disposal. They can enable host molecular and cellular modification conducive to a natural cellular microenvironment, modifying the properties of the scaffold, and improving chemotaxis, cell adhesion, migration, proliferation, differentiation, and angiogenesis; HGs can be created and biofunctionalized with linked growth factors and synthetic peptides tailored to positively influence scaffold colonization and functional biocompatibility. This review aims to collect the most relevant information regarding biofunctionalization of HGs used for vascular tissue regeneration, their biological effects, and their clinical implications. While most biofunctionalized HGs are still under investigation, some of them have been studied in vitro, ex vivo, and in vivo with promising results. In this regard, in vivo studies have shown that biofunctionalized scaffolds with peptides such as chitosan hydrogel with LL-37 promotes angiogenesis and healing of pressure ulcers. Also, the GHK tripeptide is widely used in trials focused on guided tissue remodeling.
During the last decades in the field of biomedicine, enormous efforts have been made to achieve therapies focused on restoring the function of tissues that have suffered critical tissue damage through surgical reconstruction, mechanical devices, or transplants that will provide them with a better quality of life (Klar et al., 2017; Adamowicz et al., 2021). However, the lack of donors and the lack of biocompatible biomaterials prompted the development of adequate and personalized therapies, restoring the function of the injured tissue and organ. These therapies must imitate almost entirely the extracellular matrix, allowing the passage of nutrients and oxygen, as well as the release of waste and metabolites typical of the products of reabsorption of the implemented materials (Ali et al., 2014; Lanza et al., 2020). Implementing in vitro tissue engineering allows to evaluate the cellular and molecular conditions of human physiology; these studies include 3D assays, which integrate physical, chemical, and mechanical signals, developing an environment similar to the niche to be studied, resulting in the understanding of the integral system, that is, cellular and molecular interactions enhancing invasion, proliferation, differentiation, and apoptosis (Härmä et al., 2014; Chen et al., 2017a). The ultimate purpose of tissue engineering is to evaluate the best strategies that achieve tissue restoration, either by maintaining or improving the function of damaged tissue and in very specific cases, even by replacing entire organs (Capuana et al., 2021; Manzini et al., 2021; Xue et al., 2021). In this sense, the generation of three-dimensional scaffolds of diverse properties has been achieved, according to the required purpose, and by now are successfully implemented in cosmetology, biomedicine, pharmacology, and tissue regeneration. In this regard, different types of scaffolds induce the restoration of damaged tissue function (Huang et al., 2017). These scaffolds are natural, synthetic, or hybrid substances, or combinations of substances that have the quality of interacting with biological systems (Zhu and Marchant, 2011). For instance, in clinical practice, autologous platelet lysate is commonly used, to achieve a rapid integration of an implant or graft, which minimizes surgical interventions and is free of immunological rejection (Chakar et al., 2015; Davies and Kuiper, 2019).
The biomaterials used for scaffolding can be designed based on electrospinning, self-assembly of peptides, aerogels, based on nanocellulose, crosslinking, and gelation (He et al., 2014; Ma et al., 2014; Carrow et al., 2015; Ding et al., 2019; Ferreira et al., 2020; Yang et al., 2021). A promising future are the scaffolds manufactured as HGs, as they are considered the best candidates in new tissue regeneration therapies, being capable of inducing changes in cellular processes such as chemotaxis, proliferation, angiogenesis, biomineralization, and expression of specific tissue biomarkers, enhancing the regeneration process (Wang et al., 2022b), they also can mimic the native structure of the ECM, allowing migration, vascularization, and tissue regeneration. The most common natural HGs used in biomedicine are based on agarose, alginate, chitosan, hyaluronan, fibrin, collagen, and extracellular matrix (ECM) (Varghese et al., 2020). On the other hand, synthetic HGs include Polylactic acid (PLA), polyethylene glycol (PEG), poly (2 hydroxyethyl methacrylate) (PHEMA), polyvinyl alcohol (vinyl alcohol) (PVA) (Munim and Raza, 2019). HGs have excellent physical, mechanical, chemical, and biological properties and can be obtained from synthetic, biological, or hybrid sources of origin (Moussa and Aparicio, 2019) (Figure 2). They can be used for the stimulation of cell enlargement, cell differentiation, and tissue formation (Wolf et al., 2012; Medberry et al., 2013), nevertheless, vascularization to support growth, functionalization, and tissue viability is a limitation for the implementation of these biomaterials (Loh and Choong, 2013; Reiffel et al., 2013; Cheng et al., 2015). Fortunately, HGs have now achieved promising results as scaffolds with enormous potential to induce vascularization (Loh and Choong, 2013; Fu et al., 2016; Elomaa et al., 2022). The success obtained so far is attributed to the fact that HGs follow the precepts of the triad in tissue regeneration (Wang et al., 2022b): A) a three-dimensional scaffold, B) bioactive molecules that allow modulation in cell development and function, and C) the cellular component that is imperative for the neoformation of tissues (Sakata et al., 2015). For this reason, these biomaterials can form 3D networks that allow a great uptake of fluids, promoting the diffusion of molecules and cells (Goker et al., 2019), modulating cell behavior, and influencing the results of biological, physical, and chemical remodeling, at the same time that it allows its progressive degradation (Chai et al., 2017; Bashari et al., 2018; Caldas et al., 2021). Therefore, HGs have great potential to induce angiogenic and vasculogenic processes, achieving complete tissue regeneration both in vitro and in vivo, when implemented as biomaterials to replace critical defects (Wang et al., 2020).
Nowadays, biomaterials also should be easily applied by means such as injection since this allows their application in vivo. HGs formulations meet that criterion as they are physical and chemical cross-linked hydrophilic scaffolds that can be biofunctionalized with biological molecules, and need a 3D environment like native ECM (Xu et al., 2017), thus HGs are currently considered the biomaterial of choice for tissue engineering applications given their characteristics that emulate the natural ECM, survival of cells, enabling proliferation and vascularization (Fan and Wang, 2017). In this regard, the optimization of HGs makes them capable of inducing vascularization and tissue regeneration for their successful application in regenerative medicine clinical trials. Therefore, this review aims to compile the most widely used HGs in tissue engineering and regenerative medicine, emphasizing those that have been studied for induction of vascularization, their biological effects, and their clinical implications.
Several properties can be analyzed when studying HGs. Understanding the physical properties of these biomaterials is important since treatments can be based on their resistance, compression, and elasticity. The type of raw material and the manufacturing procedure influence the properties of the HGs these can be derived from organic (including collagen, extracellular matrix, chitosan, silk, keratin, gelatin, hyaluronic acid, alginate, and agarose), synthetic (formulated from hydrophilic homopolymers, copolymers, and/or macromers and their derivatives, and peptides) or combinations better known as hybrid biomaterials (Wang et al., 2022b). These HGs can be potentiated and expand their use by conjugating with bioactive molecules and diverse cellular lines, complying with the principles of the triad in tissue regeneration, and achieving an ideal formulation of the organ or tissue that needs to be regenerated (Das and Basu, 2019). The formulation will depend on the physical, chemical and biological characteristics of the tissue to be restored, they can be synthesized in solid, semi-solid, mucoadhesive, bioadhesive or fluid hydrogel (Bashir et al., 2020). One of the most outstanding properties of HGs is the swelling capacity, which directly depends on the crosslinking density, due to the biomaterial to be synthesized, flexible, rigid, hydrophilic, or hydrophobic. Since in polymers with high crosslinking density the pore and surface area increase, producing the opposite in low density polymers, while rigid crosslinking increases thermostability compared to flexible crosslinking (Mane et al., 2015). The hydrolysis rate will strictly depend on the crosslinking density, since it decreases as the density increases, due to, the fact that they contain more hydrophobic monomers (Kim et al., 2015). While the increase in hydrophilicity improves and preserves the assembly of the scaffolds preserving their structure, which leads to the permeability or diffusion of bioactive molecules directly influencing the viability and proliferation of the cells within them (Wei et al., 2015). With properties of biocompatibility, biodegradable, non-toxic, which gives a responsible stimulus, also the different interactions act as a driving force that induces the self-assembly process, in oligopeptides and amino acids that are conjugated with aromatic groups which have the capacity for hydrogelation forming nanoscale, plate-shaped assemblies, including nanospheres and nanotubes (Tamamis et al., 2009). It is also known that, when these components are mixed with water or buffered solutions at a neutral pH, they induce co-assembly in supramolecular HGs by electrostatic attraction interactions (Fichman and Gazit, 2014).
The chemical characteristics will depend on the needs of each researcher; there are numerous methodologies to synthesize HGs. According to Akhtar, et al. methods for hydrogel synthesis can be divided into physical cross-linking and chemical cross-link (Akhtar et al., 2016) (Table 1); the most used method at present is the cross-linking by chemical reaction of complementary groups, either by aldehydes, addition or condensation reactions, high energy radiation, polymerization by free radicals or by enzymes. However, metabolites produced by scaffolds have been reported to cause toxicity. An alternative is the characterization of HGs physically crosslinked by hydrogen bonds, amphiphilic graft and block polymers and hydrophobized polysaccharides, there is also crosslinking by crystallization in homopolymer systems or by formation of stereo complexes ionic interactions and crosslinking by protein interaction, this to formulate scaffolds free from the toxicity effect caused by the crosslinking itself, all these will depend on the light source, regulation of temperature and pH (Singh and Lee, 2014). Other methods of gelation are photo-crosslinking and self-assembly (Carvalho and Mansur, 2017; Ferreira et al., 2018). While the manufacturing methods of tissue engineering scaffolds are divided into Conventional, Electrospinning, Casting, Three-dimensional printing, Combination of molding techniques and photolithography (Zhao et al., 2018). Their properties include water uptake, swelling capacity, thermal stability, and degradation rate (Kaczmarek et al., 2020).
TABLE 1. According to Akhtar, et al. methods for hydrogel synthesis can be divided into physical cross-linking and chemical cross-link (Akhtar et al., 2016).
To understand the chemical mechanisms of HGs, it is important to consider the critical role of intermolecular interactions, amino acid influences, peptide signals, and the effect of chirality (Cho et al., 2023). The effect of the interaction with amino acids has also been a crucial role in the formation of HGs (Nebot et al., 2012). For instance, the peptide Fmoc-Gly Phe which crystallizes and does not form gels, at <1% can forms HGs, which means that the oligopeptide sequence turns out to be crucial for the hydrogelation (Jayawarna et al., 2006). This change in self-assembly kinetics could be explained by the presence of aromatic moieties in Fmoc-Phe-Gly (Orbach et al., 2009). Similar behavior is also observed with the peptides Nap-Ala-Gly and Nap-Gly Ala since the alteration of the pH induces gelation in Nap-Gly-Ala, but crystallization in Nap-Ala-Gly (Adams et al., 2010). Chirality also plays a crucial role in hydrogelation providing a supramolecular architecture and consequently macroscopic properties (Smith, 2009). For example, the tripeptides DLeu-Phe-Phe, DPhe-Phe-Val, and DVal-Phe-Phe at physiological pH can form HGs, meanwhile, their natural counterparts of L-amino acids did not share the same characteristic, due to steric factors (Marchesan et al., 2012). The design of HGs biomaterials allows the self-assembly and crosslinking of oligopeptides in three-dimensional matrices in the form of HGs (DeVolder and Kong, 2012), gelling and integrating molecules or cells without affecting the physical, chemical, and biological properties, enabling biocompatibility. However, the ability to predict the probability of success in the formation of HGs is complicated, the slightest change in any of these factors directly and proportionally affects their architecture, impacting their molecular behavior, influencing the interactions with cells or in tissues (Ham et al., 2016), which require a large supply of nutrients and oxygen, as well as allowing the circulation of their own waste by metabolites products of tissue remodeling or regeneration, orchestrated by various signaling molecules and inducers of cell recruitment and proliferation in the area, as well as the self-degradation of the scaffold for the substitution of a tissue similar to the injured one (Gao et al., 2022). Hydrogels have all these characteristics, since, when used to fill critical defects in cartilage and bone, in direct infiltrates in myocardium, regeneration of the pancreas or as dressings in skin wounds, among others, they influence tissue regeneration, initially influencing the vascular network which allows optimal vascularization inducing guided tissue regeneration (Wang et al., 2022b).
The formation of blood vessels is orchestrated mainly by two different mechanisms: vasculogenesis and angiogenesis, the first occurs in the early stages of embryonic development, from hemangioblasts that will form the primitive vascular plexus and the heart (Dumitrescu et al., 2021), while angiogenesis is the formation of blood vessels from islets of blood by endothelial progenitor cells with the ability to differentiate into vascular cells (Chambers et al., 2021). However, although angiogenesis is the formation of postnatal blood vessels, it has a primary function of vascular remodeling to achieve vascular fusion, that is, the larger blood vessels are reduced and segmented to form primary and secondary vascular plexuses allowing their interconnection and circulation of the blood and its products (Yin et al., 2021; Nitzsche et al., 2022; Ribatti, 2022), inducing the formation of a hierarchical network known as the “Vascular Tree”, which includes capillaries, venules, arterioles, veins and arteries (de Silva et al., 2022; Ghanem, 2022).
Vascular remodeling is influenced by colony-forming endothelial cells (ECFC) in physiological conditions, which are mobilized from the bone marrow and enter the bloodstream searching signals for migration, proliferation, and differentiation (Meyer et al., 2021; Tian et al., 2022). This process is highly influenced by the biochemical and biophysical composition of the ECM and its derivatives such as growth factors (Khanna et al., 2021; Yu et al., 2021). The cells that have been most studied to evaluate these effects both in vitro and in vivo are the Human Umbilical Cord Vein Endothelial Cells (HUVEC), endothelial progenitors, and mesenchymal stem cells (MSCs), which are used to evaluate the formation of vascular vessels networks and the induction capacity of perfusive blood vessels (Jun et al., 2022; Ye et al., 2022). In this regard, one of the first inducing signals of remodeling is the hypoxic environment, which is considered to be the initiator of angiogenesis starting with hypoxia-inducible factor 1 alpha (HIF-1α) escaping degradation (Jun et al., 2022). In addition, the vascular endothelial growth factor (VEGF) is one of the key factors involved in all events since it induces cell migration, proliferation, differentiation, and survival, and it influences all stages from germination, maturation, and anastomosis of each of the new vessels formed (Yanev et al., 2022). Other important angiogenic growth factors include fibroblast growth factor (FGF) (Wazzani et al., 2021), platelet-derived growth factor (PDGF) (Huang et al., 2022), and hepatocyte growth factor (HGF) (Chhabra et al., 2022) (Figure 1).
FIGURE 1. The formation and remodeling of blood vessels begin at the angiogenesis stage in the embryonic process (A). Vasculogenesis begins in pregnant stages from blood islets, where vascular progenitor cells will differentiate into angioblasts, while in adult stages vascular progenitor cells will differentiate into pericytes supporting the physiological process of angiogenesis to form or renew blood vessels are formed or renewed from pre-existing vessels (B), Schematic illustration of the key growth factors and proteins and cells that stimulate these processes vasculogenesis and angiogenesis.
Among the most widely used therapies for vascular tissue regeneration, is cell therapy for autologous implants, which increases the probability of success of the treatment. However, this implies double surgical intervention (one in the donor area and the second in the recipient area) and is not functional in people with autoimmune syndromes, degenerative diseases, or immunodeficiencies (Leal et al., 2021; Park et al., 2022). This type of limitation provided the opportunity to merge different disciplines focused on designing, evaluating, and implementing new regeneration biomaterials including tissue engineering using three-dimensional scaffolds capable of providing support and structure to both native tissue and cells that will be recruited there. One of the main obstacles that tissue engineering must overcome in large-scale clinical applications is the optimal formation of a vascular network that allows the newly created tissue to be easily accepted by the host, and to have network support that allows the perfusion of both oxygen and nutrients and waste elimination (Vu et al., 2022). In this regard, synthesize biomaterials for tissue culture have proven to be excellent for vascular remodeling but their thicknesses of more than 500 µm reduce their application (Ma et al., 2022), as the cells migrate not more than 300 µm from the surface and then they become quiescent due to the low diffusion of oxygen and nutrients (Török et al., 2021).
The neoformation of blood vessels is mandatory for tissue regeneration, since it is the main step for the success of the new tissue functions, allowing rapid adaptation and providing microvessels that connect with the host’s existing vessels, achieving prompt homeostasis (HUA et al., 2021; Szklanny et al., 2021). The development of different techniques to convert embryonic cells into vascularized tissues enable the formation of specialized tissues and even mini-organs, which are capable of creating a vascular network when they are auto transplanted, but also when they are grafted onto extraembryonic membrane systems (Nahaboo et al., 2022). For example, the Chorioallantoic Membrane System (CAM), which is one of the most widely used assays for the study of vasculogenesis, allows easy handling and manipulation. In this system, the organs, cells, or biomaterials are placed on the membrane, slightly tearing the surface to generate a vascular connection (Demcisakova et al., 2022). However, this process is limited by extrinsic factors that affect it, such as the environment and the lack of a perfused fluid or microfluid that allows the circulation of nutrients and waste. In this sense, the neoformed blood vessels grown in three-dimensional scaffolds as an option for in vivo models (Marew and Birhanu, 2021; Vu et al., 2022) are designed to work together with the organs and the lymphatic system to transport nutrients and oxygen and eliminate waste directly from them (Hablitz and Nedergaard, 2021), this ensures that the tissues can be in optimal operating conditions (Vu et al., 2022).
Attempts have been made to formulate pre-vascularized tissues, cultivating on extracellular matrix scaffolds cell aggregates or synthetic analogs with endothelial cells that can spontaneously form vascular networks (Szklanny et al., 2021; Zhang et al., 2022a). These assays have shown that it is not necessary to place proangiogenic molecules to induce vasculogenesis, since endothelial cells can form primitive networks in an avascular tissue or environment (Subbiah et al., 2021). However, they are only capable of forming an immature network of vascular cords with a limited lumen, which reduces their ability to be perfused (Subbiah et al., 2021). Recent studies show a potentiated system performing a pool of cells involved in physiological angiogenesis co-culturing MSCs cells, mural cells, embryonic or umbilical cord fibroblasts, and endothelial cells on the scaffolds, achieving stable, mature, and vascular structures with large lumen that could be easily perfused at the time of implantation (Szklanny et al., 2021). This is relevant since a pre-vascularized scaffold could be implanted containing mural cells to regulate vascular permeability, achieving the distribution of blood and its components and reducing interstitial pressure, thus physiologically is translated into the decrease of edema (Claesson-Welsh, 2015; Laschke and Menger, 2016), achieving implant survival with a rapid connection to the native vasculature and excellent perfusion (Cui et al., 2019).
Angiogenesis plays an important role in the healing of injuries since it facilitates the transport of nutrients and oxygen to the site of the injury (Wang et al., 2022a), it is the most efficient and fastest way that injured tissue has to create new blood vessels that promote support for cellular functions related to the repair process (Liu et al., 2022). In the case of bone regeneration, angiogenesis is of vital importance since bone is a highly vascularized tissue, a characteristic that facilitates the interaction between blood vessels and bone cells helping to maintain integrity for the rehabilitation of deteriorated bone (Zhang et al., 2022b). Soluble growth factors are administered to tissue injury sites to promote angiogenesis, temporarily improving the function of adjacent cells and tissues; however, they are usually not very effective as they cannot be maintained at the injury site and their regenerative activity decreases since it is implemented as an infiltrate in solution allowing prompt absorption and elimination by the body. Hence, is important to create environments where these molecules can act more efficiently and in a prolonged manner. These environments can be given by HGs that function as scaffolds for tissue regeneration and have the ability to be functionalized (Xing et al., 2021).
HGs formulated from micro and nanomaterials are currently a viable platform aimed at various approaches from the sustained production of proteins and drugs, as well as the use of biomaterials in tissue engineering, due to their great affinity and biocompatibility both in vitro and in vivo, since they can be formulated with different structures allowing an adjustment of their porosity and size thanks to their high reactivity, excellent selectivity and mild reaction conditions (Liu et al., 2020). Microgels are composed of cross-linked water-soluble macromolecules; with dimensions on the order of 10 nm–100 μm. Which are small enough to allow injection, easily adapting to irregular body surfaces or used in membranes as biological barriers (Kittel et al., 2022). While Nanogels are defined as hydrophilic polymeric networks of nanometric size less than 100–200 nm in diameter. Induces cell recruitment through receptor-influenced endocytosis (Wang et al., 2017a). Providing the ability to deliver various drugs, from anti-carcinogens and peptides (Griffin et al., 2017; Li et al., 2021). Several reactions allow the formation of such microgels and nanogels, among them are the thiol-ene chemistry mediated by radicals, the photo-click chemistry of tetrazole-alkene, the oxime, the Dielse-Alder Reaction and the azide cycloaddition, strain-promoted alkyne (Palmese et al., 2019). These HGs have a unique characteristic since they have the capacity for bio-orthogonal formation, which does not interfere with bioactive encapsulations, or functionalization with drugs, proteins, or cells, but on the contrary, it potentiates biomimetic micropatterns, achieving functional micro and nanogels (Jiang et al., 2014).
The Thiol-Michael HGs based on thiolated hyaluronic acid (HA), thiolated gelatin, and Poly ethylene glycol diacrylate (PEGDA) have been investigated for the repair of ischemic myocardial infarctions and osteochondral defects, showing improved cell activity, propagation and viability (Toh et al., 2010; Xu et al., 2013). Hoffman et al. reported elevated fibroblast proliferation within hybrid microgel which contained thiolated heparin and PEGDA, resulting in increased addition of heparin-binding molecules such as fibrinogen (Tae et al., 2007). Similarly, chitosan-PEG hybrid HGs were developed to encapsulate cells such as smooth muscle, achieving cell survival (Kim et al., 2007; Tae et al., 2007). They have also been used to stimulate dermal fibroblast migration during wound repair assay using fibronectin-HA-PEG HGs (Yu et al., 2011). Engler et al. used thiol-functionalized HA HGs and PEGDA to assess temporal tissue stiffness changes during cardiac muscle development (Toh et al., 2010). One of the most outstanding studies is the one developed by Kao et al. where the gelatin PEG HG passed through the Thiol-Michael Reaction to formulate a three-dimensional scaffold that was also functionalized with MSCs to evaluate re-epithelialization in cutaneous wounds using a murine Biomodel of the Sprague Dawley strain, resulting in acceleration in wound closure and neovascularization (Xu et al., 2013). Finally, Filippi et al. formulated magnetic nanocomposite HGs, which stimulate the in vivo vasculogenic profile of cells derived from adipose tissue (Filippi et al., 2019).
To evaluate angiogenesis, it is necessary to carry out functional tests with scaffolds and biomaterials that can maintain biocompatibility and that promote the effect of cell differentiation and penetration to be used in preclinical trials. One of the most used tests in vitro is tubule formation, using three-dimensional scaffolds in gel formulation. Matrigel® is a scaffold formulated from mouse sarcoma basement membrane which contains ECM proteins, laminin, entactin, proteoglycans, heparan sulfate, and collagen (Godugu and Singh, 2016). The Geltrex® matrix in its different presentations is also used in both 2D and 3D tests (Godugu and Singh, 2016). AlgiMatrix™ is another scaffold that, when diluted with trisodium citrate, becomes a gel and has been studied in vitro assays for the characterization of spheroids (Godugu and Singh, 2016). HGs from rat tail collagen scaffolds or bovine tendons have also been accepted for various assays.
HGs have been biofunctionalized with various cell lines, either alone or in coculture, for the evaluation of angiogenesis and vasculogenesis. Ling et al. developed in vitro assays of a novel chitosan arginine HG functionalized with polydopamine (CA-pDA) with self-healing capacity observing, angiogenesis in vitro enhanced by CA-pDA HGs with HUVECs cells developing tubular network (Ling et al., 2021). The hypothesis is that L-Arg induces the production of NO, triggering the proliferation and migration of endothelial cells, where an increased expression of bFGF and VEGF was observed. In addition, they carried out in vivo model evaluations to analyze the enhanced antibacterial capacity to accelerate wound healing in the skin of the back in Sprague Dawley rats, observing great infiltration of fibroblasts and neovascularization at the wound site on day 7, a process that accelerated healing by deposition of granulation tissue and wound contraction (Ling et al., 2021). Cardiac regeneration has also made progress, since in situ cardiac regeneration was evaluated in BALB/c mice using substance P neuropeptide and IGF-1C peptide-releasing cardiac patches, demonstrating the capacity for neovascularization with capillaries positive for isolectin B2 and blood vessels positive for CD31 (Shafiq et al., 2018). Lovecchio et al. designed a dynamic culture platform that improves the efficiency of the Geltrex-based 3D culture-based tube formation assay which showed an activity of the angiogenic compound pro-(dual antiplatelet therapy [DAPT]) that accelerates and improves the formation of endothelial tubes by HUVEC in vitro at 3 h after culture (Lovecchio et al., 2020). Abdeen et al. evaluated the mechanical properties and protein composition of ECM that influences MSCs towards proangiogenic signaling, using a polyacrylamide hydrogel functionalized with fibronectin, collagen I, and laminin to 3D cultures of Matrigel in coculture with human microvascular endothelial cells (HMVEC). They showed that the degree of tubulogenesis depends on the matrix proteins that remain included and on the rigidity of the substrate, achieving a better effect than the growth factor cocktails that are available up to now (Abdeen et al., 2014).
This section enlists functionalized HGs with bioactive molecules. It can be found functionalized HGs with soluble molecules, exomes, extracellular components, growth factors, and peptides, which enhance tissue-specific regeneration.
It has been reported that the products of cell cultures, recovery of supernatants, and tissue maceration, among others, can be used in new assays focused on the modulation and development of new tissues, functionalizing them with various scaffolds, such is the case of Hsu et al. who used a cross-linked hydrogel containing a combination of gelatin and HA functionalized with recombinant human thrombomodulin (rhTM) in diabetic mice, exhibiting excellent mechanical properties, as well as great capacity for cell anchoring and adherence, inducing deposits of ordered collagen and granulation tissue, developing angiogenesis and re-epithelialization (Hsu et al., 2019). Ruehle et al. reported that in an in vitro model, they characterized a decorin-loaded collagen hydrogel (DCN) to determine the effects of 3D microvascular growth, resulting in an excellent inducer of vascular growth, fibrillogenesis, and vascular growth enhancer (Ruehle et al., 2017). Poranki et al. used keratin-based scaffolds applied to in vivo wound defects in a Yorkshire pig model demonstrating an efficient re-epithelialization rate, as well as excellent neovascularization in the damaged area (Poranki et al., 2016). Kim et al. used a Pectin + Polyvinyl Alcohol (PVA) hydrogel, which was loaded with Hippophaerhamnoides L, potentiating wound healing in a rat model. Furthermore, histological results revealed that there was re-epithelialization and orderly revascularization after 21 days post-application (Kim and Lee, 2017). Gurel et al. studied vasculogenesis-inducing biphasic scaffolds for bone tissue engineering, characterizing materials consisting of fibrous poly (lactide-co-glycolide) (PLGA) and poly (lactide-co-glycolide)-block-poly (ethylene glycol) block-poly (lactide-co-glycolide) (PLGA-PEG-PLGA), some loaded with VEGF and others with GS4012. In vitro, it was shown that the hydrogel induces cell proliferation, VEGF induction, and expression of Runx2, Col I, OC, and ALP genes in rat bone marrow mesenchymal stem cells (rBMSCs) promoters of osteogenesis. In vivo in a rat calvaria defect model, it was demonstrated that the biomaterial containing GS4012 improved vascular endothelial cell recruitment, increased vascularity, and allowed rapid and orderly bone healing (Gurel Pekozer et al., 2021).
Therapies focused on revascularization or neovascularization have growth factors as allies since they direct promote and modulate tissue regeneration in an orderly manner. Furthermore, by loading these growth factors into the HGs, the physiological needs of the injured tissues are further enhanced. VEGF is considered the gold standard in terms of vascularization as it promotes proliferation, migration, and lumen formation of endothelial cells, and also influences increased vascular permeability (Xu et al., 2018) (Hsieh et al., 2017). However, other factors also exhibit excellent results such as the formation of new capillary networks, capillary induction, and neovascularization. In this sense, Xu et al. developed a PVA hydrogel functionalized with platelet-rich fibrin granules for wound healing, showing that it promotes rapid re-epithelialization and notable neovascularization (Xu et al., 2018). Hsieh et al. used an in vivo biodegradable self-healing hydrogel in a model of limb ischemia in mice, formulated from chitosan and fibrin functionalized with VEGF, demonstrating the promotion of angiogenesis and neo-capillary formation at 15 days of infiltration (Hsieh et al., 2017). Samberg et al. optimized a polyethylene glycol (PEGylated) hydrogel, modified with platelet-rich plasma (PRP) and biofunctionalized with stem cells derived from human adipose tissue (ASC), to evaluate it in a rat skin excision model, the result was that it considerably increases the number of blood vessels improving wound healing (Samberg et al., 2019). Zhang et al. stimulated wound healing by bioinspired HGs with basic fibroblast growth factor (bFGF), resulting in improved cell proliferation, effective cell migration, wound re-epithelialization, collagen deposition, as well as neovascularization after 14 days (Zhang et al., 2018).
Peptides are small molecules that can conduct various functions, signaling through protein-protein interactions and interacting in essential functions for cellular bioprocesses. Being such small and stable molecules, they have been widely used in biomedical applications, and due to their versatility, they have raised the possibility of their use in future innovative applications, which makes them new promises in biotherapy (Apostolopoulos et al., 2021). They can be developed depending on their structure and the ligand or receptor to be stimulated, and because they are such small molecules, they are easy to synthesize, redesign and modify, which is why they are very safe, effective, and highly selective, showing low immunogenicity, making them excellent candidates for use in pharmacology, cell therapy and tissue engineering (Fosgerau and Hoffmann, 2015).
The main function of proangiogenic peptides is being a mimetic signal that can be recognized by the receptors of growth factors that trigger the formation of blood vessels (D'Andrea et al., 2009). Although there are many angiogenic peptides used in vitro and in vivo assays, such as QK, RoY, LL-37, PBA2-1c, Exendin-4, Peptide derivatives of Osteonectin (OPD, SPARC113, and SPARC118) and Peptide derivative of osteopontin (OPD), in this section we will include those that have been used to bio functionalize HGs.
Some peptides that mimic angiopoietin-1 can influence the modulation of angiogenesis in vivo. Three peptides have been synthesized to carry out this function, vasculotide, Comp Ang1, and the peptide derived from the integrin binding domain of Ang1 (Cho et al., 2004). Another peptide is PAB2-1C, a peptide mimetic of platelet-derived growth factor (PDGF) with angiogenic capacity (Lin et al., 2007). There is also the VEGFR1D2 sequence peptide, which exerts its proangiogenic activity by being recognized by the α5β1 integrin (Soro et al., 2008).
On the other hand, peptides not related to growth factors have also been used, as possible modulators of tissue regeneration or anaphylactic adjuvants which have triggered frank angiogenesis, such as the GHK peptide, which binds to the SPARC domain of Osteonectin (Pickart, 2008). GHK has a high affinity for copper, which has been shown to have the ability to restore blood flow in injured tissues by orchestrating vasodilation, anticoagulation, and angiogenesis, as well as significantly increasing the expression of basic fibroblast growth factor and endothelial growth factor at wound sites (Pickart, 2008). In vitro tests show that is a potent capillary endothelial cell chemoattractant (Zoughaib et al., 2021) and in vivo, in a rabbit model, it is capable of inducing angiogenesis in the cornea (Ali et al., 2013). Although these peptides have been studied in several areas, the ones that stand out the most are those focused on tissue regeneration and placed in vivo wound closure tests and very few of them have extended their functionalized use with HGs. However, there are conclusive results that demonstrate that the biofunctionalization of scaffolds with angiogenic peptides can modulate tissue repair in an orderly manner, starting with non-invasive and controlled angiogenesis. As is the case of Seow et al. who developed a hydrogel-based scaffold functionalizing them with a peptide sequence called LK6C, in a murine model in female SCID mice with a complete and thick excision wound, achieving excellent re-epithelialization and neovascularization (Seow et al., 2016).
Carrejo et al. manufactured a sucrose-based multidomain peptide hydrogel used for in vivo studies in a murine model of wound closure in diabetic rats, showing that has excellent biocompatibility with re-epithelialization capacity and hair follicle formation at day 21 post-inoculation, and with neurogenesis and angiogenesis after 14 days (Carrejo et al., 2018). There is also the antimicrobial peptide LL-37 which is the only host defense peptide derived from cathelicidin present in humans, demonstrating the effective elimination of multi-resistant pathogens (Steinstraesser et al., 2011). Yang Xu et al. studied a chitosan hydrogel encapsulated with LL-37 peptide to evaluate the healing of deep tissue injuries in a mouse model, demonstrating that the biofunctionalized scaffold with LL-37 induces the synthesis of anti-inflammatory and proangiogenic cytokines such as HIF-1α and VEGF-A in deep tissue lesions 21 days after implantation, allowing microcirculation and regeneration of damaged tissue (Lin et al., 2020), this finding is supported by the fact that the peptide LL-37 promotes lymphangiogenesis in lymphatic endothelial cells through the ERK and Akt signaling pathways (Yanagisawa et al., 2020). Also Tovar-Castillo et al. reported that LL-37 is expressed in human keratinocytes in skin lesions, promoting the induction of VEGF-A (Tovar-Castillo et al., 2007). Another peptide is the VEGF-derived peptide QK which was designed to mimic the structure of the 17–25 helical region of the proangiogenic factor (D'Andrea et al., 2005). This peptide is known to interact with receptors Kdr and Flt-1. On Matrigel scaffolds in vitro, it can induce the tubulogenesis of HUVECs and in vivo in murine biomodels in hindlimb ischemia and open wound assays (Santulli et al., 2009). Besides, Pal et al. designed double-crosslinked biohybrid injectable HGs based on poly (N-isopropylacrylamide) for vascularization, which were functionalized with peptide QK to recruit endothelial cells and support angiogenesis in an in vivo model showing that the injection of vasculogenic hydrogel (HG-PNGQK200), allowed the infiltration of endothelial cells and a cellular arrangement that allows the formation of capillaries and blood vessels that lead to the identification of a clearly defined lumen (Pal et al., 2020). Meanwhile, Flora et al. characterized an elastin-like recombinant (ELR) hydrogel anchored to peptide QK to enhance angiogenesis in vivo, demonstrating that the use of this hydrogel promotes and enhances angiogenesis 3 weeks after infiltration, forming prominent CD31-positive capillaries (Flora et al., 2019). More information regarding angiogenic peptides can be found in Table 2.
Clinical trials have confirmed that HGs are an effective therapy for various diseases such as vertebral arthrodesis, maxillofacial trauma, type 2 diabetes, and chronic kidney disease (McFetridge et al., 2018; Almawash et al., 2022; Øvrebø et al., 2022). At the same time, various types of HGs have been used in biomedicine to develop 3D models of multiple diseases (Ou and Hosseinkhani, 2014; Xue et al., 2019). Hydrogel-based scaffolds have also been explored in pathophysiological medicine for the study of pathogenesis, while in pharmacology they have been studied for their use as an ideal means to release drugs in a controlled and efficient manner (Ziemba and Gilbert, 2017; Ghane et al., 2020). HGs have been highlighted as favorable scaffolds for cell encapsulation and expansion in vitro and in vivo, allowing tissue regeneration and promoting vascularization with potential use in multiple pathologies (Cascone and Lamberti, 2020; Cao et al., 2021). Cell-free bioactive hydrogel-based scaffolds have been extensively tested as effective guides for tissue regeneration, with possible clinical application in wound dressings with positive effects on tissue regeneration, healing acceleration, and healing removal of the exudate from the wound (Smith et al., 2016; Ayala-Ham et al., 2021; Zhang et al., 2021; Zhu et al., 2021). In this sense, the development of HGs has provided excellent opportunities for the engineering of vascular analogues and the regeneration of vascularized tissues, so detailed research could promote the optimization of these biomaterials to ensure the application of hydrogel-based scaffolds in clinical trials tissue regeneration medicine (Wang et al., 2022b). In the case of commercial scaffolds, it is known that Geltrex contained the virus that elevates lactate dehydrogenase (LDEV), which causes conflict for certain applications, such as xenografts or other preclinical investigations (Aisenbrey and Murphy, 2020; Prasad et al., 2021). Different authors comment that alginate, collagen, fibrin, and polyethylene glycol (PEG) HGs, among others, have the characteristic of being resilient, providing distensibility similar to that of soft tissues; however, they are difficult to manipulate and have low physiological load. Also, it is difficult to encapsulate hydrophobic molecules on HGs, which makes it unfavorable for drug delivery (Su et al., 2019). In the case of HGs obtained by chemical means, they generally require an enzymatic initiator, which can produce adverse effects while it is metabolized or produces its residual products, causing cross-reactions with proteins of the scaffold, with encapsulated drugs and producing immunogenicity, this makes them non-specific materials in the area of biomedicine (Jiang et al., 2014). The manufacture of injectable HGs from micro and nanomaterials provides minimal invasion in clinical practice and a great capacity for filling and modeling irregular defects in critical lesions. HGs made from ceramic nanomaterials have been reported to provide a high surface-to-volume ratio, in which they can be load growth factors or various inducer proteins (Wang et al., 2017b). On the other hand, HGs have been characterized from natural and synthetic peptides that provide a more precise signal, producing a desirable molecular mimicry. In the case of biofunctionalized scaffolds with peptides, we found that the chitosan hydrogel with LL-37 which promotes angiogenesis and healing of pressure ulcers. However, the instability of this peptide within the wound niche limits its clinical use (Lin et al., 2020). In the same order is the GHK tripeptide, which used in trials focused on guided tissue remodeling; however, it has been reported that HGs formulated with GHK-CU induce extreme vasodilation, a non-physiological clinical process when using another type of scaffolds, this due to the activation of the tissue by the reduction of Cu, Zn superoxide dismutase that supplies copper ions, which causes accumulation of nitric oxide, the vasodilatory molecule, lengthening its action by protection of superoxide dismutase (Pickart, 2008).
The clinical implementation of hydrogel-based scaffolds depends on other factors such as patient-dependent parameters and material-specific therapeutic application methods, the quality and function analysis of which has not yet been optimized, so it could result in poor performance, incorrect methodology, and irregular administration of the dose of the material, resulting in the appearance of secondary effects such as fibrosis, inflammation, pain, joint leakage, and paracrine cell crosstalk; furthermore, the cellular behavior and molecular signaling associated with the hydrogel response have not yet been fully explored (Maisani et al., 2017; Radulescu et al., 2022). Besides, there are some unresolved limitations in the use of HGs as scaffolds in tissue regeneration and vascularization, such as design challenges and dimensions of the gel network, the presence of inert modules that can destabilize microporous and macroporous structures, as well as the difficulty of producing rational scaffold designs that can efficiently mimic the morphology of the extracellular matrix (Xu et al., 2022). Complementing and merging already established methodologies would provide us with new tools for understanding biomaterials that are biomimetic and inducers of vasculogenesis, as well as such as the adaptability of the scaffold without triggering undesirable immunological mechanisms.
Scaffolds characterized as HGs used for tissue regeneration focused on vasculogenesis, induce the incorporation of cells and functional molecules into their structures while allowing their degradation to make room for new healthy tissue. This provides great advantages for use in research and application in the biomedical area. These have a great capacity to absorb liquids, which allows and influences the transport of nutrients and waste, in addition to having the characteristics of being flexible and elastic, allowing adaptation in critical defects, whether in bone, skin, or another organ, and achieving a great mimicry with the native ECM. Scaffolds formulated in HGs can be biofunctionalized to influence molecular, cellular, and physiological behavior by modulating chemotaxis, proliferation, differentiation, and neovascularization.
This type of scaffolding is the subject of exhaustive research worldwide, which is why, more and more, different researchers are closing gaps in elucidating the mechanisms that biomaterials provide. In turn, new findings on tissue vascularization that are very promising for regenerative medicine emerge every day, which encourages preclinical trials. Nowadays, biofunctionalized scaffolds with peptides such as chitosan hydrogel with LL-37 promote angiogenesis and healing of pressure ulcers but their instability within the wound niche limits their clinical use (Lin et al., 2020). Also, the GHK tripeptide is widely used in trials focused on guided tissue remodeling; however, it has been reported that HGs formulated with GHK-CU induce extreme vasodilation, a non-physiological clinical process when using another type of scaffolds, this is due to the activation of the tissue by the reduction of Cu, Zn superoxide dismutase that supplies copper ions, which causes accumulation of nitric oxide, the vasodilatory molecule, lengthening its action by the protection of superoxide dismutase (Pickart, 2008). Therefore, tissue engineering requires characterized and optimized hydrogel-based scaffolds that can overcome existing limitations, to promote vascularization and achieve efficient tissue regeneration at preclinical and clinical levels.
JL-G, MB, RR-P, and MA-M conceived and designed the content of this review, AA-H, JL-G, GS-S, CV-M, HC-U, RR-P, and MB wrote the paper, JR-Q and HC-U contributed revising the paper critically for important intellectual content. All authors contributed to the final version of the manuscript.
The authors declare that the research was conducted in the absence of any commercial or financial relationships that could be construed as a potential conflict of interest.
All claims expressed in this article are solely those of the authors and do not necessarily represent those of their affiliated organizations, or those of the publisher, the editors and the reviewers. Any product that may be evaluated in this article, or claim that may be made by its manufacturer, is not guaranteed or endorsed by the publisher.
Abdeen, A. A., Weiss, J. B., Lee, J., and Kilian, K. A. (2014). Matrix composition and mechanics direct proangiogenic signaling from mesenchymal stem cells. Tissue Eng. Part A 20 (19-20), 2737–2745. doi:10.1089/ten.tea.2013.0661
Adamowicz, J., Kluth, L. A., Pokrywczynska, M., and Drewa, T. (2021). Tissue engineering and its potential to reduce prostate cancer treatment sequelae—narrative review. Front. Surg. 8, 644057. doi:10.3389/fsurg.2021.644057
Adams, D. J., Morris, K., Chen, L., Serpell, L. C., Bacsa, J., and Day, G. M. (2010). The delicate balance between gelation and crystallisation: Structural and computational investigations. Soft Matter 6 (17), 4144–4156. doi:10.1039/c0sm00409j
Aisenbrey, E. A., and Murphy, W. L. (2020). Synthetic alternatives to Matrigel. Nat. Rev. Mater. 5 (7), 539–551. doi:10.1038/s41578-020-0199-8
Akhtar, M. F., Hanif, M., and Ranjha, N. M. (2016). Methods of synthesis of hydrogels … A review, A review. Saudi Pharm. J. 24 (5), 554–559. doi:10.1016/j.jsps.2015.03.022
Ali, M. M., Li, F., Zhang, Z., Zhang, K., Kang, D-K., Ankrum, J. A., et al. (2014). Rolling circle amplification: A versatile tool for chemical biology, materials science and medicine. Chem. Soc. Rev. 43 (10), 3324–3341. doi:10.1039/c3cs60439j
Ali, S., Saik, J. E., Gould, D. J., Dickinson, M. E., and West, J. L. (2013). Immobilization of cell-adhesive laminin peptides in degradable PEGDA hydrogels influences endothelial cell tubulogenesis. BioResearch open access 2 (4), 241–249. doi:10.1089/biores.2013.0021
Almawash, S., Osman, S. K., Mustafa, G., and El Hamd, M. A. (2022). Current and future prospective of injectable hydrogels—design challenges and limitations. Pharmaceuticals 15 (3), 371. doi:10.3390/ph15030371
Apostolopoulos, V., Bojarska, J., Chai, T-T., Elnagdy, S., Kaczmarek, K., Matsoukas, J., et al. (2021). A global review on short peptides: Frontiers and perspectives. Molecules 26 (2), 430. doi:10.3390/molecules26020430
Ayala-Ham, A., López-Gutierrez, J., Bermúdez, M., Aguilar-Medina, M., Sarmiento-Sánchez, J. I., López-Camarillo, C., et al. (2021). Hydrogel-based scaffolds in oral tissue engineering. Front. Mater. 8, 708945. doi:10.3389/fmats.2021.708945
Bashari, A., Rouhani Shirvan, A., and Shakeri, M. (2018). Cellulose-based hydrogels for personal care products. Polym. Adv. Technol. 29 (12), 2853–2867. doi:10.1002/pat.4290
Bashir, S., Hina, M., Iqbal, J., Rajpar, A., Mujtaba, M., Alghamdi, N., et al. (2020). Fundamental concepts of hydrogels: Synthesis, properties, and their applications. Polymers 12 (11), 2702. doi:10.3390/polym12112702
Bloise, N., Rountree, I., Polucha, C., Montagna, G., Visai, L., Coulombe, K. L., et al. (2020). Engineering immunomodulatory biomaterials for regenerating the infarcted myocardium. Front. Bioeng. Biotechnol. 8, 292. doi:10.3389/fbioe.2020.00292
Caldas, B. S., Nunes, C. S., Panice, M. R., Scariot, D. B., Nakamura, C. V., and Muniz, E. C. (2021). Manufacturing micro/nano chitosan/chondroitin sulfate curcumin-loaded hydrogel in ionic liquid: A new biomaterial effective against cancer cells. Int. J. Biol. Macromol. 180, 88–96. doi:10.1016/j.ijbiomac.2021.02.194
Cao, H., Duan, L., Zhang, Y., Cao, J., and Zhang, K. (2021). Current hydrogel advances in physicochemical and biological response-driven biomedical application diversity. Signal Transduct. Target. Ther. 6 (1), 426. doi:10.1038/s41392-021-00830-x
Capuana, E., Lopresti, F., Carfì Pavia, F., Brucato, V., and La Carrubba, V. (2021). Solution-based processing for scaffold fabrication in tissue engineering applications: A brief review. Polymers 13 (13), 2041. doi:10.3390/polym13132041
Carrejo, N. C., Moore, A. N., Lopez Silva, T. L., Leach, D. G., Li, I-C., Walker, D. R., et al. (2018). Multidomain peptide hydrogel accelerates healing of full-thickness wounds in diabetic mice. ACS biomaterials Sci. Eng. 4 (4), 1386–1396. doi:10.1021/acsbiomaterials.8b00031
Carrow, J. K., Kerativitayanan, P., Jaiswal, M. K., Lokhande, G., and Gaharwar, A. K. (2015). Polymers for bioprinting. Essentials of 3D biofabrication and translation. Elsevier, 229–248.
Carvalho, I. C., and Mansur, H. S. (2017). Engineered 3D-scaffolds of photocrosslinked chitosan-gelatin hydrogel hybrids for chronic wound dressings and regeneration. Mater. Sci. Eng. C 78, 690–705. doi:10.1016/j.msec.2017.04.126
Cascone, S., and Lamberti, G. (2020). Hydrogel-based commercial products for biomedical applications: A review. Int. J. Pharm. 573, 118803. doi:10.1016/j.ijpharm.2019.118803
Chai, Q., Jiao, Y., and Yu, X. (2017). Hydrogels for biomedical applications: Their characteristics and the mechanisms behind them. Gels 3 (1), 6. doi:10.3390/gels3010006
Chakar, C., Soffer, E., Cohen, N., Petite, H., Naaman, N., and Anagnostou, F. (2015). Vertical bone regeneration with deproteinised bovine bone mineral or biphasic calcium phosphate in the rabbit calvarium: Effect of autologous platelet lysate. J. Mater. Sci. Mater. Med. 26 (1), 23–29. doi:10.1007/s10856-014-5339-5
Chambers, S. E., Pathak, V., Pedrini, E., Soret, L., Gendron, N., Guerin, C. L., et al. (2021). Current concepts on endothelial stem cells definition, location, and markers. Stem cells Transl. Med. 10 (S2), S54–S61. doi:10.1002/sctm.21-0022
Chen, G., Zhou, D., Li, X-Z., Jiang, Z., Tan, C., Wei, X-Y., et al. (2017). A natural chalcone induces apoptosis in lung cancer cells: 3D-QSAR, docking and an in vivo/vitro assay. Sci. Rep. 7 (1), 10729–10810. doi:10.1038/s41598-017-11369-9
Chen, X., Zhang, M., Wang, X., Chen, Y., Yan, Y., Zhang, L., et al. (2017). Peptide-modified chitosan hydrogels promote skin wound healing by enhancing wound angiogenesis and inhibiting inflammation. Am. J. Transl. Res. 9 (5), 2352–2362.
Cheng, P., Bai, H., Zawacka, N., Andersen, T., Liu, W., Bundgaard, E., et al. (2015). Advanced science. Weinheim, Germany: Baden-Wurttemberg.
Chhabra, A., Song, H-H. G., Grzelak, K. A., Polacheck, W. J., Fleming, H. E., Chen, C. S., et al. (2022). A vascularized model of the human liver mimics regenerative responses. Proc. Natl. Acad. Sci. 119 (28), e2115867119. doi:10.1073/pnas.2115867119
Cho, C-H., Kammerer, R. A., Lee, H. J., Steinmetz, M. O., Ryu, Y. S., Lee, S. H., et al. (2004). COMP-Ang1: A designed angiopoietin-1 variant with nonleaky angiogenic activity. Proc. Natl. Acad. Sci. 101 (15), 5547–5552. doi:10.1073/pnas.0307574101
Cho, N. H., Guerrero-Martínez, A., Ma, J., Bals, S., Kotov, N. A., Liz-Marzán, L. M., et al. (2023). Bioinspired chiral inorganic nanomaterials. Nat. Rev. Bioeng. 1 (2), 88–106. doi:10.1038/s44222-022-00014-4
Claesson-Welsh, L. (2015). Vascular permeability—The essentials. Upsala J. Med. Sci. 120 (3), 135–143. doi:10.3109/03009734.2015.1064501
Cui, H., Zhu, W., Huang, Y., Liu, C., Yu, Z-X., Nowicki, M., et al. (2019). In vitro and in vivo evaluation of 3D bioprinted small-diameter vasculature with smooth muscle and endothelium. Biofabrication 12 (1), 015004. doi:10.1088/1758-5090/ab402c
D'Andrea, L. D., Del Gatto, A., De Rosa, L., Romanelli, A., and Pedone, C. (2009). Peptides targeting angiogenesis related growth factor receptors. Curr. Pharm. Des. 15 (21), 2414–2429. doi:10.2174/138161209788682235
D'Andrea, L. D., Iaccarino, G., Fattorusso, R., Sorriento, D., Carannante, C., Capasso, D., et al. (2005). Targeting angiogenesis: Structural characterization and biological properties of a de novo engineered VEGF mimicking peptide. Proc. Natl. Acad. Sci. 102 (40), 14215–14220. doi:10.1073/pnas.0505047102
Das, S., and Basu, B. (2019). An overview of hydrogel-based bioinks for 3D bioprinting of soft tissues. J. Indian Inst. Sci. 99, 405–428. doi:10.1007/s41745-019-00129-5
Davies, R. L., and Kuiper, N. J. (2019). Regenerative medicine: A review of the evolution of autologous chondrocyte implantation (ACI) therapy. Bioengineering 6 (1), 22. doi:10.3390/bioengineering6010022
de Silva, L., Bernal, P. N., Rosenberg, A., Malda, J., Levato, R., and Gawlitta, D. (2022). Biofabricating the vascular tree in engineered bone tissue. Acta Biomater. 156, 250–268. doi:10.1016/j.actbio.2022.08.051
Demcisakova, Z., Luptakova, L., Tirpakova, Z., Kvasilova, A., Medvecky, L., De Spiegelaere, W., et al. (2022). Evaluation of angiogenesis in an acellular porous biomaterial based on polyhydroxybutyrate and chitosan using the chicken ex ovo chorioallantoic membrane model. Cancers 14 (17), 4194. doi:10.3390/cancers14174194
Deng, B., Shen, L., Wu, Y., Shen, Y., Ding, X., Lu, S., et al. (2015). Delivery of alginate-chitosan hydrogel promotes endogenous repair and preserves cardiac function in rats with myocardial infarction. J. Biomed. Mater. Res. Part A 103 (3), 907–918. doi:10.1002/jbm.a.35232
DeVolder, R., and Kong, H. J. (2012). Hydrogels for in vivo-like three-dimensional cellular studies. Wiley Interdiscip. Rev. Syst. Biol. Med. 4 (4), 351–365. doi:10.1002/wsbm.1174
Ding, J., Zhang, J., Li, J., Li, D., Xiao, C., Xiao, H., et al. (2019). Electrospun polymer biomaterials. Prog. Polym. Sci. 90, 1–34. doi:10.1016/j.progpolymsci.2019.01.002
Dumitrescu, A. M., Costea, C. F., Furnică, C., Turliuc, M. D., Cucu, A. I., Bogdănici, C. M., et al. (2021). Morphological aspects of the vasculogenesis and angiogenesis during prenatal edification of the circle of willis: A review. Romanian J. Morphol. Embryology 62 (3), 679–687. doi:10.47162/RJME.62.3.04
Elomaa, L., Lindner, M., Leben, R., Niesner, R., and Weinhart, M. (2022). In vitro vascularization of hydrogel-based tissue constructs via a combined approach of cell sheet engineering and dynamic perfusion cell culture. Biofabrication 15 (1), 015004. doi:10.1088/1758-5090/ac9433
Fan, C., and Wang, D-A. (2017). Macroporous hydrogel scaffolds for three-dimensional cell culture and tissue engineering. Tissue Eng. Part B Rev. 23 (5), 451–461. doi:10.1089/ten.teb.2016.0465
Ferreira, F. V., Otoni, C. G., Kevin, J., Barud, H. S., Lona, L. M., Cranston, E. D., et al. (2020). Porous nanocellulose gels and foams: Breakthrough status in the development of scaffolds for tissue engineering. Mater. Today 37, 126–141. doi:10.1016/j.mattod.2020.03.003
Ferreira, N., Ferreira, L., Cardoso, V., Boni, F., Souza, A., and Gremião, M. (2018). Recent advances in smart hydrogels for biomedical applications: From self-assembly to functional approaches. Eur. Polym. J. 99, 117–133. doi:10.1016/j.eurpolymj.2017.12.004
Fichman, G., and Gazit, E. (2014). Self-assembly of short peptides to form hydrogels: Design of building blocks, physical properties and technological applications. Acta biomater. 10 (4), 1671–1682. doi:10.1016/j.actbio.2013.08.013
Filippi, M., Dasen, B., Guerrero, J., Garello, F., Isu, G., Born, G., et al. (2019). Magnetic nanocomposite hydrogels and static magnetic field stimulate the osteoblastic and vasculogenic profile of adipose-derived cells. Biomaterials 223, 119468. doi:10.1016/j.biomaterials.2019.119468
Flora, T., de Torre, I. G., Alonso, M., and Rodríguez-Cabello, J. C. (2019). Tethering QK peptide to enhance angiogenesis in elastin-like recombinamer (ELR) hydrogels. J. Mater. Sci. Mater. Med. 30 (2), 30–12. doi:10.1007/s10856-019-6232-z
Fosgerau, K., and Hoffmann, T. (2015). Peptide therapeutics: Current status and future directions. Drug Discov. today 20 (1), 122–128. doi:10.1016/j.drudis.2014.10.003
Fu, J., Fan, C., Lai, W. S., and Wang, D. (2016). Enhancing vascularization of a gelatin-based micro-cavitary hydrogel by increasing the density of the micro-cavities. Biomed. Mater. 11 (5), 055012. doi:10.1088/1748-6041/11/5/055012
Gao, E., Li, G., Cao, R., Xia, H., Xu, Y., Jiang, G., et al. (2022). Bionic tracheal tissue regeneration using a ring-shaped scaffold comprised of decellularized cartilaginous matrix and silk fibroin. Compos. Part B Eng. 229, 109470. doi:10.1016/j.compositesb.2021.109470
Ghane, N., Beigi, M-H., Labbaf, S., Nasr-Esfahani, M-H., and Kiani, A. (2020). Design of hydrogel-based scaffolds for the treatment of spinal cord injuries. J. Mater. Chem. B 8 (47), 10712–10738. doi:10.1039/d0tb01842b
Ghanem, A. N. (2022). Capillary ultrastructure anatomy and physiology: What is known, what is unknown or missing, what is wrong and what is new? GSC Adv. Res. Rev. 10 (2), 073–094. doi:10.30574/gscarr.2022.10.2.0049
Godugu, C., and Singh, M. (2016). AlgiMatrix™-based 3D cell culture system as an in vitro tumor model: An important tool in cancer research. Cancer Chemoprevention: Springer. 117–128.
Goker, F., Larsson, L., Del Fabbro, M., and Asa’ad, F. (2019). Gene delivery therapeutics in the treatment of periodontitis and peri-implantitis: A state of the art review. Int. J. Mol. Sci. 20 (14), 3551. doi:10.3390/ijms20143551
Griffin, J. I., Cheng, S. K. K., Hayashi, T., Carson, D., Saraswathy, M., Nair, D. P., et al. (2017). Cell-penetrating peptide CGKRK mediates efficient and widespread targeting of bladder mucosa following focal injury. Nanomedicine Nanotechnol. Biol. Med. 13 (6), 1925–1932. doi:10.1016/j.nano.2017.04.004
Gurel Pekozer, G., Abay Akar, N., Cumbul, A., Beyzadeoglu, T., and Torun Kose, G. (2021). Investigation of vasculogenesis inducing biphasic scaffolds for bone tissue engineering. ACS Biomaterials Sci. Eng. 7 (4), 1526–1538. doi:10.1021/acsbiomaterials.0c01071
Hablitz, L. M., and Nedergaard, M. (2021). The glymphatic system: A novel component of fundamental neurobiology. J. Neurosci. 41 (37), 7698–7711. doi:10.1523/jneurosci.0619-21.2021
Ham, T. R., Lee, R. T., Han, S., Haque, S., Vodovotz, Y., Gu, J., et al. (2016). Tunable keratin hydrogels for controlled erosion and growth factor delivery. Biomacromolecules 17 (1), 225–236. doi:10.1021/acs.biomac.5b01328
Härmä, V., Schukov, H-P., Happonen, A., Ahonen, I., Virtanen, J., Siitari, H., et al. (2014). Quantification of dynamic morphological drug responses in 3D organotypic cell cultures by automated image analysis. PloS one 9 (5), e96426. doi:10.1371/journal.pone.0096426
He, B., Yuan, X., and Jiang, D. (2014). Molecular self-assembly guides the fabrication of peptide nanofiber scaffolds for nerve repair. RSC Adv. 4 (45), 23610–23621. doi:10.1039/c4ra01826e
Hsieh, F-Y., Tao, L., Wei, Y., and Hsu, S-H. (2017). A novel biodegradable self-healing hydrogel to induce blood capillary formation. Npg Asia Mater. 9 (3), e363–e. doi:10.1038/am.2017.23
Hsu, Y-Y., Liu, K-L., Yeh, H-H., Lin, H-R., Wu, H-L., and Tsai, J-C. (2019). Sustained release of recombinant thrombomodulin from cross-linked gelatin/hyaluronic acid hydrogels potentiate wound healing in diabetic mice. Eur. J. Pharm. Biopharm. 135, 61–71. doi:10.1016/j.ejpb.2018.12.007
Hua, X., Ding, R., Ke, J., Ding, Z., Liu, P., and Zheng, X. (2021). Microsurgical anatomy of the arterial network of submental flap. Chin. J. Microsurg., 56–59.
Huang, M., Yang, F., Zhang, D., Lin, M., Duan, H., El-Mayta, R., et al. (2022). Endothelial plasticity drives aberrant vascularization and impedes cardiac repair after myocardial infarction. Nat. Cardiovasc. Res. 1 (4), 372–388. doi:10.1038/s44161-022-00047-3
Huang, Y., Zhang, X. F., Gao, G., Yonezawa, T., and Cui, X. (2017). 3D bioprinting and the current applications in tissue engineering. Biotechnol. J. 12 (8), 1600734. doi:10.1002/biot.201600734
Jayawarna, V., Ali, M., Jowitt, T. A., Miller, A. F., Saiani, A., Gough, J. E., et al. (2006). Nanostructured hydrogels for three-dimensional cell culture through self-assembly of fluorenylmethoxycarbonyl–dipeptides. Adv. Mater. 18 (5), 611–614. doi:10.1002/adma.200501522
Ji, X., Yuan, X., Ma, L., Bi, B., Zhu, H., Lei, Z., et al. (2020). Mesenchymal stem cell-loaded thermosensitive hydroxypropyl chitin hydrogel combined with a three-dimensional-printed poly (ε-caprolactone)/nano-hydroxyapatite scaffold to repair bone defects via osteogenesis, angiogenesis and immunomodulation. Theranostics 10 (2), 725–740. doi:10.7150/thno.39167
Jiang, Y., Chen, J., Deng, C., Suuronen, E. J., and Zhong, Z. (2014). Click hydrogels, microgels and nanogels: Emerging platforms for drug delivery and tissue engineering. Biomaterials 35 (18), 4969–4985. doi:10.1016/j.biomaterials.2014.03.001
Jun, J. H., Park, S., Kim, J. Y., Lim, J-Y., Park, G. T., Kim, J. H., et al. (2022). Combination therapy of placenta-derived mesenchymal stem cells with WKYMVm promotes hepatic function in a rat model with hepatic disease via vascular remodeling. Cells 11 (2), 232. doi:10.3390/cells11020232
Kaczmarek, B., Nadolna, K., and Owczarek, A. (2020). The physical and chemical properties of hydrogels based on natural polymers. Hydrogels based on natural polymers, 151–172.
Khanna, A., Zamani, M., and Huang, N. F. (2021). Extracellular matrix-based biomaterials for cardiovascular tissue engineering. J. Cardiovasc. Dev. Dis. 8 (11), 137. doi:10.3390/jcdd8110137
Kim, J., and Lee, C-M. (2017). Wound healing potential of a polyvinyl alcohol-blended pectin hydrogel containing Hippophae rahmnoides L. extract in a rat model. Int. J. Biol. Macromol. 99, 586–593. doi:10.1016/j.ijbiomac.2017.03.014
Kim, M. S., Choi, Y. J., Noh, I., and Tae, G. (2007). Synthesis and characterization of in situ chitosan-based hydrogel via grafting of carboxyethyl acrylate. J. Biomed. Mater. Res. Part A Official J. Soc. Biomaterials 83 (3), 674–682. The Japanese Society for Biomaterials, and The Australian Society for Biomaterials and the Korean Society for Biomaterials. doi:10.1002/jbm.a.31278
Kim, S., Linker, O., Garth, K., and Carter, K. R. (2015). Degradation kinetics of acid-sensitive hydrogels. Polym. Degrad. Stab. 121, 303–310. doi:10.1016/j.polymdegradstab.2015.09.014
Kittel, Y., Kuehne, A. J., and De Laporte, L. (2022). Translating therapeutic microgels into clinical applications. Adv. Healthc. Mater. 11 (6), 2101989. doi:10.1002/adhm.202101989
Klar, A. S., Zimoch, J., and Biedermann, T. (2017). Skin tissue engineering: Application of adipose-derived stem cells. BioMed Res. Int. 2017, 1–12. doi:10.1155/2017/9747010
Kuss, M. A., Wu, S., Wang, Y., Untrauer, J. B., Li, W., Lim, J. Y., et al. (2018). Prevascularization of 3D printed bone scaffolds by bioactive hydrogels and cell co-culture. J. Biomed. Mater. Res. Part B Appl. Biomaterials 106 (5), 1788–1798. doi:10.1002/jbm.b.33994
Lanza, R., Langer, R., Vacanti, J. P., and Atala, A. (2020). Principles of tissue engineering. Academic Press.
Laschke, M. W., and Menger, M. D. (2016). Prevascularization in tissue engineering: Current concepts and future directions. Biotechnol. Adv. 34 (2), 112–121. doi:10.1016/j.biotechadv.2015.12.004
Leal, B. B., Wakabayashi, N., Oyama, K., Kamiya, H., Braghirolli, D. I., and Pranke, P. (2021). Vascular tissue engineering: Polymers and methodologies for small caliber vascular grafts. Front. Cardiovasc. Med. 7, 592361. doi:10.3389/fcvm.2020.592361
Li, Y., Xu, T., Tu, Z., Dai, W., Xue, Y., Tang, C., et al. (2020). Bioactive antibacterial silica-based nanocomposites hydrogel scaffolds with high angiogenesis for promoting diabetic wound healing and skin repair. Theranostics 10 (11), 4929–4943. doi:10.7150/thno.41839
Li, Z., Huang, J., and Wu, J. (2021). pH-Sensitive nanogels for drug delivery in cancer therapy. Biomaterials Sci. 9 (3), 574–589. doi:10.1039/d0bm01729a
Lin, X., Takahashi, K., Liu, Y., Derrien, A., and Zamora, P. O. (2007). A synthetic, bioactive PDGF mimetic with binding to both α-PDGF and β-PDGF receptors. Growth factors. 25 (2), 87–93. doi:10.1080/08977190701553449
Lin, Z., Li, R., Liu, Y., Zhao, Y., Ao, N., Wang, J., et al. (2020). Histatin1-modified thiolated chitosan hydrogels enhance wound healing by accelerating cell adhesion, migration and angiogenesis. Carbohydr. Polym. 230, 115710. doi:10.1016/j.carbpol.2019.115710
Ling, Z., Chen, Z., Deng, J., Wang, Y., Yuan, B., Yang, X., et al. (2021). A novel self-healing polydopamine-functionalized chitosan-arginine hydrogel with enhanced angiogenic and antibacterial activities for accelerating skin wound healing. Chem. Eng. J. 420, 130302. doi:10.1016/j.cej.2021.130302
Liu, R., Dai, L., Xu, C., Wang, K., Zheng, C., and Si, C. (2020). Lignin-based micro-and nanomaterials and their composites in biomedical applications. ChemSusChem 13 (17), 4266–4283. doi:10.1002/cssc.202000783
Liu, Y., Hu, Q., Dong, W., Liu, S., Zhang, H., and Gu, Y. (2022). Alginate/gelatin-based hydrogel with soy protein/peptide powder for 3D printing tissue-engineering scaffolds to promote angiogenesis. Macromol. Biosci. 22 (4), 2100413. doi:10.1002/mabi.202100413
Loh, Q. L., and Choong, C. (2013). Three-dimensional scaffolds for tissue engineering applications: Role of porosity and pore size.
Lovecchio, J., Pannella, M., Giardino, L., Calzà, L., and Giordano, E. (2020). A dynamic culture platform enhances the efficiency of the 3D HUVEC-based tube formation assay. Biotechnol. Bioeng. 117 (3), 789–797. doi:10.1002/bit.27227
Lu, J., Guan, F., Cui, F., Sun, X., Zhao, L., Wang, Y., et al. (2019). Enhanced angiogenesis by the hyaluronic acid hydrogels immobilized with a VEGF mimetic peptide in a traumatic brain injury model in rats. Regen. Biomater. 6 (6), 325–334. doi:10.1093/rb/rbz027
Ma, B., Wang, X., Wu, C., and Chang, J. (2014). Crosslinking strategies for preparation of extracellular matrix-derived cardiovascular scaffolds. Regen. Biomater. 1 (1), 81–89. doi:10.1093/rb/rbu009
Ma, C., Li, W., Li, D., Chen, M., Wang, M., Jiang, L., et al. (2022). Photoacoustic imaging of 3D-printed vascular networks. Biofabrication 14 (2), 025001. doi:10.1088/1758-5090/ac49d5
Maisani, M., Pezzoli, D., Chassande, O., and Mantovani, D. (2017). Cellularizing hydrogel-based scaffolds to repair bone tissue: How to create a physiologically relevant micro-environment? J. Tissue Eng. 8, 204173141771207. doi:10.1177/2041731417712073
Mane, S., Ponrathnam, S., and Chavan, N. (2015). Effect of chemical cross-linking on properties of polymer microbeads: A review. Can. Chem. Trans. 3 (4), 473–485.
Manzini, B. M., Machado, L. M. R., Noritomi, P. Y., and da Silva, J. V. L. (2021). Advances in bone tissue engineering: A fundamental review. J. Biosci. 46 (1), 17–18. doi:10.1007/s12038-020-00122-6
Marchesan, S., Waddington, L., Easton, C. D., Winkler, D. A., Goodall, L., Forsythe, J., et al. (2012). Unzipping the role of chirality in nanoscale self-assembly of tripeptide hydrogels. Nanoscale 4 (21), 6752–6760. doi:10.1039/c2nr32006a
Marew, T., and Birhanu, G. (2021). Three dimensional printed nanostructure biomaterials for bone tissue engineering. Regen. Ther. 18, 102–111. doi:10.1016/j.reth.2021.05.001
McFetridge, M. L., Del Borgo, M. P., Aguilar, M-I., and Ricardo, S. D. (2018). The use of hydrogels for cell-based treatment of chronic kidney disease. Clin. Sci. 132 (17), 1977–1994. doi:10.1042/cs20180434
Medberry, C. J., Crapo, P. M., Siu, B. F., Carruthers, C. A., Wolf, M. T., Nagarkar, S. P., et al. (2013). Hydrogels derived from central nervous system extracellular matrix. Biomaterials 34 (4), 1033–1040. doi:10.1016/j.biomaterials.2012.10.062
Meyer, N., Brodowski, L., Richter, K., von Kaisenberg, C. S., Schröder-Heurich, B., and von Versen-Höynck, F. (2021). Pravastatin promotes endothelial colony-forming cell function, angiogenic signaling and protein expression in vitro. J. Clin. Med. 10 (2), 183. doi:10.3390/jcm10020183
Moussa, D. G., and Aparicio, C. (2019). Present and future of tissue engineering scaffolds for dentin-pulp complex regeneration. J. tissue Eng. Regen. Med. 13 (1), 58–75. doi:10.1002/term.2769
Munim, S. A., and Raza, Z. A. (2019). Poly (lactic acid) based hydrogels: Formation, characteristics and biomedical applications. J. Porous Mater. 26 (3), 881–901. doi:10.1007/s10934-018-0687-z
Nahaboo, W., Eski, S. E., Despin-Guitard, E., Vermeersch, M., Versaevel, M., Saykali, B., et al. (2022). Keratin filaments mediate the expansion of extra-embryonic membranes in the post-gastrulation mouse embryo. EMBO J. 41 (7), e108747. doi:10.15252/embj.2021108747
Nebot, V. J., Armengol, J., Smets, J., Prieto, S. F., Escuder, B., and Miravet, J. F. (2012). Molecular hydrogels from bolaform amino acid derivatives: A structure–properties study based on the thermodynamics of gel solubilization. Chemistry–A Eur. J. 18 (13), 4063–4072. doi:10.1002/chem.201103193
Nitzsche, B., Rong, W. W., Goede, A., Hoffmann, B., Scarpa, F., Kuebler, W. M., et al. (2022). Coalescent angiogenesis—Evidence for a novel concept of vascular network maturation. Angiogenesis 25 (1), 35–45. doi:10.1007/s10456-021-09824-3
Orbach, R., Adler-Abramovich, L., Zigerson, S., Mironi-Harpaz, I., Seliktar, D., and Gazit, E. (2009). Self-assembled Fmoc-peptides as a platform for the formation of nanostructures and hydrogels. Biomacromolecules 10 (9), 2646–2651. doi:10.1021/bm900584m
Ou, K-L., and Hosseinkhani, H. (2014). Development of 3D in vitro technology for medical applications. Int. J. Mol. Sci. 15 (10), 17938–17962. doi:10.3390/ijms151017938
Øvrebø, Ø., Perale, G., Wojciechowski, J. P., Echalier, C., Jeffers, J. R., Stevens, M. M., et al. (2022). Design and clinical application of injectable hydrogels for musculoskeletal therapy. Bioeng. Transl. Med. 7 (2), e10295. doi:10.1002/btm2.10295
Pal, A., Smith, C. I., Palade, J., Nagaraju, S., Alarcon-Benedetto, B. A., Kilbourne, J., et al. (2020). Poly (N-isopropylacrylamide)-based dual-crosslinking biohybrid injectable hydrogels for vascularization. Acta Biomater. 107, 138–151. doi:10.1016/j.actbio.2020.02.041
Palmese, L. L., Thapa, R. K., Sullivan, M. O., and Kiick, K. L. (2019). Hybrid hydrogels for biomedical applications. Curr. Opin. Chem. Eng. 24, 143–157. doi:10.1016/j.coche.2019.02.010
Park, S-S., Park, M., and Lee, B-T. (2022). Autologous stromal vascular fraction-loaded hyaluronic acid/gelatin-biphasic calcium phosphate scaffold for bone tissue regeneration. Mater. Sci. Eng. C 132, 112533. doi:10.1016/j.msec.2021.112533
Pickart, L. (2008). The human tri-peptide GHK and tissue remodeling. J. Biomaterials Sci. Polym. Ed. 19 (8), 969–988. doi:10.1163/156856208784909435
Poranki, D., Goodwin, C., and Van Dyke, M. (2016). Assessment of deep partial thickness burn treatment with keratin biomaterial hydrogels in a swine model. BioMed Res. Int. 2016, 1–10. doi:10.1155/2016/1803912
Prasad, M., Kumar, R., Buragohain, L., Kumari, A., and Ghosh, M. (2021). Organoid technology: A reliable developmental biology tool for organ-specific nanotoxicity evaluation. Front. Cell Dev. Biol. 9, 696668. doi:10.3389/fcell.2021.696668
Radulescu, D-M., Neacsu, I. A., Grumezescu, A-M., and Andronescu, E. (2022). New insights of scaffolds based on hydrogels in tissue engineering. Polymers 14 (4), 799. doi:10.3390/polym14040799
Reiffel, A. J., Perez, J. L., Fullerton, N., Lekic, N., Campbell, R., and Spector, J. A. (2013). Vascular smooth muscle cell optimization of vasculogenesis within naturally derived, biodegradable hybrid hydrogel scaffolds. Plastic Reconstr. Surg. 132 (6), 952e–963e. doi:10.1097/prs.0b013e3182a805df
Ruehle, M. A., Krishnan, L., LaBelle, S. A., Willett, N. J., Weiss, J. A., and Guldberg, R. E. (2017). Decorin-containing collagen hydrogels as dimensionally stable scaffolds to study the effects of compressive mechanical loading on angiogenesis. MRS Commun. 7 (3), 466–471. doi:10.1557/mrc.2017.54
Sakata, R., Iwakura, T., and Reddi, A. H. (2015). Regeneration of articular cartilage surface: Morphogens, cells, and extracellular matrix scaffolds. Tissue Eng. Part B Rev. 21 (5), 461–473. doi:10.1089/ten.teb.2014.0661
Samberg, M., Stone, R., Natesan, S., Kowalczewski, A., Becerra, S., Wrice, N., et al. (2019). Platelet rich plasma hydrogels promote in vitro and in vivo angiogenic potential of adipose-derived stem cells. Acta Biomater. 87, 76–87. doi:10.1016/j.actbio.2019.01.039
Santulli, G., Ciccarelli, M., Palumbo, G., Campanile, A., Galasso, G., Ziaco, B., et al. (2009). In vivo properties of the proangiogenic peptide QK. J. Transl. Med. 7 (1), 41–10. doi:10.1186/1479-5876-7-41
Seow, W. Y., Salgado, G., Lane, E. B., and Hauser, C. A. (2016). Transparent crosslinked ultrashort peptide hydrogel dressing with high shape-fidelity accelerates healing of full-thickness excision wounds. Sci. Rep. 6 (1), 32670–32712. doi:10.1038/srep32670
Shafiq, M., Zhang, Y., Zhu, D., Zhao, Z., Kim, D-H., Kim, S. H., et al. (2018). In situ cardiac regeneration by using neuropeptide substance P and IGF-1C peptide eluting heart patches. Regen. Biomater. 5 (5), 303–316. doi:10.1093/rb/rby021
Singh, N. K., and Lee, D. S. (2014). In situ gelling pH-and temperature-sensitive biodegradable block copolymer hydrogels for drug delivery. J. Control. Release 193, 214–227. doi:10.1016/j.jconrel.2014.04.056
Smith, D. J., Brat, G. A., Medina, S. H., Tong, D., Huang, Y., Grahammer, J., et al. (2016). A multiphase transitioning peptide hydrogel for suturing ultrasmall vessels. Nat. Nanotechnol. 11 (1), 95–102. doi:10.1038/nnano.2015.238
Smith, D. K. (2009). Lost in translation? Chirality effects in the self-assembly of nanostructured gel-phase materials. Chem. Soc. Rev. 38 (3), 684–694. doi:10.1039/b800409a
Soro, S., Orecchia, A., Morbidelli, L., Lacal, P. M., Morea, V., Ballmer-Hofer, K., et al. (2008). A proangiogenic peptide derived from vascular endothelial growth factor receptor-1 acts through α5β1 integrin. J. Am. Soc. Hematol. 111 (7), 3479–3488. doi:10.1182/blood-2007-03-077537
Steinstraesser, L., Kraneburg, U., Jacobsen, F., and Al-Benna, S. (2011). Host defense peptides and their antimicrobial-immunomodulatory duality. Immunobiology 216 (3), 322–333. doi:10.1016/j.imbio.2010.07.003
Su, Y., Wang, H., Mishra, B., Lakshmaiah Narayana, J., Jiang, J., Reilly, D. A., et al. (2019). Nanofiber dressings topically delivering molecularly engineered human cathelicidin peptides for the treatment of biofilms in chronic wounds. Mol. Pharm. 16 (5), 2011–2020. doi:10.1021/acs.molpharmaceut.8b01345
Subbiah, R., Thrivikraman, G., Parthiban, S. P., Jones, J. M., Athirasala, A., Xie, H., et al. (2021). Prevascularized hydrogels with mature vascular networks promote the regeneration of critical-size calvarial bone defects in vivo. J. tissue Eng. Regen. Med. 15 (3), 219–231. doi:10.1002/term.3166
Szklanny, A. A., Machour, M., Redenski, I., Chochola, V., Goldfracht, I., Kaplan, B., et al. (2021). 3D bioprinting of engineered tissue flaps with hierarchical vessel networks (VesselNet) for direct host-to-implant perfusion. Adv. Mater. 33 (42), 2102661. doi:10.1002/adma.202102661
Tae, G., Kim, Y-J., Choi, W-I., Kim, M., Stayton, P. S., and Hoffman, A. S. (2007). Formation of a novel heparin-based hydrogel in the presence of heparin-binding biomolecules. Biomacromolecules 8 (6), 1979–1986. doi:10.1021/bm0701189
Tamamis, P., Adler-Abramovich, L., Reches, M., Marshall, K., Sikorski, P., Serpell, L., et al. (2009). Self-assembly of phenylalanine oligopeptides: Insights from experiments and simulations. Biophysical J. 96 (12), 5020–5029. doi:10.1016/j.bpj.2009.03.026
Tian, Y., Seeto, W. J., Páez-Arias, M. A., Hahn, M. S., and Lipke, E. A. (2022). Endothelial colony forming cell rolling and adhesion supported by peptide-grafted hydrogels. Acta Biomater. 152, 74–85. doi:10.1016/j.actbio.2022.08.047
Toh, W. S., Lee, E. H., Guo, X-M., Chan, J. K., Yeow, C. H., Choo, A. B., et al. (2010). Cartilage repair using hyaluronan hydrogel-encapsulated human embryonic stem cell-derived chondrogenic cells. Biomaterials 31 (27), 6968–6980. doi:10.1016/j.biomaterials.2010.05.064
Török, M., Merkely, P., Monori-Kiss, A., Horváth, E. M., Sziva, R. E., Péterffy, B., et al. (2021). Network analysis of the left anterior descending coronary arteries in swim-trained rats by an in situ video microscopic technique. Biol. sex Differ. 12 (1), 37–17. doi:10.1186/s13293-021-00379-y
Tovar-Castillo, L. E., Cancino-Díaz, J. C., García-Vázquez, F., Cancino-Gómez, F. G., León-Dorantes, G., Blancas-González, F., et al. (2007). Under-expression of VHL and over-expression of HDAC-1, HIF-1α, LL-37, and IAP-2 in affected skin biopsies of patients with psoriasis. Int. J. dermatology 46 (3), 239–246. doi:10.1111/j.1365-4632.2006.02962.x
Varghese, S. A., Rangappa, S. M., Siengchin, S., and Parameswaranpillai, J. (2020). NatuRal polymers and the hydrogels prepared from them Hydrogels Based on Natural Polymers. Elsevier, 17–47.
Vu, M., Pramanik, A., Basak, A., Prakash, C., and Shankar, S. (2022). Progress and challenges on extrusion based three dimensional (3D) printing of biomaterials. Bioprinting 27, e00223. doi:10.1016/j.bprint.2022.e00223
Wang, J., Wang, X., Yan, G., Fu, S., and Tang, R. (2017). pH-sensitive nanogels with ortho ester linkages prepared via thiol-ene click chemistry for efficient intracellular drug release. J. colloid interface Sci. 508, 282–290. doi:10.1016/j.jcis.2017.08.051
Wang, L., Wang, C., Wu, S., Fan, Y., and Li, X. (2020). Influence of the mechanical properties of biomaterials on degradability, cell behaviors and signaling pathways: Current progress and challenges. Biomaterials Sci. 8 (10), 2714–2733. doi:10.1039/d0bm00269k
Wang, Y., Cao, Z., Wei, Q., Ma, K., Hu, W., Huang, Q., et al. (2022). VH298-loaded extracellular vesicles released from gelatin methacryloyl hydrogel facilitate diabetic wound healing by HIF-1α-mediated enhancement of angiogenesis. Acta Biomater. 147, 342–355. doi:10.1016/j.actbio.2022.05.018
Wang, Y., Kankala, R. K., Ou, C., Chen, A., and Yang, Z. (2022). Advances in hydrogel-based vascularized tissues for tissue repair and drug screening. Bioact. Mater. 9, 198–220. doi:10.1016/j.bioactmat.2021.07.005
Wang, Z., Wen, F., Lim, P. N., Zhang, Q., Konishi, T., Wang, D., et al. (2017). Nanomaterial scaffolds to regenerate musculoskeletal tissue: Signals from within for neovessel formation. Drug Discov. Today 22 (9), 1385–1391. doi:10.1016/j.drudis.2017.03.010
Wazzani, R., Pallu, S., Bourzac, C., Ahmaïdi, S., Portier, H., and Jaffré, C. (2021). Physical activity and bone vascularization: A way to explore in bone repair context? Life 11 (8), 783. doi:10.3390/life11080783
Wei, D., Xiao, W., Sun, J., Zhong, M., Guo, L., Fan, H., et al. (2015). A biocompatible hydrogel with improved stiffness and hydrophilicity for modular tissue engineering assembly. J. Mater. Chem. B 3 (14), 2753–2763. doi:10.1039/c5tb00129c
Wolf, M. T., Daly, K. A., Brennan-Pierce, E. P., Johnson, S. A., Carruthers, C. A., D'Amore, A., et al. (2012). A hydrogel derived from decellularized dermal extracellular matrix. Biomaterials 33 (29), 7028–7038. doi:10.1016/j.biomaterials.2012.06.051
Xing, Z., Zhao, C., Wu, S., Zhang, C., Liu, H., and Fan, Y. (2021). Hydrogel-based therapeutic angiogenesis: An alternative treatment strategy for critical limb ischemia. Biomaterials 274, 120872. doi:10.1016/j.biomaterials.2021.120872
Xu, B., Li, Y., Deng, B., Liu, X., Wang, L., and Zhu, Q. L. (2017). Chitosan hydrogel improves mesenchymal stem cell transplant survival and cardiac function following myocardial infarction in rats. Exp. Ther. Med. 13 (2), 588–594. doi:10.3892/etm.2017.4026
Xu, F., Dawson, C., Lamb, M., Mueller, E., Stefanek, E., Akbari, M., et al. (2022). Hydrogels for tissue engineering: Addressing key design needs toward clinical translation. Front. Bioeng. Biotechnol. 10, 849831. doi:10.3389/fbioe.2022.849831
Xu, F., Zou, D., Dai, T., Xu, H., An, R., Liu, Y., et al. (2018). Effects of incorporation of granule-lyophilised platelet-rich fibrin into polyvinyl alcohol hydrogel on wound healing. Sci. Rep. 8 (1), 14042–14110. doi:10.1038/s41598-018-32208-5
Xu, K., Cantu, D. A., Fu, Y., Kim, J., Zheng, X., Hematti, P., et al. (2013). Thiol-ene Michael-type formation of gelatin/poly (ethylene glycol) biomatrices for three-dimensional mesenchymal stromal/stem cell administration to cutaneous wounds. Acta biomater. 9 (11), 8802–8814. doi:10.1016/j.actbio.2013.06.021
Xue, K., Wang, X., Yong, P. W., Young, D. J., Wu, Y. L., Li, Z., et al. (2019). Hydrogels as emerging materials for translational biomedicine. Adv. Ther. 2 (1), 1800088. doi:10.1002/adtp.201800088
Xue, X., Hu, Y., Deng, Y., and Su, J. (2021). Recent advances in design of functional biocompatible hydrogels for bone tissue engineering. Adv. Funct. Mater. 31 (19), 2009432. doi:10.1002/adfm.202009432
Yanagisawa, T., Ishii, M., Takahashi, M., Fujishima, K., and Nishimura, M. (2020). Human cathelicidin antimicrobial peptide LL-37 promotes lymphangiogenesis in lymphatic endothelial cells through the ERK and Akt signaling pathways. Mol. Biol. Rep. 47 (9), 6841–6854. doi:10.1007/s11033-020-05741-8
Yanev, P., van Tilborg, G. A., van der Toorn, A., Kong, X., Stowe, A. M., and Dijkhuizen, R. M. (2022). Prolonged release of VEGF and Ang1 from intralesionally implanted hydrogel promotes perilesional vascularization and functional recovery after experimental ischemic stroke. J. Cereb. Blood Flow Metabolism 42 (6), 1033–1048. doi:10.1177/0271678x211069927
Yang, X., Guo, J-L., Han, J., Si, R-J., Liu, P-P., Zhang, Z-R., et al. (2020). Chitosan hydrogel encapsulated with LL-37 peptide promotes deep tissue injury healing in a mouse model. Mil. Med. Res. 7, 20–10. doi:10.1186/s40779-020-00249-5
Yang, Y., Ritchie, A. C., and Everitt, N. M. (2021). Recombinant human collagen/chitosan-based soft hydrogels as biomaterials for soft tissue engineering. Mater. Sci. Eng. C 121, 111846. doi:10.1016/j.msec.2020.111846
Ye, Y-C., Chang, Z-H., Wang, P., Wang, Y-W., Liang, J., Chen, C., et al. (2022). Infarct-preconditioning exosomes of umbilical cord mesenchymal stem cells promoted vascular remodeling and neurological recovery after stroke in rats. Stem Cell Res. Ther. 13 (1), 378–415. doi:10.1186/s13287-022-03083-9
Yin, J., Heutschi, D., Belting, H-G., and Affolter, M. (2021). Building the complex architectures of vascular networks: Where to branch, where to connect and where to remodel? Curr. Top. Dev. Biol. 143, 281–297.
Yu, C., Yang, H., Wang, L., Thomson, J. A., Turng, L-S., and Guan, G. (2021). Surface modification of polytetrafluoroethylene (PTFE) with a heparin-immobilized extracellular matrix (ECM) coating for small-diameter vascular grafts applications. Mater. Sci. Eng. C 128, 112301. doi:10.1016/j.msec.2021.112301
Yu, N., Li, Y., Wang, Y., Xu, H., Ye, F., and Fu, Q. (2022). Healing effect of carboxymethyl chitosan-plantamajoside hydrogel on burn wound skin. Burns.
Yu, Y., Deng, C., Meng, F., Shi, Q., Feijen, J., and Zhong, Z. (2011). Novel injectable biodegradable glycol chitosan-based hydrogels crosslinked by Michael-type addition reaction with oligo (acryloyl carbonate)-b-poly (ethylene glycol)-b-oligo (acryloyl carbonate) copolymers. J. Biomed. Mater. Res. Part A 99 (2), 316–326. doi:10.1002/jbm.a.33199
Zhang, J., Zheng, Y., Lee, J., Hua, J., Li, S., Panchamukhi, A., et al. (2021). A pulsatile release platform based on photo-induced imine-crosslinking hydrogel promotes scarless wound healing. Nat. Commun. 12 (1), 1670. doi:10.1038/s41467-021-21964-0
Zhang, R., Liu, Y., Qi, Y., Zhao, Y., Nie, G., Wang, X., et al. (2022). Self-assembled peptide hydrogel scaffolds with VEGF and BMP-2 enhanced in vitro angiogenesis and osteogenesis. Oral Dis. 28 (3), 723–733. doi:10.1111/odi.13785
Zhang, X., Chen, X., Hong, H., Hu, R., Liu, J., and Liu, C. (2022). Decellularized extracellular matrix scaffolds: Recent trends and emerging strategies in tissue engineering. Bioact. Mater. 10, 15–31. doi:10.1016/j.bioactmat.2021.09.014
Zhang, X., Kang, X., Jin, L., Bai, J., Liu, W., and Wang, Z. (2018). Stimulation of wound healing using bioinspired hydrogels with basic fibroblast growth factor (bFGF). Int. J. nanomedicine 13, 3897–3906. doi:10.2147/ijn.s168998
Zhao, P., Gu, H., Mi, H., Rao, C., Fu, J., and Turng, L-S. (2018). Fabrication of scaffolds in tissue engineering: A review. Front. Mech. Eng. 13, 107–119. doi:10.1007/s11465-018-0496-8
Zhu, D., Li, Z., Huang, K., Caranasos, T. G., Rossi, J. S., and Cheng, K. (2021). Minimally invasive delivery of therapeutic agents by hydrogel injection into the pericardial cavity for cardiac repair. Nat. Commun. 12 (1), 1412. doi:10.1038/s41467-021-21682-7
Zhu, J., and Marchant, R. E. (2011). Design properties of hydrogel tissue-engineering scaffolds. Expert Rev. Med. devices 8 (5), 607–626. doi:10.1586/erd.11.27
Ziemba, A. M., and Gilbert, R. J. (2017). Biomaterials for local, controlled drug delivery to the injured spinal cord. Front. Pharmacol. 8, 245. doi:10.3389/fphar.2017.00245
Keywords: extracellular matrix, hydrogel, biological scaffolds, tissue engineering, biocompatibility, vasculogenesis, angiogenesis
Citation: López-Gutierrez J, Ramos-Payán R, Ayala-Ham A, Romero-Quintana JG, Castillo-Ureta H, Villegas-Mercado C, Bermúdez M, Sanchez-Schmitz G and Aguilar-Medina M (2023) Biofunctionalization of hydrogel-based scaffolds for vascular tissue regeneration. Front. Mater. 10:1168616. doi: 10.3389/fmats.2023.1168616
Received: 17 February 2023; Accepted: 29 March 2023;
Published: 06 April 2023.
Edited by:
Janay Vacharasin, Francis Marion University, United StatesReviewed by:
Samuel Ebhodaghe, University of Benin, NigeriaCopyright © 2023 López-Gutierrez, Ramos-Payán, Ayala-Ham, Romero-Quintana, Castillo-Ureta, Villegas-Mercado, Bermúdez, Sanchez-Schmitz and Aguilar-Medina. This is an open-access article distributed under the terms of the Creative Commons Attribution License (CC BY). The use, distribution or reproduction in other forums is permitted, provided the original author(s) and the copyright owner(s) are credited and that the original publication in this journal is cited, in accordance with accepted academic practice. No use, distribution or reproduction is permitted which does not comply with these terms.
*Correspondence: Maribel Aguilar-Medina, bWFyaWJlbGFndWlsYXJAdWFzLmVkdS5teA==
Disclaimer: All claims expressed in this article are solely those of the authors and do not necessarily represent those of their affiliated organizations, or those of the publisher, the editors and the reviewers. Any product that may be evaluated in this article or claim that may be made by its manufacturer is not guaranteed or endorsed by the publisher.
Research integrity at Frontiers
Learn more about the work of our research integrity team to safeguard the quality of each article we publish.