- 1UMR CNRS 5223 Ingénierie des Matériaux Polyméres, Université de Lyon, INSA Lyon, Villeurbanne, France
- 2Université de. Lyon, INSA Lyon, DEEP Laboratory, Villeurbanne, France
Bio-based and (bio)degradable polymers constitute an important material innovation because they reduce the amount of waste materials inducing persistent microplastics and can offer similar benefits to conventional polymer materials. Poly(butylene succinate) and poly(lactic acid) blends exhibit interesting properties and can be possible alternatives to some traditional polymers. Some of their properties can be tailored by adding small proportions of ionic liquids (IL) that can act as interfacial agents between PBS and PLA. In our study, samples formulated with ionic liquids display a broader morphology with thermal properties close to the PBS/PLA reference, whereas Young’s modulus is lowered in the presence of one of the IL studied. Nevertheless, the blends have a rather different ability to (bio)degrade. Indeed, disintegration experiments show that PBS/PLA/IL exhibit higher weight losses and faster fragmentation. DSC thermograms display an important decrease of PLA melting temperature after composting experiment, indicating that PLA phases are affected the most by degradation at 58°C. Our study shows that elaborating polymer materials, for which degradation processes are preferentially located in a “predegraded” dispersed phase, can be considered as a way to speed up macroscopic (bio)degradation. In the present work, morphologies, mechanical properties as well as (bio)degradability can be tailored by adding a small amount of ionic liquids.
Introduction
The development of polymer blends has been attracting the attention of academic and industrial research for years, and represents both important scientific and economic challenges (Wu, 1985; Wu, 1987; Yu et al., 2006). Indeed, binary and ternary mixtures are promising routes for the production of high performance polymers with cost-effectiveness. In the field of polymer materials, many authors have highlighted the advantages of combining the properties of neat polymers. There are many examples of commercial polymer blends made of 1) polyolefins such as polypropylene (PP), polyethylene (PE) and its copolymers (Fel et al., 2016); 2) engineering polymers such as polyamide (PA), polyethylene terephthalate (PET) (Utracki, 1998; Lins et al., 2015; Quitadamo et al., 2017).
Concerning biodegradable polymers, many authors have developed blends containing poly(lactic acid) (PLA), which is well-known to have excellent properties, such as a high stiffness and good water vapor barrier properties (Ma, 2004; Bordes et al., 2009; Okamoto and John, 2013) that make it suitable for packaging and medical applications. However, its major drawback, i.e. its low stretchability, limits its use in packaging applications (Kopinke et al., 1996; Taubner and Shishoo, 2001). To circumvent this problem, PLA is widely blended with more ductile biodegradable polymers such as poly(butylene-adipate-co-terephtalate) (PBAT), poly(butylene-succinate) (PBS) or polycaprolactone (Na et al., 2002; Furukawa et al., 2005; Jiang et al., 2006). In such a context, poly(butylene succinate) (PBS) and poly(lactic acid) (PLA), two bio-based polyesters, combine satisfying mechanical and thermal properties, as well as an ability for (bio)degradation (Bhatia et al., 2007; Su et al., 2019).
PBS, obtained by polycondensation of fossil or bio-based succinic acid and 1,4 butanediol, can be a relevant alternative to polyethylene for various uses, such as packaging, films. PBS and its copolymers are able to biodegrade in diverse environments (Xu et al., 2010a), with potential regulation of biodegradation as a function of the formulations. In an objective of reinforcement, PBS can be blended with PLA, with which it is not miscible, at proportions higher than 20%wt of PBS in PLA (Shibata et al., 2006; Bhatia et al., 2007; Deng and Thomas, 2015). It was found that PBS particles in PLA acted as a nucleating agent for PLA (Yokohara and Yamaguchi, 2008; Wu et al., 2012; Deng and Thomas, 2015; Ostrowska et al., 2019). Qiu et al. characterized and modelled the mechanical behavior of PLA/PBS blends with different proportions (Qiu et al., 2016). More recently, Deng et al. blended PBS and PLA as well, and found that the addition of PBS in PLA matrix increases the ductility due to a continuous phase morphology (Deng and Thomas, 2015). Morphology, as well as thermal and mechanical properties, can be adjusted by selecting appropriate formulations (Livi et al., 2015). Moreover, parameters such as crystallinity, molecular weight, hydrophobicity of polymer materials, influence their behavior. For example, crystalline regions biodegrade less easily, and small molecular weights facilitate biodegradation. Hydrophilicity is preferred for improving affinity with bacteria and enzymes able to catalyze chain scissions. Weak phases of multiphase materials, leading to voids after degradation, can facilitate diffusion of bacteria and enzymes. Biodegradation is also easier at temperatures close or higher than the glass transition temperature (Tg) (Agarwal et al., 1998; Andersson et al., 2010; Delamarche et al., 2020a). Studies mentioning biodegradation of PBS/PLA blends showed that adding PBS in a PLA matrix leads to a slower degradation rate in compost at 58°C due to PBS phases being highly crystalline (Luzi et al., 2016). On the contrary, at ambient temperature in soil, degradability of PBS/PLA blends increases with increasing the PBS content (Zhang et al., 2013).
In addition, from a thermodynamical point of view, it is well-known that mixtures of polymers lead to immiscible blends, with a high interfacial tension, making melt mixing very difficult, and inducing the formation of unstable morphologies with weak interfacial adhesion, which are the main causes of poor final properties, such as mechanical performances. In fact, for polymer blends composed of two immiscible polymers denoted A and B, two types of macromolecular chains tend to minimize their contact interface, due to their mutual repulsion. This phenomenon results in a macro-separated phase leading to their bad mutual adhesion and therefore to the poor quality of the blends formed. Depending on the proportion of each polymer phase, two types of morphologies can be generated: 1) nodular morphology where the minor phase forms the dispersed nodular phase in the continuous phase of the second polymer and 2) co-continuous morphology where an increase of the minor phase induces a percolation phenomenon of this phase. For these reasons, many authors have used ionic liquids to produce polymer blends with enhanced final properties. For example, Fortunati et al. studied the influence of tributyl acetate citrate and isosorbide diester as plasticizers in PLA/PBS blends (Fortunati et al., 2017). Compatibilization agents used in PLA/PBS blends were listed by Su et al. (Su et al., 2019). The two polyesters being immiscible, hydrolysis mechanisms can take place faster than for neat polymers, due to the presence of voids at the interfaces between PBS and PLA phases (Wang et al., 2016).
In 2015, Lins et al. have investigated the effect of ILs based on trihexyltetradecyl phosphonium cation with different counter anions, on blends composed of poly(butylene-adipate-co-terephtalate) (PBAT) and poly(lactid acid) (PLA) (Lins et al., 2015). Phosphonium ILs combined with chloride (IL-Cl), phosphinate (IL-TMP), bistriflimide (IL-TFSI), hexanoate (IL-EHT) and phosphate (IL-EHP) have been introduced in PBAT/PLA blends by using a DSM micro-extruder with co-rotating screws leading to the formation of PLA fibrils or small droplets associated with the generation of gradient interfacial zones between PLA and PBAT polymers, depending on the interactions between both polymers and the counter anion, in agreement with Luzi et al. (Luzi et al., 2016). More recently, Megevand et al. have revealed the formation of interfacial mixture between PBAT and PLA compatibilized by ILs, with Atomic Force Microscopy. The authors have demonstrated that IL-Cl led to the formation of a thick interphase generating a good local miscibility of PBAT and PLA phases while IL-TMP induced the formation of an interface layer ensuring interactions with the two polymers (Megevand et al., 2016).
The first part of this work has consisted in investigating the influence of ILs based on ammonium or phosphonium cations on the final properties of PBS/PLA blends. In particular, an important loss of PLA molecular weight in presence of phosphonium ionic liquid was attained during melt processing, impacting its (bio)degradability.
In order to tailor use properties and (bio)degradation, we have chosen a PLA 005 grade suitable for good mixing with our PBS and IL, a ratio PBS/PLA of 60/40 to attain Young’s modulus, convenient for applications such as packaging. 1% by weight of IL was selected as it is enough for IL to act as an interfacial agent confined in the material. For amounts in the range of 2–5%, the IL could migrate out of the material and to the PLA phase with increased plasticization and degradation of the PLA.
The second part deals with the study of both degradation and biodegradation of PLA/PBS/ionic liquids blends, with the objective of attaining a compromise between thermal, mechanical properties and ability for (bio)degradation. Indeed, physical, chemical degradations can be associated with biodegradation (Krzan et al., 2006).
Polyesters, polyamides, polysaccharides and polycarbonates, and in general polymers synthesized by polycondensation, are particularly subject to hydrolysis, in neutral, acid, and also in alkaline conditions (Hawkins, 1984).
Abiotic hydrolysis can be performed at neutral or basic pH when it is known that this promotes degradation (Cho et al., 2001; Zhou and Xanthos, 2008). They then allow the study of abiotic hydrolysis of polymers, which may be the limiting mechanism in the degradation of biodegradable polymers in complex media. In abiotic environments, PLA degrades faster in basic solutions than in neutral or acidic solutions (Makino et al., 1986; Schliecker et al., 2003; Xu et al., 2010). Hydrolysis of PBS is also favoured in basic media (Xu and Guo, 2010b).
As a consequence, in this study, 3-months-abiotic hydrolysis was conducted in deionized water and in alkaline solution. We have chosen to perform the abiotic tests under the same temperature conditions (58°C) as composting experiment. 3-months-composting was conducted in open containers, under the temperature of 58°C as ISO (2012). These identical temperature conditions allow us to determine whether abiotic hydrolysis is the limiting mechanism for biodegradation of the tested polymers.
Moreover, 58°C is not only a temperature required for ISO experiments but is also very close to the glass transition temperature of PLA and superior to the one of PBS.
To summarize, the present work aims at studying the relations between polymer materials properties and (bio)degradation. In fact, characteristics such as molecular weights, crystallinities, morphologies, surface properties are well known parameters that impact the biodegradability (Delamarche et al., 2020b). 3-months degradation essays were conducted on polyester samples to evaluate their degradability 1) under composting conditions, 2) in water at 58°C, 3) under soil burial conditions, and 4) in humid atmosphere at room temperature. Weight loss, 1H NMR spectra, and thermal properties measurements were used to follow degradation.
Materials and methods
Products
Products used in this study are listed in Table 1. PBS and PLA pellets were supplied by Natureplast (PBE 003 and PLA 005 grades, respectively). l-lactic acid/d-lactic acid content for PLA is 94:6 mol% (Dorigato et al., 2012). Trihexyl(tetradecyl)phosphonium chloride (denoted in the text as P-Cl) was purchased from Cytec. Tris(2-hydroxyethyl)methylammonium methylsulfate (denoted in the text as A-M) was purchased from Sigma Aldrich.
Sample preparation
Polymer pellets were dried in an oven at 70°C for 12 h. Pellets and additives were extruded using a 15g-capacity DSM microextruder (Midi 2000 Heerlen, Netherlands) with co-rotating screws (L/D ratio of 18) at 190°C, with a 100 rpm speed, for 3 min. PBS/PLA (60:40 wt. ratio), PBS/PLA/P-Cl (60:40:1 wt. ratio) and PBS/PLA/A-M (60:40:1 wt. ratio) were extruded. Extruded samples were injected in a 10 cm3 mould at 30°C to obtain 2 mm thick and 4 mm wide dumbbell-shaped specimens. 0.2 mm thin films were processed under compression at 210°C and cut for ageing experiments.
Characterization
Morphologies
Transmission electron microscopy (TEM) was carried out at the Technical Center of Microstructures of Lyon on a Philips CM 120 microscope with an accelerating voltage of 80 kV. 80–100 nm thin samples, which were cut using an ultramicrotome equipped with a diamond knife, were set on copper grids.
1H NMR
One dimensional 1H NMR spectroscopy was used to determine the PBS and PLA contents of the blends. Samples, cut from films, were dissolved in CDCl3 and analyzed at 25°C using a Bruker Advance III spectrometer (400 MHz), equipped with a 5 mm multinuclear broadband probe (BBFO+). In order to evaluate the weight percentage of PBS and PLA in the blends, peak resonance (a) of PLA (CH) at 5.1 ppm and resonance peak (a) of PBS (CH2) at 2.6 ppm were integrated to calculate the weight content of PBS:
with
End chain group analysis was carried out to calculate mean molecular weights of PBS. It was assumed that each polyester chain exhibits one hydroxyl and one carboxylic end groups. Resonance peak of hydroxyl end-group (CH2-OH) of PBS, appearing at 3.7 ppm (Labruyère et al., 2014), was integrated as well as CH2 (a) one in the monomer repeating unit at 2.6 ppm
with
In order to evaluate the molecular weight of PLA phase after processing, hydroxyl end-group of PLA (CH-OH) signed at 4.34 ppm and CH (a) of the monomer at 5.1 ppm were analyzed to calculate the molecular weights. However, this peak is close to peak (b) of PBS. Hence, PLA end-chain titration was carried out after extracting PLA from the PBS/PLA blend with the following protocol. First, samples were dissolved in CHCl3. Then, tetrahydrofuran was added to induce precipitation of PBS. After filtration and solvent evaporation, the remaining PLA and PBS oligomers (soluble in THF) were analyzed by NMR in CDCl3.
with
Thermal properties
Differential scanning calorimetry analyses were carried out using a model Q20 TA Instruments equipment (TA Instruments, New Castle, DE, United States). Samples were subjected twice to a cycle of heating and cooling ramps of 10°C/min, from -70 to 200°C and from 200°C to −70°C.
Thermogravimetric analyses were carried out using a model Q500 TA Instruments equipment. Samples were subjected to a heating rate of 20°C min−1 under nitrogen atmosphere from 25 to 600°C. Temperatures at which 1% of the initial weight was lost T1% deg (°C), and degradation temperatures TdegPLA and TdegPBS (obtained from the maxima of the derivative curves of the weight loss as a function of temperature) were determined.
Mechanical properties
Uniaxial tensile tests were conducted using an INSTRON 33R4469 (Instron, Norwood, MA, United States) tensile machine at 25°C. Dumbbell specimens were tested for a 50 mm/min elongation speed. Young’s modulus, maximum and average strain at break were determined.
Dynamic mechanical analysis
Dynamic mechanical measurements were carried out using an ARES G2 rheometer (TA Instruments, New Castle, DE, United States). The heating rate was 3°C min−1 from -60–90°C at a frequency of 1Hz, (0.1% oscillation strain). The change of shear storage modulus, G′, and shear loss modulus, G″, were determined. tan(δ) was considered to determine the alpha-relaxation temperatures, Tα, related to Tg of PBS and PLA. ∆Tα was defined as the difference between T(α(PBS)) and T(α(PLA)).
Molecular weights
Molecular weights were determined using a steric exclusion chromatography (SEC) equipment, comprising Agilent Technologies columns (Argilent Technologies, Palo Alto, CA, United States), a light scattering detector, and a Shimadzu RID-10A detector. 3 mg samples were cut and dissolved in chloroform (1 mg/ml). Analysis took place at 30°C under a 1 ml/min flow.
Degradatation experiments
Degradation experiments for 3 months were performed considering films exposed to various environments. Every month, two samples of each formulation were recovered, washed with deionized water, and gently dried on absorbent paper. Then, they were dried in a vacuum oven at 30°C for 12 h and kept in a closed desiccator. Weight losses were calculated as follows (
Abiotic hydrolysis
3-months-abiotic hydrolysis was conducted at 58°C in deionized water and in alkaline solution (NaOH 0.01 M). 1.25*1.5*0.02 cm films were disposed in 10 ml of aqueous solution in individual closed flasks.
Composting experiment
3-months-composting was conducted at 58°C in open containers. Compost was from composting facility of Racine - Ecopole la Rize (Décines-Charpieu, France). Organic matter percentage was obtained by calcination of dry samples and was found to be 58.2 ± 0.3 wt%. The water content and ability to retain water were analyzed. 2.5*1.5*0.02 cm polymer samples were buried 15 cm beneath the surface. Moisture content was regularly adjusted to be 90% of the maximum capacity of water retention.
Soil burial
3-months-soil burial experiment was conducted at room temperature in open containers. Soil was from LyonTech Campus La Doua, Villeurbanne, France. Organic matter percentage was obtained by calcination of dry samples and was found to be 21 ± 3 wt%. Water composition and ability to retain water of the soil were analyzed as well. 2.5*1.5*0.02 cm polymer samples were buried 10 cm beneath the surface. Moisture content was regularly adjusted to 90% of the maximum capacity of water retention.
Humid atmosphere
2.5*1.5*0.02 cm polymer samples were disposed in a closed transparent chamber with humidity-saturated air at room temperature for 3 months.
Results and Discussion
Tailoring PBS/PLA blends properties with ILs
TEM micrographs (Figure 1) show examples of the dispersion of PLA phases (white domains) in the PBS matrix, confirming that PBS and PLA are immiscible (Bhatia et al., 2007). PLA phases in PBS/PLA blend without IL are irregularly dispersed (Figure 1A), and their maximum surface area is about 0.8 µm2. The non-uniform dispersion of PLA domains has been observed by Deng et al., and it was assumed that, since PBS exhibits a lower melt viscosity than PLA, it is not able to break down PLA droplets (Deng and Thomas, 2015). Similar compatible blends have been prepared in previous studies, including PBAT/PLA/LI and PBAT/PLA/lignin/LI blends (Lins et al., 2015; Livi et al., 2015) where it was clearly demonstrated that ionic liquids act as compatibilizing agents. Indeed, ionic liquids were located at the interface of the PLA domains and when increasing the amount of ionic liquids, a migration of ionic liquids in the minority phase was observed (Megevand et al., 2016). In the present study, the addition of ionic liquids induces the presence of larger PLA domains (up to 27 μm2 with P-Cl). Furthermore, PLA domains have ovoid shapes. Then, inclusions of PBS are observed in PLA domains. Since the PBS/PLA weight proportion is 60/40, phase inversion is likely to occur. Indeed, Wu et al. reported that, if the weight proportion of PBS is 60% or more, it becomes the continuous phase, and the inversion point occurs at around 50/50 (Wu et al., 2012), which can explain the increase of PLA phase. At molecular scale, ionic liquids are observed at the interfaces.
The fact that adding ionic liquids induces larger PLA domains in the PBS matrix was not expected regarding our earlier studies. Lins et al. added 1% of phosphonium ILs in PBAT/PLA blends and showed that ILs generated interfacial zones due to a strong attraction to ester groups, leading to a decrease of the interfacial tension (Lins et al., 2015).
Tensile properties of PBS/PLA blends, reported in Table 2, show that neat PBS/PLA exhibits a rather high Young’s modulus due to brittle PLA phases, acting as reinforcing agent, and a high strain at break due to PBS being ductile (Qiu et al., 2016). In presence of IL, complex morphologies, at ratio PBS/PLA 60/40 close to the inversion phase make the discussion rather difficult to establish relationship between tensile properties and morphology of the blends. Young’s modulus and strain at break are slightly improved with ammonium IL, which could suggest the IL acting as an interfacial agent. The increase of modulus can also be explained by the fact that PLA domains are larger, and hence constitute more effective reinforcing agents. However, regarding PBS/PLA blend with phosphonium IL, the stiffness is reduced while elongation at break is significantly higher. PLA domains do not behave as a reinforcing agent in this blend. Elongation at break is probably enhanced due to a plasticization process, which could be induced by small PLA chains and phosphonium IL. It has been seen in the literature that phosphonium IL can plasticize PLA, as well as catalyze its degradation during processing (Park and Xanthos, 2009). By using Phosphonium IL when processing the PBS/PLA blend, it is not necessary to add a preliminary step aiming at cutting commercially available PLA chains in order to facilitate biodegradation. Hence, the increase of strain at break could be a consequence of low molecular weight PLA chains (
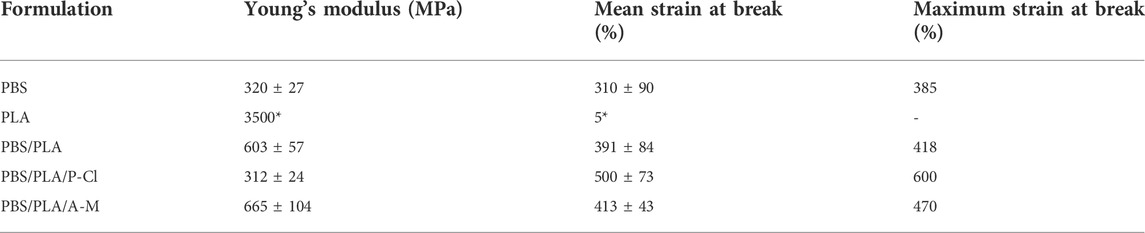
TABLE 2. Mechanical properties of neat PLA and PBS and PBS/PLA blends. *Data provided in product datasheet.
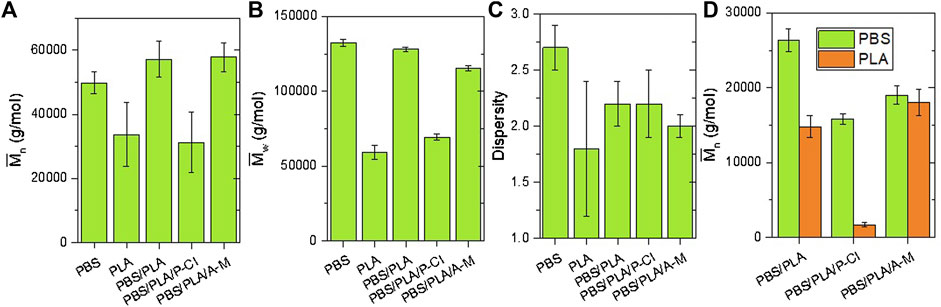
FIGURE 2.
Considering environmental and safety issues, it is well known that ILs, which catalyze the glycolysis of PET, can be used to depolymerize many polymers such as polyethylene terephthalate (Kamber et al., 2010). In our study, only a small amount of ILs is used (1 wt%), acting as interfacial agents and migrating into PLA matrix, which considerably limits environmental and safety issues. In our work, in presence of ionic liquid, the degradation products of PLA with the lowest molecular weights, might be lactic acid, that is neither considered dangerous nor very volatile (Song et al., 2014; Elsawy et al., 2017).
Data acquired with dynamic mechanical analyses are displayed in Table 3. Two distinct alpha-relaxation temperatures confirm that PBS and PLA are not fully miscible at the studied proportions (Deng and Thomas, 2015). A slight merging of the alpha-relaxation temperatures of PLA and PBS with phosphonium ionic liquid is observed. This small shift may be due to partial miscibility of low molecular weight chains (Lins et al., 2015). The decrease of the Tα of PLA is also consistent with a migration of P-Cl ionic liquid in the PLA phase, leading to reduced molecular weights (Figure 2) and lower Tα. Ammonium IL does not have a significant impact on the alpha-relaxation temperatures. These results are in agreement with partial miscibility and/or perhaps transesterification in presence of phosphonium IL.
Number average molecular weight (
Regarding SEC measurements on injected samples, PBS/PLA blends with phosphonium IL exhibit significantly lower molecular weights than PBS/PLA without additive. Using NMR titration, it is observed that the
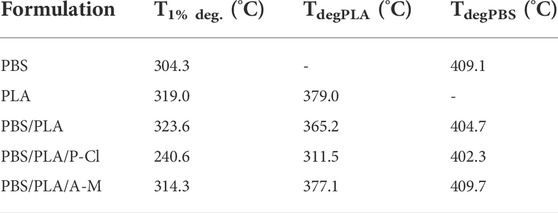
TABLE 4. Degradation temperatures of PLA and PBS phases and degradation temperature for 1% wt. loss from thermogravimetric analyses.
As reported in our previous article (Delamarche et al., 2020a), temperature at 1% degradation (T1% deg.) and at the maxima of the derivative curves of the weight loss as a function of temperature, were 304 and 409°C for PBS; 319 and 379°C for PLA. This is consistent with the fact that PLA phases degraded to a greater extent during processing with P-Cl. Results are also in agreement with differential scanning calorimetry (DSC) results (Table 5), where it can be seen that melting temperature of PLA, during the second heating ramp, decreases with phosphonium IL (from 174.4°C on the first heating ramp to 169.5°C), while for neat PBS/PLA and PBS/PLA with ammonium IL, the melting temperature remains similar between the first and second heating ramp. This can be explained by a degradation of PLA phases taking place during the measurement, at the end of the first heating ramp, leading to smaller molecular weight PLA chains. Melting temperature indeed depends on the molecular weights: under a critical value of molecular weight, the melting temperature drops, whereas above this critical value, the melting temperature is constant (Fatou and Mandelkern, 1965; Sánchez-Soto et al., 2002). Xiang et al. observed that melting temperature of PLA diminishes as the molecular weight diminishes (Xiang et al., 2016).

TABLE 5. Glass transition, melting, and crystallization temperatures of PBS, PLA, PBS/PLA (60:40 phr), PBS/PLA/P-Cl and PBS/PLA/A-M (60:40:1 phr) films determined using DSC (second heating/cooling ramp) before exposure. **Hardly visible peak.
For thermogravimetric analyses, as the IL contents are only 1%, we have assumed their own thermal stability is not influencing significantly the blends behavior. PBS/PLA blend with ammonium IL exhibits slightly higher thermal degradation temperatures (+11.9°C for PLA, +5°C for PBS), which is consistent with a higher molecular weight of PLA measured earlier, and a possible transesterification phenomenon. This could also be due to good interfacial interactions between the ammonium IL and the polyesters, which increase the activation energy necessary to cause degradation (Lins et al., 2015). Therefore, thermogravimetric analysis, like molecular weight measurements, show that the phosphonium IL degrades more specifically PLA phases during processing, which can no more play the role of a reinforcing agent: it can be assumed that PLA chains were degraded to an extent where PLA domains could not provide stiffness to the blend. Ammonium ionic liquid, however, leads to a better stability of the blend.
In PBS/PLA blends, glass transition temperatures appear to be difficult to determine using DSC, because, in the case of PBS, they are wide transitions, and in the case of PLA, they are close to a low intensity melting endotherm of PBS, known in the literature as an annealing peak (Wang et al., 2007). However, we can still observe similar tendencies as the ones observed for alpha relaxation temperatures measured using dynamic mechanical analysis: the Tg of PBS increases by 4°C with the addition of ILs P-Cl, by 1.8°C with IL M-A, whereas, the Tg of PLA phases do not show significant changes (Table 5). As discussed earlier, the shift of glass transition temperature of PBS can be due to a partial miscibility of PLA oligomers in the PBS matrix. Indeed, it has been seen in the literature, that PBS and PLA can exhibit partial miscibility in the amorphous phases to a small extent (Deng and Thomas, 2015; Ostrowska et al., 2019).
Melting and crystallization curves (second ramp) are plotted in Figure 4. Both polymers lead to two melting peaks looking like those of each neat polymer (Bhatia et al., 2007). Melting peak appearing at Tm(PBS) exhibits a more pronounced separation with ILs at second heating ramp. This peak division is typical of PBS melting behavior, and usually appears after crystallization at temperatures above 80°C, with the generation of crystals that have different thermal stability (Wang et al., 2007). Here, adding IL in PBS/PLA blends seems to have the same effect on the crystallinity of PBS as an annealing treatment. This is in agreement with a certain affinity of ILs with PBS. Regarding PLA, melting peak of PLA in PBS/PLA/P-Cl blend appears at lower temperature than for neat PBS/PLA blend on the second heating ramp, suggesting the presence of small crystallites, probably due to low molecular weight PLA chains that were formed during processing and first heating ramp. The melting curve of PBS/PLA blend with ammonium IL is very similar to PBS/PLA without additive, except for a slight separation of melting peak of PBS.
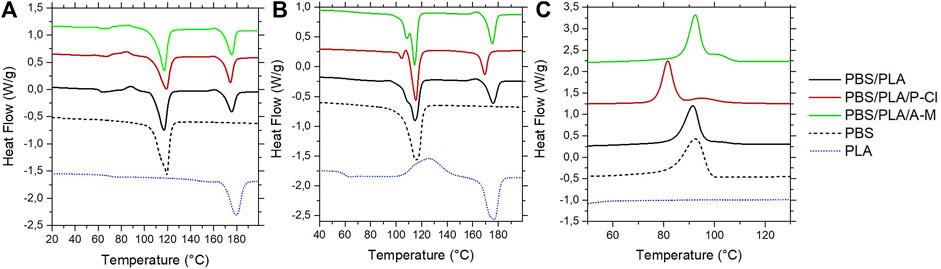
FIGURE 4. Melting (first ramp (A); second ramp (B)) and crystallization (C) curves of PBS, PLA, PBS/PLA (60:40 phr), PBS/PLA/P-Cl and PBS/PLA/A-M (60:40:1 phr) films determined using DSC (heating/cooling rate of 10°C.min−1 under nitrogen atmosphere) before exposure.
It is well-known that the use of IL-modified nanoparticles may induce a heteregenous nucleation effect as well as a lamellar ordering effect in the polymer matrix (Du et al., 2007; Xie et al., 2016). But in this case, we have used only 1 wt% of ILs (without nanoparticles) and we have demonstrated in a previous paper that a small amount has no significant effect on the crystallization temperature of PBAT matrix (Livi et al., 2014).
Neat PBS/PLA blend shows two crystallization peaks, a broad one looking similar to the melting peak of PBS alone, and a small peak that merges and appears at higher temperature. PBS/PLA blend with ammonium IL shows a similar curve, although the small peak appearing at higher temperature is more pronounced, yet not completely separated. Phosphonium IL induces a crystallization peak division. The larger peak, appearing at lower temperature might be PBS crystallization, while the small peak could be associated with PLA crystallization. Another explanation can be that PLA crystallites act as nucleating agents for PBS once they are formed.
Degradation in water, soda solution, compost and soil
Visual observations and weight loss
Fragmentation of samples is reported in Figure 5. After 1 month in deionized water and in NaOH solution at 58°C, one of the two samples of PBS/PLA blends with phosphonium IL was fragmented, whereas both samples of neat blend and PBS/PLA with ammonium IL maintained their integrity. In alkaline medium, PBS/PLA blend and PBS/PLA/A-M exhibit similar weight loss (Figure 6B) except on the third month, where the blend with ammonium IL shows a higher weight loss, while blends with P-Cl exhibit a more important weight loss in the first month (more than 20% weight loss). Data could not be collected for blend PBS/PLA/P-Cl after 2 months because not all the fragments could be recovered. In deionized water, it is observed that PBS/PLA blends containing ionic liquids lose more weight than neat PBS/PLA blend (Figure 6A). This weight loss dramatically increases with phosphonium IL, and is slightly higher with ionic liquid A-M. Regarding the surface aspect, circles are observed on the surface of samples of PBS/PLA/A-M after degradation in NaOH solution, which is less pronounced on neat PBS/PLA blend (Figure 7). This can indicate a preferential degradation at weak points. Figure 8 shows that samples containing P-Cl undergo enhanced visual degradation, both in compost at 58°C for 2 & 3 months as well as in soil at RT, for 3 months.
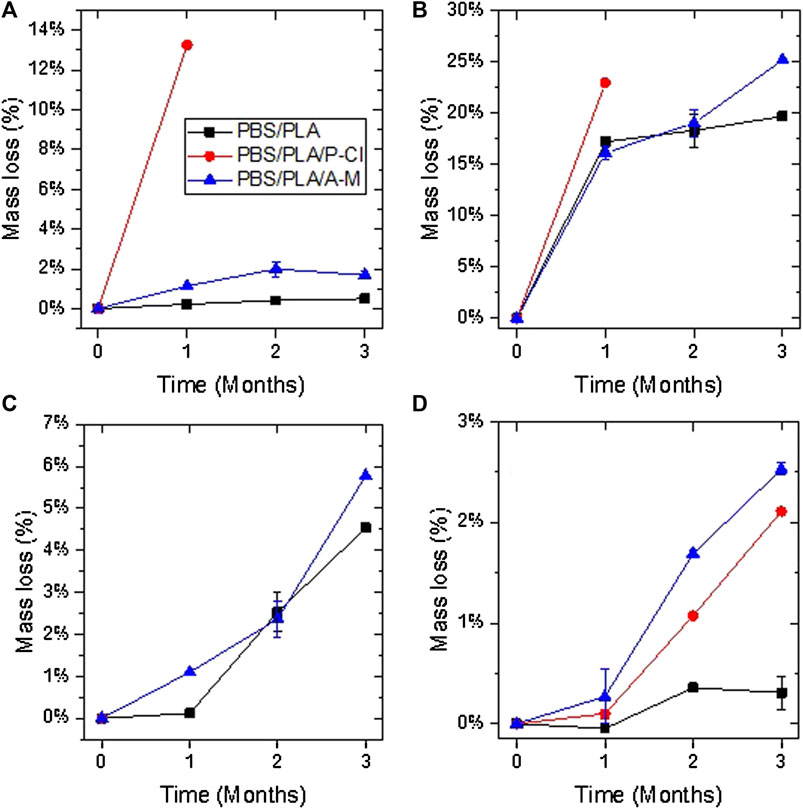
FIGURE 6. Weight loss in deionized water at 58°C (A), in NaOH solution at 58°C (B), under composting conditions at 58°C (C), and under soil burial conditions at room temperature (D). Results are given with standard deviation. If not shown, only one sample could be recovered and weighted.
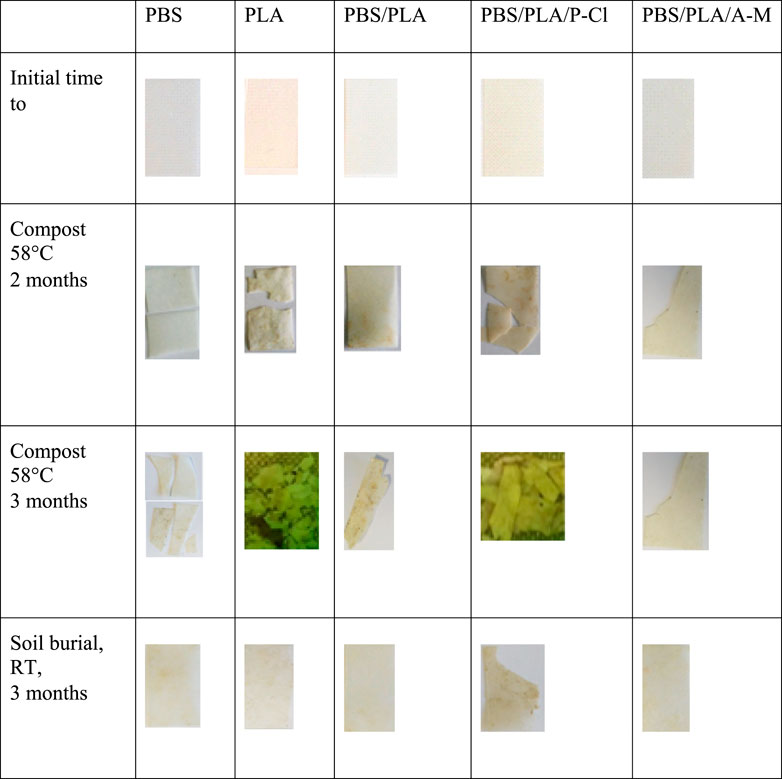
FIGURE 8. Photographs of the samples at initial time, in compost at 58°C after 2 and 3 months, in soil at RT after 3 months.
Under composting conditions at 58°C, all samples with phosphonium IL were fragmented after 1 month. After 2 months, one of the two samples with A-M was fragmented. After 3 months, all blends showed fragmentation. Weight loss (Figure 6C) of the blends with ammonium ionic liquid is similar to the reference without ionic liquid. Samples with P-Cl could not be weighed because of their advanced stage of fragmentation, making it impossible to recover all the fragments. Hence, PBS/PLA blend with phosphonium IL degrade faster than PBS/PLA and PBS/PLA/A-M at 58°C. This blend undergoes the most important degradation during processing due to the addition of IL, as discussed earlier. Since the initial molecular weight of blends with P-Cl is lower, integrity is lost more rapidly during random chain scission caused by hydrolysis. Furthermore, it is well known that PLA degrades well at 58°C (Agarwal et al., 1998; Itävaara et al., 2002; Yagi et al., 2009). Since it was observed, here, that PLA domains were degraded the most by the ionic liquid during processing, shorter chains can constitute weak points in the matrix.
Under soil burial conditions at room temperature, only PBS/PLA blend with phosphonium IL undergoes fragmentation after 3 months. However, PBS/PLA/A-M shows a slightly higher weight loss (Figure 6D). It is not clear yet if exudation of ionic liquids occurs, and if so, at which extent. In humid atmosphere, cracks appeared on PBS/PLA/P-Cl blend after 3 months, indicating that samples became brittle.
Evolution of molecular weights
Molecular weights analyzed using size exclusion chromatography are reported in Figures 9, 10. Since it is not possible to distinguish PLA and PBS phases on SEC chromatograms, the dn/dc of neat PBS (0.06) was used to calculate the average molecular weight of both polymers. The resulting
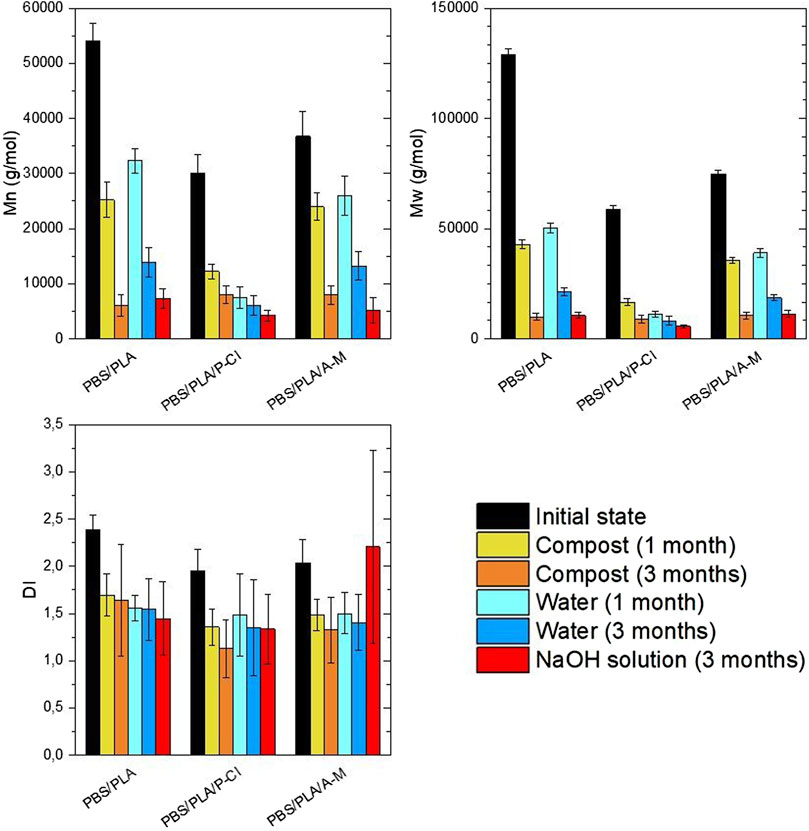
FIGURE 9.
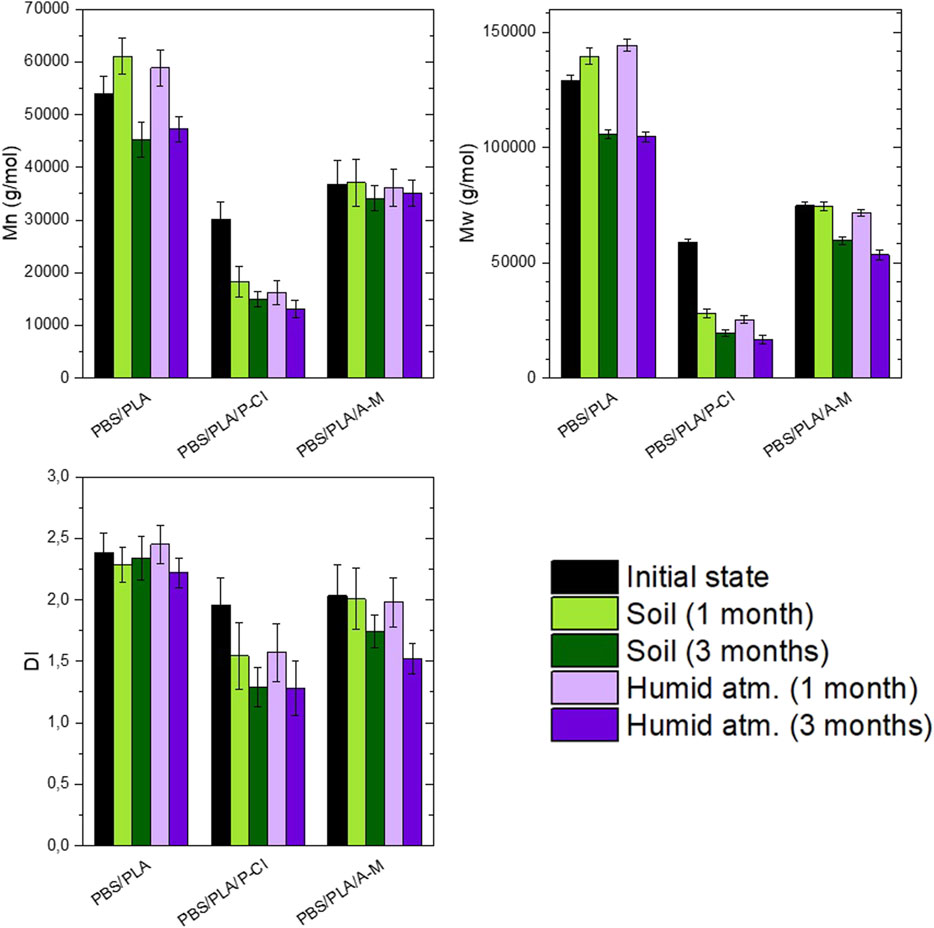
FIGURE 10.
Number average molecular weight of PBS phases was determined by end group analysis using 1H NMR spectroscopy. As said previously, these molecular weights might be underestimated, since it is assumed that PBS chains are not branched.
After experiments at 58°C, under composting conditions and in deionized water, molecular weight loss is significant from the first month and does not importantly decrease afterwards for PBS/PLA blends with phosphonium IL, suggesting that
Under soil burial conditions and in humid atmosphere (Figure 10), at room temperature, PBS/PLA blends with phosphonium IL exhibit a molecular weight loss in the first month, whereas PBS/PLA and PBS/PLA/A-M show no sign of degradation during the first month. In the third month, all blends exhibit molecular weight loss. Molecular weight loss in soil is similar to its loss in humid atmosphere, suggesting that the predominant degradation mechanism under soil burial conditions is abiotic hydrolysis. The more molecular weights decrease, the more the dispersity index decreases, which is a consequence of bond breaking in long chains, as said earlier.
Molecular scale analysis
After 3 months, samples were analyzed in CDCl3 using 1H NMR spectroscopy, to observe the evolution of PBS/PLA proportions (Figure 11). It can be seen that, after 3 months in deionized water and under composting conditions at 58°C, weight proportions of PBS and PLA in PBS/PLA/P-Cl changed from 60:40 to about 70:30, and to about 80:20 in alkaline conditions, and remained unchanged under soil burial conditions. PBS/PLA and PBS/PLA/A-M did not undergo a change in weight proportions. This result is consistent with the assumption that PLA domains degraded faster than PBS, and diffused out of the matrix at 58°C.
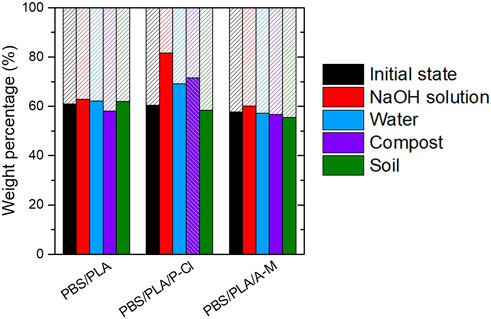
FIGURE 11. Weight proportions of PBS and PLA determined by 1H NMR titration in CDCl3 after 3 months-degradation experiments (*except PBS/PLA/P-Cl after 2 months-composting experiment). Plain columns: PBS wt%, hatched columns: PLA wt%.
Melting and crystallization behavior of PBS and PLA based blends were studied by using differential scanning calorimetry (Figure 12). Melting temperature of PLA in blend PBS/PLA/P-Cl considerably decreases after degradation at 58°C in water (loss of roughly 30°C) and is absent in alkaline conditions. This drop has been observed by Pantani et al. and is certainly attributed to the low molecular weight chains (Pantani and Sorrentino, 2013). The evolution of melting temperatures of PLA and PBS in blend PBS/PLA/A-M is similar to the reference without additive, i.e. a slight decrease of melting temperature of PLA is observed after degradation under composting conditions and in alkaline medium. This confirms that PLA phases are importantly degraded in PBS/PLA/P-Cl blends during degradation experiments at 58°C, which can be observed through the decrease of melting temperature of PLA.
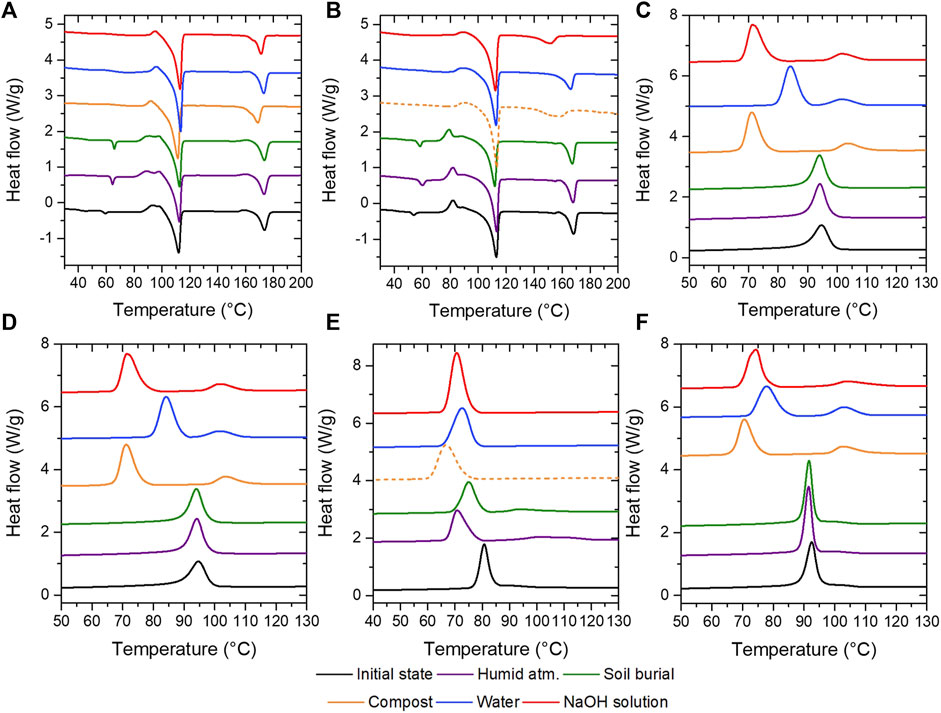
FIGURE 12. Melting curves (first heating ramp) of (A) PBS/PLA, (B) PBS/PLA/P-Cl, and (C) PBS/PLA/A-M blends, and crystallization curves of (D) PBS/PLA, (E) PBS/PLA/P-Cl, and (F) PBS/PLA/A-M blends.
Crystallization of PBS/PLA blends was studied using a 10°C/min cooling ramp. PBS/PLA without additive showed no change of crystallization behavior after degradation at room temperature (under soil burial conditions and in humid atmosphere). The crystallization temperature remains unchanged and is about 94°C. However, after degradation at 58°C, a peak separation is observed. A first peak, seen at about 103°C, probably corresponds to PLA crystallization, and a second peak at lower temperature, corresponds to PBS crystallization. A second peak reaches a maximum at about 71°C after degradation experiment in alkaline water and under composting conditions, while it is at about 84°C after degradation in deionized water. A similar behavior is observed with PBS/PLA/A-M formulation.
PBS/PLA/P-Cl shows a more pronounced crystallization peak separation after degradation in humid atmosphere and under soil burial conditions (Figure 12). PLA melting peak appears to be broad and flat. After degradation at 58°C, this peak is hardly observable. As seen earlier, this is due to PLA degrading and diffusing out of the PBS matrix.
Finally, evolutions of weight losses, molecular weights show that abiotic degradation can be associated with possible biodegradation in presence of microrganisms and that both are enhanced for blends containing P-Cl.
Conclusion
In this study, PBS/PLA blends were processed with ionic liquids P-Cl and A-M, that could act as interfacial agents between PBS and PLA phases. Surprisingly, it was seen that PLA phases in the PBS matrix were considerably larger in PBS/PLA blends with additives than in neat PBS/PLA blend. Furthermore, it was seen, through molecular weight measurements, that phosphonium ionic liquid induced a significant decrease of molecular weight, leading to the formation of PLA oligomers, which induces a lower thermal stability. It had already been observed earlier that phosphonium ionic liquid catalyzes PLA degradation during processing (Park and Xanthos, 2009; Lins et al., 2015). Consequently, PLA oligomers do not provide stiffness to the PBS matrix in PBS/PLA/P-Cl blend. Then, the slight merging of alpha relaxation temperatures, observed in DMA, suggests that small chains of PLA might be miscible in PBS phases, and vice versa. Blend with ammonium IL exhibits a higher thermal stability, and improved mechanical properties, assessing the role of interfacial agent of A-M. The mechanism is not established yet, although the increase of
Degradation experiments conducted at 58°C indicate that the blends degrading faster are the blends undergoing the most important degradation during processing (PBS/PLA/P-Cl>>>PBS/PLA/A-M ≥ PBS/PLA). It was also observed that PLA domains were affected the most during degradation, through the decrease of melting temperature and PLA weight percentage, which, macroscopically, leads to a significant weight loss and to fragmentation. It can easily be assumed that PLA phases play the role of weak points in the matrix, leading to a rapid loss of integrity. This shows that “predegraded” phases in a polymer matrix can accelerate macroscopic degradation, without compromising the whole material’s uses.
However, PLA phases were not affected to the same extent after degradation at room temperature, which was expected since it is well known that PLA do not easily degrade under mesophilic conditions (Agarwal et al., 1998; Itävaara et al., 2002; Yagi et al., 2009). At room temperature, no significant change was observed using DSC, hence it is not clear yet whether any polymer constitutes a weak point. 1H NMR results show no serious change in PBS/PLA proportions. Nevertheless, under soil burial conditions, all blends containing either IL show a more important weight loss than neat PBS/PLA blend.
Regarding SEC measurements, it is observed that the molecular weight loss is very similar in abiotic and biotic conditions at same temperatures, which suggests that hydrolysis is the main degradation mechanism in the studied polyester blends.
This study shows that ionic liquids can be used to tune mechanical properties of polymer blends as well as their (bio)degradability. However, further investigation is needed to understand degradation mechanisms at room temperature. Moreover, it is necessary to identify degradation products and to study their eco-toxicity. Moreover, potential toxicity of ILs has been raising interest in the literature indeed (Zhao et al., 2007; Petkovic et al., 2010; Thuy Pham et al., 2010; Ventura et al., 2012; Hou et al., 2013; El-Harbawi et al., 2014; Ventura et al., 2014; Radošević et al., 2015). Affinity with microorganisms of the tested IL is under study. It can be assumed, through a change in morphology, that ILs induce surface modification, and hence affect interactions of the material with microorganisms.
Data availability statement
The original contributions presented in the study are included in the article/Supplementary Material, further inquiries can be directed to the corresponding author.
Author contributions
Conceptualization, SL and VM; Methodology, SL, AM, ED, VM, RB, and J-FG; Formal Analysis, AM, ED, VM, SL, and J-FG; Investigation, AM, ED, VM, SL, and J-FG; Data Curation, all authors; Validation, all authors; Visualization, ED, AM, and VM; Writing—Original Draft Preparation, ED, VM, and SL; Writing—Review and Editing, all authors; Resources, SL, RB, and VM; Project Administration, SL,VM, and RB. All authors have read and agreed to the published version of the manuscript.
Acknowledgments
The authors would like to thank the French Ministry of High Education and Research for PhD grant funding. The authors wish to thank C. Boule from the Centre Technologique des Microstructures CTµ of the University of Lyon I for TEM micrographs, A. Crepet for Size Exclusion Chromatography (SEC) experiments, Carlos Fernandez de Alba and Fernande da Cruz for NMR experiments, and Pulsalys—SATT Lyon Saint-Etienne for its financial support.
Conflict of interest
The authors declare that the research was conducted in the absence of any commercial or financial relationships that could be construed as a potential conflict of interest.
Publisher’s note
All claims expressed in this article are solely those of the authors and do not necessarily represent those of their affiliated organizations, or those of the publisher, the editors and the reviewers. Any product that may be evaluated in this article, or claim that may be made by its manufacturer, is not guaranteed or endorsed by the publisher.
References
Agarwal, M., Koelling, K. W., and Chalmers, J. J. (1998). Characterization of the degradation of polylactic acid polymer in a solid substrate environment. Biotechnol. Prog. 14 (3), 517–526. doi:10.1021/bp980015p
Andersson, S. R., Hakkarainen, M., and Albertsson, A. C. (2010). Tuning the polylactide hydrolysis rate by plasticizer architecture and hydrophilicity without introducing New migrants. Biomacromolecules 11 (12), 3617–3623. doi:10.1021/bm101075p
Bhatia, A., Gupta, R. K., Bhattacharya, S. N., and Choi, H. J. (2007). Compatibility of biodegradable poly (lactic acid) (PLA) and poly (butylene succinate) (PBS) blends for packaging application. Korea Aust. Rheol. J. 19 (3), 125–131. doi:10.1155/2010/287082
Bordes, P., Pollet, E., and Avérous, L. (2009). Nano-Biocomposites: Biodegradable polyester/nanoclay systems. Prog. Polym. Sci. 34 (2), 125–155. doi:10.1016/j.progpolymsci.2008.10.002
Cho, K., Lee, J., and Kwon, K. (2001). Hydrolytic degradation behavior of poly(butylene succinate)s with different crystalline morphologies. J. Appl. Polym. Sci. 79 (6), 1025–1033.
Delamarche, E., Massardier, V., Bayard, R., and Dos Santos, E. (2020). “A review to guide eco-design of polymer materials,” in Reactive and functional polymers. Editor T. J. Gutierrez (Cham: Springer), 2.
Delamarche, E., Mattlet, A., Livi, S., Gérard, J., Bayard, R., and Massardier, V. (2020). Tailoring biodegradability of poly ( butylene succinate )/poly ( lactic acid ) blends with a deep eutectic solvent. Front. Mat. 7, 1–13. doi:10.3389/fmats.2020.00007
Deng, Y., and Thomas, N. L. (2015). Blending poly(butylene succinate) with poly(lactic acid): Ductility and phase inversion effects. Eur. Polym. J. 71, 534–546. doi:10.1016/j.eurpolymj.2015.08.029
Dorigato, A., Sebastiani, M., Pegoretti, A., and Fambri, L. (2012). Effect of silica nanoparticles on the mechanical performances of poly(lactic acid). J. Polym. Environ. 20 (3), 713–725. doi:10.1007/s10924-012-0425-6
Du, L., Qu, B., and Zhang, M. (2007). Thermal properties and combustion characterization of nylon 6/MgAl-LDH nanocomposites via organic modification and melt intercalation. Polym. Degrad. Stab. 92 (3), 497–502. doi:10.1016/j.polymdegradstab.2006.08.001
El-Harbawi, M., Yusri, Y. S. B., and Hossain, M. I. (2014). Toxicity assessment of phosphonium based ionic liquids towards female guppy fish. Asian J. Chem. 26 (10), 2997–3000. doi:10.14233/ajchem.2014.16276
Elsawy, M. A., Kim, K. H., Park, J. W., and Deep, A. (2017). Hydrolytic degradation of polylactic acid (PLA) and its composites. Renew. Sustain. Energy Rev. 79, 1346–1352. doi:10.1016/j.rser.2017.05.143
Fatou, J. G., and Mandelkern, L. (1965). The effect of molecular weight on the melting temperature and fusion of polyethylene 1. J. Phys. Chem. 69 (2), 417–428. doi:10.1021/j100886a010
Fel, E., Khrouz, L., Massardier, V., Cassagnau, P., and Bonneviot, L. (2016). Comparative study of gamma-irradiated PP and PE polyolefins Part 2: properties of PP/PE blends obtained by reactive processing with radicals obtained by high shear or gamma-irradiation. Polym. Guildf. 82, 217–227. doi:10.1016/j.polymer.2015.10.070
Fortunati, E., Puglia, D., Iannoni, A., Terenzi, A., Kenny, J. M., and Torre, L. (2017). Processing conditions, thermal and mechanical responses of stretchable poly (lactic acid)/poly (butylene succinate) films. Mater. (Basel) 10 (7), 809. doi:10.3390/ma10070809
Freyermouth, F. (2014). Etude et Modification Des Propriétés Du Poly (Butylène Succinate), Un Polyester Biosourcé et Biodégradable. Lyon, France: INSA de Lyon.
Furukawa, T., Sato, H., Murakami, R., Zhang, J., Duan, Y. X., Noda, I., et al. (2005). Structure, dispersibility, and crystallinity of poly(hydroxybutyrate)/poly(L-lactic acid) blends studied by FT-IR microspectroscopy and differential scanning calorimetry. Macromolecules 38 (15), 6445–6454. doi:10.1021/ma0504668
Hou, X. D., Liu, Q. P., Smith, T. J., Li, N., and Zong, M. H. (2013). Evaluation of toxicity and biodegradability of cholinium amino acids ionic liquids. PLoS One 8 (3), e59145. doi:10.1371/journal.pone.0059145
ISO (2012). Determination of the ultimate aerobic biodegradability of plastic materials under controlled composting conditions - Method by analysis of evolved carbon dioxide-Part 1: General method. ISO 14855-1:2012.
Itävaara, M., Karjomaa, S., and Selin, J. F. (2002). Biodegradation of polylactide in aerobic and anaerobic thermophilic conditions. Chemosphere 46 (6), 879–885. doi:10.1016/S0045-6535(01)00163-1
Jiang, L., Wolcott, M. P., and Zhang, J. (2006). Study of biodegradable polylactide/poly (butylene adipate-Co-terephthalate) blends. Biomacromolecules 7, 199–207. doi:10.1021/bm050581q
Kamber, N. E., Tsujii, Y., Keets, K., Waymouth, R. M., Pratt, R. C., Nyce, G. W., et al. (2010). The depolymerization of poly(ethylene terephthalate) (PET) using N-heterocyclic carbenes from ionic liquids. J. Chem. Educ. 87 (5), 519–521. doi:10.1021/ed800152c
Krzan, A., Hemjinda, S., Miertus, S., Corti, A., and Chiellini, E. (2006). Standardization and certification in the area of environmentally degradable plastics. Polym. Degrad. Stab. 91 (12), 2819–2833. doi:10.1016/j.polymdegradstab.2006.04.034
Kopinke, F. D., Remmler, M., Mackenzie, K., Möder, M., and Wachsen, O. (1996). Thermal decomposition of biodegradable polyesters - II. Poly(Lactic acid). Polym. Degrad. Stab. 53 (3), 329–342. doi:10.1016/0141-3910(96)00102-4
Labruyère, C., Talon, O., Berezina, N., Khousakoun, E., and Jérôme, C. (2014). Synthesis of poly(butylene succinate) through oligomerization - cyclization - ROP route. RSC Adv. 4, 38643–38648. doi:10.1039/c4ra03373f
Lins, L. C., Livi, S., Duchet-Rumeau, J., and Gérard, J.-F. (2015). Phosphonium ionic liquids as New compatibilizing agents of biopolymer blends composed of poly(butylene-adipate-Co-Terephtalate)/Poly(Lactic acid) (PBAT/PLA). RSC Adv. 5 (73), 59082–59092. doi:10.1039/C5RA10241C
Livi, S., Bugatti, V., Marechal, M., Soares, B. G., Barra, G. M. O., Duchet-Rumeau, J., et al. (2015). Ionic liquids–lignin combination: An innovative way to improve mechanical behaviour and water vapour permeability of eco-designed biodegradable polymer blends. RSC Adv. 5, 1989–1998. doi:10.1039/C4RA11919C
Livi, S., Bugatti, V., Soares, B. G., and Duchet-Rumeau, J. (2014). Structuration of ionic liquids in a poly(butylene-adipate-Co-terephthalate) matrix: its influence on the water vapour permeability and mechanical properties. Green Chem. 16 (8), 3758–3762. doi:10.1039/c4gc00969j
Livi, S., Duchet-Rumeau, J., Gérard, J. F., and Pham, T. N. (2015). Polymers and ionic liquids: a successful wedding. Macromol. Chem. Phys. 216 (4), 359–368. doi:10.1002/macp.201400425
Luzi, F., Fortunati, E., Jiménez, A., Puglia, D., Pezzolla, D., Gigliotti, G., et al. (2016). Production and characterization of PLA_PBS biodegradable blends reinforced with cellulose nanocrystals extracted from hemp fibres. Ind. Crops Prod. 93, 276–289. doi:10.1016/j.indcrop.2016.01.045
Ma, P. X. (2004). Scaffolds for tissue fabrication. Mat. Today 7 (5), 30–40. doi:10.1016/S1369-7021(04)00233-0
Malmgren, T., Mays, J., and Pyda, M. (2006). Characterization of poly(lactic acid) by size exclusion chromatography, differential refractometry, light scattering and thermal analysis. J. Therm. Anal. Calorim. 83 (1), 35–40. doi:10.1007/s10973-005-7066-0
Makino, K., Ohshima, H., and Kondo, T. (1986). Mechanism of hydrolytic degradation of poly(L-lactide) microcapsules: Effects of pH, ionic strength and buffer concentration. J. Microencapsul. 3 (3), 203–212. doi:10.3109/02652048609031574
Megevand, B., Pruvost, S., Lins, L. C., Livi, S., Gérard, J. F., and Duchet-Rumeau, J. (2016). Probing nanomechanical properties with AFM to understand the structure and behavior of polymer blends compatibilized with ionic liquids. RSC Adv. 6 (98), 96421–96430. doi:10.1039/c6ra18492h
Na, Y. H., He, Y., Shuai, X., Kikkawa, Y., Doi, Y., and Inoue, Y. (2002). Compatibilization effect of poly (ε-Caprolactone)-b-Poly(Ethylene glycol) block copolymers and phase morphology analysis in immiscible poly(lactide)/poly(ε-caprolactone) blends. Biomacromolecules 3 (6), 1179–1186. doi:10.1021/bm020050r
Okamoto, M., and John, B. (2013). Synthetic biopolymer nanocomposites for tissue engineering scaffolds. Prog. Polym. Sci. 38 (10–11), 1487–1503. doi:10.1016/j.progpolymsci.2013.06.001
Ostrowska, J., Sadurski, W., Paluch, M., Tyński, P., and Bogusz, J. (2019). The effect of poly(butylene succinate) content on the structure and thermal and mechanical properties of its blends with polylactide. Polym. Int. 68, 1271–1279. doi:10.1002/pi.5814
Pantani, R., and Sorrentino, A. (2013). Influence of crystallinity on the biodegradation rate of injection-moulded poly(lactic acid) samples in controlled composting conditions. Polym. Degrad. Stab. 98 (5), 1089–1096. doi:10.1016/j.polymdegradstab.2013.01.005
Park, K. I., and Xanthos, M. (2009). A study on the degradation of polylactic acid in the presence of phosphonium ionic liquids. Polym. Degrad. Stab. 94 (5), 834–844. doi:10.1016/j.polymdegradstab.2009.01.030
Petkovic, M., Ferguson, J. L., Gunaratne, H. Q. N., Ferreira, R., Leitão, M. C., Seddon, K. R., et al. (2010). Novel biocompatible cholinium-based ionic liquids - toxicity and biodegradability. Green Chem. 12 (4), 643–649. doi:10.1039/b922247b
Qiu, T. Y., Song, M., and Zhao, L. G. (2016). Testing, characterization and modelling of mechanical behaviour of poly (Lactic-Acid) and poly (butylene succinate) blends. Mech. Adv. Mat. Mod. Process. 2 (1), 7. doi:10.1186/s40759-016-0014-9
Quitadamo, A., Massardier, V., Valente, M., Ayoub, A. S., and Lucia, L. A. (2017). “Oil-based and bio-derived thermoplastic polymer blends and composites,” in Introduction to renewable biomaterials. Editors A. S. Ayoub, and L. A. Lucia (Washington, DC: American Chemical Society), 239–268.
Radošević, K., Cvjetko Bubalo, M., Gaurina Srček, V., Grgas, D., Landeka Dragičević, T., and Redovniković, R. I. (2015). Evaluation of toxicity and biodegradability of choline chloride based deep eutectic solvents. Ecotoxicol. Environ. Saf. 112, 46–53. doi:10.1016/j.ecoenv.2014.09.034
Sánchez-Soto, P. J., Ginés, J. M., Arias, M. J., Novák, C., and Ruiz-Conde, A. (2002). Effect of molecular mass on the melting temperature, enthalpy and entropy of hydroxy-terminated PEO. J. Therm. Anal. Calorim. 67 (1), 189–197. doi:10.1023/A:1013758518721
Shibata, M., Inoue, Y., and Miyoshi, M. (2006). Mechanical properties, morphology, and crystallization behavior of blends of poly(l-lactide) with poly(butylene succinate-Co-l-Lactate) and poly(butylene succinate). Polymer 47 (10), 3557–3564. doi:10.1016/j.polymer.2006.03.065
Schliecker, G., Schmidt, C., Fuchs, S., and Kissel, T. (2003). Characterization of a homologous series of D,L-lactic acid oligomers; a mechanistic study on the degradation kinetics in vitro. Biomaterials 24 (21), 3835–3844. doi:10.1016/s0142-9612(03)00243-6
Song, X., Wang, H., Yang, X., Liu, F., Yu, S., and Liu, S. (2014). Hydrolysis of poly(lactic acid) into calcium lactate using ionic liquid [bmim] [OAc] for chemical recycling. Polym. Degrad. Stab. 110, 65–70. doi:10.1016/j.polymdegradstab.2014.08.020
Su, S., Kopitzky, R., Tolga, S., and Kabasci, S. (2019). Polylactide (PLA) and its blends with poly(butylene succinate) (PBS): a brief review. Polym. (Basel) 11 (7), 1193–1214. doi:10.3390/polym11071193
Taubner, V., and Shishoo, R. (2001). Influence of processing parameters on the degradation of poly(L-lactide) during extrusion. J. Appl. Polym. Sci. 79 (12), 2128–2135. doi:10.1002/1097-4628(20010321)79:12<2128::AID-APP1020>3.0.CO;2-#
Thuy Pham, T. P., Cho, C. W., and Yun, Y. S. (2010). Environmental fate and toxicity of ionic liquids: a review. Water Res. 44 (2), 352–372. doi:10.1016/j.watres.2009.09.030
Ventura, S. P. M., e Silva, F. A., Gonçalves, A. M. M., Pereira, J. L., Gonçalves, F., and Coutinho, J. A. P. (2014). Ecotoxicity analysis of cholinium-based ionic liquids to Vibrio fischeri marine bacteria. Ecotoxicol. Environ. Saf. 102 (1), 48–54. doi:10.1016/j.ecoenv.2014.01.003
Ventura, S. P. M., Marques, C. S., Rosatella, A. A., Afonso, C. A. M., Gonçalves, F., and Coutinho, J. A. P. (2012). Toxicity assessment of various ionic liquid families towards Vibrio fischeri marine bacteria. Ecotoxicol. Environ. Saf. 76 (1), 162–168. doi:10.1016/j.ecoenv.2011.10.006
Wang, X., Zhou, J., and Li, L. (2007). Multiple melting behavior of poly(butylene succinate). Eur. Polym. J. 43 (8), 3163–3170. doi:10.1016/j.eurpolymj.2007.05.013
Wang, Y. P., Xiao, Y. J., Duan, J., Yang, J. H., Wang, Y., and Zhang, C. L. (2016). Accelerated hydrolytic degradation of poly(lactic acid) achieved by adding poly(butylene succinate). Polym. Bull. 73 (4), 1067–1083. doi:10.1007/s00289-015-1535-9
Wu, D., Yuan, L., Laredo, E., Zhang, M., and Zhou, W. (2012). Interfacial properties, viscoelasticity, and thermal behaviors of poly(butylene succinate)/polylactide blend. Ind. Eng. Chem. Res. 51 (5), 2290–2298. doi:10.1021/ie2022288
Wu, S. (1987). formation of dispersed phase in incompatible polymer blends: interfacial and rheological effects. Polym. Eng. Sci. 27 (5), 335–343. doi:10.1002/pen.760270506
Wu, S. (1985). Phase structure and adhesion in polymer blends: a criterion for rubber toughening. Polymer 26 (12), 1855–1863. doi:10.1016/0032-3861(85)90015-1
Xiang, S., Jun, S., Li, G., Bian, X. C., Feng, L. D., Chen, X. S., et al. (2016). Effects of molecular weight on the crystallization and melting behaviors of poly(L-lactide). Chin. J. Polym. Sci. 34 (1), 69–76. doi:10.1007/s10118-016-1727-2
Xie, J., Zhang, K., Wu, J., Ren, G., Chen, H., and Xu, J. (2016). Bio-nanocomposite films reinforced with organo-modified layered double hydroxides: preparation, morphology and properties. Appl. Clay Sci. 126, 72–80. doi:10.1016/j.clay.2016.02.025
Xu, J., and Guo, B.-H. (2010a). “Microbial succinid acid, its polymer poly(butylene succinate), and applications,” in Plastics from bacteria: natural functions and applications. Editor G. Q. Chen (Berlin, Heidelberg: Springer-Verlag), 14, 85–119. doi:10.1007/978-3-642-03287
Xu, J., and Guo, B. H. (2010b). Poly(butylene succinate) and its copolymers: Research, development and industrialization. Biotechnol. J. 5 (11), 1149–1163. doi:10.1002/biot.201000136
Yagi, H., Ninomiya, F., Funabashi, M., and Kunioka, M. (2009). Anaerobic biodegradation tests of poly(lactic acid) under mesophilic and thermophilic conditions using a New evaluation system for methane fermentation in anaerobic sludge. Int. J. Mol. Sci. 10 (9), 3824–3835. doi:10.3390/ijms10093824
Yokohara, T., and Yamaguchi, M. (2008). Structure and properties for biomass-based polyester blends of PLA and PBS. Eur. Polym. J. 44 (3), 677–685. doi:10.1016/j.eurpolymj.2008.01.008
Yu, L., Dean, K., and Li, L. (2006). Polymer blends and composites from renewable Resources. Prog. Polym. Sci. 31 (6), 576–602. doi:10.1016/j.progpolymsci.2006.03.002
Zhang, S. J., Tang, Y. W., and Cheng, L. H. (2013). Biodegradation behavior of PLA/PBS blends. Adv. Mat. Res. 821–822, 937–940. doi:10.4028/www.scientific.net/amr.821-822.937
Zhao, D., Liao, Y., and Zhang, Z. D. (2007). Toxicity of ionic liquids. Clean. Soil Air Water 35 (1), 42–48. doi:10.1002/clen.200600015
Keywords: PLA, PBS, ionic liquids, thermal properties, morphologies, (bio)degradation
Citation: Delamarche E, Mattlet A, Livi S, Gérard J-F, Bayard R and Massardier V (2022) Impact of Ionic Liquids on the (bio)degradability of Poly(butylene succinate)/Poly(lactic acid) blends. Front. Mater. 9:975438. doi: 10.3389/fmats.2022.975438
Received: 22 June 2022; Accepted: 29 July 2022;
Published: 12 September 2022.
Edited by:
Miroslav Slouf, Institute of Macromolecular Chemistry (ASCR), CzechiaReviewed by:
Kasama Jarukumjorn, Suranaree University of Technology, ThailandPiotr Rychter, Jan Długosz University, Poland
Copyright © 2022 Delamarche, Mattlet, Livi, Gérard, Bayard and Massardier. This is an open-access article distributed under the terms of the Creative Commons Attribution License (CC BY). The use, distribution or reproduction in other forums is permitted, provided the original author(s) and the copyright owner(s) are credited and that the original publication in this journal is cited, in accordance with accepted academic practice. No use, distribution or reproduction is permitted which does not comply with these terms.
*Correspondence: Valérie Massardier, valerie.massardier-nageotte@insa-lyon.fr