- Department of Spine Surgery, The First Hospital of Jilin University, Changchun, China
Bone defects, which can be caused by factors such as trauma, tumor, or osteomyelitis, are clinically common. They lessen the weight a bone is able to bear and cause severe pain to the patient. Although bone transplantation is the gold standard for treating bone defects, it is not suitable for all patients due to its poor availability, risk of spreading disease, and possibility of requiring a secondary surgery. Bone cement as a filler for bone defects can fill any shape of bone defect, and can quickly solidify when injected, providing mechanical strength sufficient for supporting the normal physiological activities of the bone. However, traditional bone cement lacks the ability to induce bone regeneration. Recently, various methods for enhancing the bone regeneration ability of bone cement have been developed, such as adding bone morphogenetic proteins, mesenchymal stem cells, and inorganic substances to bone cement. These methods not only ensure the original biological properties of the bone cement, but also improve the bone cement in terms of its mechanical strength and ability to induce bone regeneration. The aim of this review is to overview the process of bone regeneration, introduce improved bone cement formulations designed to promote bone regeneration, and discuss the clinical application of bone cement and its possibilities for future improvement.
Introduction
Bone has a strong regenerative capacity under normal conditions. The repair potential of bone and its surrounding microenvironment, including inflammatory cells, endothelial cells, and Schwann cells, persists throughout adulthood, enabling damaged bone tissues to return to their homeostatic functional state (Schlundt et al., 2021). However, this self-healing method can only repair small bone defects. Infection, trauma, and bone tumor resection cause large bone defects; so, the normal physiological structure of the bone cannot be regained without medical intervention (Gillman and Jayasuriya, 2021). Autologous bone grafting, allogeneic bone grafting, and xenografting are the best ways to solve bone defects in clinic, among which autologous bone grafting is considered the gold standard for bone grafting (Schmidt, 2021). Since an autologous bone has the same function as the bone surrounding the defect, the success rate and fitting rate of autologous bone grafting are high; thus, autologous bone is an ideal filler for repairing bone defects. However, the availability of autologous bone for transplantation is limited, and not everyone is a good candidate for autologous bone grafting. Moreover, infection and massive blood loss with autologous bone grafting can occur at the donor site (Myerson et al., 2019). These unfavorable factors limit the widespread application of autologous bone grafting. Bone allografts and xenografts, although widely available, are costly and difficult due to strict requirements for aseptic preservation and aseptic implantation. Furthermore, allografts and xenografts still pose a risk of disease transmission (Artas et al., 2018; P Baldwin et al., 2019; Hurle et al., 2021), and bone xenografts can even lead to immune rejection. Therefore, bone grafting methods are gradually moving toward the use of biomaterials to repair bone defects.
Biomaterials suitable to replace bone grafts need to have several properties (Elgali et al., 2017). The first is biological histocompatibility; that is, the material must be able to adapt to the injury environment in the local bone defect, and it must be compatible with the surrounding bone tissues and extracellular matrix so as to avoid damaging surrounding cells. A biocompatible scaffold can provide a favorable microenvironment for cell migration, proliferation, and differentiation, while possessing a degradation rate that matches bone repair and regeneration (García-Gareta et al., 2015; Zhu et al., 2021a). The second is pores conducive to the transport of nutrient metabolites and the inward regeneration of bone. Studies have shown that a small pore size is not conducive to cell infiltration and migration, and a large pore size cannot provide sufficient attachment area for bone regeneration (Zhu et al., 2021a). Excessive pore size is also not conducive to the stability of the filler. The third is mechanical tolerance; that is, the filler should locally adapt to the mechanical strength of the surrounding bone. This can be achieved through a phenomenon called mechanotransduction, in which cells respond to mechanical signals from their surroundings (Dupont et al., 2011). The fourth is being capable of inducing osteogenesis; bone cement can include bone morphogenetic proteins (BMPs) or other factors conducive to bone regeneration to achieve this (Li and Liu, 2017).
In recent years, many bio-tissue engineering materials for bone repair and regeneration have emerged, such as bone blocks (Chaushu et al., 2019), bone chips, bioglass (Duan et al., 2020), and implantable bandages for inducing osteogenic differentiation (Okuchi et al., 2021). These fillers are good substitutes for autologous bone and xenografts for bone defect repair. However, bone blocks need to be cut according to the shape and size of the defect during the operation, which increases the operation time and is not suitable for clinical application (Chaushu et al., 2019). Transplantable bandages induce bone regeneration but fail to compensate for mechanical strength in bone defects (Okuchi et al., 2021). The poor toughness of bioglass is also not conducive to topical application (Duan et al., 2020). Although 3D printing technology can be used to fabricate fillers with good mechanical strength, printing must be started a few days before surgery (Liaw and Guvendiren, 2017). Compared to these fillers, bone cement has unique advantages as a filler for small bone defects. Bone cement can fill the bone defect at the injection site and set quickly to provide good mechanical strength (Palmer et al., 2016). This can save operation time. Moreover, bone cement has good cytocompatibility and biocompatibility and can induce osteogenesis (Bimis et al., 2017). However, it has some serious potential complications, including bone cement implantation syndrome (BCIS) (Hines, 2018). BCIS can lead to severe cardiac arrhythmias, hypotensive shock, and even cardiac arrest. In addition, bone cement can leak into the surrounding environment, such as the venous system, which can lead to cardiac embolism (Song et al., 2020). Although the incidence of these adverse reactions is low, researchers still need to improve the setting properties of bone cement. The addition of natural organic substances such as alginate, chitosan, and gelatin to bone cement can improve the toughness, mechanical strength, and porosity of bone cement, thereby enhancing the ability of bone cement to induce bone regeneration and reducing its sequelae (Liu et al., 2021a; Wu et al., 2021). New bone formation is mainly caused by the joint action of osteoblasts, osteoclasts, and extracellular matrix at the site of injury (Katsimbri, 2017). Bone cement is relatively weak in regulating the osteogenic microenvironment. In recent years, platelet-rich plasma (PRP), an osteogenic growth factor, has been added to bone cement to promote bone repair (Hakimi et al., 2010; Shen et al., 2021; Tian et al., 2021). This new type of bone cement not only retains the mechanical strength and biocompatibility of the original bone cement but also provides growth factors for bone formation (Scheme 1; Table1, 2). In this review article, we introduce new types of bone cement developed to promote bone repair and regeneration, and we discuss the clinical application of bone cement, as well as possible directions for future improvement.
Bone regeneration
Bone regeneration is usually accomplished by the mutual regulation of osteoblasts, mesenchymal stem cells (MSCs) and extracellular matrix (ECM).
Osteoblasts
Osteoblasts are the only cells that can generate bone in vertebrates. Osteoblasts produce collagen to provide a scaffold for matrix mineralization. When the bone tissue matures, osteoblasts are embedded in the bone matrix and undergo structural changes to become osteocytes (Bonewald, 2011). After bone formation, active osteoblasts quiescent on the bone surface gradually evolve into bone lining cells. When a bone defect occurs, the quiescent bone lining cells on the bone surface turn into osteoblasts. Bone-lining cells are an important source of osteoblasts in adulthood (Mizoguchi and Ono, 2021). Osteoclasts, which are derived from hematopoietic stem cells, are capable of bone resorption. Under normal conditions, osteoblasts and osteoclasts are in relative balance in vivo to regulate osteogenesis and development (McDonald et al., 2021). When a bone defect occurs, the balance of osteoblasts and osteoclasts is disrupted. Induces bone mineral deposition through the regulation of calcium and phosphorus.
Mesenchymal stem cells
Stem cells have a strong ability to self-renew and differentiate into specific cells in the body. MSCs are the most commonly used stem cells for the treatment of bone diseases in preclinical research and clinical work (Saeed et al., 2016). MSCs in the bone marrow can differentiate into osteoblasts, adipocytes, or chondrocytes depending on the environment in which they exist. MSCs are considered precursors of osteoblasts and modulators of osteoclasts (Shang et al., 2020). The differentiation of MSCs into osteoblasts mainly depends on BMPs (Ponzetti and Rucci, 2021). BMPs have been reported to recruit bone marrow mesenchymal stromal/stem cells (BMMSCs) to the resorbed site during bone resorption to prevent bone resorption (Bal et al., 2020). MSCs reach the damaged site to generate a primary cartilaginous callus, which subsequently undergoes revascularization and calcification to gradually form normal bone tissues (Yorukoglu et al., 2017; Shang et al., 2020). Intramembranous osteogenesis and endochondral osteogenesis of bone regeneration depend on MSCs (Gresham et al., 2020).
Bone extracellular matrix
ECM is secreted by cells into the matrix and is mainly composed of hydroxyapatite, collagen fibers (type I in bone tissues and type II in cartilages), and trace elements (Gresham et al., 2020). As a scaffold for cell adhesion and proliferation, the ECM is primarily responsible for bone strength. Bone ECM dynamically interacts with osteoblasts and osteoclasts to regulate new bone formation during regeneration (Alcorta-Sevillano et al., 2020; Lin et al., 2020). In addition, studies have found that ECM has a unique ability to induce osteogenesis through type I collagen (Komatsu et al., 2018). Hydroxyapatite is the main inorganic component of bone tissues with osteoinductive and osteoconductive properties. Angiogenesis precedes osteogenesis during bone regeneration (Oliveira et al., 2021). Various cytokines can be used in bone regeneration delivery technology to modulate the ECM to promote bone regeneration, such as platelet-derived growth factors (PDGFs), BMPs, insulin-like growth factors (IGFs), transforming growth factors (TGFs-ß), vascular endothelial growth factors (VEGFs), and osteoinductive GFs (Khojasteh et al., 2013). Therefore, the currently common bone cements that promote bone regeneration mainly regulate osteoblasts, MSCs and ECM. Next, we will introduce the currently commonly used bone cements to promote bone repair.
Improve bone cement
Polymethyl methacrylate bone cement
Polymethyl methacrylate (PMMA), a thermoplastic synthetic polymer synthesized by the polymerization of MMA monomers, has excellent properties, such as transparency and good tensile strength, mechanical properties, and processability (Forte et al., 2021). PMMA is widely used clinically in corneal transplantation (Talati et al., 2018), cranioplasty (Siracusa et al., 2021), denture repair (Tieh et al., 2021), vertebral fracture repair (Patel et al., 2021), and joint replacement (Rupp et al., 2021). However, PMMA bone cement hinders bone formation due to biological inertness, non-degradability, and potential cytotoxicity. High polymerization temperatures also lead to the death of surrounding osteoblasts and osteoblast-related cells, and the subsequent formation of a fibrous membrane hinders the osseointegration of PMMA cement to bone (Li et al., 2020a). Studies have found that bone tissue necrosis occurs within 1 min under conditions of more than 50° (Li et al., 2020a). In addition, free radicals from monomers after polymerization are a major source of adverse events (Saruta et al., 2021; Paz et al., 2019). Based on these unfavorable factors, PMMA cannot form biological bone tissues locally. Therefore, research on modified bone cement based on PMMA has been conducted in recent years (as Table1).
Bone morphogenetic protein-2 (BMP-2) is an important osteogenic growth factor in bone tissue regeneration. The technical difficulty encountered in using BMP-2 to repair bone defects is in delivering BMP-2 to the defect site while maintaining its biological activity and local survival for a long time (Shen et al., 2021). De Witte et al. (2020) prepared degradable PMMA nanoparticles for the delivery of BMP-2. P (MMA-co-MAA) nanoparticles optimized for the controlled delivery of BMP-2. The hydrophobicity of PMMA and the hydroxyl groups on the surface of MMA increased the affinity of the nanoparticles for BMP-2. The addition of ester groups in the cross-linking increased the degradability of the nanoparticles and facilitated the release of BMP-2. This improved method not only promoted the degradation of PMMA but also increased the delivery of BMP-2. The study confirmed that the nanoparticles had a 100% BMP-2 loading rate and had the potential to promote osteogenesis and angiogenesis to form vascularized bone (De Witte et al., 2020). Although nanoparticles can deliver BMP-2 to promote bone repair, they cannot change the unfavorable factors of PMMA bone cement itself, such as the lack of osseointegration and high elastic modulus (Rho et al., 2012). Mineralized collagen (MC) consists of type I collagen fibers and hydroxyapatite. The use of MC to improve PMMA in the treatment of osteoporotic pyramidal compression fractures has been reported (Hakimi et al., 2010). Zhu et al. (2021b) improved PMMA bone cement for the treatment of cone collapse using MC (Zhu et al., 2019). Compared with PMMA bone cement, MC-PMMA reduces the elastic modulus of the original PMMA bone cement and possesses biological activity more suitable for bone regeneration. Furthermore, it was found via computed tomography (CT) that the MC in MC-PMMA bone cement was replaced by new bone, and the new bone was more stable than PMMA. The incidence of re-fractures was also significantly reduced in patients after the application of MC-modified PMMA bone cement. However, not all mineralization improvements to bone cement can improve the performance of bone cement. For example, adding hydroxyapatite to improve PMMA reduces the compressive strength of bone cement (Kim et al., 2004). Wang et al. used magnesium-based polylactic acid microsheets to modify PMMA. This modification not only reduced the damage caused by PMMA to osteoblasts due to high polymerization temperature but also released magnesium ions to promote osteogenesis. In addition, the microsphere structure on the surface after lactate dehydrogenase degradation was also beneficial for bone formation (Figure 1) (Wang et al., 2021). In addition, Sharma et al. improved PMMA bone cement with amine group-functionalized graphene, which reduced cytotoxicity and improved the toughness of bone cement. Significant calcification was observed 20 days after bone injury (Saeed et al., 2016; Tavakoli et al., 2020). PMMA bone cement can also be improved by mixing it with magnesium oxide or calcium phosphate since these compounds promote bone formation (Zhang et al., 2018; Li et al., 2020b).
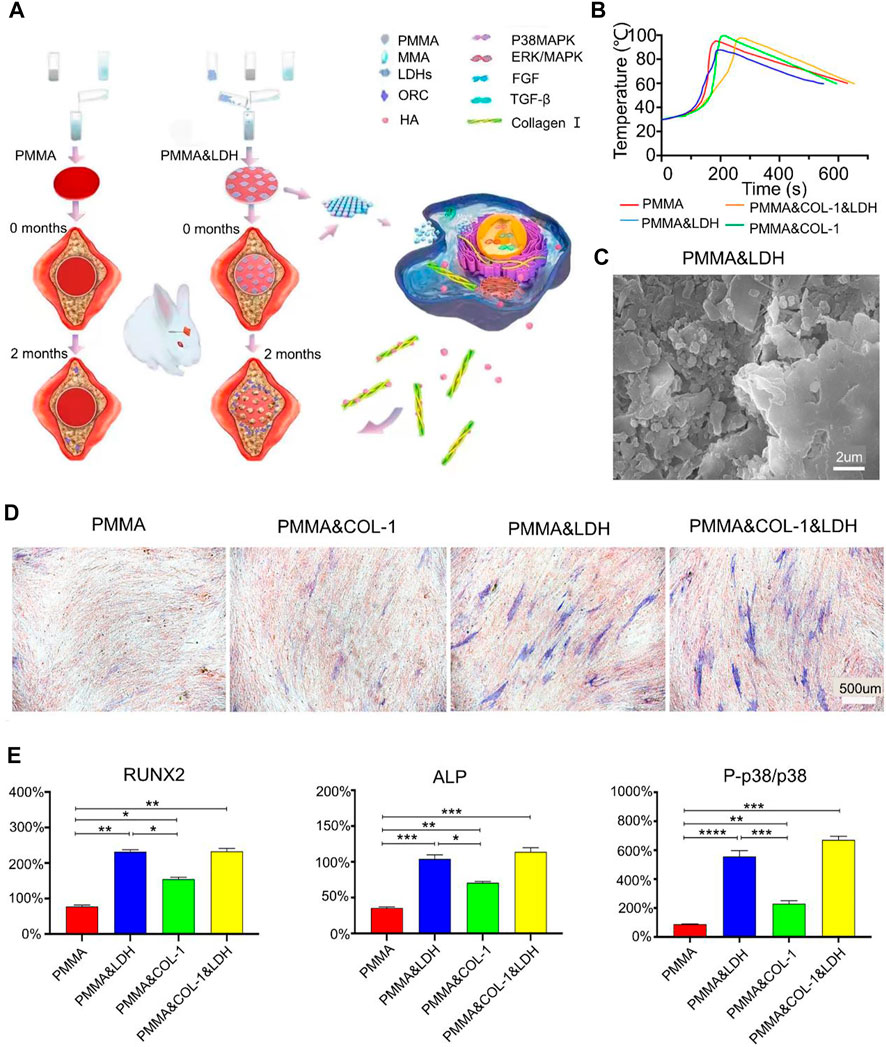
FIGURE 1. (A) Schematic illustration of layered double hydroxide-modified bone cement for promoting bone repair. (B) Temperature change during the reaction of the B polymer. (C) Scanning electron microscope image of PMMA and LDH. (D) Osteogenic differentiation ability was assessed by alkaline phosphatase (ALP) staining assay on day 14 of in vitro cell experiments (blue stained area represents alkaline phosphatase). (E) In vivo assay to assess osteogenic differentiation. Relative gene transcript levels of osteogenic markers (i.e., runt-related transcription factor 2 (Runx2), ALP, p38, and P-p38) in human bone marrow-derived mesenchymal stem cells on day 7. An increased ratio of P-p38 to p38 indicates the activation of the p38 MAPK signaling pathway. Reproduced with permission from (Wang et al., 2021).
In conclusion, various inorganic materials can be mixed with PMMA bone cement to promote bone repair and regeneration. However, the porosity, biocompatibility, and mechanical strength obtained by mixing different ratios of PMMA with inorganic materials are different. In clinical practice, we can design bone cements with different characteristics according to the needs of different diseases for clinical selection.
Calcium phosphate cement
Calcium phosphate has unique biocompatibility, osteoconductivity, and bone-mineral-rich fractions that make it a promising bone-replacement material (Best et al., 2008). Calcium phosphate bone cement has plasticity and can be set into a suitable shape to fill bone defects as needed. However, calcium phosphate cement has a dense structure and poor biodegradability, which is not conducive to the ingrowth of living tissues. Additionally, its mechanical strength cannot meet the load-bearing capacity of the bone in the defect (Lobenhoffer et al., 2002; Liu et al., 2019). In recent years, researchers have optimized the properties of calcium phosphate bone cement to ensure that it has good biocompatibility and bone regeneration ability (as Table 2).
Tan et al. improved calcium phosphate bone cement using MgO (Tan et al., 2021). MgO is added to maintain the morphological structure after in situ curing. It was found that the addition of MgO reduced the temperature released during the curing process of bone cement, and released magnesium ions promoted the increase of M2 macrophages in the anti-inflammatory immune microenvironment (Gu et al., 2021). Macrophages persist at the site of injury for 2–3 weeks, providing a favorable environment for bone repair. At the same time, a large number of osteoblasts are present around the microspheres at the 12th week. Macrophages, as inflammatory and immune-related cells, can release growth factors and chemokines (such as BMP-2, BMP-4, and TGF-β1) in bone defects to promote the recruitment of fibroblasts to the defect (Yang and Liu, 2021). However, studies show that macrophages are not involved in the early stages of fracture healing (Schlundt et al., 2018).
Alendronate sodium, the most commonly used drug for the treatment of osteoporosis, increases bone mineral density (Vertesich et al., 2021). Hence, researchers have speculated whether the application of alendronate sodium can maintain the bone density of the bone defect in the early stage. In an animal study, mice treated with alendronate for 4 weeks exhibited a 139% increase in bone volume (Vertesich et al., 2021). In one study, alendronate was added to calcium phosphate bone cement, and poly (lactic-co-glycolic acid) (PLGA) was used to make porogen to promote the degradation of CPC/PLGA to release alendronate and form a porous structure. Although the calcium phosphate cement itself did not degrade, alendronate was released from the modified cement for up to 148 days. When alendronate sodium is clinically used for the treatment of osteoporosis patients, it promotes local bone formation. However, the alendronate released from the cement did not increase bone formation within the defect. Instead, new bone formation was detected around the defect area. Although there was no good explanation, the researchers speculated that it may have been due to the lack of alendronate sodium to induce the migration of preosteoblast cells to the center of the defect (van Houdt et al., 2018).
Raja et al. (2021) replaced alendronate with quercetin and used calcium-deficient hydroxyapatite-alginate to fabricate core-shell bone cement (Raja et al., 2021). Quercetin is to control the metabolism of bone tissue regeneration through sustained release over a long period of time. Alginate is an anionic polysaccharide that changes shape when cross-linked. The purpose of the core-shell structure was to deliver preosteoblast cells to the site of bone injury. Cells were loaded on core-shell beads for 50 days (Figure 2). Cell growth was evident at the center of the nucleocapsid structure, but not in alginate. In addition, it was found that adding antibiotics could also control local infection, which is beneficial to bone formation (Liu et al., 2021a). Trace elements such as magnesium ions, zinc ions, strontium ions, and copper ions can also regulate the phenotype of macrophages and promote bone repair and regeneration (Lin et al., 2019; Liang et al., 2020; Miao et al., 2020; Li et al., 2021a; Tao et al., 2021).
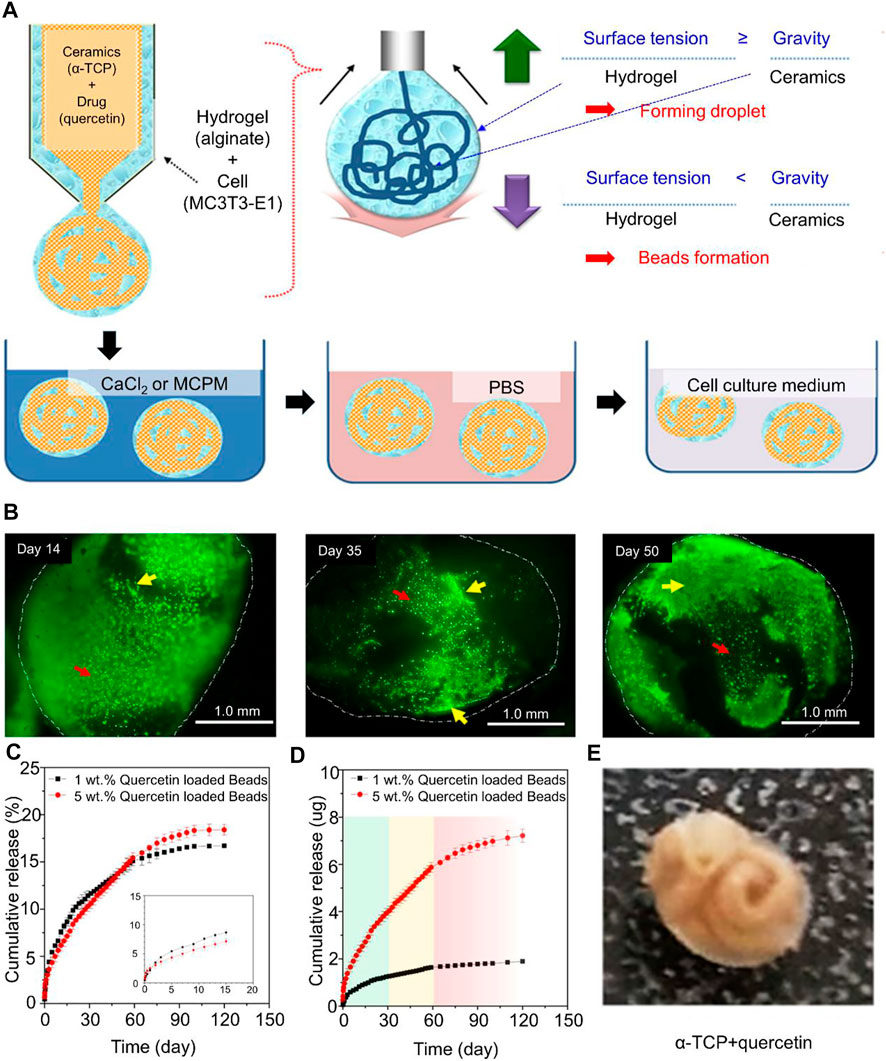
FIGURE 2. (A) Schematic of the processing of core−shell beads. (B) Fluorescence microscopy images of live/dead cells in core−shell beads (indicated with white dotted lines) cemented in PBS for 14, 35, and 50 days. Yellow arrows indicate cells showing growth and elongation, and red arrows indicate cells that retain their spherical shape in alginate. (C) Drug release profile from core−shell beads over the course of 120 days with different concentrations of quercetin. (D) Cumulative drug release at specific time points (in days). 0–30 days (green region): fast drug release, followed by decreased but stable release; 30–60 days (yellow region): constant and stable drug release; and 60–120 days (red region): declining drug release with time. (E) X-ray diffraction patterns of final core−shell beads after the addition of quercetin. Reproduced with permission from (Raja et al., 2021).
A simple bone cement material has insufficient pores in the middle, and osteocytes cannot grow toward the center of the bone cement; so, stable new bone cannot be formed. Degradable particles mixed with bone cement can remedy the lack of voids in bone cement. Biphasic calcium phosphate bone cements (BCPCs) composed of hydroxyapatite and tricalcium phosphate have good biodegradability (Gauthier et al., 2001; Rattanachan et al., 2020). Lee et al. prepared a bone substitute material (CPC-ccMCG-B) by adsorbing BMP-2 and porous particles to the surface of HAp through collagen fibers (Lee et al., 2017). The incorporation of porous particles facilitates the maintenance of the biocompatibility of the material. The study found that CPC-ccMCG-B provided sustained release of BMP-2 over 30 days. Compared with pure calcium phosphate cement, CPC-ccMCG-B can also significantly induce angiogenesis. Neovascularization helps osteoblasts recruit and form new bone in bone defects. A significant increase in the quantity of trabecular bone was observed within 4 weeks of implantation. However, the liquid-to-powder ratio (L/P) affected the compressive strength of CPC-ccMCG-B. The higher the L/P ratio, the greater the fluidity of the cementitious material and MCG, and the lower the compressive strength of CPC-ccMCG-B (Lee et al., 2017). Adding chitosan fiber as a reinforcing material to bone cement can improve the compressive strength of bone cement (Lee et al., 2019; Miao et al., 2020; Rattanachan et al., 2020).
MSCs have excellent inductive differentiation potential, which is conducive to promoting bone regeneration (Saeed et al., 2016). Li et al. cultured autologous BMMSCs for 7 days and encapsulated them in calcium phosphate bone cement in combination with PRP (Li et al., 2021b). PRP releases growth factors such as PDGF, TGF-β1, and VEGF, which benefit angiogenesis and macrophage activation, thereby stimulating host bone formation (Hakimi et al., 2010). This new bone cement has better mechanical properties and excellent biocompatibility than previous CPC. Although different implantation methods have certain negative effects on PRP-induced bone regeneration, the combination with stem cells is beneficial for bone healing (Li et al., 2021b). At 6 and 12 weeks after implantation, calcium phosphate cement mixed with BMMSCs and PRP had stronger osteogenic ability than calcium phosphate cement alone. Although the internal loading factors of bone cement can promote bone regeneration, the surface compatibility of calcium phosphate bone cement is still a problem. Dopamine-encapsulated strontium-doped calcium phosphate improves the mechanical properties of bone cement and enhances the cytocompatibility of the cement surface with surrounding bone tissues (Wang et al., 2020).
In conclusion, calcium phosphate bone cement is currently the most commonly used inorganic bone substitute material, and it still has great untapped potential. Thus far, it has been demonstrated that chitosan, alginate, and inorganic ions can be used to improve the mechanical strength and porosity of calcium phosphate bone cement. However, a major technical difficulty remaining to be solved is in delivering growth factors to the defect site and in increasing the duration of action while ensuring their survival rate in order to enhance bone defect repair.
Magnesium phosphate cement
Magnesium phosphate bone cement is known for its rapid setting, high strength, and rapid biodegradation capabilities (Mestres and Ginebra, 2011). In the process of bone repair, the ability of magnesium ions to promote the proliferation and differentiation of osteoblasts gives magnesium phosphate bone cement unique advantages over other types of bone cements (Wang et al., 2014). Although not as rich in bone minerals as calcium phosphate bone cement, magnesium phosphate bone cement can still have a wide range of applications due to the magnesium ions. However, under humid conditions in the body, the phosphate in the magnesium phosphate cement matrix will dissolve, resulting in changes in the surrounding pH and a decrease in the mechanical strength of the cement (Liu et al., 2019). This is undesirable since mechanical strength is an important factor for bone defect repair. Moreover, magnesium phosphate bone cement will generate a lot of heat before setting, and this heat will damage the cells around the defect, causing these cells to lose their ability to repair bone (Ostrowski et al., 2016). Therefore, magnesium phosphate cement alone is not a good material for local filling in bone repair. Reducing the changes to pH caused by magnesium phosphate bone cement is a relatively difficult work; so, there have been few studies on improving magnesium phosphate bone cement.
Magnesium phosphate and ammonium ions can form struvite with high absorbability under neutral conditions (Fuchs et al., 2021). Struvite has high mechanical strength, which can, to a certain extent, compensate for the decrease in mechanical strength caused by pH changes. However, the synthesis of struvite results in amine emissions (Wenisch et al., 2003), which is a difficult problem to solve. Gong et al. (2020) used potassium dihydrogen phosphate (KH2PO4) as a source of phosphate to solve the problem of amine emissions and simultaneously synthesized K-struvite. They also introduced non-toxic and degradable natural oxy-carboxymethyl chitosan (O-CMC) into magnesium phosphate cement (MPC), denoting the product as OMPC, to improve the cement’s compressive strength. The addition of oxygen-carboxymethyl chitosan enhances the mechanical properties and cytocompatibility of potassium MPC. The study confirmed that the improvement in compressive strength was related to the added O-CMC. The porosity of the OMPC-2.5% group is the lowest at 13.8%, while the compressive strength reaches the maximum value of 33.8 MPa. The study also demonstrated that OMPC released magnesium ions to promote cell proliferation and bone repair. Furthermore, O-CMC reduced the pH value of the solution, which made up for the decrease in bone cement strength caused by phosphate dissolution (Figure 3) (Gong et al., 2020).
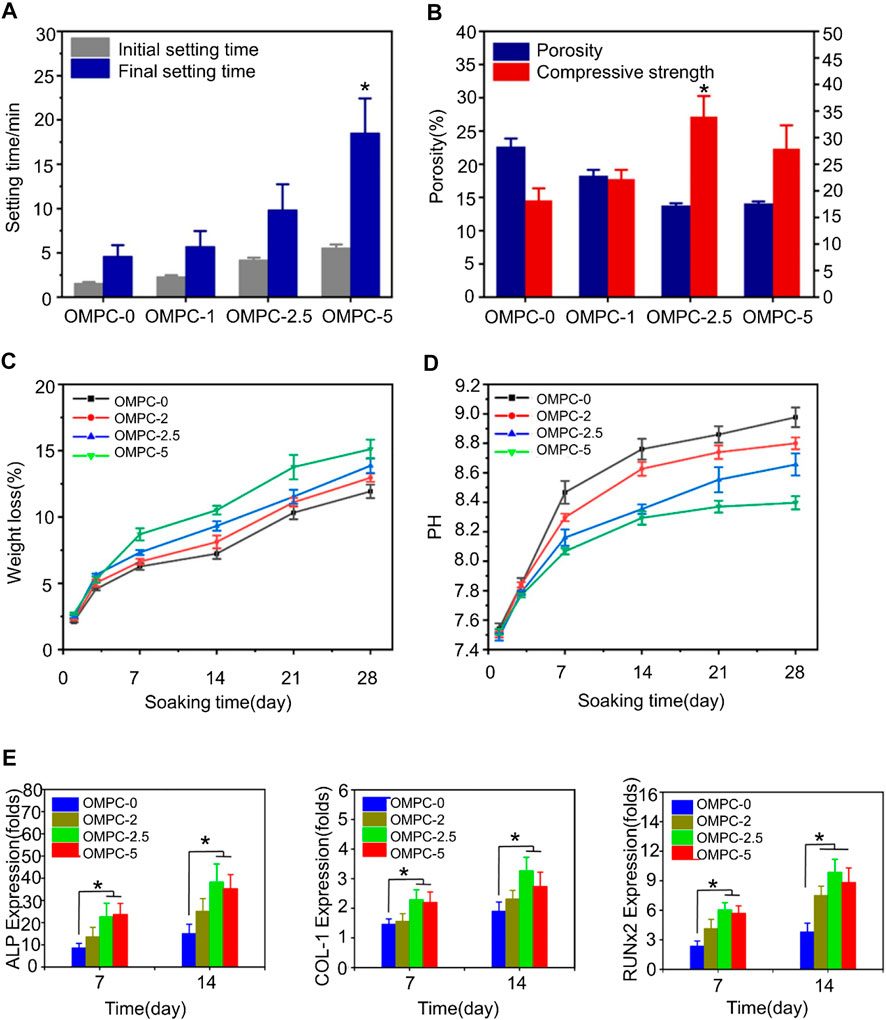
FIGURE 3. (A) Clotting time of OMPC scaffolds. (B) Porosity and compressive strength of OMPC scaffolds. (C) In vitro degradation of OMPC scaffolds. In vitro weight loss rate of OMPC scaffolds immersed in Tris-HCl solution. The first 3 days had the fastest degradation rate, with a loss of about 5% of the total weight of all OMPC groups. On day 28, the weight reduction rate of OMPC-5 reached 15.11 wt%. (D) pH values for all OMPC groups. The incorporation of O-CMC slightly lowered the final pH of OMPC. (E) Osteogenic differentiation results of MC3T3-E1 cells cultured on OMPC scaffolds. The expression levels of osteogenic differentiation-related genes were measured on days 7 and 14. Reproduced with permission from (Gong et al., 2020).
Although potassium magnesium phosphate bone cement has many advantages, its short clotting time is not conducive to clinical application (Wang et al., 2019). Shi mixed different proportions of chondroitin sulfate (CS) with MPC to synthesize new CS-MPC composites (Shi et al., 2021). CS promotes calcium deposition in the extracellular matrix and osteogenic differentiation of BMMSCs (Manton et al., 2007). It was found that 2.5% CS-MPC had the highest compressive strength, at 29.67 ± 1.45 MPa, and the lowest porosity, at 12.2%. Cells on CS-MPCs were detected on days 3 and 5, and it was found that the expression levels of osteogenesis-related genes COL1, RUNX2, and OCN were also higher than those in the MPC group. In addition, magnesium phosphate powder was added to calcium phosphate bone cement to prepare premixed cement CaxMg(3-x) (PO4)2 (x = 0.25 and 0.75) (Ewald et al., 2019). It was found that the porosity of premixed bone cement in PBS in vitro increased to 16%–25% after 30 days without a consequent decrease in compressive strength. This may be related to the release of the oil phase. In addition, citrate can inhibit hydration and improve the mechanical strength of bone cement, and it can also regulate the energy metabolism pathway of MSCs to induce osteogenic differentiation and angiogenesis of MSCs (Ma et al., 2018; Binu et al., 2013; Hurle et al., 2018). Wu et al. loaded citrate into magnesium phosphate bone cement and found that it not only improved the curing time, compressive strength, and cytocompatibility of bone cement but also promoted peripheral vascular and osteogenic differentiation (Wu et al., 2020).
In conclusion, although magnesium phosphate bone cement has its unique advantages, it is difficult to improve and has significant negative effects. Hence, little research on improving magnesium phosphate bone cement has been conducted. Although natural bioactive substances can make up for certain drawbacks, magnesium phosphate bone cement is still rarely used clinically. Some researchers have mixed calcium phosphate cement with magnesium phosphate cement to prepare a new type of cement. This new type of bone cement has certain minerals and mechanical strength, but it is difficult to manufacture. Different ratios of calcium phosphate and magnesium phosphate will need to be comprehensively assessed in order to guide the development of new bone cements that meet specific needs. Overall, magnesium phosphate bone cement still requires much development before it becomes suitable for clinical application.
Portland bone cement
Portland cement is widely used in the treatment of dental pulp, and its main components are tricalcium silicate (C3S) and Dicalcium silicate (C2S) (Kaur et al., 2017). C3S and C2S can stimulate the proliferation and osteogenic differentiation of bone, and they both have good biocompatibility. However, Portland cement has low resistance to early compression and a long setting time, which do not meet the clinical requirements of bone defect repair (Wu et al., 2015). When C2S and C3S come in contact with water, silicic acid and calcium hydroxide are immediately produced. Phosphate and calcium hydroxide form hydroxyapatite (Sharma et al., 2020). Ding et al. (2018) mixed magnesium glycerophosphate (MG) with Portland cement to synthesize a new type of bone cement. MG can not only promote the degradation of bone cement to release phosphate but can also release magnesium necessary for bone regeneration. Glycerol phosphate is the basic substance of metabolism throughout the life cycle. The study showed that the compressive strength of bone cement reached 30 MPa on the 7th day, and hydroxyapatite was formed on the surface, which provided a good foundation for new bone formation (Ding et al., 2018). In addition, studies have shown that chitosan can induce silicate bone cement to upregulate osteogenic markers and induce matrix mineralization (Subhi et al., 2021). However, Portland cement is hydraulically hard and reduces porosity over time (Camilleri et al., 2014). Once the porosity is reduced, it is not conducive to cell growth and nutrient metabolism. A possible solution is to use 3D printed scaffolds to tune pore morphology and porosity as needed (Pei et al., 2016). Yang et al. (2017) used silicate bone cement to prepare scaffolds to enhance bone repair and regeneration (Figure 4). The formation of nano-needle-like structures on the surface of scaffold pores was conducive to the attachment and diffusion of bone marrow stem cells. The scaffolds had a maximum compressive strength of 12.9 MPa and a porosity of 61%. This porosity is too high, which is a common problem among 3D printed scaffolds. Although larger porosity is beneficial for cell growth, it leads to a decrease in compressive strength (Yang et al., 2017). Studies have shown that although the compressive strength of this 3D-printed scaffold is not as good as that of calcium phosphate cement, it has exceeded that reported in other literature (0.9–8.7 MPa) (Camilleri et al., 2014; Zuleta et al., 2017). This type of scaffold is only suitable for the repair of non-load-bearing bones.
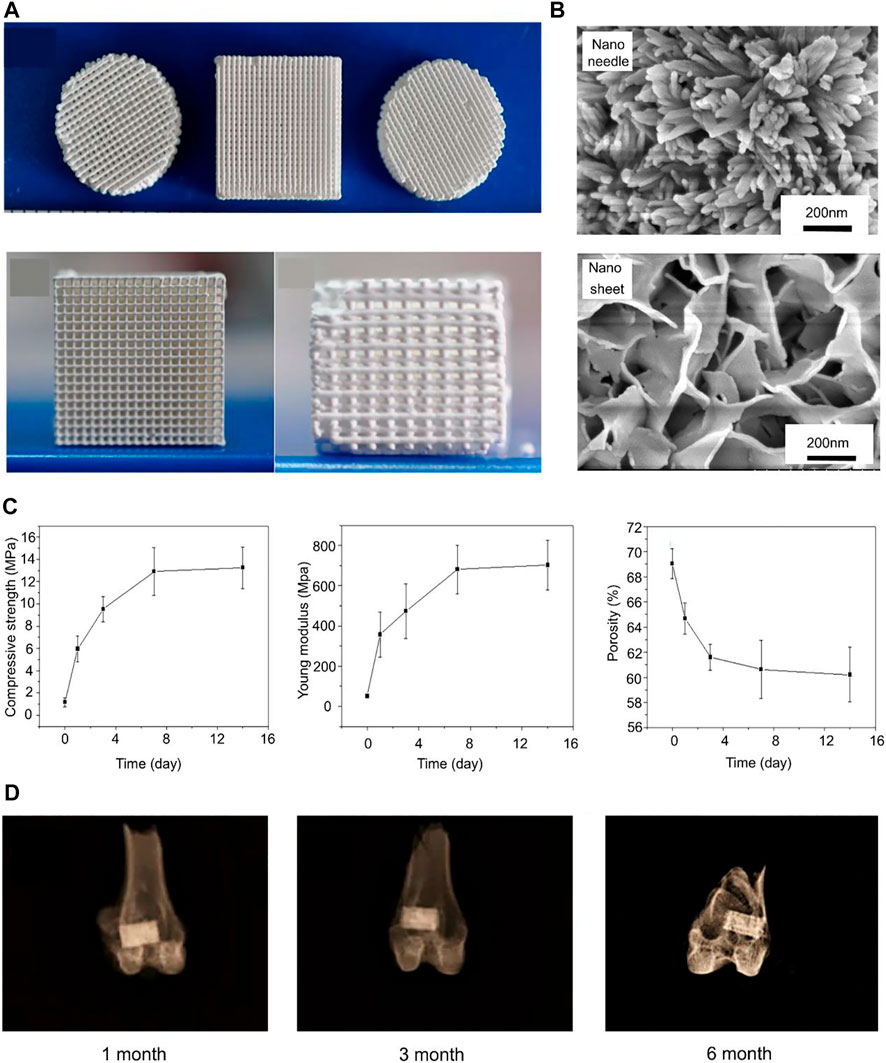
FIGURE 4. (A) 3D printed C3S bone cements with different shapes. (B) Nanoneedle and nanosheet structures formed by C3S bone cement scaffolds in Na2HPO4 aqueous solution and (NH4)2HPO4 aqueous solution. (C) Compressive strength, Young’s modulus, and porosity of C3S scaffolds at different curing times (0, 1, 3, 7, 14 days). (D) X-rays of C3S–NN scaffolds after being implanted for 1 month, 3 months, and 6 months. Reproduced with permission from (Yang et al., 2017).
Mesoporous bioactive glasses (MBGs), most of which are silicates, have excellent biocompatibility and tunable surface porosity (Wu and Chang, 2012). In bone regeneration, MBGs can promote the expression of osteogenesis-related genes (Wu et al., 2010). However, MBGs are brittle at low sintering temperatures, leading to rapid collapse of the porous structure (Ghamor-Amegavi et al., 2020). Gou et al. developed yolk-shell granule-like bone cement with silicate and MBG for promoting bone regeneration (Ghamor-Amegavi et al., 2020). This bidirectional calcium phosphate bone cement rapidly degraded and released MBGs in rabbits. The highest bone volume fractions (BV/TV) were shown at 8–12 weeks post-implantation. Bone fragility caused by osteoporosis was also lessened (Wang et al., 2014). In another study, MBG was mixed with calcium sulfate to form a high BG content injectable BG/calcium sulfate composite cement. The composite bone cement degraded slowly, and the mass loss of calcium sulfate cement reached 52% after soaking in simulated body fluid (SBF) for 4 weeks. Although its compressive strength is not high, it satisfies the bone repair of vertebral body compression fractures (Zhu et al., 2017). As previously mentioned, metal ions can improve the biological properties of Portland cement. Copper ions, for example, can induce bone marrow mesenchymal cells to release hypoxia-inducible factor and vascular endothelial growth factor, which contribute to the revascularization of bone defects (Bejarano et al., 2017). Copper ion-doped hydroxyapatite exhibits antibacterial activity and promotes bone regeneration (Zhang et al., 2020).
Portland cement is not as widely used as calcium phosphate cement. However, for small bone defects, such as those in teeth, Portland cement still has a certain application value. Due to its low compressive strength, Portland cement cannot be used for the defect repair of limb bones. Improved Portland cement can be used to repair vertebral fractures, but its application is currently limited to animals. Additionally, silicate bone cement needs to be further improved to expand its clinical applicability.
Borate/borosilicate bone cement
Borate bone cement is a kind of bioactive glass made by partially or totally replacing SiO2 with B2O3 (Huang et al., 2006). The degradation rate can be regulated by changing the ratio of B2O3 and SiO2. Boron can reduce the hydration temperature and delay the solidification reaction. Borate cement with vancomycin can repair 87% of bone defects in the treatment of osteomyelitis (Cui et al., 2014). Strontium (Sr) can stimulate the expression of the MSC solid gene and inhibit the activity of osteoclasts to promote bone regeneration (Zhang et al., 2015). Zhang developed an injectable glass bone cement (Sr-BBG) by mixing strontium and chitosan with borate bone cement (Figure 5) (Zhang et al., 2015). Bone cement has good biological activity, osteogenesis capacity and the release of Sr in a controlled manner. The setting time of Sr-BBG bone cement was 10.6 ± 1.2 min. The expression of osteogenesis-related genes could be detected within 7 days in vivo. Increasing the proportion of strontium resulted in longer setting times but did not affect the compressive strength. In addition, the new bone formation and implant contact index of Sr-BBG were significantly better than those of BBG (Cui et al., 2020). In view of the rapid setting of magnesium phosphate bone cement, Li et al. incorporated magnesium phosphate into borosilicate bone cement to prepare a composite bone cement (MPC-BG). The study found that the coagulation time of magnesium phosphate bone cement was delayed after adding BG. However, the compressive strength was affected when the MPC/BG ratio exceeded 3:1 (Li et al., 2020a).
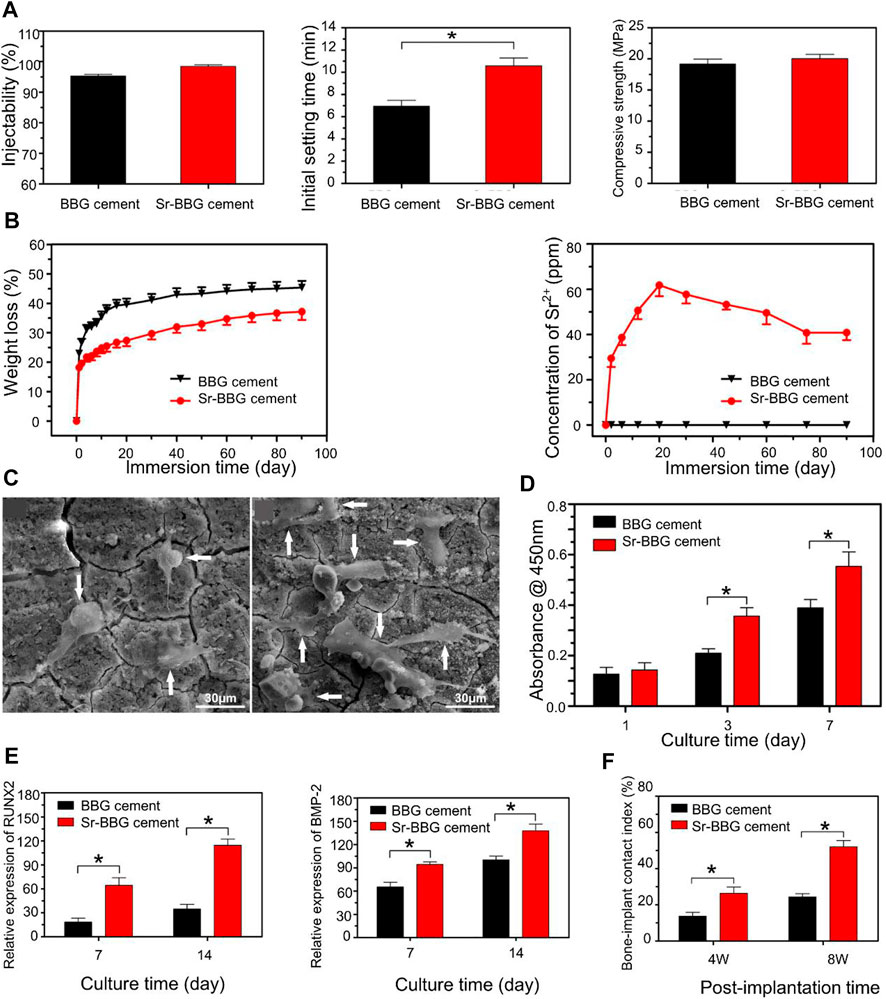
FIGURE 5. (A) Physical properties of BBG and Sr-BBG cements: injectability, initial setting time, and compressive strength. (B) Weight loss of BBG and Sr-BBG cement, and the amount of Sr2+ ions released from the Sr-BBG cement into the SBF. (C) SEM images showing the attachment of human bone marrow-derived mesenchymal stem cells (hBMSCs) on BBG and Sr-BBG cement after culturing for 3 days. (D) CCK8 proliferation experiments on days 1, 3, and 7: hBMSCs cultured on BBG and Sr-BBG cement. (E) The expression levels of osteogenic differentiation-related genes were measured on days 7 and 14. (F) Newly formed bone and bone-implant contact (BIC) index of BBG and Sr-BBG cement implanted at 4 and 8 weeks in critical-sized rabbit femoral condyle defects. Reproduced with permission from (Zhang et al., 2015).
Although borosilicate-loaded growth factors have achieved certain success in promoting bone repair, their inherent stability is poor. Most borate cements are used to improve the mechanical strength of PMMA cements (Cui et al., 2017; Funk et al., 2018; Cole et al., 2020), since it is still difficult to use borosilicate bone cement alone in clinical practice.
Calcium aluminate bone cement
Calcium aluminate cement has higher mechanical properties and a lower curing temperature than calcium phosphate cement (Oh et al., 2004). However, this kind of bone cement has a long setting time, which is not conducive to clinical application. A study found that the addition of lithium chloride can reduce the setting time of calcium aluminate cement without affecting the compressive strength of the cement (Acuña-Gutiérrez et al., 2017). In vivo experiments also confirmed the absence of cytotoxicity after the addition of lithium chloride. However, this modification method cannot ensure that there are sufficient calcium ions around the cement to promote bone repair. Calcium chloride modified calcium aluminate bone cement can ensure that calcium aluminate bone cement releases calcium ions for 84 h (Castro-Raucci et al., 2018). Nonetheless, calcium aluminate cements are not as useful as other types of cements, possibly due to their inferior fabrication methods and biohistocompatibility compared to other types of bone cements. Among the few calcium aluminate cements that have been studied, most have been used to improve other types of bone cements.
Inorganic nanohybrid bone cement
In addition to the above-mentioned bone cements commonly used in clinical practice, polymer bone cements also have unique advantages. Unlike traditional bone cements, the crosslinking and stabilization of these polymers depend on thienyl groups and double bonds (Liu et al., 2021b). In order to speed up the formation of chemical bonds, it is often necessary to use catalysts to speed up the reaction process. However, most of the catalysts contain aromatic groups or heavy metal ions that are harmful to the body. Strain-promoted alkyne-azide cycloaddition (SPAAC) click chemistry is a mild and fast click reaction (Gordon et al., 2012). This reaction can be accomplished without the addition of initiators and catalysts. Liu et al. developed an organic-inorganic nanohybrid (click-ON) injectable bone cement system using SPAAC technology. The hybrid was mainly composed of propylene glycol fumarate and esters. Studies have shown that with different ratios of the two substances, the gelation time of the hybrid varies from a few minutes to 30 min. In vivo experiments indicated that on the 21st day, the content of MSCs and osteogenic markers was significantly increased. Significant osteogenesis was observed 4 weeks after the scaffold was injected. Compared with PMMA bone cement, the hybrid has low toxicity, strong degradability, and can promote the proliferation and differentiation of MSCs.
Polymer bone cement has the advantages of low toxicity and easy availability, which traditional bone cement does not have, and it also has advantages for in vivo osteogenesis (Tang et al., 2021). Whether it can provide compressive strength around the bone defect is questionable since no studies have reported on this topic. We speculate that polymer bone cement probably has lower strength compared to traditional bone cement. Therefore, polymer bone cement needs to be further improved to promote its clinical application.
Challenges and limitations
To date, PMMA, calcium phosphate, and magnesium phosphate bone cements have been widely used in the field of orthopedics. However, these traditional bone cements have drawbacks that limit their clinical application. The regeneration of bone into normal bone tissue is a complex process. Traditional bone cements provide support for bone regeneration, but they do not promote the induction of bone regeneration. Today, biomaterials have made great breakthroughs in tissue engineering. Traditional bone cements can be improved by natural bioactive substances such as chitosan and alginate to form new types of bone cements that are more stable and that have greater mechanical strength while retaining the other mechanical properties of bone cement. Improved bone cement with the ability to induce bone regeneration can also be developed. Loading osteogenic growth factor on bone cement can form a microenvironment favorable for bone formation in situ. Appropriate porosity not only satisfies the compressive strength of new bone but also facilitates the inward formation of new bone. Better osteogenic properties of new types of bone cements have been observed in animal models. Therefore, new bone cements have great promise for clinical application in bone repair.
Future perspective and conclusion
However, the optimal mixing ratios of conventional bone cements and natural active substances remain unknown. The mechanical strength and porosity provided by mixtures of different proportions are different, and the characteristics of bone cement required for repairing various bone defects are also different. There is an urgent need for a new type of bone cement suitable for repairing most bone defects in clinical practice. To date, Improved bone cements have only been validated in animals. Furthermore, new types of bone cements can repair small bone defects, but it remains unclear whether they can repair large bone defects. For large bone defects, the repair time after bone cement filling is long, and patients may need a second operation or even autologous bone implantation, which is troubling for both patients and clinicians. At present, for large bone defects, only 3D printing stent technology can provide a good basis for treatment. Therefore, there is still an urgent need for bone cement suitable for repairing large bone defects.
Data availability statement
The original contributions presented in the study are included in the article/supplementary material, further inquiries can be directed to the corresponding author.
Author contributions
All authors read and approved the final manuscript. YX wrote the initial manuscript. CF and YX contributed new ideas. HW created the figures. YL and HW created Table 1. YX, HY, and YL revised the manuscript and approved the final version.
Funding
This work was supported by the National Natural Science Foundation of China (Grant Nos. 82071391), the Science and Technology Development Program of Jilin Province (Grant No. 20200404182YY), the Provincial Health Special Project of Jilin Province (Grant No. JLSWSRCZX 2020-104), the “13th Five-Year” Science and Technology Research Planning Project of Jilin Province (Grant No. JLKHJJKH20190042KJ), and the Achievement Transformation Fund of the First Hospital of Jilin University (Grant No. JDYYZH-2102052).
Acknowledgments
We would like to express our appreciation to everyone who was involved in the drafting and preparation of the manuscript.
Conflict of interest
The authors declare that the research was conducted in the absence of any commercial or financial relationships that could be construed as a potential conflict of interest.
Publisher’s note
All claims expressed in this article are solely those of the authors and do not necessarily represent those of their affiliated organizations, or those of the publisher, the editors and the reviewers. Any product that may be evaluated in this article, or claim that may be made by its manufacturer, is not guaranteed or endorsed by the publisher.
References
Acuña-Gutiérrez, I. O., Escobedo-Bocardo, J. C., Almanza-Robles, J., Cortes-Hernandez, D., Saldivar-Ramirez, M., Resendiz-Hernandez, P., et al. (2017). Development of LiCl-containing calcium aluminate cement for bone repair and remodeling applications. Mater. Sci. Eng. C 70, 357–363. doi:10.1016/j.msec.2016.09.022
Alcorta-Sevillano, N., Macías, I., Infante, A., and Rodríguez, C. I. (2020). Deciphering the relevance of bone ECM signaling. Cells 9 (12), 2630. doi:10.3390/cells9122630
Artas, G., Gul, M., Acikan, I., Kirtay, M., Bozoglan, A., Simsek, S., et al. (2018). A comparison of different bone graft materials in peri-implant guided bone regeneration. Braz. oral Res. 32, e59. doi:10.1590/1807-3107bor-2018.vol32.0059
Bal, Z., Kushioka, J., Kodama, J., Kaito, T., Yoshikawa, H., Korkusuz, P., et al. (2020). BMP and TGFβ use and release in bone regeneration. Turk. J. Med. Sci. 50 (SI-2), 1707–1722. doi:10.3906/sag-2003-127
Bejarano, J., Detsch, R., Boccaccini, A. R., and Palza, H. (2017). PDLLA scaffolds with Cu- and Zn-doped bioactive glasses having multifunctional properties for bone regeneration. J. Biomed. Mat. Res. A 105 (3), 746–756. doi:10.1002/jbm.a.35952
Best, S. M., Porter, A. E., Thian, E. S., and Huang, J. (2008). Bioceramics: Past, present and for the future. J. Eur. Ceram. Soc. 28 (7), 1319–1327. doi:10.1016/j.jeurceramsoc.2007.12.001
Bimis, A., Canal, L. P., Karalekas, D., and Botsis, J. (2017). On the mechanical characteristics of a self-setting calcium phosphate cement. J. Mech. Behav. Biomed. Mat. 68, 296–302. doi:10.1016/j.jmbbm.2017.02.017
Binu, S., Soumya, S. J., and Sudhakaran, P. R. (2013). Metabolite control of angiogenesis: Angiogenic effect of citrate. J. Physiol. Biochem. 69 (3), 383–395. doi:10.1007/s13105-012-0220-9
Bonewald, L. F. (2011). The amazing osteocyte. J. Bone Min. Res. 26 (2), 229–238. doi:10.1002/jbmr.320
Camilleri, J., Laurent, P., and About, I. (2014). Hydration of Biodentine, Theracal LC, and a prototype tricalcium silicate-based dentin replacement material after pulp capping in entire tooth cultures. J. Endod. 40 (11), 1846–1854. doi:10.1016/j.joen.2014.06.018
Castro-Raucci, L. M. S., Teixeira, L. N., Barbosa, A. F. S., Fernandes, R. R., Raucci-Neto, W., Jacobovitz, M., et al. (2018). Calcium chloride-enriched calcium aluminate cement promotes in vitro osteogenesis. Int. Endod. J. 51 (6), 674–683. doi:10.1111/iej.12883
Chaushu, L., Chaushu, G., Kolerman, R., Vered, M., Naishlos, S., and Nissan, J. (2019). Anterior atrophic mandible restoration using cancellous bone block allograft. Clin. Implant Dent. Relat. Res. 21 (5), 903–909. doi:10.1111/cid.12744
Cole, K. A., Funk, G. A., Rahaman, M. N., and McIff, T. E. (2020). Mechanical and degradation properties of poly(methyl methacrylate) cement/borate bioactive glass composites. J. Biomed. Mat. Res. 108 (7), 2765–2775. doi:10.1002/jbm.b.34606
Cui, X., Huang, C., Zhang, M., Ruan, C., Peng, S., Li, L., et al. (2017). Enhanced osteointegration of poly(methylmethacrylate) bone cements by incorporating strontium-containing borate bioactive glass. J. R. Soc. Interface 14 (131), 20161057. doi:10.1098/rsif.2016.1057
Cui, X., Zhang, Y., Wang, J., Huang, C., Wang, Y., Yang, H., et al. (2020). Strontium modulates osteogenic activity of bone cement composed of bioactive borosilicate glass particles by activating Wnt/β-catenin signaling pathway. Bioact. Mater. 5 (2), 334–347. doi:10.1016/j.bioactmat.2020.02.016
Cui, X., Zhao, C., Gu, Y., Li, L., Wang, H., Huang, W., et al. (2014). A novel injectable borate bioactive glass cement for local delivery of vancomycin to cure osteomyelitis and regenerate bone. J. Mat. Sci. Mat. Med. 25 (3), 733–745. doi:10.1007/s10856-013-5122-z
De Witte, T. M., Wagner, A. M., Fratila-Apachitei, L. E., Zadpoor, A. A., and Peppas, N. A. (2020). Degradable poly(methyl methacrylate)-co-methacrylic acid nanoparticles for controlled delivery of growth factors for bone regeneration. Tissue Eng. Part A 26 (23-24), 1226–1242. doi:10.1089/ten.tea.2020.0010
Ding, Z., Li, H., Wei, J., Li, R., and Yan, Y. (2018). Developing a novel magnesium glycerophosphate/silicate-based organic-inorganic composite cement for bone repair. Mater. Sci. Eng. C 87, 104–111. doi:10.1016/j.msec.2018.03.001
Duan, H., Cao, C., Wang, X., Tao, J., Li, C., Xin, H., et al. (2020). Magnesium-alloy rods reinforced bioglass bone cement composite scaffolds with cortical bone-matching mechanical properties and excellent osteoconductivity for load-bearing bone in vivo regeneration. Sci. Rep. 10 (1), 18193. doi:10.1038/s41598-020-75328-7
Dupont, S., Morsut, L., Aragona, M., Enzo, E., Giulitti, S., Cordenonsi, M., et al. (2011). Role of YAP/TAZ in mechanotransduction. Nature 474 (7350), 179–183. doi:10.1038/nature10137
Elgali, I., Omar, O., Dahlin, C., and Thomsen, P. (2017). Guided bone regeneration: Materials and biological mechanisms revisited. Eur. J. Oral Sci. 125 (5), 315–337. doi:10.1111/eos.12364
Ewald, A., Kreczy, D., Bruckner, T., Gbureck, U., Bengel, M., Hoess, A., et al. (2019). Development and bone regeneration capacity of premixed magnesium phosphate cement pastes. Mater. (Basel, Switz. 12 (13), 2119. doi:10.3390/ma12132119
Forte, M. A., Silva, R. M., Tavares, C. J., and E Silva, R. F. (2021). Is poly(methyl methacrylate) (PMMA) a suitable substrate for ALD? Polym. (Basel). 13 (8), 1346. doi:10.3390/polym13081346
Fuchs, A., Kreczy, D., Bruckner, T., Gbureck, U., Stahlhut, P., Bengel, M., et al. (2021). Bone regeneration capacity of newly developed spherical magnesium phosphate cement granules. Clin. Oral Investig. 26, 2619–2633. doi:10.1007/s00784-021-04231-w
Funk, G. A., Burkes, J. C., Cole, K. A., Rahaman, M. N., and McIff, T. E. (2018). Antibiotic elution and mechanical strength of PMMA bone cement loaded with borate bioactive glass. J. Bone Jt. Infect. 3 (4), 187–196. doi:10.7150/jbji.27348
García-Gareta, E., Coathup, M. J., and Blunn, G. W. (2015). Osteoinduction of bone grafting materials for bone repair and regeneration. Bone 81, 112–121. doi:10.1016/j.bone.2015.07.007
Gauthier, O., Goyenvalle, E., Bouler, J. M., Guicheux, J., Pilet, P., Weiss, P., et al. (2001). Macroporous biphasic calcium phosphate ceramics versus injectable bone substitute: A comparative study 3 and 8 weeks after implantation in rabbit bone. J. Mat. Sci. Mat. Med. 12 (5), 385–390. doi:10.1023/a:1011284517429
Ghamor-Amegavi, E. P., Yang, X., Qiu, J., Xie, L., Pan, Z., Wang, J., et al. (2020). Composition control in biphasic silicate microspheres on stimulating new bone regeneration and repair of osteoporotic femoral bone defect. J. Biomed. Mat. Res. 108 (2), 377–390. doi:10.1002/jbm.b.34396
Gillman, C. E., and Jayasuriya, A. C. (2021). FDA-approved bone grafts and bone graft substitute devices in bone regeneration. Mater. Sci. Eng. C 130, 112466. doi:10.1016/j.msec.2021.112466
Gong, C., Fang, S., Xia, K., Chen, J., Guo, L., and Guo, W. (2020). Enhancing the mechanical properties and cytocompatibility of magnesium potassium phosphate cement by incorporating oxygen-carboxymethyl chitosan. Regen. Biomater. 8 (1), rbaa048. doi:10.1093/rb/rbaa048
Gordon, C. G., Mackey, J. L., Jewett, J. C., Sletten, E. M., Houk, K. N., and Bertozzi, C. R. (2012). Reactivity of biarylazacyclooctynones in copper-free click chemistry. J. Am. Chem. Soc. 134 (22), 9199–9208. doi:10.1021/ja3000936
Gu, Y., Xie, X., Zhuang, R., Weir, M. D., Oates, T. W., Bai, Y., et al. (2021). A biphasic calcium phosphate cement enhances dentin regeneration by dental pulp stem cells and promotes macrophages M2 phenotype in vitro. Tissue Eng. Part A 27 (17-18), 1113–1127. doi:10.1089/ten.tea.2020.0257
H Gresham, R. C., Bahney, C. S., and Leach, J. K. (2020). Growth factor delivery using extracellular matrix-mimicking substrates for musculoskeletal tissue engineering and repair. Bioact. Mater. 6 (7), 1945–1956. doi:10.1016/j.bioactmat.2020.12.012
Hakimi, M., Jungbluth, P., Sager, M., Betsch, M., Herten, M., Becker, J., et al. (2010). Combined use of platelet-rich plasma and autologous bone grafts in the treatment of long bone defects in mini-pigs. Injury 41 (7), 717–723. doi:10.1016/j.injury.2009.12.005
Huang, W., Day, D. E., Kittiratanapiboon, K., and Rahaman, M. N. (2006). Kinetics and mechanisms of the conversion of silicate (45S5), borate, and borosilicate glasses to hydroxyapatite in dilute phosphate solutions. J. Mat. Sci. Mat. Med. 17 (7), 583–596. doi:10.1007/s10856-006-9220-z
Hurle, K., Oliveira, J. M., Reis, R. L., Pina, S., and Goetz-Neunhoeffer, F. (2021). Ion-doped brushite cements for bone regeneration. Acta Biomater. 123, 51–71. doi:10.1016/j.actbio.2021.01.004
Hurle, K., Weichhold, J., Brueckner, M., Gbureck, U., Brueckner, T., and Goetz-Neunhoeffer, F. (2018). Hydration mechanism of a calcium phosphate cement modified with phytic acid. Acta Biomater. 80, 378–389. doi:10.1016/j.actbio.2018.09.002
Katsimbri, P. (2017). The biology of normal bone remodelling. Eur. J. Cancer Care (Engl). 26 (6), e12740. doi:10.1111/ecc.12740
Kaur, M., Singh, H., Dhillon, J. S., Batra, M., and Saini, M. (2017). MTA versus biodentine: Review of literature with a comparative analysis. J. Clin. Diagn. Res. 11 (8), ZG01–ZG05. doi:10.7860/JCDR/2017/25840.10374
Khojasteh, A., Behnia, H., Naghdi, N., Esmaeelinejad, M., Alikhassy, Z., and Stevens, M. (2013). Effects of different growth factors and carriers on bone regeneration: A systematic review. Oral Surg. Oral Med. Oral Pathology Oral Radiology 116 (6), e405–e423. doi:10.1016/j.oooo.2012.01.044
Kim, S. B., Kim, Y. J., Yoon, T. L., Park, S. A., Cho, I. H., et al. (2004). The characteristics of a hydroxyapatite–chitosan–PMMA bone cement. Biomaterials 25 (26), 5715–5723. doi:10.1016/j.biomaterials.2004.01.022
Komatsu, N., Kajiya, M., Motoike, S., Takewaki, M., Horikoshi, S., Iwata, T., et al. (2018). Type I collagen deposition via osteoinduction ameliorates YAP/TAZ activity in 3D floating culture clumps of mesenchymal stem cell/extracellular matrix complexes. Stem Cell. Res. Ther. 9 (1), 342. doi:10.1186/s13287-018-1085-9
Lee, G. H., Makkar, P., Paul, K., and Lee, B. (2017). Incorporation of BMP-2 loaded collagen conjugated BCP granules in calcium phosphate cement based injectable bone substitutes for improved bone regeneration. Mater. Sci. Eng. C 77, 713–724. doi:10.1016/j.msec.2017.03.296
Lee, H. J., Kim, B., Padalhin, A. R., and Lee, B. T. (2019). Incorporation of chitosan-alginate complex into injectable calcium phosphate cement system as a bone graft material. Mater. Sci. Eng. C 94, 385–392. doi:10.1016/j.msec.2018.09.039
Li, C., Sun, J., Shi, K., Long, J., Li, L., Lai, Y., et al. (2020b). Preparation and evaluation of osteogenic nano-MgO/PMMA bone cement for bone healing in a rat critical size calvarial defect. J. Mat. Chem. B 8 (21), 4575–4586. doi:10.1039/d0tb00074d
Li, W. H., Hao, W., Wu, C., Tao, J., Ai, F., Xin, H., et al. (2020a). Injectable and bioactive bone cement with moderate setting time and temperature using borosilicate bio-glass-incorporated magnesium phosphate. Biomed. Mat. 15 (4), 045015. doi:10.1088/1748-605x/ab633f
Li, G., Shen, W., Tang, X., Mo, G., Yao, L., and Wang, J. (2021). Combined use of calcium phosphate cement, mesenchymal stem cells and platelet-rich plasma for bone regeneration in critical-size defect of the femoral condyle in mini-pigs. Regen. Med. 16 (5), 451–464. doi:10.2217/rme-2020-0099
Li, X., Li, G., Zhang, K., Pei, Z., Zhao, S., and Li, J. (2021). Cu-loaded Brushite bone cements with good antibacterial activity and operability. J. Biomed. Mat. Res. 109 (6), 877–889. doi:10.1002/jbm.b.34752
Li, Y., and Liu, C. (2017). Nanomaterial-based bone regeneration. Nanoscale 9 (15), 4862–4874. doi:10.1039/c7nr00835j
Liang, W., Gao, M., Lou, J., Bai, Y., Zhang, J., Lu, T., et al. (2020). Integrating silicon/zinc dual elements with PLGA microspheres in calcium phosphate cement scaffolds synergistically enhances bone regeneration. J. Mat. Chem. B 8 (15), 3038–3049. doi:10.1039/c9tb02901j
Liaw, C. Y., and Guvendiren, M. (2017). Current and emerging applications of 3D printing in medicine. Biofabrication 9 (2), 024102. doi:10.1088/1758-5090/aa7279
Lin, S. H., Zhang, W. J., and Jiang, X. Q. (2019). Applications of bioactive ions in bone regeneration. Chin. J. Dent. Res. 22 (2), 93–104. doi:10.3290/j.cjdr.a42513
Lin, X., Patil, S., Gao, Y-G., and Qian, A. (2020). The bone extracellular matrix in bone formation and regeneration. Front. Pharmacol. 11, 757. doi:10.3389/fphar.2020.00757
Liu, J., Liao, J., Li, Y., Yang, Z., Ying, Q., Xie, Y., et al. (2019). Bioactive tetracalcium phosphate/magnesium phosphate composite bone cement for bone repair. J. Biomater. Appl. 34 (2), 239–249. doi:10.1177/0885328219845597
Liu, S. M., Chen, W. C., Ko, C. L., Chang, H. T., Chen, Y. S., Haung, S. M., et al. (2021a). In vitro evaluation of calcium phosphate bone cement composite hydrogel beads of cross-linked gelatin-alginate with gentamicin-impregnated porous scaffold. Pharm. (Basel) 14 (10), 1000. doi:10.3390/ph14101000
Liu, X., Camilleri, E. T., Li, L., Gaihre, B., Rezaei, A., Park, S., et al. (2021b). Injectable catalyst-free “click” organic-inorganic nanohybrid (click-ON) cement for minimally invasive in vivo bone repair. Biomaterials 276, 121014. doi:10.1016/j.biomaterials.2021.121014
Lobenhoffer, P., Gerich, T., Witte, F., and Tscherne, H. (2002). Use of an injectable calcium phosphate bone cement in the treatment of tibial plateau fractures: A prospective study of twenty-six cases with twenty-month mean follow-up. J. Orthop. Trauma 16 (3), 143–149. doi:10.1097/00005131-200203000-00001
Ma, C., Tian, X., Kim, J. P., Xie, D., Ao, X., Shan, D., et al. (2018). Citrate-based materials fuel human stem cells by metabonegenic regulation. Proc. Natl. Acad. Sci. U. S. A. 115 (50), E11741–E11750. doi:10.1073/pnas.1813000115
Manton, K. J., Leong, D. F., Cool, S. M., and Nurcombe, V. (2007). Disruption of heparan and chondroitin sulfate signaling enhances mesenchymal stem cell-derived osteogenic differentiation via bone morphogenetic protein signaling pathways. Stem Cells 25 (11), 2845–2854. doi:10.1634/stemcells.2007-0065
McDonald, M. M., Kim, A. S., Mulholland, B. S., and Rauner, M. (2021). New insights into osteoclast biology. JBMR plus 5 (9), e10539. doi:10.1002/jbm4.10539
Mestres, G., and Ginebra, M. P. (2011). Novel magnesium phosphate cements with high early strength and antibacterial properties. Acta Biomater. 7 (4), 1853–1861. doi:10.1016/j.actbio.2010.12.008
Miao, Q., Yang, S., Ding, H., and Liu, J. (2020). Controlled degradation of chitosan-coated strontium-doped calcium sulfate hemihydrate composite cement promotes bone defect repair in osteoporosis rats. Biomed. Mat. 15 (5), 055039. doi:10.1088/1748-605x/ab9fcf
Mizoguchi, T., and Ono, N. (2021). The diverse origin of bone-forming osteoblasts. J. Bone Min. Res. 36 (8), 1432–1447. doi:10.1002/jbmr.4410
Myerson, C. L., Myerson, M. S., Coetzee, J. C., McGaver, R. Stone, and Giveans, M. R. (2019). Subtalar arthrodesis with use of adipose-derived cellular bone matrix compared with autologous bone graft: A multicenter, randomized controlled trial. J. Bone Jt. Surg. 101 (21), 1904–1911. doi:10.2106/jbjs.18.01300
Oh, S. H., Finones, R., Jin, S., Choi, S. Y., and Kim, K. N. (2004). Influence of tricalcium aluminate phase on in vitro biocompatibility and bioactivity of calcium aluminate bone cement. J. Mat. Res. 19 (4), 1062–1067. doi:10.1557/jmr.2004.0139
Okuchi, Y., Reeves, J., Ng, S. S., Doro, D. H., Junyent, S., Liu, K. J., et al. (2021). Wnt-modified materials mediate asymmetric stem cell division to direct human osteogenic tissue formation for bone repair. Nat. Mat. 20 (1), 108–118. doi:10.1038/s41563-020-0786-5
Oliveira, É. R., Nie, L., Podstawczyk, D., Allahbakhsh, A., Ratnayake, J., Brasil, D. L., et al. (2021). Advances in growth factor delivery for bone tissue engineering. Int. J. Mol. Sci. 22 (2), 903. doi:10.3390/ijms22020903
Ostrowski, N., Roy, A., and Kumta, P. N. (2016). Magnesium phosphate cement systems for hard tissue applications: A review. ACS Biomater. Sci. Eng. 2 (7), 1067–1083. doi:10.1021/acsbiomaterials.6b00056
P Baldwin, D. J. Li, Li, D. J., Auston, D. A., Mir, H. S., Yoon, R. S., and Koval, K. J. (2019). Autograft, allograft, and bone graft substitutes: Clinical evidence and indications for use in the setting of orthopaedic trauma surgery. J. Orthop. Trauma 33 (4), 203–213. doi:10.1097/bot.0000000000001420
Palmer, I., Nelson, J., Schatton, W., Dunne, N. J., Buchanan, F., and Clarke, S. A. (2016). Biocompatibility of calcium phosphate bone cement with optimised mechanical properties: An in vivo study. J. Mat. Sci. Mat. Med. 27 (12), 191. doi:10.1007/s10856-016-5806-2
Patel, A., Petrone, B., and Carter, K. R. (2021). “Percutaneous vertebroplasty and kyphoplasty,” in StatPearls (Treasure Island (FL): StatPearls Publishing).
Paz, E., Ballesteros, Y., Abenojar, J., Del Real, J. C., and Dunne, N. J. (2019). Graphene oxide and graphene reinforced PMMA bone cements: Evaluation of thermal properties and biocompatibility. Mater. (Basel) 12 (19), 3146. doi:10.3390/ma12193146
Pei, P., Qi, X., Du, X., Zhu, M., Zhao, S., and Zhu, Y. (2016). Three-dimensional printing of tricalcium silicate/mesoporous bioactive glass cement scaffolds for bone regeneration. J. Mat. Chem. B 4 (46), 7452–7463. doi:10.1039/c6tb02055k
Ponzetti, M., and Rucci, N. (2021). Osteoblast differentiation and signaling: Established concepts and emerging topics. Int. J. Mol. Sci. 22 (13), 6651. doi:10.3390/ijms22136651
Raja, N., Park, H., Choi, Y-J., and Yun, H-s. (2021). Multifunctional calcium-deficient hydroxyl apatite–alginate core–shell-structured bone substitutes as cell and drug delivery vehicles for bone tissue regeneration. ACS Biomater. Sci. Eng. 7 (3), 1123–1133. doi:10.1021/acsbiomaterials.0c01341
Rattanachan, S. T., Srakaew, N. L., Thaitalay, P., Thongsri, O., Dangviriyakul, R., Srisuwan, S., et al. (2020). Development of injectable chitosan/biphasic calcium phosphate bone cement and in vitro and in vivo evaluation. Biomed. Mat. 15 (5), 055038. doi:10.1088/1748-605x/ab8441
Rho, Y. J., Choe, W. J., and Chun, Y. I. (2012). Risk factors predicting the new symptomatic vertebral compression fractures after percutaneous vertebroplasty or kyphoplasty. Eur. Spine J. 21 (5), 905–911. doi:10.1007/s00586-011-2099-5
Rupp, M. N. Walter, Ismat, A., and Alt, V. (2021). Polymethyl methacrylate cement coating of intramedullary implants : A new technique for revision surgery with the example of a temporary knee arthrodesis. Video article. Orthopade 50 (9), 758–762. doi:10.1007/s00132-021-04111-x
Saeed, H., Ahsan, M., Saleem, Z., Iqtedar, M., Islam, M., Danish, Z., et al. (2016). Mesenchymal stem cells (MSCs) as skeletal therapeutics - an update. J. Biomed. Sci. 23, 41. doi:10.1186/s12929-016-0254-3
Saruta, J., Ozawa, R., Hamajima, K., Saita, M., Sato, N., Ishijima, M., et al. (2021). Prolonged post-polymerization biocompatibility of polymethylmethacrylate-tri-n-butylborane (PMMA-TBB) bone cement. Mater. (Basel) 14 (5), 1289. doi:10.3390/ma14051289
Schlundt, C., El Khassawna, T., Serra, A., Dienelt, A., Wendler, S., Schell, H., et al. (2018). Macrophages in bone fracture healing: Their essential role in endochondral ossification. Bone 106, 78–89. doi:10.1016/j.bone.2015.10.019
Schlundt, C., Fischer, H., Bucher, C. H., Rendenbach, C., Duda, G. N., and Schmidt-Bleek, K. (2021). The multifaceted roles of macrophages in bone regeneration: A story of polarization, activation and time. Acta Biomater. 133, 46–57. doi:10.1016/j.actbio.2021.04.052
Schmidt, A. H. (2021). Autologous bone graft: Is it still the gold standard? Injury 52 (2), S18–s22. doi:10.1016/j.injury.2021.01.043
Shang, F., Yu, Y., Liu, S., Ming, L., Zhang, Y., Zhou, Z., et al. (2020). Advancing application of mesenchymal stem cell-based bone tissue regeneration. Bioact. Mater. 6 (3), 666–683. doi:10.1016/j.bioactmat.2020.08.014
Sharma, K., Sharma, S., Thapa, S., Bhagat, M., Kumar, V., and Sharma, V. (2020). Nanohydroxyapatite-Gelatin-and acrylic acid-based novel dental restorative material. ACS Omega 5 (43), 27886–27895. doi:10.1021/acsomega.0c03125
Sharma, R., Kapusetti, G., Bhong, S. Y., Roy, P., Singh, S. K., Singh, S., et al. (2017). Osteoconductive amine-functionalized graphene–poly(methyl methacrylate) bone cement composite with controlled exothermic polymerization. Bioconjug. Chem. 28 (9), 2254–2265. doi:10.1021/acs.bioconjchem.7b00241
Shen, H., Zhi, Y., Zhu, F., Si, J., Shi, J., and Shen, S. G. (2021). Experimental and clinical evaluation of BMP2-CPC graft versus deproteinized bovine bone graft for guided bone regeneration: A pilot study. Dent. Mat. J. 40 (1), 191–201. doi:10.4012/dmj.2019-437
Shi, Y. L. Yu, Yu, L., Gong, C., Li, W., Zhao, Y., and Guo, W. (2021). A bioactive magnesium phosphate cement incorporating chondroitin sulfate for bone regeneration. Biomed. Mat. 16 (3), 035034. doi:10.1088/1748-605x/abf5c4
Siracusa, V., Maimone, G., and Antonelli, V. (2021). State-of-Art of standard and innovative materials used in cranioplasty. Polym. (Basel) 13 (9), 1452. doi:10.3390/polym13091452
Song, Y., Huang, X., and Wu, L. (2020). Removal of intracardiac bone cement embolism after percutaneous kyphoplasty: A case report. Med. Baltim. 99 (11), e19354. doi:10.1097/md.0000000000019354
Subhi, H., Husein, A., Mohamad, D., Nik Abdul Ghani, N. R., and Nurul, A-A. (2021). Chitosan-based accelerated Portland cement promotes dentinogenic/osteogenic differentiation and mineralization activity of SHED. Polymers 13 (19), 3358. doi:10.3390/polym13193358
Talati, R. K., Hallak, J. A., Karas, F. I., de la Cruz, J., and Cortina, M. S. (2018). Retroprosthetic membrane formation in boston keratoprosthesis: A case-control-matched comparison of titanium versus PMMA backplate. Cornea 37 (2), 145–150. doi:10.1097/ico.0000000000001462
Tan, S., Wang, Y., Du, Y., Xiao, Y., and Zhang, S. (2021). Injectable bone cement with magnesium-containing microspheres enhances osteogenesis via anti-inflammatory immunoregulation. Bioact. Mater. 6 (10), 3411–3423. doi:10.1016/j.bioactmat.2021.03.006
Tang, G., Liu, Z., Liu, Y., Yu, J., Wang, X., Tan, Z., et al. (2021). Recent trends in the development of bone regenerative biomaterials. Front. Cell. Dev. Biol. 9, 665813. doi:10.3389/fcell.2021.665813
Tao, Z. S., Zhou, W. S., Zhang, R. T., Li, Y., Xu, H. G., Wei, S., et al. (2021). Co-modification of calcium phosphate cement to achieve rapid bone regeneration in osteoporotic femoral condyle defect with lithium and aspirin. Am. J. Transl. Res. 13 (3), 952–966.
Tavakoli, M., Bakhtiari, S. S. E., and Karbasi, S. (2020). Incorporation of chitosan/graphene oxide nanocomposite in to the PMMA bone cement: Physical, mechanical and biological evaluation. Int. J. Biol. Macromol. 149, 783–793. doi:10.1016/j.ijbiomac.2020.01.300
Tian, Y., Liu, J., et al. (2021). Clinical study of calcium phosphate cement loaded with recombinant human bone morphogenetic protein 2 combined with calcium phosphate cement loaded with antibiotic for chronic osteomyelitis with bone defect. Zhongguo Xiu Fu Chong Jian Wai Ke Za Zhi 35 (5), 573–578.
Tieh, M. T., Waddell, J. N., and Choi, J. J. E. (2021). Optical properties and color stability of denture teeth-A systematic review. J. Prosthodont. 31, 385–398. doi:10.1111/jopr.13429
van Houdt, C. I. A., Gabbai-Armelin, P. R., Lopez-Perez, P. M., Ulrich, D. J. O., Jansen, J. A., Renno, A. C. M., et al. (2018). Alendronate release from calcium phosphate cement for bone regeneration in osteoporotic conditions. Sci. Rep. 8 (1), 15398. doi:10.1038/s41598-018-33692-5
Vertesich, K., Sosa, B. R., Niu, Y, Ji, G, Suhardi, V, Turajane, K, et al. (2021). Alendronate enhances osseointegration in a murine implant model, J. Orthop. Res. 39, 719–726. doi:10.1002/jor.24853
Wang, G., Li, J., Zhang, W., Xu, L., Pan, H., Wen, J., et al. (2014). Magnesium ion implantation on a micro/nanostructured titanium surface promotes its bioactivity and osteogenic differentiation function. Int. J. Nanomedicine 9, 2387–2398. doi:10.2147/ijn.s58357
Wang, S., Xu, C., Yu, S., Wu, X., Jie, Z., and Dai, H. (2019). Citric acid enhances the physical properties, cytocompatibility and osteogenesis of magnesium calcium phosphate cement. J. Mech. Behav. Biomed. Mat. 94, 42–50. doi:10.1016/j.jmbbm.2019.02.026
Wang, X., Peng, X., Yue, P., Qi, H., Liu, J., Li, L., et al. (2020). A novel CPC composite cement reinforced by dopamine coated SCPP fibers with improved physicochemical and biological properties. Mater. Sci. Eng. C 109, 110544. doi:10.1016/j.msec.2019.110544
Wang, Y., Shen, S., Hu, T., Williams, G. R., Bian, Y., Feng, B., et al. (2021). Layered double hydroxide modified bone cement promoting osseointegration via multiple osteogenic signal pathways. ACS Nano 15 (6), 9732–9745. doi:10.1021/acsnano.1c00461
Wenisch, S., Stahl, J. P., Horas, U., Heiss, C., Kilian, O., Trinkaus, K., et al. (2003). In vivo mechanisms of hydroxyapatite ceramic degradation by osteoclasts: Fine structural microscopy. J. Biomed. Mat. Res. 67A (3), 713–718. doi:10.1002/jbm.a.10091
Wu, C., and Chang, J. (2012). Mesoporous bioactive glasses: Structure characteristics, drug/growth factor delivery and bone regeneration application. Interface Focus 2 (3), 292–306. doi:10.1098/rsfs.2011.0121
Wu, C., Zhang, Y., Zhu, Y., Friis, T., and Xiao, Y. (2010). Structure-property relationships of silk-modified mesoporous bioglass scaffolds. Biomaterials 31 (13), 3429–3438. doi:10.1016/j.biomaterials.2010.01.061
Wu, S., Lei, L., Bao, C., Liu, J., Weir, M. D., Ren, K., et al. (2021). An injectable and antibacterial calcium phosphate scaffold inhibiting Staphylococcus aureus and supporting stem cells for bone regeneration. Mater. Sci. Eng. C 120, 111688. doi:10.1016/j.msec.2020.111688
Wu, X., Dai, H., Yu, S., Zhao, Y., Long, Y., Li, W., et al. (2020). Magnesium calcium phosphate cement incorporating citrate for vascularized bone regeneration. ACS Biomater. Sci. Eng. 6 (11), 6299–6308. doi:10.1021/acsbiomaterials.0c00929
Wu, Y., Tang, X., Chen, J., Tang, T., Guo, H., Tang, S., et al. (2015). Improvement of bioactivity, degradability, and cytocompatibility of biocement by addition of mesoporous magnesium silicate into sodium-magnesium phosphate cement. J. Mat. Sci. Mat. Med. 26 (9), 238. doi:10.1007/s10856-015-5579-z
Yang, C., Wang, X., Ma, B., Zhu, H., Huan, Z., Ma, N., et al. (2017). 3D-Printed bioactive Ca(3)SiO(5) bone cement scaffolds with nano surface structure for bone regeneration. ACS Appl. Mat. Interfaces 9 (7), 5757–5767. doi:10.1021/acsami.6b14297
Yang, N., and Liu, Y. (2021). The role of the immune microenvironment in bone regeneration. Int. J. Med. Sci. 18 (16), 3697–3707. doi:10.7150/ijms.61080
Yorukoglu, A. C., Kiter, A. E., Akkaya, S., Satiroglu-Tufan, N. L., and Tufan, A. C. (2017). A concise review on the use of mesenchymal stem cells in cell sheet-based tissue engineering with special emphasis on bone tissue regeneration. Stem cells Int., 2374161–2374213. doi:10.1155/2017/2374161
Zhang, F., Zhou, M., Gu, W., Shen, Z., Ma, X., Lu, F., et al. (2020). Zinc-/copper-substituted dicalcium silicate cement: Advanced biomaterials with enhanced osteogenesis and long-term antibacterial properties. J. Mat. Chem. B 8 (5), 1060–1070. doi:10.1039/c9tb02691f
Zhang, X., Kang, T., Liang, P., Tang, Y., and Quan, C. (2018). Biological activity of an injectable biphasic calcium phosphate/PMMA bone cement for induced osteogensis in rabbit model. Macromol. Biosci. 18 (3), 1700331. doi:10.1002/mabi.201700331
Zhang, Y., Cui, X., Zhao, S., Wang, H., Rahaman, M. N., Liu, Z., et al. (2015). Evaluation of injectable strontium-containing borate bioactive glass cement with enhanced osteogenic capacity in a critical-sized rabbit femoral condyle defect model. ACS Appl. Mat. Interfaces 7 (4), 2393–2403. doi:10.1021/am507008z
Zhu, J., Yang, S., Yang, Y., Yao, T., Liu, G., Fan, S., et al. (2021). Modified poly(methyl methacrylate) bone cement in the treatment of Kümmell disease. Regen. Biomater. 8 (1), rbaa051. doi:10.1093/rb/rbaa051
Zhu, J., Zhang, K., Luo, K., Qiu, Z., Yang, S., Cui, F., et al. Mineralized collagen modified polymethyl methacrylate bone cement for osteoporotic compression vertebral fracture at 1-year follow-up. Spine 1976), 2019. 44(12): p. 827–838. doi:10.1097/brs.0000000000002971
Zhu, T., Ren, H., Li, A., Liu, B., cui, C., Dong, Y., et al. (2017). Novel bioactive glass based injectable bone cement with improved osteoinductivity and its in vivo evaluation. Sci. Rep. 7 (1), 3622. doi:10.1038/s41598-017-03207-9
Zhu, Y., Goh, C., and Shrestha, A. (2021). Biomaterial properties modulating bone regeneration. Macromol. Biosci. 21 (4), e2000365. doi:10.1002/mabi.202000365
Keywords: bone regeneration, PMMA, calcium phosphate cement, osteoblasts, BMP-1
Citation: Xia Y, Wang H, Li Y and Fu C (2022) Engineered bone cement trigger bone defect regeneration. Front. Mater. 9:929618. doi: 10.3389/fmats.2022.929618
Received: 27 April 2022; Accepted: 07 July 2022;
Published: 22 August 2022.
Edited by:
Le Yu, Ohio University, United StatesReviewed by:
Chenghe Qin, Guangdong Second Provincial General Hospital, ChinaZhiwei Fang, Johns Hopkins University, United States
Ganjun Feng, West China Hospital, Sichuan University, China
Copyright © 2022 Xia, Wang, Li and Fu. This is an open-access article distributed under the terms of the Creative Commons Attribution License (CC BY). The use, distribution or reproduction in other forums is permitted, provided the original author(s) and the copyright owner(s) are credited and that the original publication in this journal is cited, in accordance with accepted academic practice. No use, distribution or reproduction is permitted which does not comply with these terms.
*Correspondence: Changfeng Fu, ZnVjZkBqbHUuZWR1LmNu