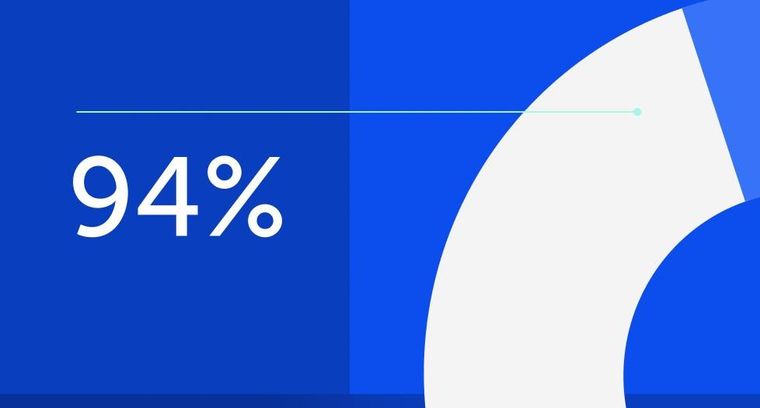
94% of researchers rate our articles as excellent or good
Learn more about the work of our research integrity team to safeguard the quality of each article we publish.
Find out more
REVIEW article
Front. Mater., 07 July 2022
Sec. Polymeric and Composite Materials
Volume 9 - 2022 | https://doi.org/10.3389/fmats.2022.929501
This article is part of the Research TopicRecent Advances in Sustainable Polymer MaterialsView all 5 articles
Due to the inherent hydrophilic nature and porosity of the paper fibers, hydrophobic polymeric materials, waxes, and inorganic fillers have been widely utilized as coatings and fillers, respectively, on a fiber-based substrate. Coatings also impart oxygen, aroma, and oil barrier properties desirable for food packaging applications. In addition, coatings improve the functional properties and characteristics of paper, including reduced water absorbance, enhanced surface finish, gloss finish, printability, readability, dimensional stability of the substrate, and antimicrobial performance. Such functional properties are highly desirable for consumer packaging applications. However, such coatings may limit the repulpability, recyclability, biodegradability, and compostability of paper and paperboard. In addition, the contamination of the substrate by-product also limits the recyclability of the fiber-based substrates, and the paper, paperboard, or corrugated material ends up in landfill sites. This review focuses on bioderived, biodegradable, compostable, and functional organic, inorganic, and hybrid hydrophobic coatings, which promote the circular economy by improving the repulpability or reduces carbon footprints.
Consumer awareness, corporate sustainability push, limited landfill sites, anti-plastic sentiments, and waste management issues have provided fresh impetus to paper and paper board-based materials. Paper is considered the ecologically most sustainable material for packaging applications due to its renewable origin and compostability under industrial composting conditions (Nechita and Roman, 2020; Xiong et al., 2020; Oloyede and Lignou, 2021). The natural abundance and low cost of lignocellulosic materials make them a very attractive choice for packaging applications. Porous nature and hydroxyl groups in the cellulosic material bind water, which reduces their functional performance, including mechanical strength and load-bearing capacity. Therefore, coatings are required for fiber-based substrates. However, coatings may potentially limit the recyclability of fibrous material and hinder the compostability of paper-based substrates. In addition, there are environmental concerns related to omniphobic coating materials, such as polyfluorinated materials (PFAS), which enter the wastewater stream during the repulping process (Kansal et al., 2020; Glenn et al., 2021). Recently, a bipartisan bill has been introduced to ban PFAS in the United States, and at least seven states have adopted a law to ban it in food packaging applications. Therefore, alternative bio-based, bio-degradable, and compostable organic or inorganic hydrophobic coating materials have been widely researched, which can allow either repulpability or promote compostability (Shankar and Rhim, 2018; Hamdani et al., 2020; Kansal et al., 2020; Nechita and Roman, 2020; Xiong et al., 2020; Glenn et al., 2021). Such coatings can promote the circular economy and reduce the landfill burden and environmental contamination.
The final properties of the substrate vary by precoating sizing filler treatment and the type of the final coating and coating thickness/weight. For example, inorganic fillers such as CaCO3 are added to improve the optical properties, whiteness, printing, and glossiness. However, surface adhesion of these fillers requires a thin polymer or adhesive matrix. The type of paper surface treatments, including adhesives, mineral pigments, inks, and coatings, can affect the repulpability and recyclability of fibers. The separation of fibrous and non-fibrous materials is critical for achieving the high circularity of such substrates.
As per US Environmental Protection Agency (US-EPA) estimates, 20.8% of the paper container and packaging, excluding corrugation, were recycled. It is significantly lower than other categories such as newspaper (64.8%) and corrugated material (96.5%) (US-EPA) (EPA, 2022). Environmental awareness and recent bills to ban traditional omniphobic coatings such as fluorinated chemicals in food packaging also provide impetus to bio-derived, bio-degradable, or compostable coatings (Hamdani et al., 2020; Tyagi et al., 2021).
For certain applications, such as fresh produce or seafood packaging and shipment, the hydrophobic coating of corrugated boxes is inevitable as water sorption by the corrugated board during various post-harvest or post-processing treatments such as hydro-cooling, ice-cooling, forced air cooling, may affect the strength, stiffness, mechanical performance, and functionality of the corrugated board (Sun et al., 2010; Tyagi et al., 2021). In addition, high water content present in such products can affect the moisture content in the corrugated board. Paraffin wax coatings are widely prevalent to achieve a hydrophobic corrugated structure. However, wax coatings limit the recyclability of these boxes (Andersson, 2008; Deshwal et al., 2019), and at the end of life cycle energy is recovered from such materials by pyrolysis (Sotoudehnia et al., 2021). It is hard to recycle polyolefin and other synthetic coatings (Cheng et al., 2015; Shanmugam et al., 2019).
This review focuses on bioderived, biodegradable, compostable, and functional hydrophobic coatings, which promote the circular economy by improving the repulpability or reducing the carbon footprints. In the first section, we discuss degradable/compostable polymer coatings covering bioderived polymers, petro-derived polymers, and polymer blends. In the second section, we address functional nanocomposite coatings, which include nanoclay-based, nanocellulose-based, nanocarbon-based, and other nanoparticle-based coating materials. We conclude by exploring new materials for alternative green-coated food packaging boxes.
Compostability and biodegradability are assessed per the local standards derived from a basic framework and norms accepted worldwide. In the US, industrial compost facility conditions are simulated as described in the ASTM D6400 for compostability certification for plastics (Kijchavengkul et al., 2010). However, laminated or extrusion-coated paper products are governed by ASTM D-6868, which requires all layers should to meet the compostability requirements for certification (Kijchavengkul and Auras, 2008). Bio-based content is estimated using ASTM D6866. Table 1 represents a list of compostability certifying institutions worldwide and associated standards followed by these agencies.
Polysaccharides are a broad category of macromolecules composed of 10 or more monosaccharides linked together by a glycosidic bond. Some popular molecules suitable for paper coating with unique functional applications, such as starch, lignocellulose, pectin, chitin, chitosan, and hemicellulose, have been widely studied by researchers. The degree of their hydrophilicity is linked to their molecular architecture, molecular weight, intermolecular interactions, any functional or chemical treatment, and degree of crystallinity (Seidi et al., 2022). Hydroxyl functionalization and cross-linking of polysaccharides can control viscosity and coating thickness, affecting the functional performance of coated substrates (Gatto et al., 2019).
Chitin is a natural, second most abundant, environmentally benign macromolecule made up of N-acetyl-D-glucosamine, existing in three crystalline polymorphic forms popularly known as α-chitin, β-chitin, and γ-chitin. α-Chitin is a rhombic crystalline structure, primarily obtained from crab and shrimp shells. Its most widely available and stronger hydrogen bonding interactions exist between carbonyl and hydroxyl groups because neighboring chitin polymer chains are lined up in antiparallel form, generating a stable, compact structure (Jin et al., 2021). Chitin nanowhiskers (CNW) have been studied by various researchers. Peng and Chen (2018) reported that adding 0.1% CNW in the PVOH matrix improved the strength and increased stiffness and transparency of PVOH CNW-based films. The oxygen transmission rate of the PVOH-0.1% CNW films decreased by 65% compared to the base PVOH films. CNW-starch nanocomposite films have demonstrated antimicrobial properties against Gram-positive bacteria such as Listeria monocytogenes (Qin et al., 2016). Cellulose–positively charged CNW (obtained by partial deacetylation) coating significantly improved the wet strength of the paper (Tang et al., 2020). Chitin can serve as a great reinforcing agent for cellulosic material.
Chitosan, the deacetylated form of chitin, has been reported to increase the wet web strength, dry strength, and mechanical properties. Sun et al. (2010) fabricated chitosan-guanidine complexes by cross-linking chitosan and polyhexamethylene guanidine hydrochloride.
Gatto et al. (2019) applied cardboard with 2 GSM (g.m−2) thick chitosan coating of 2% degree of acetylation. Acetylation decreases hydrophilicity and increases the degree of crystalline phase in chitosan (Pillai et al., 2009), which decreased water sorption by 12% as measured by the cobb test and improved mechanical resistance. The Taber stiffness improved by approximately 5% and 20% in the machine direction and cross direction of the paper board (Gatto et al., 2019). Chitosan-based coatings can impart antimicrobial characteristics; control coagulation; and improve water, oxygen, oil, and grease barrier performance.
Brodnjak and Tihile (2020) studied chitosan, zein, and rosemary essential oil-based coating to impart water and oil resistance characteristics to the paper. Two-layer coating applied using a layer-by-layer coating process, with chitosan as the first layer and zein rosemary oil as the second layer, improved mechanical performance and significant water and grease resistance. Tensile strength improved from 25 to 44 MPa and 58 to 75 MPa in the Machine Direction and Cross Direction, respectively. Water vapor permeability dropped by approximately 60% from 600 to 254 g m−2d−1, and moisture absorptivity, as measured by the cobb test, decreased by ∼ 50% approximately. It was observed that chitosan, zein-rosemary dual-coated paper had less than 5% area grease-stained after 8 h of exposure compared to 75% for uncoated paper. A smooth surface finish was observed in the coated paper as observed by images captured using SEM.
Starch is a hydrophilic high molecular weight biopolymer due to the presence of hydroxyl groups. These hydroxyl groups help in its water suspension for coating formulation and superior binding performance with paper fibers. It is cheap and abundantly available and exists in two polysaccharide forms: amylose, a primarily linear form with α-1,4 linkages, and amylopectin, a branched form joined by α-1,6 linkages. It provides good oil resistance. However, its hydrophilic nature limits its functional performance, such as mechanical strength, stiffness, and creasing as a coating. Compared to other polysaccharides such as cellulose and hemicellulose, the helices chain structure of starch somewhat limits its hydrogen bonding tendency. Chemical modifications such as oxidization, graft copolymerization, and crosslinking are used to fabricate starch-based coating binders with tailored properties or surface sizing agents (Ni et al., 2018). Copolymerization and crosslinking also help obtain the desired structure, hardness, and various other functional characteristics (Li et al., 2019a). Enzyme degraded starch copolymerized or crosslinked offers a replacement to synthetic latex binders. Starch-based bio-latex and its derivatives can exist in various morphologies, represented in Figure 1, which depend on the coating constituents and processing parameters. Core-shell morphology has been widely reported to enhance mechanical performance, including good stiffness-toughness balance.
FIGURE 1. Typical morphology variants of particles composed of two types of polymers. The final morphology of particles synthesized by seeded polymerization using polymer seed (black color denotes polymer (1) swollen with monomer 2 with the formation of polymer 2 (white color) can be shown in (A) (ideal core–shell morphology) or in (B) inverted core-shell structure. Composite particle morphology can be composed of one type of polymer (polymer 2) with embedded domains of polymer 1 (in case of the particle (C) in the whole particle, (D) domains present in the particle shell region), or vice versa. The favorable morphology of particles exhibits the minimum interfacial free energy change. Shell chemical composition may also change gradually, that is, particle’s shell is enriched with a latter component going from a particle’s core to its surface (in case of (E)). Redrawn from Gosecka and Gosecki (2015).
Kansal et al. (2020) utilized a dual-layer strategy to mitigate the water sensitivity of starch coating; the researcher recoated the starch coating with zein coating as the top layer for achieving both water- and oil-resistant coating. Cobb 60 values (water) of coated paper dropped from 29.3 to 6.18 g m−2 compared to uncoated paper. The authors promoted such dual-layer coating to the cost competency of both layers, bio-based origin, and potential ease of recycling the fiber.
Starch has been blended with beeswax to obtain hydrophobic and active packaging and improved barrier coatings. However, such coatings require emulsifiers. Trinh et al. (2022) reported such coating for edible application on fresh produce. Such technologies can commercially be employed as a coating on the printed paper label; one such example is marketed under the trade name StixFresh™.
Hendrawati et al. (2021) studied sago starch foam coated with beeswax. They have reported that chemical treatments such as acid hydrolysis reduce the hydrophilicity of starch. Such chemical modifications in starch may make it expensive. The various beeswax coating compositions with beeswax 0–10% (wt./wt.) were applied with application times ranging 30–150 s. Water sorption decreased with increasing beeswax composition, and application time was ascribed to the increased thickness of the hydrophobic coating. However, coating thickness and application time did not significantly impact its mechanical strength. Biodegradation studies were performed using EN 13432; coatings with 4 wt% beeswax formulation and 150 s application time demonstrated 73%–87% degradation in 28 days.
Poly(lactic acid) (PLA) has progressively generated thrust as a mass bio-polymer across various industries, including packaging and paper coating, due to its biodegradability, composability, and commercial viability (Detyothin et al., 2010; Kathuria et al., 2013). Approximately 400,000 tonnes of PLA was produced in 2020, and it is expected to reach 790,000 tonnes in 2026 (Nova Institute and European Bioplastics, 2022). In the United States, it is commercially available under the brand Ingeo™ by NatureWorks LLC. The chemical structure of PLA is represented in Figure 2; it is a rigid polyester with a glass transition temperature (Tg) ∼ 60°C and melting point (Tm) ∼ 160°C. It is widely used for food contact packaging applications, including beverage cups and fruit boxes (Singh et al., 2020).
Sundar et al. (2020) coated unbleached virgin kraft paper (100 g m−2) with PLA using 5%, 10%, 15%, 20%, and 25% PLA-methylene dichloride solutions. The coating viscosity, weight, and thickness increased linearly with an increase in the PLA concentration in the solution. The coating weight of approximately 2.5–9 GSM was reported for 5%–25% PLA-methylene dichloride. Good adhesion of the coating on the substrate was observed using SEM. Burst strength of uncoated PLA increased from 3.75 to nearly 6.25 kg cm−2 with a 9 GSM coating. Similarly, linear mechanical performance improvement trends were observed in tensile strength and wet tensile strength tests as a function of coating weight. The 30 s cobb values for kraft paper and kraft-9 GSM coating were reported as 25.6 and 3.17 g m−2, respectively. PLA can also undergo hydrolysis during the recycling process.
Singh et al. (2020) studied PLA-30 wt% cellulose bio-composites. The cellulose was chemically modified with poly(ethylene oxide) (PEO) prior to melting the blend in a twin-screw extruder. The PEO improved compatibility, reduced interfacial tension, and enhanced cellulose dispersion and processability. PEO served as a plasticizer. The combination of PLA, cellulose, and PEO provided a good stiffness toughness balance with approximately 140% elongation at break. Such modifications can provide a pathway for industrially scalable coatings. However, during the repulping process of organic molecules, filler and particles may end up in the rejected material stream along with the coating, and the accumulation of molecules and sub-micron particles in the environment may pose environmental challenges that need careful deliberations (Zhang et al., 2016).
Poly(butylene succinate) (PBS) is an aliphatic polyester made by condensation polymerization of succinic acid and 1,4 butanediol. PBS can be completely bio-derived as both succinic acid and 1,4 butanediol (Liminana et al., 2018; Thurber and Curtzwiler, 2020). Its chemical structure is represented in Figure 3. It is a flexible polymer with Tg ∼ −35°C, Tm ∼ 115°C, and elongation at a break of ∼300%. Due to its fast degradation rate, it has been used as an alternative to polyolefin coatings for fiber-based materials (Thurber and Curtzwiler, 2020; Rafiqah et al., 2021).
Bi et al. (2021) reported using PBS and its co-polyesters for coating paper used in the mulch applications fabricated by blade coating. PBS coating helped achieve a tailored balance between strength, biodegradation, and release kinetics. PBS-coated kraft paper also reduced water vapor loss compared to starch-coated mulch paper.
Thurber and Curtzwiler (2020) evaluated microwave heat and grease resistance of recycled kraft paper melt coated with PBS. The coated papers were exposed to the microwave heat treatment in the presence of oil to simulate fatty food and evaluated for oil penetration. The authors observed some whitening and dimpling. It was ascribed to the localized heat generated by microwaves leading to melting and recrystallization of certain areas on the package. Such changes in the coating morphology may affect the customer usage and perception of the product.
Functionalized bioderived hydrophobic lipid coatings have been reported to offer improved water barrier performance, good adherence, smoothness, gloss, and biological activity (Zhang et al., 2014a; Fratini et al., 2016; York et al., 2019; Hutton-Prager et al., 2021; Wang et al., 2021; Chungsiriporn et al., 2022). In particular, beeswax and Carnauba wax can be extracted on an industrial scale (de Freitas et al., 2019; Navarro-Hortal et al., 2019). Beeswax is a mixture of various long-chain fatty acids, alcohols, and esters with a surface energy of ∼16 mJ m−2 and a melting point ranging from 62°C to 64°C (Zhang et al., 2014a). It is an approved food additive by EU (E901) (EC, 1995) and GRAS by US FDA (Ramos-García et al., 2012; Starowicz et al., 2021). It is widely used in the food, pharmaceutical, and cosmetics industries (Rortais et al., 2021). It has been reported to be effective against various gram-positive and gram-negative bacteria such as S. aureus and E. coli (Sawicki et al., 2022).
Chungsiriporn et al. (2022) applied beeswax–chitosan homogenized solution (30% beeswax and 1% chitosan) between 40 and −50°C on palm fibrous pulp with coarse, medium, and fine textures. Coating significantly improved the mechanical performance, credited to improved bonding and densification. Reduced porosity and pore filling by hydrophobic coating reduced water absorbency. However, densification and coating slowed the degradation process. Zhang et al. (2014b) developed various beeswax–carnauba emulsified wax blend compositions and applied them to the copier paper. The water contact angle of paper increased from 110 (control) to 140 o –167o range depending on the beeswax–carnauba wax ratio and annealing temperature.
Buxoo and Jeetah (2020) evaluated beeswax coating for producing compostable cups from pineapple peels, orange peels, and hemp leaves agro-waste for potential minimization of deforestation. Hemp–pineapple peel (40/60) cup coated with 0.7 mm beeswax provided the max burst resistance (0.25 kPa m2/g) and successfully retained water for 30 min without any leakage. Beeswax did not interfere with the compostability of the cups, and cups degraded in 5–6 weeks. For higher peel percentages, crack emerged in the cup.
Carnauba wax found in carnauba palms, native to Brazil, melts around 90°C. It demonstrates elasticity and deformation resistance and has a relatively lower viscosity, which helps facilitate its application process (Lacerda et al., 2011; de Campos et al., 2019). Wang et al. (2017) fabricated hydrophobic filter paper using carnauba wax/hexadecyltrimethoxysilane- (HDTMS-) TiO2 emulsions using alcohol to separate water and diesel. In addition to the wax, the hydrophobic HDTMS-TiO2 particles further reduced the surface energy of the coating. The contact angle of the paper increased from 0 (uncoated) to >140o for coatings above 2.5% wt. of the filter paper.
Animal and plant proteins can be used to tailor the surface characteristics of fiber-based substrates. Such proteins include zein, soy protein, whey protein, and wheat gluten (Coltelli et al., 2015; Sartori et al., 2019). Wheat gluten or its derivatives can be used as a binder for paper and cardboard coatings as they offer good tackiness (Sartori et al., 2019). Rhim et al. (2006) reported that soy protein coating improved the printability of coated paper substrates. Such natural polymeric protein structures can substitute for petro-derived non-biodegradable coatings as they offer good compatibility with polar substrates. However, they have poor water barrier properties due to the hydrophobic nature of these protein structures.
Poly(butylene adipate-co-terephthalate) PBAT is a highly flexible petro-derived biodegradable and compostable polymer sold by BASF, Inc. under the brand name ecoflex®. The chemical structure of PBAT is represented in Figure 4. It is a polyester with Tg∼−30°C, Tm ∼ 120°C. However, certain grades are partly bio-based content, as one of the monomers can be derived from plant oil. It offers relatively better moisture resistance and barrier compared to polysaccharides and other natural bio-macromolecules. Shorey and Mekonnen (2022) fabricated PBAT-coatings, water, and oil absorptivity cobb values measured as per TAPPI T441 dropped by approximately 25% and 33% from 36 to 27 GSM and 150 to 100 GSM, respectively. The PBAT coating improved the dry strength, wet tensile strength, and oxygen barrier properties. Shankar and Rhim (2018) coated kraft paper with PBAT. The base coating reduced surface roughness and improved hydrophobicity and tensile strength. The basis weight and thickness of the paper increased after coating.
Poly(ε-caprolactone) (PCL), a petroleum-derived flexible biodegradable aliphatic polyester with low Tg (−60°C) and Tm (55–70°C) is produced by ring-opening polymerization of ε-caprolactone (ε-CL) (Detyothin et al., 2010). The Chemical structure of PCL is represented in Figure 5. Its relatively higher hydrophobicity and degree of crystallinity (45–60%) slow down its degradation rate (Detyothin et al., 2010).
Lo Faro et al. (2021) fabricated a PCL bioplastic coating solution with 5%, 10%, and 20% polyethylene glycol (PEG) plasticizer by dissolving them in chloroform. They coated the calendared bleached paper with a bioplastic coating and evaluated it for water and oil resistance. The regular homogenous surface coating was achieved as observed with the SEM images. Simple inverse linear correlation was established between WVTR and coating grammage. In other words, WVTR decreased with an increase in the coating grammage from 3,500 (uncoated) to 1,200 g m−2 d−1 for coating of ∼10 GSM. No significant effect of plasticizer was observed on the WVTR due to its low Tg and high segmental mobility of its chains. Improved oil resistance was observed with the coating application.
Various researchers have also explored methods to improve the functional performance of PCL (Koenig and Huang, 1994; Lyu et al., 2019). Lyu et al. (2019) blended PCL with grapefruit extract using a twin-screw extruder to improve the antimicrobial performance of the coating for food packaging applications. Koenig and Huang (1994) crosslinked poly(caprolactone) to obtain hydrophobic coating.
As one polymer may not meet the processing, performance, and functional characteristics, polymer blends are desirable as they are an economically viable way to achieve the desired functional results (Wang et al., 2001; Lai et al., 2005; Siegenthaler et al., 2012; Moustafa et al., 2017). However, most blends are thermodynamically immiscible due to high molecular weight and the low entropy of mixing (Detyothin et al., 2010; Siegenthaler et al., 2012). However, at times, good mechanical properties can be achieved by blending partly miscible polymers at specific pre-determined blending proportions, which can be used as coatings.
Sundar et al. (2020) studied PLA-PCL blends for coating kraft paper. PCL is a flexible polymer that reduces the brittle behavior of PLA. PLA with 10% PCL blends demonstrated better seal strength (76.6 kPa) and improved gas barrier properties. PLA-PBAT blends commercially available under the registered trade name “Ecovio® PS1606” have been commercially used to coat fiber-based materials (Muthuraj et al., 2019).
Shorey et al. studied PBAT, PBAT-lignin, PBAT-esterified lignin blends based coatings. Lignin, a natural biomass macromolecule, constitutes 15%–30% of the biomass. It exhibits thermal degradation and poor compatibility with plastics due to the abundance of -OH functional groups. Esterification of lignin with fatty acids reduces hydrophilicity and interfacial tension between PBAT and lignin. Esterified lignin demonstrated improved dispersion, compatibility, superior wet strength, tensile strength, and water barrier performance (Shorey and Mekonnen, 2022). The contact angle of PBAT coated paper increased from 80o to 130o with the addition of 50% esterified lignin in the coating.
As biodegradable and compostable polymer coatings show great promise for food packaging with the circular economy, the pure polymers typically face demerits of mechanical weakness, high gas/vapor permeability, and low antimicrobial effects (Johansson et al., 2012; Calva-Estrada et al., 2019; Mustățea et al., 2019; Mellinas et al., 2020; Menzel, 2020; Bilal et al., 2021; Díaz‐montes and Castro‐muñoz, 2021; Tyagi et al., 2021). Adding nanoparticles to the polymer matrices paves the way for enhancing coating functionalities, including mechanical, barrier, antioxidant, and antimicrobial properties because of the high specific surface area and unique functionalities of nanoparticles (Bandyopadhyay and Ray, 2019; Kassem et al., 2019; Silva et al., 2020; Singha and Hedenqvist, 2020; Qu and Luo, 2021). As a result, food quality, nutrient, safety, and bioactive compound delivery are increased. The application of nanomaterials in the food packaging films and coatings is an emerging research field; however, various nanomaterials have been investigated, such as two-dimensional (2D) nanoclay and graphene, one-dimensional (1D) nanocellulose and nanotubes, and zero-dimensional (0D) nanodots (Li et al., 2018; Cui et al., 2020a; Barra et al., 2020; Ahankari et al., 2021; Nath et al., 2022). The resultant functional nanocomposite coatings exhibit enhanced functional properties demonstrating great potential for food packaging applications.
Nanoclays are natural or synthetic 2D nanoscale minerals consisting of a certain amount of aluminum, silica, iron, and magnesium (Bandyopadhyay and Ray, 2019; Bumbudsanpharoke and Ko, 2019; Nath et al., 2022). Nanoclays exist as the layered nanoplatelets showing a well-structured lamellar arrangement. Each layer has a thickness of ∼1 nm and a lateral size from hundreds of nanometers to a few micrometers. Nanoclays have been explored in the development of food packaging materials due to their high aspect ratio, high mechanical strength, and super barrier properties (Nath et al., 2022). One of the most common nanoclays in food packaging films and coatings is montmorillonite (MMT) (Souza et al., 2018). Nanoclays contain charged oxygen and hydroxyl functional groups on the surface and exist as platelet clusters due to hydrogen bonding and ionic interactions between nanoplatelets. In order to increase the surface area exposed to the polymer binder, nanoclays must be intercalated or exfoliated in the polymer matrix (Bumbudsanpharoke and Ko, 2019). In general, there are three types of polymer-clay structures (Figure 6A): tactoid, intercalation, and exfoliation. The tactoid structure is not a true nanocomposite structure as the platelets of nanoclays are not expanded, showing a poor affinity with the polymer matrix. The intercalation structure demonstrates a moderate expansion of interlayers between nanoplatelets, and the polymer chains penetrate the basal spacing of the nanoclay. However, the nature of the layered stack in nanoclays remains unchanged, resulting in a moderate affinity between the polymer and nanoclays. The exfoliation structure is a true nanocomposite structure in which the separated single nanoplatelets are homogeneously distributed throughout the continuous polymer matrix leading to maximum enthalpic interactions between the polymer and nanoclays. The intercalated or exfoliated structures provide a tortuous path to hinder the molecular diffusion of the permeants and therefore improve the barrier properties (Figure 6B) (Singha and Hedenqvist, 2020; Qu and Luo, 2021; Nath et al., 2022).
FIGURE 6. Schematic illustrations of (A) three possible microstructures in nanoclay-polymer nanocomposites and (B) permeability mechanism of molecules through nanoclay-polymer coatings on the substrate.
The addition of intercalated or exfoliated nanoclays into the biopolymer matrix can improve the mechanical, barrier, and antimicrobial properties of food packaging films and coatings. Mahmoodi et al. (2019) studied nanoclay-PLA composite films for food packaging applications. Nanoclays were surface-modified by an organic dye to increase the miscibility with the PLA matrix. Imaging analysis revealed partially intercalated/exfoliated structures of the composites (Figure 7A). The composites containing 3 wt% nanoclays exhibited a 20% increase in storage modulus and 12
FIGURE 7. (A) HRTEM micrograph of the nanoclay-PLA composite film. Red and black arrows demonstrate the intercalated and exfoliated nanoclay platelets, respectively. (B) Storage modulus versus temperature and (C) oxygen permeability (OP) and water vapor permeability (WVP) of PLA composite films with different nanoclay contents. Reprinted with permission from Mahmoodi et al. (2019). Copyright 2019 American Chemistry Society. (D) Antimicrobial mechanism of AgNP-doped nanoclay-chitosan composite films. Redrawn from Li et al. (2020). (E) Antimicrobial activity of AgNP-doped nanoclay-chitosan composite films against four food pollution microorganisms. Redrawn from Wu et al. (2018).
The addition of metal or metal oxide nanoparticles to the nanoclay-polymer nanocomposites can generate a synergistic effect on the mechanical and barrier reinforcement and antimicrobial properties (Uysal Unalan et al., 2014; Vaezi et al., 2019; Ahari et al., 2021; Paidari and Ibrahim, 2021). In a study of MMT-chitosan composite coatings (Rehan et al., 2018), the silver nanoparticles (AgNPs) were synthesized using UV radiation in the MMT-chitosan nanocomposites. In addition to enhanced tensile strength and thermal resistance, AgNPs immobilized nanocomposite coatings exhibited excellent antibacterial activity against S. aureus, E. coli, and A. niger. It was suggested that chitosan efficiently interacted with silver through the amino and hydroxyl groups in chitosan to form chitosan-Ag chelate that contained AgNPs (Figure 7D). When contacted with the bacteria, chitosan-Ag chelate was released from the composite and subsequently destroyed the cell wall and entered the cell. Simultaneously, Ag+ ions were released from AgNPs, resulting in the production of reactive oxygen species (ROS) that killed the cell (Figure 7D). In another work of MMT-starch composite films incorporated with AgNPs/CuO/ZnO (Peighambardoust et al., 2019), the combinatorial use of AgNP/CuO/ZnO in MMT-starch nanocomposite films exhibited a strong synergistic effect in improving mechanical and antimicrobial properties. The nanocomposites had strong antibacterial activity against S. aureus and E. coli.
Nanoclay-polymer nanocomposite coatings with high moisture and gas barrier properties can significantly inhibit bacterial growth and oxidative stress leading to the extended shelf life in the real food system. In a study on sliced fatty salami packaged with MMT-PLA nanocomposite films (Vilarinho et al., 2018), the lipid oxidation was monitored during different storage times to evaluate the shelf life. Both primary and secondary lipid oxidization products were analyzed. The presence of MMT tended to decrease the lipid oxidation and extend the shelf life, which was attributed to the enhanced oxygen and water barrier properties in the composite film. In another work of litchis wrapped with AgNPs immobilized nanoclay-chitosan nanocomposite films (Nath et al., 2022), the nanocomposite films exhibited antimicrobial activity against four typical fruit pollution microorganisms such as S. aureus, E. coli, A. niger, and P. citrinum (Figure 7E). As a result, litchis maintained freshness for up to 7 days, demonstrating the extended shelf life. The extended shelf life was also reported in the study of fish (bluefin tuna) wrapped with MMT-protein nanocomposite films (Echeverría et al., 2018). Seven typical fish pollution microorganisms were analyzed, and the microorganism counts in fish wrapped with nanocomposite films remained one or two magnitudes lower than the control. Fish maintained freshness for up to 15 days, which exceeded in 1 week compared to the control.
Nanocellulose is a nanoscale particle derived from cellulose. It is used as a reinforcing nanofiller in polymer nanocomposite coatings because of the large specific surface area, high tensile strength and modulus, low density, and biodegradability (Silva et al., 2020; Ahankari et al., 2021). There are three main forms of nanocellulose according to dimensions, preparation methods, and functions (Figure 8): cellulose nanocrystals (CNCs), cellulose nanofibrils (CNFs), and bacterial nanocellulose (BNC). CNCs have a rod-like or whisker-like shape with typical dimensions of 2–10 nm in diameter and 50–500 nm in length, depending on the source and chemistry treatments (Phanthong et al., 2018). They are typically extracted from natural micro-fibrillated cellulose via chemical pre-treatment followed by acid hydrolysis. After acid hydrolysis, the amorphous regions are hydrolyzed and removed, whereas the crystalline regions are retained. The resultant CNCs contain a high degree of crystallinity (∼80%), showing a short-rod shape. CNFs are long and flexible fibril-like nanocellulose with a diameter of 3–15 nm and a length of 1–10
FIGURE 8. (A) Schematic illustration of the hierarchical microstructure of cellulose in plant-based biomass. Adapted from Ghasemlou et al. (2021). (B) Schematic illustration of the synthesis of cellulose nanocrystals (CNCs) and cellulose nanofibrils (CNFs) from cellulose microfibrils vial acid hydrolysis and mechanical disintegration, respectively. (C) Schematic illustration of biosynthesis of bacterial nanocellulose (BNC) and SEM micrograph adapted from Ifuku et al. (2007).
Nanocellulose-based coatings have been widely used in food packaging because of their unique properties, including biodegradability, transparency, barrier, antimicrobial and mechanical properties (Nazrin et al., 2020; Silva et al., 2020; Ahankari et al., 2021; Clarkson et al., 2021). Food packaging applications require a good barrier against grease, miner oils, oxygen, and water vapor. Koppolu et al. (2019) reported multilayer coatings of CNC-PLA composites on a paperboard exhibiting excellent barrier properties. Slot-die coating of CNCs followed by extrusion coating of PLA was used in tandem to alternately coat CNCs and PLA onto a paperboard in a continuous process. The resultant multilayer coatings exhibited a significant reduction in oxygen transmission, water vapor permeability, and grease penetration compared to the control. In another work of CNF-protein nanocomposite films (Yu et al., 2019), the composite containing 15 wt% CNFs exhibited an increased tensile strength of protein by 300%, showing a great mechanical reinforcing effect. The incorporation of pine needle extract (PNE) into the nanocomposite film led to strong antioxidant activity against ABTS free radicals and significantly improved antimicrobial activity against foodborne pathogens, including E. coli, S. aureus, S. typhimurium, and L. monocytogenes. As all nanocellulose forms (CNCs, CNFs, and BNCs) act as reinforcing nanofillers to improve the mechanical properties and decrease the water vapor permeability of the polymer matrix, they have different reinforcement efficiency due to their different morphology and functions (Silva et al., 2020). It has been reported that BNC-PLA nanocomposites exhibited stronger mechanical performance in terms of tensile strength and modulus and lower water vapor permeability compared to CNF-PLA nanocomposites (Gitari et al., 2019). While CNFs and BNCs decreased the oxygen permeability of PHB films, CNCs substantially reduced it (Silva et al., 2020).
In order to improve the compatibility between hydrophilic nanocellulose and hydrophobic biopolymers, various chemical grafting methods are used to surface-modify nanocellulose (Figure 9) (Dufresne, 2013; Sharma et al., 2019; Ghasemlou et al., 2021). One of the most effective approaches is silane coupling (Frank et al., 2018). Methoxy and ethoxy silanes are highly reactive to the hydroxyl groups of nanocellulose; thus, hydrophobic hydrocarbon chains are covalently attached to the nanocellulose surface by the silane coupling process. Silylated nanocellulose exhibits high solubility in organic solvents and high compatibility with polymer matrices. Raquez et al. (2012) reported that CNCs were modified in the presence of trialkoxysilanes bearing a methacryloxy moiety. The surface-modified CNCs were incorporated into the PLA matrix by melt extrusion. The resultant CNC-PLA nanocomposites showed high melting temperature and high storage modulus. The enhancement of thermal and mechanical properties was attributed to the effective reinforcement of surface-modified CNCs as nanofillers. Acetylation is a widely studied esterification reaction to introduce hydrophobic hydrocarbon groups onto nanocellulose surfaces (Ghasemlou et al., 2021). The reaction mechanism is to substitute the hydroxyl groups of nanocellulose with acetyl moieties. Acetylated nanocellulose nanofillers have strong interactions with hydrophobic polymer matrix that result in effective mechanical reinforcement in the nanocellulose-polymer nanocomposites. In a study of CNF-PLA nanocomposite films (Ying et al., 2018), acetylated CNFs were mixed with PLA via solution mixing to produce CNF-PLA nanocomposites. The addition of 10 wt% CNF substantially increased the glass transition temperature of PLA and its mechanical performance in terms of tensile strength and elastic modulus. It was suggested that the high compatibility and strong interactions between surface-modified CNFs and PLA led to thermal and mechanical reinforcements. Ring-opening polymerization (ROP) is a very useful technique for attaching long polymer chains to nanocellulose surfaces (Ghasemlou et al., 2021). ROP is widely used to synthesize polymers and biopolymers such as nylon 6, poly(ethylene oxide), PLA, and PCL (Nuyken and Pask, 2013). Typically, ROP in the presence of nanocellulose occurs without an initiator as the hydroxyl groups of nanocellulose can act as an initiating species of polymerization. Wu et al. (2016) investigated CNC-PLA composites using PLA grafted CNCs and their reinforcement effects. The PLA grafted CNCs were synthesized via surface-initiated ROP of lactide in the presence of tin(II) ethyl hexanoate as a catalyst. Surface-modified CNCs were highly soluble in chloroform and incorporated into the PLA matrix to produce CNC-PLA nanocomposites via solution mixing. The addition of a small amount of PLA grafted CNCs significantly accelerated the crystallization of PLA because of the heterogeneous nucleation of CNCs and excellent interactions between modified CNCs and PLA matrix. To this end, the CNC-PLA composites greatly improved storage modulus and heat distortion resistance. In addition, other common chemical grafting methods to surface functionalize nanocellulose include urethanization via isocyanate-hydroxyl reaction, etherification via epoxide-hydroxyl reaction, urethanization via isocyanate-hydroxyl reaction, and surface-initiated radical polymerization (Ghasemlou et al., 2021).
FIGURE 9. Various chemical grafting methods for surface modification of cellulose nanoparticles (CNs). Adapted from Dufresne (2013)
Nanocellulose-polymer composite films/coatings display high antimicrobial activity against a wide range of bacteria and fungi to prevent food deterioration, resulting in extended shelf life in the real food system (Arora and Padua, 2010; Nazrin et al., 2020; Ahankari et al., 2021). Costa et al. (2021) reported CNC/chitosan nanocomposite films as active packaging membranes for chicken meat packages to prolong the shelf life. The addition of 25 wt% CNCs to the polymer matrix resulted in an 80% reduction in oxygen permeability. The significant increase in oxygen barrier properties was attributed to the increased crystallinity and the reduced free volume of the polymer when CNCs were incorporated into chitosan. The CNC-chitosan composite films showed high antimicrobial activity against bacteria S. aureus and E. coli and fungi C. albicans. The addition of only 10 wt% CNCs provided a complete antimicrobial character to the nanocomposite films. The nanocomposite films were used as active packing membranes to reduce the microbial growth in the packed chicken breast under refrigeration conditions. The nanocomposite films reduced the growth of Pseudomonas and Enterobacteriaceae bacteria in chicken meat and decreased the total volatile basic nitrogen, demonstrating great efficiency in retarding meat’s spoilage and prolonging shelf life. In another study, CNC-PLA nanocomposites containing green tea extract were prepared via direct melt processing for preserving fatty food (Vilarinho et al., 2021). The salami was packaged in a vacuum with nanocomposite films and stored under refrigeration conditions. The composite films containing 2 wt% CNCs exhibited a 27% reduction of oxygen permeability, whereas further addition of 1 wt% green team extract showed an oxygen permeability reduction of 60%. This observation indicated the synergistic effect of the presence of both CNC and green team extract. The improved barrier properties of nanocomposite films were also confirmed by reduced water vapor permeability. The barrier effect was attributed to the high crystallinity of the polymer and high surface hydrophobicity. The lipid oxidation of the salami was evaluated using malondialdehyde and p-anisidine. The CNC-PLA composite films containing green tea extract showed inhibition of lipid oxidation of salami, whereas the composite films without green extract exhibited reduced oxidization. This was attributed to the improved oxygen and water barrier properties. Recently, BNC-gelatin nanocomposites containing chitosan nanofibers were prepared as probiotic films to preserve chicken fillets (Salimiraad et al., 2022). The growth of gram-positive and gram-negative bacteria in chicken fillets was evaluated during refrigerated storage. BNC-based nanocomposite films reduced oxidative and microbial spoilage indices compared to the control. Thus, the probiotic composite films improved the quality and shelf life of chicken fillets.
Nanocarbons are nanomaterials consisting of only carbon atoms bonded with each other to form various allotropes (Figure 10A), such as 0D carbon dots, 1D carbon nanotubes, and 2D graphene and graphene oxide (Liu et al., 2018; Anand et al., 2019; Xin et al., 2019; Cui et al., 2020b; Raul et al., 2022). Most carbon nanomaterials are synthesized via a green process generally used in food science and technology (Raul et al., 2022). Nanocarbons act as antibacterial agents and bio-fillers in the applications of active food packaging materials (Azizi-Lalabadi et al., 2020). The antimicrobial mechanism of nanocarbons highly depends on carbon nanostructures, surface functionalities, and target microorganisms. As shown in Figure 10B, nanocarbons’ contact with the cell wall and the interaction of nanocarbons with the bacteria lead to electron evacuation from the microbial surface. The sharp edge of nanocarbons enables disrupting the membrane and entering the cell. In addition, the formation of toxic ROS will damage DNA and proteins. As a result, microorganisms are killed.
FIGURE 10. (A) Carbon nanostructures: carbon dot (CD), single-walled nanotube (SWNT), multiwalled nanotubes (MWNT), graphene, and graphene oxide (GO). (B) Schematic illustration of antimicrobial activity of nanocarbons.
Carbon dots (CDs) are a new type of 0D spherical carbon nanoparticles with a size below 20 nm (Figure 10A). They have a carbon center functionalized with various chemical groups such as hydroxyl, carbonyl, and amine groups (Liu et al., 2020). CDs have exceptional properties of high chemical stability, tunable photoluminescence, low toxicity, biocompatibility, antimicrobial properties, and low-cost sources. They are used for wide applications in the fields of food packaging, biomedicine, optoelectronic devices, and catalysis. Carbon nanotubes (CNTs) are 1D tube-shaped carbon nanomaterials containing sp2 hybridized carbon atoms, classified as single-walled nanotubes (SWNTs) and multiwalled nanotubes (MWNTs) (Figure 10A) according to the number of graphene layers imaginarily rolled up to form CNTs (Anzar et al., 2020). CNTs, in general, have a very high aspect ratio >1,000 and exhibit excellent mechanical properties, high electrical and thermal conductivities, and great antibacterial properties. CNTs are used as reinforcing nanofillers (Abdou et al., 2016) in the nanocomposites for various applications such as structural materials, active packing materials, optoelectrical devices, and biomedical materials. Graphene is a 2D monatomic layer of sp2 hybridized carbon atoms in a honeycomb lattice (Figure 10A). Graphene and its derivatives, including graphene oxide (GO) and reduced graphene oxide (rGO), possess a unique combination of exceptional mechanical properties, intriguing electronic properties, great antibacterial properties, and high thermal conductivities (Barra et al., 2020; Lin et al., 2021; Lin et al., 2022). Similar to CNTs, graphene and its derivatives are used as reinforcing nanofillers and antimicrobial agents for active food packaging, structural nanocomposites, biomedicine, and electronics (Anand et al., 2019; Barra et al., 2020).
Over the years, the reinforcing efficiency and antibacterial activity of nanocarbons have been investigated for active packaging applications (Cui et al., 2020b). Li et al. (2018) studied biodegradable carbon dots synthesized from vitamin C by the one-step electrochemical process. As-prepared CDs displayed a broad spectrum of antibacterial and antifungal activity at low concentrations, inhibiting the growth of E. coli, B. subtilis, B. sp. WL-6, S. aureus, R. solani, and P. grisea. CDs entered the bacteria by diffusion and destroyed the bacterial wall (Figure 10B). Subsequently, CDs bound to the DNA/RNA of microbials and inhibited the important gene expressions to kill the microbials. N-doped CDs and AgNP-immobilized CDs also exhibited strong antimicrobial activity against E. coli and S. aureus (Raina et al., 2020; Saravanan et al., 2020).
The incorporation of CDs into biopolymer matrices increases the mechanical performance, barrier properties, and antimicrobial activity of food packaging films. In a study of CD-gelatin/pectin nanocomposite films (Ezati et al., 2022), the mechanical properties of the composite films in terms of tensile strength and modulus were slightly increased with 2 wt% CD content. The water vapor permeability of the composite films was reduced by 30%. The increased water vapor barrier was ascribed to the tortuous patch of water vapor diffusion in the presence of CDs. The composite films also displayed high antioxidant activity against ABTS (96%) and DPPH (80%) free radicals and strong antibacterial activity against food-borne pathogenic bacteria, L. monocytogenes and E. coli (Figure 11A). Riahi et al. (2022) reported modified CD-cellulose composite coatings for active food packaging applications. CDs were prepared by the hydrothermal synthesis in the chitosan solution, producing chitosan-modified CDs with negligible cytotoxicity. The addition of 5 wt% CDs increased the tensile strength by 30% and elastic modulus by 60%. The composite films displayed strong antioxidant activity against ABTS (100%) and DPPH (88%) free radical and high antimicrobial activity against foodborne bacteria, E. coli and L. monocytogenes and mold A. niger and P. chrysogenum. The lemon coated with composite films maintained the original taste and color after 21 storage days, whereas in the control, spoilage occurred after 7 storage days and mold grew on the lemon surface after 21 storage days.
FIGURE 11. (A) Antioxidant activity of CD-cellulose composite films using ABTS and DPPH methods. Redrawn from Riahi et al. (2022). (B) Tensile strength and Young’s modulus of PHBV composite films incorporated with different PHBV-g-MWNT contents. (C) Overall migration of isooctane and ethanol (10%) in the PHBV composite films incorporated with different PHBV-g-MWNT contents. Redrawn from Yu et al. (2014). (D) AFM amplitude image of E. coli cells after incubation with GO nanosheets. The scale bar is 1
CNTs and functionalized CNTs are used as antimicrobial agents to inhibit the fungal and bacterial growth in active packaging applications (Liu et al., 2018). Because of the large specific surface areas, both SWNTs and MWNTs enhance bacterial inactivation efficiency when they have direct contact with bacterial cell membranes (Figure 11B). The antimicrobial efficiency is highly dependent on the size of CNTs. In general, SWNTs display more antibacterial activity than MWNTs against the microbes. Metal or metal oxide immobilized CNTs, in particular, exhibit an improved bacterial inactivation efficiency. Nanoparticles such as Ag, ZnO, Fe3O4 TiO2, and CuO have been used to dope CNTs for antimicrobial enhancement due to the synergistic effect between CNTs and nanoparticles (Xia et al., 2018; Alias and Abd–Alsada, 2021; A. Sukkar et al., 2019; Sapkota et al., 2020).
The introduction of CNTs into biopolymer films, in general, improves mechanical reinforcing efficiency, barrier properties, and antimicrobial activity. In a study of MWNT-PHBV nanocomposites for food packaging (Yu et al., 2014), MWNTs were surface-functionalized with PHBV by a graft-to-chemical method, and the PHBV-g-MWNTs were then mixed with PHBV to produce nanocomposites. PHBV-g-MWNTs acted as a heterogeneous nucleation agent to promote PHB crystallization. Nanocomposites with 7 wt% PHBV-g-MWNT content exhibited an 88% increase in tensile strength and a 172% increase in elastic modulus compared to the control (Figure 11B). This improvement in mechanical reinforcing efficiency was attributed to effective load transfer from the polymer matrix to nanofillers (Zhang et al., 2008). The water uptake and water vapor permeability of the composite films were reduced by 67% and 33%, respectively, compared to the control. Such improved barrier properties were ascribed to the increased crystallinity of PHBV and tortuous path. In addition, the migration levels of simulants in the composite films were reduced below the legislative migration limits for food packaging materials (Figure 11C). Cui et al. (2020a) studied antimicrobial films of MWNT-PLA nanocomposites with the incorporation of cinnamaldehyde. With 1 wt% MWNTs, the composite exhibited significantly enhanced UV resistance. CNTs acted as a carrier system to prolong the release of volatile cinnamaldehyde. The resultant composite films showed strong antibacterial activity against E. coli and S. aureus. Similar observations of antimicrobial properties were reported in CNT-cellulose composites (Jatoi et al., 2020), CNT-chitosan composites (Kassem et al., 2019), and CNT-gelatin composites (Pattanshetti et al., 2020).
Graphene and its derivatives (GO and rGO) show potent antimicrobials as they affect the cell membrane and cell wall of microorganisms through physical demolition and chemical oxidation (Anand et al., 2019). Typically, three steps are involved in the antibacterial activity of graphene-based nanoparticles (Figure 10B) (Anand et al., 2019): 1) nanosheet deposition on the cell surface, 2) membrane disruption by the sharp edge of nanosheets, and 3) ROS formation. In general, GO has stronger antimicrobial activity than graphene and rGO against microbials because oxygen-containing functional groups on GO have stronger interactions with the cell to induce cell membrane stress. This physical membrane stress disturbs the cell integrity for bacterial inactivation. On the contrary, the formation of ROS results in oxidative stress, leading to the fragmentation of genomic DNA and cell death. The level of antimicrobial activity is determined by the synergistic effect of physical membrane stress and chemical oxidative stress (Anand et al., 2019). Liu et al. (2012) reported the intrinsic antibacterial activity of GO. Bacterial inactivation occurred in the first hour of incubation, and the cell death rate increased with GO concentration and the lateral size. It was suggested that the large GO nanosheets more easily covered the cells to block the active sites on membranes leading to cell death (Figure 11D). When metal or metal oxide nanoparticles are incorporated into GOs, doped GOs display enhanced antimicrobial activity due to the synergistic effect. Truong et al. (2020) reported the synergistic antibacterial activity of AgNP immobilized GOs. AgNP@GOs had a better bactericidal effect on E. coli (73%) and S. aureus (98.5%) than GO or AgNP. GO nanosheets folded entire bacteria while AgNPs penetrated the inner cell to kill the cell. Whitehead et al. (2017) studied the comparison of antibacterial effect between AgNP-doped GO and ZnO-doped GO. AgNP-doped GO was more effective than ZnO-doped GO in terms of antibacterial activity against E. coli, E. faecium, S. aureus, and K pneumoniae.
Graphene and its derivatives are widely used as reinforcing nanofillers and antibacterial agents in the biopolymer nanocomposites for active packaging applications. Arfat et al. reported GO-PLA nanocomposite films incorporated with clove essential oil via solution casting for food packaging applications (Arfat et al., 2018). The addition of 1 wt% GO led to a 40% reduction of oxygen permeability of the film, indicating a strong barrier effect of GO due to a tortuous path for permeation. The composite film exhibited strong inhibition against S. aureus and E. coli. Similar antimicrobial effects were also reported in electrospun mats of PLA incorporated with catechol-grafted GO (Zhang et al., 2018). Gouvêa et al. (2018) studied ZnO-doped rGO-PHBV nanocomposites for active food packaging. ZnO-doped rGO hybrids were synthesized by reducing GO and zinc acetate in a single reaction step. The incorporation of ZnO-doped rGO into the PHBV matrix enhanced the mechanical performance, and the composite films exhibited strong antibacterial activity against E. coli upon direct contact between bacterial cells and composite film. No GO nanosheets migrated to food simulants, whereas the amount of migrated ZnO was below the recommended level. Li et al. (2019b) studied PHBV composite films incorporated with CNC-GO hybrids for high-performance food packaging. Compared to neat PHBV, the tensile strength and Young’s modulus of the composites containing 1 wt% CNC-GO hybrids increased by 170 and 140%, respectively. The strong synergetic effect was attributed to strong interfacial interactions between the nanofiller and polymer matrix. The water absorption and water vapor permeability values of the 1 wt% hybrid-composite films decreased by 70% and 73%, respectively (Figure 11E). The increased barrier properties were ascribed to improved tortuosity of the permeant path and the enhanced interfacial interactions between the CNC-GO nanofiller and PHBV matrix. Compared to the control, the overall migration levels in isooctane and ethanol (10%) of the composite films were reduced by 62% and 50%, respectively (Figure 11F). In addition, the composite films exhibited very strong antimicrobial activity against E. coli and S. aureus. The authors also studied the composite films containing a single component nanofiller (CNC or GO) and found that hybrid reinforced composites displayed better properties than CNC or GO reinforced composites in terms of mechanical, thermal, and barrier properties as well as antibacterial activity, indicating a strong synergistic effect of CNC-GO hybrids.
Besides nanoclays, nanocelluloses, and nanocarbons described above, many other nanoparticles are also incorporated into biopolymers to improve mechanical and barrier properties and antimicrobial activity for food packaging applications (Shatrohan Lal, 2014; Uysal Unalan et al., 2014; Arias et al., 2018; Ahari et al., 2021; Paidari and Ibrahim, 2021). Typically, the nanoparticles are classified into inorganic and organic nanoparticles that possess various shapes, including spheres, rods, discs, and sheets. The nanoparticles and their derivatives doped with other chemicals exhibit the synergistic effect on the protection of food against microbial spoilage once they are incorporated into the polymer matrix.
Metal and metal oxide nanoparticles, such as Ag, Au, Cu, Zn, ZnO, CuO, MgO, and TiO2, are widely used as nanobiocides to inhibit microbial growth (Uysal Unalan et al., 2014; Arias et al., 2018; Lallo da Silva et al., 2019). Nanoparticles can also act as nanobiosensors in food packaging to detect foodborne pathogens or identify environmental factors of food storage conditions. AgNPs possess excellent antimicrobial activity against a broad spectrum of bacteria and fungi (Ahari et al., 2021). AgNPs are readily incorporated into various biopolymers such as gelatin, cellulose, alginate, starch, and chitosan. The resultant AgNP-based nanocomposites exhibit high antibacterial activity against gram-positive and gram-negative bacteria (Kraśniewska et al., 2020).
In a study of AgNP-chitosan nanocomposites (Rehan et al., 2018), AgNP was in situ synthesized by reducing silver nitrate in the chitosan solution. The resultant composite film showed that AgNPs were homogeneously dispersed in the polymer matrix. As expected, the AgNP-chitosan composites displayed high antimicrobial activity against S. aureus, E. coli, and C. albicans. It was suggested that AgNPs had a rapid interaction with the pathogen cells to induce the disruption of the cell membrane resulting in cell death. Importantly, the composite films exhibited reduced or totally excluded cytotoxicity on mammalian somatic and tumoral cells. The incorporation of nanoclays into AgNP-chitosan composites further increased antimicrobial properties due to the synergistic effect. Cao et al. (2020) studied biopolymer films incorporated by TiO2-doped AgNPs for food packaging applications. TiO2-doped AgNPs acted as obstacles to significantly reduce water vapor permeability and oxygen transmission rate of the composite film, indicating increased barrier properties. The composite film containing 5 wt% TiO2-doped AgNPs showed unique synergistic antimicrobial activity against S. aureus and E. coli. The composite film was used to preserve cherry tomatoes for prolonged shelf life. The improvement in food preservation was ascribed to unique antibacterial effects.
ZnO NPs are one of the most popular metal oxides to control microbial growth (Lallo da Silva et al., 2019). ZnO is a semiconductor with a wide bandgap of 3.37 eV and a high exciton binding energy of 60 meV (Zhang et al., 2011). As an antimicrobial agent, ZnO NPs have a small particle size and high specific surface area leading to improvement in surface reactivity. ZnO NPs act in a non-specific manner to fight against microbial growth. The production of ROS by ZnO NPs is one of the most popular mechanisms responsible for antimicrobial activity (Lallo da Silva et al., 2019). ZnO has a conduction band and a valence band. During the photoreaction, free electrons in the conduction band react with dissolved oxygen molecules generating ROS that can penetrate the pathogen cell to kill microorganisms. Under acidic conditions, ZnO NPs will release Zn2+ ions that attack the cell wall to kill microorganisms. ZnO NPs are photoconductors, and their high photocatalytic efficiency will contribute to the antimicrobial effect. In addition, when ZnO NPs interact with the cell wall, the pathogen cells are damaged, resulting in disorganization of the membrane.
ZnO NPs have been incorporated into biopolymers for food packaging to extend the shelf life of food. In a study of ZnO-chitosan nanocomposites for food packaging applications (Rahman et al., 2017), ZnO NPs were synthesized in a simple one-pot procedure by decomposing zinc acetate in the chitosan solution. The resultant composite films had strong inhibition against the growth of E. coli and S. aureus compared to the pure chitosan. The antimicrobial activity of the composite film increased more or less linearly with ZnO NP loading. It was suggested that ZnO NPs released ROS and Zn2+ ions that killed the bacteria. The composite films were then stitched into pouch-like bags to store fresh raw meat. After 6 days of storage under refrigerated conditions, the composite films containing 26 wt% ZnO NPs exhibited a total inhibition of bacterial growth. Zhang et al. (2021a) studied ZnO-PLA fibrous composite membranes by electrospinning for food packaging. The tensile strength of the composite membrane with 0.5 wt% ZnO NPs showed a 55% increase compared to the pure PLA membrane. This mechanical reinforcement was ascribed to the favorable interaction between PLA and ZnO NPs via hydrogen bonding. The composite membranes exhibited strong antibacterial activity against E. coli and S. aureus. The inhibition zone increased with the content of ZnO NPs, and the composite membrane with 1 wt% ZnO NPs had the maximum antibacterial effect. After 1 hour of ultrasonication, the inhibition zones of E. coli and S. aureus increased significantly. Under ultrasonication, ZnO NPs were well dispersed in the polymer matrix, which increased contact between ZnO NPs and PLA, leading to enhanced antibacterial activity.
US Food and Drug Administration (FDA) and European Food Safety Authority (EFSA) set guidelines under regulations Title 21 Code of Federal Regulations (21-CFR) and (EC) 1935/2004 for Food Contact Materials, respectively (Störmer et al., 2017). In particular, migrants from polymers or coatings may be a concern. Migration limits of various migrants have been defined and publicly available under “Specific Migration Limits” (SML) (EFSA Panel on Food Contact Materials Silano et al., 2020). EFSA has set Overall Migration Limits (OML) of 60 mg/kg (EFSA Panel on Food Contact Materials Silano et al., 2020). Acronym GRAS (generally recognized as safe) defined under of FDA is leveraged to indicate that the materials utilized are safe under the intended use conditions (Burdock and Carabin, 2004; Muncke et al., 2017). Starch, PLA, Chitin, Chitosan, Beeswax, Carnauba wax, Zein, and PBAT are identified as GRAS or approved for food contact applications (Siegenthaler et al., 2012; Zhang et al., 2015; Zhu, 2017; Ubeda et al., 2019; Szulc et al., 2020; Zhang et al., 2021b; Kritchenkov et al., 2021). However, any additives or processing aid can vary from grade to grade and supplier to supplier, as in the case with traditional petroderived mass plastics. The safety of any food contact materials and coating must be investigated at an early stage of application conceptualization.
Current studies on the toxicological effect of nanoparticle- (NP-) based coatings for food packaging on the human body are inconsistent and controversial due to the complexity of nanomaterials. The safety of NP-based coatings for food packing is highly dependent on the migration of NPs from packaging to foodstuff, which determine the toxicological profile of ingested NPs (Souza and Fernando, 2016; Ahari and Lahijani, 2021; Paidari et al., 2021). The migration behavior of NPs is considered a mass transfer process determined by many factors such as particle size, particle shape, interactions between particle and polymer matrix, heating, and storage time. In general, the migration of NPs from packaging would be slow, and the overall migration would be below the total legislative migration limit established for packaging materials. In a study of AgNP release from commercially available packaging materials in food simulants and meats, Trbojevich et al. (2020) reported that the AgNP release in meats (0.2–0.5 mg/kg) was much lower than that in food simulants (25 mg/kg) indicating an overestimating of AgNP migration in food simulants. It was reported that plate-like nanoclays exhibited lower migration than spherical AgNPs because of the larger size of nanoclays than AgNPs (Echegoyen et al., 2016). It was also observed that the release of nanoclays from packaging increased with storage temperature and time (Farhoodi et al., 2014). In a study of CNC-PLA composite films, Fortunati et al. (2012) reported that surface-modified CNCs had lower migration than pristine CNCs, which was attributed to better compatibility of modified CNCs with PLA than pristine CNCs. Recently, Yan et al. (2019) studied the graphene release from composite films into food simulants. It was found that the graphene release was a surface behavior as evidenced by the detachment of graphene-containing polymer flakes from the film surface. Similar nanocarbon release behavior from the film surface was observed in CNT-based composite films (Xia et al., 2017).
The potential health risk of the consumption of food containing NPs migrated from packaging is still far from being understood because it is highly dependent on NP sources, size, shape, surface chemistry, dose, and agglomeration (McCracken et al., 2016). The assessment of toxicity induced by digested NPs is focused on the epithelial cells of gastrointestinal (GI) tract and pathophysiological responses to oral NP administration to study basal cytotoxicity, inflammatory response, ROS generation, DNA damage, and protein dysfunction, among others (Naseer et al., 2018). In general, metal, metal oxide, and metalloid NPs are much more toxic than nanoclays, nanocarbons, and nanocellulose. Van der Zande et al. (2012) reported that AgNPs upon oral exposure were mainly present in the liver and spleen, followed by the lungs, kidney, testis, and brain, whereas the elimination of AgNPs in the testis and brain was extremely slow. McCracken et al. (2015) reported that AgNPs induced oxidative stress, cell cycle arrest, and inhibition of cell proliferation in the intestinal epithelial cells. Shipelin et al. (2020) studied the cytotoxic effects of nanoclays on the state of the barrier of the small intestine mucous membrane and observed nanoclay-induced disorders in the tissue structure. Digested nanoclays at a high dose of 100 mg/kg resulted in the formation of a dense layer of mucous overlays over the brush border of the enterocytes and absorption suppression of protein. Lopes et al. (2020) investigated the biological effect of digested CNFs in the GI tract. There was no cytotoxicity after exposure to unmodified and modified CNFs. Low or no cytotoxicity in the intestinal epithelium was also reported for pristine CNFs and CNCs (DeLoid et al., 2019). In a study of the cytotoxic effects of surface-functionalized GO, Jasim et al. (2016a) reported that the thicker GO accumulated in the liver and spleen, whereas thinner GO nanosheets were excreted through the renal pathway. There was no kidney function impairment up to 1 month after injection of individual GO at 10 mg/kg (Jasim et al., 2016b). However, the asbestos-like toxic behavior in the peritoneal cavity was reported for pristine CNTs (Poland et al., 2008). In a study of CD toxicity, Havrdova et al. (2016) reported that the neutral CDs showed high biocompatibility and no cytotoxicity, whereas surface-charged CDs caused high oxidative stress and affected the cell cycle. It should be noted that the current understanding of NP toxicity in the human body is limited, in particular for nanoclays, nanocellulose, and nanocarbons. Further investigations are needed concerning NP migration into foodstuff, NP absorption and reaction with organs, and NP excretion from the human body.
Coatings, essential for improved functional and barrier performance, can limit the repulpability and compostability of fibrous substrates. This review summarizes a multi-prong strategy to achieve sustainable repulpable barrier coatings for food packaging applications. Bio-derived macromolecular structures, bio-based compostable plastics, or petro-derived compostable polymers can be deployed. However, such structures may have some inherent limitations, which can be overcome by either deploying them as blends or as multilayer coating systems to reduce cost and achieve both water and oil barrier performance. Superior food packaging functional performance, such as antimicrobial properties and improved mechanical and barrier performance, can be achieved by adding nanoparticles, organic, inorganic, or hybrid fillers in the coating binder. Such high barrier and functional coatings promote circular bioeconomy, which reduces landfill burden generation from packaging material. Shelf life extension from active functional coatings can also contribute to reduced food wastage and carbon footprints.
The authors conceptualized the study jointly. AK and SZ both equally contributed to the writing and incorporated critical feedback from each other.
The authors declare that the research was conducted in the absence of any commercial or financial relationships that could be construed as a potential conflict of interest.
All claims expressed in this article are solely those of the authors and do not necessarily represent those of their affiliated organizations or those of the publisher, the editors, and the reviewers. Any product that may be evaluated in this article, or claim that may be made by its manufacturer, is not guaranteed or endorsed by the publisher.
Abdou, J. P., Reynolds, K. J., Pfau, M. R., van Staden, J., Braggin, G. A., Tajaddod, N., et al. (2016). Interfacial Crystallization of Isotactic Polypropylene Surrounding Macroscopic Carbon Nanotube and Graphene Fibers. Polymer 91, 136–145. doi:10.1016/j.polymer.2016.03.055
Abol-Fotouh, D., Hassan, M. A., Shokry, H., Roig, A., Azab, M. S., and Kashyout, A. E. B. (2020). Bacterial Nanocellulose from Agro-Industrial Wastes: Low-Cost and Enhanced Production by Komagataeibacter Saccharivorans MD1. Sci. Rep. 10 (1), 3491. doi:10.1038/s41598-020-60315-9
Ahankari, S. S., Subhedar, A. R., Bhadauria, S. S., and Dufresne, A. (2021). Nanocellulose in Food Packaging: A Review. Carbohydr. Polym. 255 (8), 117479. doi:10.1016/j.carbpol.2020.117479
Ahari, H., Anvar, A. A., Ataee, M., and Naeimabadi, M. (2021). Employing Nanosilver, Nanocopper, and Nanoclays in Food Packaging Production: A Systematic Review. Coatings 11 (5), 1–29. doi:10.3390/coatings11050509
Ahari, H., and Lahijani, L. K. (2021). Migration of Silver and Copper Nanoparticles from Food Coating. Coatings 11, 380. doi:10.3390/coatings11040380
Alias, M. F. A., and Abd – Alsada, A. S. (2021). The Influence of Zinc Oxide with Carbon Nanotube Composite NanoMaterials on Antibacterial Activity. J. Phys. Conf. Ser. 2114 (1), 012089. doi:10.1088/1742-6596/2114/1/012089
Anand, A., Unnikrishnan, B., Wei, S.-C., Chou, C. P., Zhang, L.-Z., and Huang, C.-C. (2019). Graphene Oxide and Carbon Dots as Broad-Spectrum Antimicrobial Agents - a Minireview. Nanoscale Horiz. 4 (1), 117–137. doi:10.1039/c8nh00174j
Andersson, C. (2008). New Ways to Enhance the Functionality of Paperboard by Surface Treatment - a Review. Packag. Technol. Sci. 21 (6), 339–373. doi:10.1002/pts.823
Anzar, N., Hasan, R., Tyagi, M., Yadav, N., and Narang, J. (2020). Carbon Nanotube - A Review on Synthesis, Properties and Plethora of Applications in the Field of Biomedical Science. Sensors Int. 1 (2), 100003. doi:10.1016/j.sintl.2020.100003
Arfat, Y. A., Ahmed, J., Ejaz, M., and Mullah, M. (2018). Polylactide/Graphene Oxide Nanosheets/Clove Essential Oil Composite Films for Potential Food Packaging Applications. Int. J. Biol. Macromol. 107 (1), 194–203. doi:10.1016/j.ijbiomac.2017.08.156
Arias, L. S., Pessan, J. P., Vieira, A. P. M., Lima, T. M. T., Delbem, A. C. B., and Monteiro, D. R. (2018). Iron Oxide Nanoparticles for Biomedical Applications: A Perspective on Synthesis, Drugs, Antimicrobial Activity, and Toxicity. Antibiot. (Basel) 7 (2). doi:10.3390/antibiotics7020046
Arora, A., and Padua, G. W. (2010). Review: Nanocomposites in Food Packaging. J. Food Sci. 75 (1), 43–49. doi:10.1111/j.1750-3841.2009.01456.x
A. Sukkar, K., Duha, S. A., A. Hussein, A., and Mohammad, R. M. (2019). Synthesis and Characterization Hybrid Materials (TiO2/MWCNTs) by Chemical Method and Evaluating Antibacterial Activity against Common Microbial Pathogens. Acta Phys. Pol. A 135 (4), 588–592. doi:10.12693/aphyspola.135.588
Azizi-Lalabadi, M., Hashemi, H., Feng, J., and Jafari, S. M. (2020). Carbon Nanomaterials against Pathogens; the Antimicrobial Activity of Carbon Nanotubes, Graphene/Graphene Oxide, Fullerenes, and Their Nanocomposites. Adv. Colloid Interface Sci. 284, 102250. doi:10.1016/j.cis.2020.102250
Bandyopadhyay, J., and Ray, S. S. (2019). Are Nanoclay-Containing Polymer Composites Safe for Food Packaging Applications?—An Overview. J. Appl. Polym. Sci. 136 (12). doi:10.1002/app.47214
Barra, A., Santos, J. D. C., Silva, M. R. F., Nunes, C., Ruiz-Hitzky, E., Gonçalves, I., et al. (2020). Graphene Derivatives in Biopolymer-Based Composites for Food Packaging Applications. Nanomater. (Basel) 10 (10), 1–32. doi:10.3390/nano10102077
Bi, S., Pan, H., Barinelli, V., Eriksen, B., Ruiz, S., and Sobkowicz, M. J. (2021). Biodegradable Polyester Coated Mulch Paper for Controlled Release of Fertilizer. J. Clean. Prod. 294, 126348. doi:10.1016/j.jclepro.2021.126348
Bilal, M., Gul, I., Basharat, A., and Qamar, S. A. (2021). Polysaccharides-Based Bio-Nanostructures and Their Potential Food Applications. Int. J. Biol. Macromol. 176, 540–557. doi:10.1016/j.ijbiomac.2021.02.107
Brodnjak, U. V., and Tihile, K. (2020). Chitosan Solution Containing Zein and Essential Oil as Bio Based Coating on Packaging Paper. Coatings 10 (1-13), 497. doi:10.3390/coatings10050497
Bumbudsanpharoke, N., and Ko, S. (2019). Nanoclays in Food and Beverage Packaging. J. Nanomater. 2019, 1–13. doi:10.1155/2019/8927167
Burdock, G. A., and Carabin, I. G. (2004). Generally Recognized as Safe (GRAS): History and Description. Toxicol. Lett. 150 (1), 3–18. doi:10.1016/j.toxlet.2003.07.004
Buxoo, S., and Jeetah, P. (2020). Feasibility of Producing Biodegradable Disposable Paper Cup from Pineapple Peels, Orange Peels and Mauritian Hemp Leaves with Beeswax Coating. SN Appl. Sci. 2 (8), 1–5. doi:10.1007/s42452-020-3164-7
Calva-Estrada, S. J., Jiménez-Fernández, M., and Lugo-Cervantes, E. (2019). Protein-Based Films: Advances in the Development of Biomaterials Applicable to Food Packaging. Food Eng. Rev. 11, 78–92. doi:10.1007/s12393-019-09189-w
Cao, C., Wang, Y., Zheng, S., Zhang, J., Li, W., Li, B., et al. (2020). Poly (Butylene Adipate-Co-Terephthalate)/Titanium Dioxide/Silver Composite Biofilms for Food Packaging Application. Lwt 132 (7), 109874. doi:10.1016/j.lwt.2020.109874
Cheng, H-Y., Yang, Y-J., Li, S-C., Hong, J-Y., and Jang, G-W. (2015). Modification and Extrusion Coating of Polylactic Acid Films. J. Appl. Polym. Sci. 132 (1-8), 42472. doi:10.1002/app.42472
Chungsiriporn, J., Khunthongkaew, P., Wongnoipla, Y., Sopajarn, A., Karrila, S., and Iewkittayakorn, J. (2022). Fibrous Packaging Paper Made of Oil Palm Fiber with Beeswax-Chitosan Solution to Improve Water Resistance. Industrial Crops Prod. 177, 114541. doi:10.1016/j.indcrop.2022.114541
Clarkson, C. M., El Awad Azrak, S. M., Forti, E. S., Schueneman, G. T., Moon, R. J., and Youngblood, J. P. (2021). Recent Developments in Cellulose Nanomaterial Composites. Adv. Mat. 33 (28), 14–17. doi:10.1002/adma.202000718
Coltelli, M.-B., Wild, F., Bugnicourt, E., Cinelli, P., Lindner, M., Schmid, M., et al. (2015). State of the Art in the Development and Properties of Protein-Based Films and Coatings and Their Applicability to Cellulose Based Products: An Extensive Review. Coatings 6 (1), 1. doi:10.3390/coatings6010001
Costa, S. M., Ferreira, D. P., Teixeira, P., Ballesteros, L. F., Teixeira, J. A., and Fangueiro, R. (2021). Active Natural-Based Films for Food Packaging Applications: The Combined Effect of Chitosan and Nanocellulose. Int. J. Biol. Macromol. 177, 241–251. doi:10.1016/j.ijbiomac.2021.02.105
Cui, F., Ye, Y., Ping, J., and Sun, X. (2020). Carbon Dots: Current Advances in Pathogenic Bacteria Monitoring and Prospect Applications. Biosens. Bioelectron. 156, 112085. doi:10.1016/j.bios.2020.112085
Cui, R., Jiang, K., Yuan, M., Cao, J., Li, L., Tang, Z., et al. (2020). Antimicrobial Film Based on Polylactic Acid and Carbon Nanotube for Controlled Cinnamaldehyde Release. J. Mater. Res. Technol. 9 (5), 10130–10138. doi:10.1016/j.jmrt.2020.07.016
de Campos, A., Claro, P. C., Luchesi, B. R., Miranda, M., Souza, F. V., Ferreira, M. D., et al. (2019). Curaua Cellulose Sheets Dip Coated with Micro and Nano Carnauba Wax Emulsions. Cellulose 26 (13), 7983–7993. doi:10.1007/s10570-019-02637-0
de Freitas, C. A. S., de Sousa, P. H. M., Soares, D. J., da Silva, J. Y. G., Benjamin, S. R., and Guedes, M. I. F. (2019). Carnauba Wax Uses in Food - A Review. Food Chem. 291, 38–48. doi:10.1016/j.foodchem.2019.03.133
DeLoid, G. M., Cao, X., Molina, R. M., Silva, D. I., Bhattacharya, K., Ng, K. W., et al. (2019). Toxicological Effects of Ingested Nanocellulose in In Vitro Intestinal Epithelium and In Vivo Rat Models. Environ. Sci. Nano 6 (7), 2105–2115. doi:10.1039/c9en00184k
Deshwal, G. K., Panjagari, N. R., and Alam, T. (2019). An Overview of Paper and Paper Based Food Packaging Materials: Health Safety and Environmental Concerns. J. Food Sci. Technol. 56, 4391–4403. doi:10.1007/s13197-019-03950-z
Detyothin, S., Kathuria, A., Jaruwattanayon, W., Seleke, S. E. M., and Auras, R. (2010). “Poly Lactic Acid Blends,” in Poly(lactic Acid): Synthesis, Structures, Properties, Processing, and Applications (Wiley). doi:10.1002/9780470649848.ch16
Díaz‐montes, E., and Castro‐muñoz, R. (2021). Trends in Chitosan as a Primary Biopolymer for Functional Films and Coatings Manufacture for Food and Natural Products. Polym. (Basel) 13 (5), 1–28.
Dufresne, A. (2013). Nanocellulose: A New Ageless Bionanomaterial. Mater. Today 16 (6), 220–227. doi:10.1016/j.mattod.2013.06.004
Echegoyen, Y., Rodríguez, S., and Nerín, C. (2016). Nanoclay Migration from Food Packaging Materials. Food Addit. Contam. Part A 33 (3), 530–539. doi:10.1080/19440049.2015.1136844
Echeverría, I., López-Caballero, M. E., Gómez-Guillén, M. C., Mauri, A. N., and Montero, M. P. (2018). Active Nanocomposite Films Based on Soy Proteins-Montmorillonite- Clove Essential Oil for the Preservation of Refrigerated Bluefin Tuna (Thunnus Thynnus) Fillets. Int. J. Food Microbiol. 266 (2), 142–149.
EFSA Panel on Food Contact Materials Silano, V., Barat Baviera, J. M., Bolognesi, C., Chesson, A., Cocconcelli, P. S., et al. (2020). Review and Priority Setting for Substances that Are Listed without a Specific Migration Limit in Table 1 of Annex 1 of Regulation 10/2011 on Plastic Materials and Articles Intended to Come into Contact with Food. EFSA J. 18 (6), e06124. doi:10.2903/j.efsa.2020.6124
EPA (2022). US EPA. Available at: https://www.epa.gov/facts-and-figures-about-materials-waste-and-recycling/paper-and-paperboard-material-specific-data.
Ezati, P., Roy, S., and Rhim, J.-W. (2022). Pectin/Gelatin-Based Bioactive Composite Films Reinforced with Sulfur Functionalized Carbon Dots. Colloids Surfaces A Physicochem. Eng. Aspects 636 (11), 128123. doi:10.1016/j.colsurfa.2021.128123
Farhoodi, M., Mousavi, S. M., Sotudeh-Gharebagh, R., Emam-Djomeh, Z., and Oromiehie, A. (2014). Migration of Aluminum and Silicon from PET/clay Nanocomposite Bottles into Acidic Food Simulant. Packag. Technol. Sci. 27 (2), 161–168. doi:10.1002/pts.2017
Fortunati, E., Peltzer, M., Armentano, I., Torre, L., Jiménez, A., and Kenny, J. M. (2012). Effects of Modified Cellulose Nanocrystals on the Barrier and Migration Properties of PLA Nano-Biocomposites. Carbohydr. Polym. 90 (2), 948–956. doi:10.1016/j.carbpol.2012.06.025
Frank, B. P., Durkin, D. P., Caudill, E. R., Zhu, L., White, D. H., Curry, M. L., et al. (2018). Impact of Silanization on the Structure, Dispersion Properties, and Biodegradability of Nanocellulose as a Nanocomposite Filler. ACS Appl. Nano Mat. 1 (12), 7025–7038. doi:10.1021/acsanm.8b01819
Fratini, F., Cilia, G., Turchi, B., and Felicioli, A. (2016). Beeswax: A Minireview of its Antimicrobial Activity and its Application in Medicine. Asian Pac. J. Trop. Med. 9 (9), 839–843. doi:10.1016/j.apjtm.2016.07.003
Gatto, M., Ochi, D., Yoshida, C. M. P., and da Silva, C. F. (2019). Study of Chitosan with Different Degrees of Acetylation as Cardboard Paper Coating. Carbohydr. Polym. 210, 56–63. doi:10.1016/j.carbpol.2019.01.053
Ghasemlou, M., Daver, F., Ivanova, E. P., Habibi, Y., and Adhikari, B. (2021). Surface Modifications of Nanocellulose: From Synthesis to High-Performance Nanocomposites. Prog. Polym. Sci. 119, 101418. doi:10.1016/j.progpolymsci.2021.101418
Gitari, B., Chang, B. P., Misra, M., Navabi, A., and Mohanty, A. K. (2019). A Comparative Study on the Mechanical, Thermal, and Water Barrier Properties of PLA Nanocomposite Films Prepared with Bacterial Nanocellulose and Cellulose Nanofibrils. BioResources 14 (1), 1867–1889.
Glenn, G., Shogren, R., Jin, X., Orts, W., Hart-Cooper, W., and Olsen, L. (2021). Per- and Polyfluoroalkyl Substances and Their Alternatives in Paper Food Packaging. Compr. Rev. Food Sci. Food Saf. 20, 2595–2625. doi:10.1111/1541-4337.12726
Gosecka, M., and Gosecki, M. (2015). Characterization Methods of Polymer Core-Shell Particles. Colloid Polym. Sci. 293, 2719–2740. doi:10.1007/s00396-015-3728-z
Gouvêa, R. F., Del Aguila, E. M., Paschoalin, V. M. F., and Andrade, C. T. (2018). Extruded Hybrids Based on Poly(3-Hydroxybutyrate-Co-3-Hydroxyvalerate) and Reduced Graphene Oxide Composite for Active Food Packaging. Food packag. Shelf Life 16 (2), 77–85.
Habibi, Y., Goffin, A.-L., Schiltz, N., Duquesne, E., Dubois, P., and Dufresne, A. (2008). Bionanocomposites Based on Poly(ε-Caprolactone)-Grafted Cellulose Nanocrystals by Ring-Opening Polymerization. J. Mat. Chem. 18 (41), 5002–5010. doi:10.1039/b809212e
Hamdani, S. S., Li, Z., Sirinakbumrung, N., and Rabnawaz, M. (2020). Zein and PVOH-Based Bilayer Approach for Plastic-free, Repulpable and Biodegradable Oil- and Water-Resistant Paper as a Replacement for Single-Use Plastics. Ind. Eng. Chem. Res. 59, 17856–17866. doi:10.1021/acs.iecr.0c02967
Havrdova, M., Hola, K., Skopalik, J., Tomankova, K., Petr, M., Cepe, K., et al. (2016). Toxicity of Carbon Dots - Effect of Surface Functionalization on the Cell Viability, Reactive Oxygen Species Generation and Cell Cycle. Carbon 99, 238–248. doi:10.1016/j.carbon.2015.12.027
Hendrawati, N., Wibowo, A., Chrisnandari, R., and Adawiyah, R. (2021). Biodegradable Foam Tray Based on Sago Starch with Beeswax as Coating Agent. IOP Conf. Ser. Mat. Sci. Eng. 1073, 012006. doi:10.1088/1757-899x/1073/1/012006
Hutton-Prager, B., Adenekan, K., Sypnewski, M., Smith, A., Meadows, M., and Calicdan, C. (2021). Hydrophobic Development and Mechanical Properties of Cellulose Substrates Supercritically Impregnated with Food-Grade Waxes. Cellulose 28 (3), 1633–1646. doi:10.1007/s10570-020-03628-2
Ifuku, S., Nogi, M., Abe, K., Handa, K., Nakatsubo, F., and Yano, H. (2007). Surface Modification of Bacterial Cellulose Nanofibers for Property Enhancement of Optically Transparent Composites: Dependence on Acetyl-Group DS. Biomacromolecules 8 (6), 1973–1978. doi:10.1021/bm070113b
Iwamoto, S., and Endo, T. (2015). 3 Nm Thick Lignocellulose Nanofibers Obtained from Esterified Wood with Maleic Anhydride. ACS Macro Lett. 4 (1), 80–83. doi:10.1021/mz500787p
Jacek, P., Dourado, F., Gama, M., and Bielecki, S. (2019). Molecular Aspects of Bacterial Nanocellulose Biosynthesis. Microb. Biotechnol. 12 (4), 633–649. doi:10.1111/1751-7915.13386
Jasim, D. A., Boutin, H., Fairclough, M., Ménard-Moyon, C., Prenant, C., Bianco, A., et al. (2016). Thickness of Functionalized Graphene Oxide Sheets Plays Critical Role in Tissue Accumulation and Urinary Excretion: a Pilot PET/CT Study. Appl. Mater. Today 4, 24–30. doi:10.1016/j.apmt.2016.04.003
Jasim, D. A., Murphy, S., Newman, L., Mironov, A., Prestat, E., McCaffrey, J., et al. (2016). The Effects of Extensive Glomerular Filtration of Thin Graphene Oxide Sheets on Kidney Physiology. ACS Nano 10 (12), 10753–10767. doi:10.1021/acsnano.6b03358
Jatoi, A. W., Ogasawara, H., Kim, I. S., and Ni, Q.-Q. (2020). Cellulose Acetate/Multi-Wall Carbon Nanotube/Ag Nanofiber Composite for Antibacterial Applications. Mater. Sci. Eng. C 110 (1), 110679. doi:10.1016/j.msec.2020.110679
Jin, T., Liu, T., Lam, E., and Moores, A. (2021). Chitin and Chitosan on the Nanoscale. Nanoscale Horiz. 6, 505–542. doi:10.1039/d0nh00696c
Johansson, C., Bras, J., Mondragon, I., Nechita, P., Plackett, D., Šimon, P., et al. (2012). Renewable Fibers and Bio-Based Materials for Packaging Applications - A Review of Recent Developments. BioRes 7 (2), 2506–2552. doi:10.15376/biores.7.2.2506-2552
Kansal, D., Hamdani, S. S., Ping, R., and Rabnawaz, M. (2020). Starch and Zein Biopolymers as a Sustainable Replacement for PFAS, Silicone Oil, and Plastic-Coated Paper. Ind. Eng. Chem. Res. 59, 12075–12084. doi:10.1021/acs.iecr.0c01291
Kassem, A., Ayoub, G. M., and Malaeb, L. (2019). Antibacterial Activity of Chitosan Nano-Composites and Carbon Nanotubes: A Review. Sci. Total Environ. 668, 566–576. doi:10.1016/j.scitotenv.2019.02.446
Kathuria, A., Abiad, M. G., and Auras, R. (2013). Toughening of Poly(l-Lactic Acid) with Cu3BTC2 Metal Organic Framework Crystals. Polymer 54, 6979–6986. doi:10.1016/j.polymer.2013.11.005
Kijchavengkul, T., and Auras, R. (2008). Compostability of Polymers. Polym. Int. 57, 793–804. doi:10.1002/pi.2420
Kijchavengkul, T., Auras, R., Rubino, M., Selke, S., Ngouajio, M., and Fernandez, R. T. (2010). Biodegradation and Hydrolysis Rate of Aliphatic Aromatic Polyester. Polym. Degrad. Stab. 95, 2641–2647. doi:10.1016/j.polymdegradstab.2010.07.018
Koenig, M. F., and Huang, S. J. (1994). Evaluation of Crosslinked Poly(caprolactone) as a Biodegradable, Hydrophobic Coating. Polym. Degrad. Stab. 45 (1), 139–144. doi:10.1016/0141-3910(94)90189-9
Koppolu, R., Lahti, J., Abitbol, T., Swerin, A., Kuusipalo, J., and Toivakka, M. (2019). Continuous Processing of Nanocellulose and Polylactic Acid into Multilayer Barrier Coatings. ACS Appl. Mat. Interfaces 11 (12), 11920–11927. doi:10.1021/acsami.9b00922
Kraśniewska, K., Galus, S., and Gniewosz, M. (2020). Biopolymers-Based Materials Containing Silver Nanoparticles as Active Packaging for Food Applications-A Review. Int. J. Mol. Sci. 21 (3). doi:10.3390/ijms21030698
Kritchenkov, A. S., Kletskov, A. V., Egorov, A. R., Tskhovrebov, A. G., Kurliuk, A. V., Zhaliazniak, N. V., et al. (2021). New Water-Soluble Chitin Derivative with High Antibacterial Properties for Potential Application in Active Food Coatings. Food Chem. 343, 128696. doi:10.1016/j.foodchem.2020.128696
Lacerda, S. P., Cerize, N. N. P., and Ré, M. I. (2011). Preparation and Characterization of Carnauba Wax Nanostructured Lipid Carriers Containing Benzophenone-3. Int. J. Cosmet. Sci. 33 (4), 312–321. doi:10.1111/j.1468-2494.2010.00626.x
Lai, S.-M., Huang, C.-K., and Shen, H.-F. (2005). Preparation and Properties of Biodegradable Poly(butylene Succinate)/starch Blends. J. Appl. Polym. Sci. 97, 257–264. doi:10.1002/app.21679
Lallo da Silva, B., Abuçafy, M. P., Berbel Manaia, E., Oshiro Junior, J. A., Chiari-Andréo, B. G., Pietro, R. C. R., et al. (2019). Relationship between Structure and Antimicrobial Activity of Zinc Oxide Nanoparticles: An Overview. Ijn 14, 9395–9410. doi:10.2147/ijn.s216204
Li, D., Gao, X., Huang, X., Liu, P., Xiong, W., Wu, S., et al. (2020). Preparation of Organic-Inorganic Chitosan@silver/Sepiolite Composites with High Synergistic Antibacterial Activity and Stability. Carbohydr. Polym. 249 (7), 116858. doi:10.1016/j.carbpol.2020.116858
Li, F., Yu, H.-Y., Wang, Y.-Y., Zhou, Y., Zhang, H., Yao, J.-M., et al. (2019). Natural Biodegradable Poly(3-Hydroxybutyrate-Co-3-Hydroxyvalerate) Nanocomposites with Multifunctional Cellulose Nanocrystals/Graphene Oxide Hybrids for High-Performance Food Packaging. J. Agric. Food Chem. 67 (39), 10954–10967. doi:10.1021/acs.jafc.9b03110
Li, H., Huang, J., Song, Y., Zhang, M., Wang, H., Lu, F., et al. (2018). Degradable Carbon Dots with Broad-Spectrum Antibacterial Activity. ACS Appl. Mat. Interfaces 10 (32), 26936–26946. doi:10.1021/acsami.8b08832
Li, H., Qi, Y., Zhao, Y., Chi, J., and Cheng, S. (2019). Starch and its Derivatives for Paper Coatings: A Review. Prog. Org. Coatings 135, 213–227. doi:10.1016/j.porgcoat.2019.05.015
Liminana, P., Garcia-sanoguera, D., Quiles-Carrillo, L., Balart, R., and Montanes, N. (2018). Development and Characterization of Environmentally Friendly Composites from Poly(butylene Succinate) (PBS) and Almond Shell Flour with Different Compatibilizers. Compos. Part B Eng. 144, 153–162. doi:10.1016/j.compositesb.2018.02.031
Lin, A. C., Xie, F., Chang, R., Beaver, N., Drewery, C., Collins, C., et al. (2021). Amyloid-Intercalated Graphene Oxide Membranes for Enhanced Nanofiltration. Carbon Trends 5, 100135. doi:10.1016/j.cartre.2021.100135
Lin, A. C., Xie, F., McCarthy, L. J., Rodgers, D. R., Hoff, K. G., von Welczeck, M. R., et al. (2022). Photonic Liquid Crystals of Graphene Oxide for Fast Membrane Nanofiltration. Carbon Trends 7, 100150. doi:10.1016/j.cartre.2022.100150
Liu, D., Mao, Y., and Ding, L. (2018). Carbon Nanotubes as Antimicrobial Agents for Water Disinfection and Pathogen Control. J. Water Health 16 (2), 171–180. doi:10.2166/wh.2018.228
Liu, J., Li, R., and Yang, B. (2020). Carbon Dots: A New Type of Carbon-Based Nanomaterial with Wide Applications. ACS Cent. Sci. 6 (12), 2179–2195. doi:10.1021/acscentsci.0c01306
Liu, S., Hu, M., Zeng, T. H., Wu, R., Jiang, R., Wei, J., et al. (2012). Lateral Dimension-dependent Antibacterial Activity of Graphene Oxide Sheets. Langmuir 28 (33), 12364–12372. doi:10.1021/la3023908
Lo Faro, E., Menozzi, C., Licciardello, F., and Fava, P. (2021). Improvement of Paper Resistance against Moisture and Oil by Coating with Poly(-3-Hydroxybutyrate-Co-3-Hydroxyvalerate) (PHBV) and Polycaprolactone (PCL). Appl. Sci. 11 (17), 8058. doi:10.3390/app11178058
Lopes, V. R., Strømme, M., and Ferraz, N. (2020). In Vitro biological Impact of Nanocellulose Fibers on Human Gut Bacteria and Gastrointestinal Cells. Nanomaterials 10 (6), 1159. doi:10.3390/nano10061159
Lyu, J. S., Lee, J.-S., and Han, J. (2019). Development of a Biodegradable Polycaprolactone Film Incorporated with an Antimicrobial Agent via an Extrusion Process. Sci. Rep. 9, 20236. doi:10.1038/s41598-019-56757-5
Mahmoodi, A., Ghodrati, S., and Khorasani, M. (2019). High-Strength, Low-Permeable, and Light-Protective Nanocomposite Films Based on a Hybrid Nanopigment and Biodegradable PLA for Food Packaging Applications. ACS Omega 4 (12), 14947–14954. doi:10.1021/acsomega.9b01731
Martucci, J. F., and Ruseckaite, R. A. (2017). Antibacterial Activity of Gelatin/Copper (II)-Exchanged Montmorillonite Films. Food Hydrocoll. 64, 70–77. doi:10.1016/j.foodhyd.2016.10.030
McCracken, C., Dutta, P. K., and Waldman, W. J. (2016). Critical Assessment of Toxicological Effects of Ingested Nanoparticles. Environ. Sci. Nano 3 (2), 256–282. doi:10.1039/c5en00242g
McCracken, C., Zane, A., Knight, D. A., Hommel, E., Dutta, P. K., and Waldman, W. J. (2015). Oxidative Stress-Mediated Inhibition of Intestinal Epithelial Cell Proliferation by Silver Nanoparticles. Toxicol. Vitro 29 (7), 1793–1808. doi:10.1016/j.tiv.2015.07.017
Mellinas, C., Ramos, M., Jiménez, A., and Garrigós, M. C. (2020). Recent Trends in the Use of Pectin from Agro-Waste Residues as a Natural-Based Biopolymer for Food Packaging Applications. Mater. (Basel) 13 (3). doi:10.3390/ma13030673
Menzel, C. (2020). Improvement of Starch Films for Food Packaging through a Three-Principle Approach: Antioxidants, Cross-Linking and Reinforcement. Carbohydr. Polym. 250 (7), 116828. doi:10.1016/j.carbpol.2020.116828
Moustafa, H., El Kissi, N., Abou-Kandil, A. I., Abdel-Aziz, M. S., and Dufresne, A. (2017). PLA/PBAT Bionanocomposites with Antimicrobial Natural Rosin for Green Packaging. ACS Appl. Mat. Interfaces 9, 20132–20141. doi:10.1021/acsami.7b05557
Muncke, J., Backhaus, T., Geueke, B., Maffini, M. V., Martin, O. V., Myers, J. P., et al. (2017). Scientific Challenges in the Risk Assessment of Food Contact Materials. Environ. Health Perspect. 125 (9), 095001. doi:10.1289/ehp644
Mustățea, G., Ungureanu, E. L., and Belc, N. (2019). Polylactic Acid (Pla) for Food Packaging Applications - a Short Overview. Ann. Food Sci. Technol. 20 (1), 9–14.
Muthuraj, R., Lacoste, C., Lacroix, P., and Bergeret, A. (2019). Sustainable Thermal Insulation Biocomposites from Rice Husk, Wheat Husk, Wood Fibers and Textile Waste Fibers: Elaboration and Performances Evaluation. Industrial Crops Prod. 135, 238–245. doi:10.1016/j.indcrop.2019.04.053
N, S., S, A. K., A, P., and S, G. (2020). Studies on Semi-crystalline Poly Lactic Acid (PLA) as a Hydrophobic Coating Material on Kraft Paper for Imparting Barrier Properties in Coated Abrasive Applications. Prog. Org. Coatings 145, 105682. doi:10.1016/j.porgcoat.2020.105682
Naseer, B., Srivastava, G., Qadri, O. S., Faridi, S. A., Islam, R. U., and Younis, K. (2018). Importance and Health Hazards of Nanoparticles Used in the Food Industry. Nanotechnol. Rev. 7 (6), 623–641. doi:10.1515/ntrev-2018-0076
Nath, D., R, S., Pal, K., and Sarkar, P. (2022). Nanoclay-Based Active Food Packaging Systems: A Review. Food Packag. Shelf Life 31 (5), 100803. doi:10.1016/j.fpsl.2021.100803
Navarro-Hortal, M. D., Orantes-Bermejo, F. J., Sánchez-González, C., Varela-López, A., Giampieri, F., Torres Fernández-Piñar, C., et al. (2019). Industrial-Scale Decontamination Procedure Effects on the Content of Acaricides, Heavy Metals and Antioxidant Capacity of Beeswax. Molecules 24 (8), 1518. doi:10.3390/molecules24081518
Nazrin, A., Sapuan, S. M., Zuhri, M. Y. M., Ilyas, R. A., Syafiq, R., and Sherwani, S. F. K. (2020). Nanocellulose Reinforced Thermoplastic Starch (TPS), Polylactic Acid (PLA), and Polybutylene Succinate (PBS) for Food Packaging Applications. Front. Chem. 8 (4), 213. doi:10.3389/fchem.2020.00213
Nechita, P., and Roman, M. (2020). Review on Polysaccharides Used in Coatings for Food Packaging Papers. Coatings 10, 566. doi:10.3390/coatings10060566
Ni, S., Zhang, H., Dai, H., and Xiao, H. (2018). Starch-Based Flexible Coating for Food Packaging Paper with Exceptional Hydrophobicity and Antimicrobial Activity. Polymers 10 (11), 1260. doi:10.3390/polym10111260
Nova Institute, European Bioplastics, (2022). Bioplastics Market Data. Available online: https://www.european-bioplastics.org/market/(accessed March 23, 2022).
Nuyken, O., and Pask, S. (2013). Ring-Opening Polymerization-An Introductory Review. Polymers 5 (2), 361–403. doi:10.3390/polym5020361
Oloyede, O. O., and Lignou, S. (2021). Sustainable Paper-Based Packaging: A Consumer's Perspective. Foods 10, 1035. doi:10.3390/foods10051035
Paidari, S., and Ibrahim, S. A. (2021). Potential Application of Gold Nanoparticles in Food Packaging: A Mini Review. Gold Bull. 54 (1), 31–36. doi:10.1007/s13404-021-00290-9
Paidari, S., Tahergorabi, R., Anari, E. S., Nafchi, A. M., Zamindar, N., and Goli, M. (2021). Migration of Various Nanoparticles into Food Samples: a Review. Foods 10 (9), 2114. doi:10.3390/foods10092114
Pattanshetti, A., Pradeep, N., Chaitra, V., and Uma, V. (2020). Synthesis of Multi-Walled Carbon Nanotubes (MWCNTs) from Plastic Waste & Analysis of Garlic Coated Gelatin/MWCNTs Nanocomposite Films as Food Packaging Material. SN Appl. Sci. 2 (4), 1–7. doi:10.1007/s42452-020-2442-8
Peighambardoust, S. J., Peighambardoust, S. H., Mohammadzadeh Pournasir, N., and Pakdel, P. (2019). Properties of Active Starch-Based Films Incorporating a Combination of Ag, ZnO and CuO Nanoparticles for Potential Use in Food Packaging Applications. Food packag. Shelf Life 22 (9). doi:10.1016/j.fpsl.2019.100420
Peng, C., and Chen, G. (2018). Preparation and Assessment of Heat-Treated α-Chitin Nanowhiskers Reinforced Poly(viny Alcohol) Film for Packaging Application. Materials 11 (10), 1883. doi:10.3390/ma11101883
Phanthong, P., Reubroycharoen, P., Hao, X., Xu, G., Abudula, A., and Guan, G. (2018). Nanocellulose: Extraction and Application. Carbon Resour. Convers. 1 (1), 32–43. doi:10.1016/j.crcon.2018.05.004
Pillai, C. K. S., Paul, W., and Sharma, C. P. (2009). Chitin and Chitosan Polymers: Chemistry, Solubility and Fiber Formation. Prog. Polym. Sci. 34, 641–678. doi:10.1016/j.progpolymsci.2009.04.001
Poland, C. A., Duffin, R., Kinloch, I., Maynard, A., Wallace, W. A. H., Seaton, A., et al. (2008). Carbon Nanotubes Introduced into the Abdominal Cavity of Mice Show Asbestos-like Pathogenicity in a Pilot Study. Nat. Nanotech 3 (7), 423–428. doi:10.1038/nnano.2008.111
Qin, Y., Zhang, S., Yu, J., Yang, J., Xiong, L., and Sun, Q. (2016). Effects of Chitin Nano-Whiskers on the Antibacterial and Physicochemical Properties of Maize Starch Films. Carbohydr. Polym. 147, 372–378. doi:10.1016/j.carbpol.2016.03.095
Qu, B., and Luo, Y. (2021). A Review on the Preparation and Characterization of Chitosan-Clay Nanocomposite Films and Coatings for Food Packaging Applications. Carbohydr. Polym. Technol. Appl. 2, 100102. doi:10.1016/j.carpta.2021.100102
Rafiqah, S. A., Khalina, A., Harmaen, A. S., Tawakkal, I. A., Zaman, K., Asim, M., et al. (2021). A Review on Properties and Application of Bio-Based Poly(Butylene Succinate). Polymers 13 (9), 1436. doi:10.3390/polym13091436
Rahman, P. M., Mujeeb, V. M. A., and Muraleedharan, K. (2017). Flexible Chitosan-Nano ZnO Antimicrobial Pouches as a New Material for Extending the Shelf Life of Raw Meat. Int. J. Biol. Macromol. 97, 382–391. doi:10.1016/j.ijbiomac.2017.01.052
Raina, S., Thakur, A., Sharma, A., Pooja, D., and Minhas, A. P. (2020). Bactericidal Activity of Cannabis Sativa Phytochemicals from Leaf Extract and Their Derived Carbon Dots and Ag@Carbon Dots. Mater. Lett. 262, 127122. doi:10.1016/j.matlet.2019.127122
Ramos-García, M., Bosquez-Molina, E., Hernández-Romano, J., Zavala-Padilla, G., Terrés-Rojas, E., Alia-Tejacal, I., et al. (2012). Use of Chitosan-Based Edible Coatings in Combination with Other Natural Compounds, to Control Rhizopus Stolonifer and Escherichia coli DH5α in Fresh Tomatoes. Crop Prot. 38, 1–6.
Raquez, J.-M., Murena, Y., Goffin, A.-L., Habibi, Y., Ruelle, B., DeBuyl, F., et al. (2012). Surface-Modification of Cellulose Nanowhiskers and Their Use as Nanoreinforcers into Polylactide: A Sustainably-Integrated Approach. Compos. Sci. Technol. 72 (5), 544–549. doi:10.1016/j.compscitech.2011.11.017
Raul, P. K., Thakuria, A., Das, B., Devi, R. R., Tiwari, G., Yellappa, C., et al. (2022). Carbon Nanostructures as Antibacterials and Active Food-Packaging Materials: A Review. ACS Omega 7 (14), 11555–11559. doi:10.1021/acsomega.2c00848
Rehan, M., El-Naggar, M. E., Mashaly, H. M., and Wilken, R. (2018). Nanocomposites Based on Chitosan/Silver/Clay for Durable Multi-Functional Properties of Cotton Fabrics. Carbohydr. Polym. 182 (11), 29–41. doi:10.1016/j.carbpol.2017.11.007
Rhim, J.-W., Lee, J.-H., and Hong, S.-I. (2006). Water Resistance and Mechanical Properties of Biopolymer (Alginate and Soy Protein) Coated Paperboards. LWT - Food Sci. Technol. 39 (7), 806–813. doi:10.1016/j.lwt.2005.05.008
Riahi, Z., Rhim, J.-W., Bagheri, R., Pircheraghi, G., and Lotfali, E. (2022). Carboxymethyl Cellulose-Based Functional Film Integrated with Chitosan-Based Carbon Quantum Dots for Active Food Packaging Applications. Prog. Org. Coatings 166 (3), 106794. doi:10.1016/j.porgcoat.2022.106794
Rortais, A., Barrucci, F., Ercolano, V., Linge, J., Christodoulidou, A., Cravedi, J.-P., et al. (2021). A Topic Model Approach to Identify and Track Emerging Risks from Beeswax Adulteration in the Media. Food control. 119, 107435. doi:10.1016/j.foodcont.2020.107435
Salimiraad, S., Safaeian, S., Basti, A. A., Khanjari, A., and Nadoushan, R. M. (2022). Characterization of Novel Probiotic Nanocomposite Films Based on Nano Chitosan/Nano Cellulose/Gelatin for the Preservation of Fresh Chicken Fillets. Lwt 162, 113429. doi:10.1016/j.lwt.2022.113429
Sapkota, K. P., Hassan, M. M., Shrestha, S., Hanif, M. A., Islam, M. A., Akter, J., et al. (2020). Heterojunction Formation between Copper(II) Oxide Nanoparticles and Single-Walled Carbon Nanotubes to Enhance Antibacterial Performance. Int. J. Pharm. 590 (6), 119937. doi:10.1016/j.ijpharm.2020.119937
Saravanan, A., Maruthapandi, M., Das, P., Ganguly, S., Margel, S., Luong, J. H. T., et al. (2020). Applications of N-Doped Carbon Dots as Antimicrobial Agents, Antibiotic Carriers, and Selective Fluorescent Probes for Nitro Explosives. ACS Appl. Bio Mat. 3 (11), 8023–8031. doi:10.1021/acsabm.0c01104
Sartori, T., Feltre, G., do Amaral Sobral, P. J., da Cunha, R. L., and Menegalli, F. C. (2019). Biodegradable Pressure Sensitive Adhesives Produced from Vital Wheat Gluten: Effect of Glycerol as Plasticizer. Colloids Surfaces A Physicochem. Eng. Aspects 560, 42–49. doi:10.1016/j.colsurfa.2018.09.069
Sawicki, T., Starowicz, M., Kłębukowska, L., and Hanus, P. (2022). The Profile of Polyphenolic Compounds, Contents of Total Phenolics and Flavonoids, and Antioxidant and Antimicrobial Properties of Bee Products. Molecules 27 (4), 1301. doi:10.3390/molecules27041301
Seidi, F., Yazdi, M. K., Jouyandeh, M., Habibzadeh, S., Munir, M. T., Vahabi, H., et al. (2022). Crystalline Polysaccharides: A Review. Carbohydr. Polym. 275, 118624. doi:10.1016/j.carbpol.2021.118624
Shankar, S., and Rhim, J.-W. (2018). Effects of Poly(butylene Adipate-Co-Terephthalate) Coating on the Water Resistant, Mechanical, and Antibacterial Properties of Kraft Paper. Prog. Org. Coatings 123, 153–159. doi:10.1016/j.porgcoat.2018.07.002
Shanmugam, K., Doosthosseini, H., Varanasi, S., Garnier, G., and Batchelor, W. (2019). Nanocellulose Films as Air and Water Vapour Barriers: A Recyclable and Biodegradable Alternative to Polyolefin Packaging. Sustain. Mater. Technol. 22, e00115. doi:10.1016/j.susmat.2019.e00115
Sharma, A., Thakur, M., Bhattacharya, M., Mandal, T., and Goswami, S. (2019). Commercial Application of Cellulose Nano-Composites - A Review. Biotechnol. Rep. 21, e00316. doi:10.1016/j.btre.2019.e00316
Shatrohan Lal, R. K. (2014). Synthesis of Organic Nanoparticles and Their Applications in Drug Delivery and Food Nanotechnology: A Review. J. Nanomater. Mol. Nanotechnol. 03 (04). doi:10.4172/2324-877710.4172/2324-8777.1000150
Shipelin, V. A., Gmoshinski, I. V., Sarkisyan, V. A., Khotimchenko, S. A., and Nikityuk, D. B. (2020). Influence of Bentonite Nanoclay on the State of the Rat Intestine Protective Barrier in the Experiment. Nanotechnologies Russ. 15 (7), 492–499. doi:10.1134/s199507802004014x
Shorey, R., and Mekonnen, T. H. (2022). Sustainable Paper Coating with Enhanced Barrier Properties Based on Esterified Lignin and PBAT Blend. Int. J. Biol. Macromol. 209, 472–484. doi:10.1016/j.ijbiomac.2022.04.037
Siegenthaler, K. O., Kunkel, A., Skupin, G., and Yamamoto, M. (2012). “Ecoflex®and Ecovio®: Biodegradable, Performance-Enabling Plastics,” in Synthetic Biodegradable Polymers. Editors B. Rieger, A. Kunel, G. W. Coates, R. Reichardt, E. Dinjus, and T. A. Zevaco (Adv Polym Sci Springer), 91–136.
Silva, F. A. G. S., Dourado, F., Gama, M., and Poças, F. (2020). Nanocellulose Bio-Based Composites for Food Packaging. Nanomater. (Basel) 10 (10), 1–29. doi:10.3390/nano10102041
Singh, A. A., Genovese, M. E., Mancini, G., Marini, L., and Athanassiou, A. (2020). Green Processing Route for Polylactic Acid-Cellulose Fiber Biocomposites. ACS Sustain. Chem. Eng. 8, 4128–4136. doi:10.1021/acssuschemeng.9b06760
Singha, S., and Hedenqvist, M. S. (2020). A Review on Barrier Properties of Poly(Lactic Acid)/Clay Nanocomposites. Polym. (Basel) 12 (5). doi:10.3390/polym12051095
Sotoudehnia, F., Mengistie, E., Alayat, A., and McDonald, A. G. (2021). Valorization of Waste Waxed Corrugated Cardboard via Pyrolysis for Recovering Wax. Environ. Prog. Sustain. Energy 40, e13566. doi:10.1002/ep.13566
Souza, V. G., and Fernando, A. L. (2016). Nanoparticles in Food Packaging: Biodegradability and Potential Migration to Food: a Review. Food Packag Shelf Life 8, 63–70.
Souza, V. G. L., Pires, J. R. A., Rodrigues, P. F., Lopes, A. A. S., Fernandes, F. M. B., Duarte, M. P., et al. (2018). Bionanocomposites of Chitosan/Montmorillonite Incorporated with Rosmarinus Officinalis Essential Oil: Development and Physical Characterization. Food Packag. Shelf Life 16 (12), 148–156. doi:10.1016/j.fpsl.2018.03.009
Starowicz, M., Hanus, P., Lamparski, G., and Sawicki, T. (2021). Characterizing the Volatile and Sensory Profiles, and Sugar Content of Beeswax, Beebread, Bee Pollen, and Honey. Molecules 26 (11), 3410. doi:10.3390/molecules26113410
Störmer, A., Bott, J., Kemmer, D., and Franz, R. (2017). Critical Review of the Migration Potential of Nanoparticles in Food Contact Plastics. Trends food Sci. Technol. 63, 39–50.
Sun, S., An, Q., Li, X., Qian, L., He, B., and Xiao, H. (2010). Synergistic Effects of Chitosan-Guanidine Complexes on Enhancing Antimicrobial Activity and Wet-Strength of Paper. Bioresour. Technol. 101, 5693–5700. doi:10.1016/j.biortech.2010.02.046
Sundar, N., Keerthana, P., Kumar, S. A., Kumar, G. A., and Ghosh, S. (2020). Dual Purpose, Bio-Based Polylactic Acid (PLA)-polycaprolactone (PCL) Blends for Coated Abrasive and Packaging Industrial Coating Applications. J. Polym. Res. 27 (1-18), 386. doi:10.1007/s10965-020-02320-0
Szulc, J., Machnowski, W., Kowalska, S., Jachowicz, A., Ruman, T., Steglińska, A., et al. (2020). Beeswax-modified Textiles: Method of Preparation and Assessment of Antimicrobial Properties. Polymers 12 (2), 344. doi:10.3390/polym12020344
Tang, H., Wu, J., Li, D., Shi, C., Chen, G., He, M., et al. (2020). High-strength Paper Enhanced by Chitin Nanowhiskers and its Potential Bioassay Applications. Int. J. Biol. Macromol. 150, 885–893. doi:10.1016/j.ijbiomac.2020.02.154
Thurber, H., and Curtzwiler, G. W. (2020). Suitability of Poly(butylene Succinate) as a Coating for Paperboard Convenience Food Packaging. Int. J. Biobased Plastics 2, 1–12. doi:10.1080/24759651.2020.1785094
Trbojevich, R. A., Khare, S., Lim, J.-H., Watanabe, F., Gokulan, K., Krohmaly, K., et al. (2020). Assessment of Silver Release and Biocidal Capacity from Silver Nanocomposite Food Packaging Materials. Food Chem. Toxicol. 145, 111728. doi:10.1016/j.fct.2020.111728
Trinh, B. M., Smith, M., and Mekonnen, T. H. (2022). A Nanomaterial-Stabilized Starch-Beeswax Pickering Emulsion Coating to Extend Produce Shelf-Life. Chem. Eng. J. 431, 133905. doi:10.1016/j.cej.2021.133905
Truong, V. I. T. T., Kumar, S. R., Pang, J. S., Liu, Y. K., Chen, D. W., and Lue, S. J. (2020). Synergistic Antibacterial Activity of Silver-Loaded Graphene Oxide towards Staphylococcus Aureus and Escherichia Coli. Nanomater. (Basel) 10 (2), 1–22. doi:10.3390/nano10020366
Tyagi, P., Salem, K. S., Hubbe, M. A., and Pal, L. (2021). Advances in Barrier Coatings and Film Technologies for Achieving Sustainable Packaging of Food Products - A Review. Trends Food Sci. Technol. 115, 461–485. doi:10.1016/j.tifs.2021.06.036
Ubeda, S., Aznar, M., Alfaro, P., and Nerín, C. (2019). Migration of Oligomers from a Food Contact Biopolymer Based on Polylactic Acid (PLA) and Polyester. Anal. Bioanal. Chem. 411 (16), 3521–3532. doi:10.1007/s00216-019-01831-0
Uysal Unalan, I., Cerri, G., Marcuzzo, E., Cozzolino, C. A., and Farris, S. (2014). Nanocomposite Films and Coatings Using Inorganic Nanobuilding Blocks (NBB): Current Applications and Future Opportunities in the Food Packaging Sector. RSC Adv. 4 (56), 29393–29428. doi:10.1039/c4ra01778a
Vaezi, K., Asadpour, G., and Sharifi, H. (2019). Effect of ZnO Nanoparticles on the Mechanical, Barrier and Optical Properties of Thermoplastic Cationic Starch/Montmorillonite Biodegradable Films. Int. J. Biol. Macromol. 124, 519–529. doi:10.1016/j.ijbiomac.2018.11.142
Van der Zande, M., Vandebriel, R. J., Van Doren, E., Kramer, E., Herrera Rivera, Z., Serrano-Rojero, C. S., et al. (2012). Distribution, Elimination, and Toxicity of Silver Nanoparticles and Silver Ions in Rats after 28-day Oral Exposure. ACS Nano 6 (8), 7427–7442. doi:10.1021/nn302649p
Vilarinho, F., Andrade, M., Buonocore, G. G., Stanzione, M., Vaz, M. F., and Sanches Silva, A. (2018). Monitoring Lipid Oxidation in a Processed Meat Product Packaged with Nanocomposite Poly(Lactic Acid) Film. Eur. Polym. J. 98 (11), 362–367. doi:10.1016/j.eurpolymj.2017.11.034
Vilarinho, F., Stanzione, M., Buonocore, G. G., Barbosa-Pereira, L., Sendón, R., Vaz, M. F., et al. (2021). Green Tea Extract and Nanocellulose Embedded into Polylactic Acid Film: Properties and Efficiency on Retarding the Lipid Oxidation of a Model Fatty Food. Food packag. Shelf Life 27, 100609. doi:10.1016/j.fpsl.2020.100609
Wang, D., Huang, J., Guo, Z., and Liu, W. (2021). Durable Mixed Edible Wax Coating with Stretching Superhydrophobicity. J. Mat. Chem. A 9 (3), 1495–1499. doi:10.1039/d0ta10638k
Wang, H., Sun, X., and Seib, P. (2001). Strengthening Blends of Poly(lactic Acid) and Starch with Methylenediphenyl Diisocyanate. J. Appl. Polym. Sci. 82 (7), 1761–1767. doi:10.1002/app.2018
Wang, Y., He, B., and Zhao, L. (2017). Fabrication of Hydrophobic Coating on Filter Paper from Self-Emulsifying Carnauba Wax-Alcohol Emulsions with Nano-TiO2 Particles for Water/diesel Separation. BioResources 12.
Whitehead, K. A., Vaidya, M., Liauw, C. M., Brownson, D. A. C., Ramalingam, P., Kamieniak, J., et al. (2017). Antimicrobial Activity of Graphene Oxide-Metal Hybrids. Int. Biodeterior. Biodegrad. 123, 182–190. doi:10.1016/j.ibiod.2017.06.020
Wu, H., Nagarajan, S., Zhou, L., Duan, Y., and Zhang, J. (2016). Synthesis and Characterization of Cellulose Nanocrystal-Graft-Poly(D-Lactide) and its Nanocomposite with Poly(L-Lactide). Polymer 103, 365–375. doi:10.1016/j.polymer.2016.09.070
Wu, Z., Huang, X., Li, Y.-C., Xiao, H., and Wang, X. (2018). Novel Chitosan Films with Laponite Immobilized Ag Nanoparticles for Active Food Packaging. Carbohydr. Polym. 199 (6), 210–218. doi:10.1016/j.carbpol.2018.07.030
Xia, L., Xu, M., Cheng, G., Yang, L., Guo, Y., Li, D., et al. (2018). Facile Construction of Ag Nanoparticles Encapsulated into Carbon Nanotubes with Robust Antibacterial Activity. Carbon 130, 775–781. doi:10.1016/j.carbon.2018.01.073
Xia, Y., Uysal Unalan, I., Rubino, M., and Auras, R. (2017). Carbon Nanotube Release from Polymers into a Food Simulant. Environ. Pollut. 229, 818–826. doi:10.1016/j.envpol.2017.06.067
Xin, Q., Shah, H., Nawaz, A., Xie, W., Akram, M. Z., Batool, A., et al. (2019). Antibacterial Carbon-Based Nanomaterials. Adv. Mat. 31 (45), e1804838. doi:10.1002/adma.201804838
Xiong, S.-J., Pang, B., Zhou, S.-J., Li, M.-K., Yang, S., Wang, Y.-Y., et al. (2020). Economically Competitive Biodegradable PBAT/Lignin Composites: Effect of Lignin Methylation and Compatibilizer. ACS Sustain. Chem. Eng. 8 (13), 5338–5346. doi:10.1021/acssuschemeng.0c00789
Yan, J.-W., Hu, C., Chen, K., and Lin, Q.-B. (2019). Release of Graphene from Graphene-Polyethylene Composite Films into Food Simulants. Food Packag. Shelf Life 20, 100310. doi:10.1016/j.fpsl.2019.100310
Ying, Z., Wu, D., Wang, Z., Xie, W., Qiu, Y., and Wei, X. (2018). Rheological and Mechanical Properties of Polylactide Nanocomposites Reinforced with the Cellulose Nanofibers with Various Surface Treatments. Cellulose 25 (7), 3955–3971. doi:10.1007/s10570-018-1862-8
York, D. W., Collins, S., and Rantape, M. (2019). Measuring the Permeability of Thin Solid Layers of Natural Waxes. J. colloid interface Sci. 551, 270–282. doi:10.1016/j.jcis.2019.03.104
Yu, H.-Y., Qin, Z.-Y., Sun, B., Yang, X.-G., and Yao, J.-M. (2014). Reinforcement of Transparent Poly(3-Hydroxybutyrate-Co-3-Hydroxyvalerate) by Incorporation of Functionalized Carbon Nanotubes as a Novel Bionanocomposite for Food Packaging. Compos. Sci. Technol. 94, 96–104. doi:10.1016/j.compscitech.2014.01.018
Yu, Z., Dhital, R., Wang, W., Sun, L., Zeng, W., Mustapha, A., et al. (2019). Development of Multifunctional Nanocomposites Containing Cellulose Nanofibrils and Soy Proteins as Food Packaging Materials. Food Packag. Shelf Life 21 (9), 100366. doi:10.1016/j.fpsl.2019.100366
Zhang, H., Bussini, D., Hortal, M., Elegir, G., Mendes, J., and Jordá Beneyto, M. (2016). PLA Coated Paper Containing Active Inorganic Nanoparticles: Material Characterization and Fate of Nanoparticles in the Paper Recycling Process. Waste Manag. 52, 339–345. doi:10.1016/j.wasman.2016.03.045
Zhang, Q., Tu, Q., Hickey, M. E., Xiao, J., Gao, B., Tian, C., et al. (2018). Preparation and Study of the Antibacterial Ability of Graphene Oxide-Catechol Hybrid Polylactic Acid Nanofiber Mats. Colloids Surfaces B Biointerfaces 172 (9), 496–505. doi:10.1016/j.colsurfb.2018.09.003
Zhang, R., Lan, W., Ji, T., Sameen, D. E., Ahmed, S., Qin, W., et al. (2021). Development of Polylactic Acid/ZnO Composite Membranes Prepared by Ultrasonication and Electrospinning for Food Packaging. Lwt 135 (7), 110072. doi:10.1016/j.lwt.2020.110072
Zhang, S., Li, M., Wang, R., Chang, L., Ju, H., Lin, W., et al. (2021). Superhydrophobic and Antioxidative Film Based on Edible Materials for Food Packaging. Langmuir 37 (16), 5066–5072. doi:10.1021/acs.langmuir.1c00637
Zhang, S., Majewski, P. W., Keskar, G., Pfefferle, L. D., and Osuji, C. O. (2011). Lyotropic Self-Assembly of High-Aspect-Ratio Semiconductor Nanowires of Single-Crystal ZnO. Langmuir 27 (18), 11616–11621. doi:10.1021/la200703u
Zhang, S., Minus, M. L., Zhu, L., Wong, C.-P., and Kumar, S. (2008). Polymer Transcrystallinity Induced by Carbon Nanotubes. Polymer 49 (5), 1356–1364. doi:10.1016/j.polymer.2008.01.018
Zhang, W., Lu, P., Qian, L., and Xiao, H. (2014). Fabrication of Superhydrophobic Paper Surface via Wax Mixture Coating. Chem. Eng. J. 250, 431–436. doi:10.1016/j.cej.2014.04.050
Zhang, W., Xiao, H., and Qian, L. (2014). Beeswax-chitosan Emulsion Coated Paper with Enhanced Water Vapor Barrier Efficiency. Appl. Surf. Sci. 300, 80–85. doi:10.1016/j.apsusc.2014.02.005
Zhang, Y., Cui, L., Che, X., Zhang, H., Shi, N., Li, C., et al. (2015). Zein-based Films and Their Usage for Controlled Delivery: Origin, Classes and Current Landscape. J. Control. release 206, 206–219. doi:10.1016/j.jconrel.2015.03.030
Keywords: sustainable coatings, paper coatings, nanocomposite, functional paper coatings, biomaterials, barrier coating, food packaging, nanoparticle coating
Citation: Kathuria A and Zhang S (2022) Sustainable and Repulpable Barrier Coatings for Fiber-Based Materials for Food Packaging: A Review. Front. Mater. 9:929501. doi: 10.3389/fmats.2022.929501
Received: 26 April 2022; Accepted: 26 May 2022;
Published: 07 July 2022.
Edited by:
João Reis, Fluminense Federal University, BrazilReviewed by:
Tong Wang, University of Tennessee, Knoxville, United StatesCopyright © 2022 Kathuria and Zhang. This is an open-access article distributed under the terms of the Creative Commons Attribution License (CC BY). The use, distribution or reproduction in other forums is permitted, provided the original author(s) and the copyright owner(s) are credited and that the original publication in this journal is cited, in accordance with accepted academic practice. No use, distribution or reproduction is permitted which does not comply with these terms.
*Correspondence: Ajay Kathuria, YWthdGh1cmlAY2FscG9seS5lZHU=; Shanju Zhang, c3poYW5nMDVAY2FscG9seS5lZHU=
Disclaimer: All claims expressed in this article are solely those of the authors and do not necessarily represent those of their affiliated organizations, or those of the publisher, the editors and the reviewers. Any product that may be evaluated in this article or claim that may be made by its manufacturer is not guaranteed or endorsed by the publisher.
Research integrity at Frontiers
Learn more about the work of our research integrity team to safeguard the quality of each article we publish.