- 1Department of Stomatology, Changhai Hospital, Navy Medical University, Shanghai, China
- 2Research Center of Developmental Biology, Department of Histology and Embryology, College of Basic Medicine, Naval Medical University, Shanghai, China
- 3State Key Laboratory of Military Stomatology and National Clinical Research Center for Oral Diseases and Shaanxi Engineering Research Center for Dental Materials and Advanced Manufacture, Department of Oral Implants, School of Stomatology, The Fourth Military Medical University, Xi’an, China
- 4Department of Stomatology, Dongfang Hospital, Tongji University, Shanghai, China
Biphasic calcium phosphate (BCP) containing hydroxyapatite (HA) and β-tricalcium phosphate (β-TCP) is considered the gold standard for bone repair. However, the complex synthesis of BCP restricts the wide application of BCP. In the preliminary work, we proved that the HA/β-TCP BCP ceramic material extracted from fishery waste had a good prospect as a bone replacement graft material for filling bone defects. This study aimed to explore the osteogenesis effect of BCP material derived from butterfish bones in vivo and in vitro. After loading human bone marrow mesenchymal stem cells (hBMSCs) with materials, we used scanning electron microscopy to observe cell adhesion and survival. Western blot analysis was used to detect osteogenic expression in vitro. Micro-computed tomography and hematoxylin–eosin staining were used to detect bone regeneration and material degradation rate in the rat femoral defect model. The results showed that hBMSCs grew well and adhered closely to the material. In vitro, the expression levels of bone formation–related markers ALP and Runx-2 of butterfish bones calcined at 900°C were generally higher than those in the other groups. Notably, in vivo, the osteogenesis ability of butterfish bones calcined at 900°C was almost comparable to that of the most commonly used Bio-Oss in clinical practice and was significantly better than that in the other groups. In summary, the BCP ceramic material derived from butterfish bones had good biocompatibility, osteoconductivity, and osteoinductivity, and had a good application prospect in the field of bone graft substitutes.
1 Introduction
Of the 1.6 million bone transplants performed in the United States each year, more than 6% are craniofacial bone transplants (Greenwald et al., 2001). More bone tissue reconstruction is needed due to medical or aesthetic reasons after the craniofacial bone defect is caused by trauma, tumor, deformity infection, and so forth, (Lee et al., 2019). Although autogenous bone grafts are the gold standard for bone grafts, autologous bone transplantation has the disadvantages of limited raw materials, secondary trauma when the bone is taken, and rapid bone resorption (Eppley et al., 2005; Neto et al., 2019).
Calcium phosphate ceramic materials are widely used as bone graft materials due to their high degree of similarity to natural bones (Boutinguiza et al., 2012). Hydroxyapatite (HA) is an inorganic component, which is the most similar to bones and teeth (Dorozhkin, 2016). Currently, HA with good biological activity has been widely studied as a substitute for bone grafts; It shows excellent osteoconductivity and has potential as an osteoinductivity material (Dorozhkin, 2013). Another calcium phosphate material, β-tricalcium phosphate (β-TCP), has lower mechanical strength but higher resorption (Jeong et al., 2019). At present, BCP ceramic materials are considered the gold standard for bone repair (Matiyal et al., 2021).
BCP can be synthesized artificially and naturally, but artificial synthesis requires complicated procedures and chemical reagents (Zhu et al., 2017; Terzioğlu et al., 2018). According to the Food and Agriculture Organization, more than 91 million tons of fish and shellfish are caught each year globally, and the discard rate is almost 50% (Fernández-Arias et al., 2021). Also, calcium phosphate materials can be obtained from fish bones by simple processing methods (Venkatesan et al., 2011; Piccirillo et al., 2013). At present, there are many studies to extract BCP from fishery waste and complete its characterization (Fernández-Arias et al., 2021; Matiyal et al., 2021; Zamri et al., 2021). In our previous study, we chose a marine fish that could be cultivated artificially—butterfish, and obtained HA and BCP from the fish bones through simple calcination at different temperatures to reduce the individual differences caused by the differences in the living environment of fish. The calcined fish bone has good pore structure, porosity, and nanograin size, and we think that it can be used as a raw material for preparing porous bioceramic bone replacement materials (Deng et al., 2021). However, most of the current research stays at the level of material preparation and characterization, with only simple biocompatibility testing, and lack of exploration of the in vitro and in vivo osteogenic properties of materials.
Based on previous findings and aiming to explore the osteogenic properties of materials, this study used tissue engineering to load human bone marrow mesenchymal stem cells (hBMSCs) with calcined butterfish bones and explore the osteogenic performance of BCP derived from butterfish bones in vivo and in vitro.
2 Materials and Methods
2.1 Material Preparation and Characterization
We completed the preparation and characterization of HA/β-TCP BCP ceramic materials derived from butterfish bones in the early stage We extracted BCP from butterfish bone that was calcined at 700–1100°C. Then, their composition and microstructure was characterized by X-ray diffraction (XRD), Fourier transform infrared spectroscopy (FTIR), inductively coupled plasma optical atom emissions spectroscopy (ICP-OES), scanning electron microscopy (SEM), and mercury intrusion porosimeter (MIP) (Deng et al., 2021).
2.2 In Vitro Cell Studies
2.2.1 Material Functional Modification
The calcined butterfish bones were ground and screened with 25-mesh and 40-mesh screens, and the diameter of the material was controlled at 450–700 µm. The screened butterfish bones and clinical bovine bones Bio-Oss (0.25–1 mm, Geistlich Pharma AG, Wolhusen, Switzerland) were sterilized using ultraviolet (UV) light, placed in a 24-well culture plate, and according to the calcination temperature separated into 700°C and 800°C, 900°C and 1000°C, and Bio-Oss (7, 8, 9, and 10, and B). The materials in each group were immersed in Sulfo-SANPAH solution (0.1 mg/ml, 22589; Thermo Scientific, MA, United States) and irradiated with UV light for 10 min. The Sulfo-SANPAH solution was then removed, and the materials were irradiated again for 5 min for hydrophilic modification of the material. Then, the materials were soaked in Matrigel (0.5 mg/ml, LDEV-free, 354234; Corning, NY, United States) and incubated in a humidified incubator at 37°C for 3 h to form a biologically active three-dimensional matrix, which simulates the structure, composition, physical properties and functions of the basement membrane of cells in vivo, which is beneficial to the culture and differentiation of cells in vitro. The substrate was rinsed with phosphate-buffered saline (PBS) before seeding the cells.
2.2.2 Cell Culture
hBMSCs were obtained from Naval Medical University (Shanghai, China). hBMSCs were cultured with 10% v/v fetal bovine serum (FBS; 10099141; Gibco, Australia) and 1% penicillin–streptomycin (10378016; Gibco) using α-minimal essential medium (α-MEM; 12561056; Gibco) as a growth medium. The cells are cultured in a 37°C, 5% CO2 incubator. The medium was changed every other day, and the cells were passaged when the density reached 75%–85%. The P4-generation cells were used in experiments. The P4-generation hBMSCs (200 μl, 1 × 106) were spread on the previously functionally modified material in the 24-well culture plate (7, 8, 9, 10, and B) and cultured in 1 ml of growth medium for 14 days.
2.2.3 Scanning Electron Microscope Analysis
A scanning electron microscope (SEM, Hitachi SU8220, Japan) was used to observe the microstructure of the calcined butterfish bone (700°C, 800°C, 900°C, 1000°C, and Bio-Oss) at high magnification (x100,000). ImageJ software was used to analyze the grain size of butterfish bones and Bio-Oss after calcination at different temperatures. The P4-generation hBMSCs were spread on the previously functionally modified material in the 24-well culture plate (7, 8, 9, 10, and B) and after culturing for 14 days, the material was washed with PBS and fixed with 2.5% glutaraldehyde at 4°C overnight. It was dehydrated with ethanol gradient series (30%–100%) and dried with a critical point dryer (Leica EM CPD300, Germany). Then, the coating and freeze fracturing system (Leica EM ACE200, Germany) was used to spray gold on the material. Finally, an SEM (Hitachi U8010, Japan) was used to observe the adhesion and growth of the cells on the material.
2.2.4 Western Blot Analysis
The P4-generation hBMSCs (200 μl, 1 × 106) were spread on the materials (7, 8, 9, 10, and B) placed in a 24-well plate and cultured for 14 days. The materials were carefully removed and placed in a new 24-well plate to avoid the adherence of cells to the bottom of the 24-well plate from affecting the experiment results. Subsequently, the cells were lysed with RIPA buffer (PC101; Epizyme, Shanghai, China) supplemented with protease and phosphatase inhibitors (A32959; Thermo Scientific), and the supernatant was taken. The protein concentration was determined with a BCA protein assay kit (P0012; Beyotime, China). The extracted protein was separated using SDS-PAGE and then transferred to a polyvinylidene fluoride membrane (ISEQ00010; MIillipore, MA, United States) by wet transfer. After blocking with rapid blocking solution buffer (PS108; Epizyme) for 30 min, the membrane and the primary antibody (Vinculin, 13901, Cell Signaling Technology; Alkaline Phosphatase, A0514, ABclonal; Runx-2, 12556, Cell Signaling Technology) were incubated overnight at 4°C and then incubated with horseradish peroxidase (HRP)-conjugated anti-rabbit (SA00001-2, Proteintech) secondary antibody at 25°C for 2 h. The membrane was subsequently reacted with the HRP substrate (WBKLS0100; Millipore) for 5 min, and the image was displayed using a chemiluminescence system (ChemiScope; Clinx Science Instruments Co. Ltd., Shanghai, China).
2.3 In Vivo
2.3.1 Rat Femoral Defect Model
Twenty-four 8-week-old Sprague–Dawley rats, weighing 250 ± 20 g, were purchased from Shanghai Jihui Laboratory Animal Technology Co. Ltd. (Shanghai, China) and divided into four groups (n = 6): control group, 700°C + hBMSCs, 900°C + hBMSCs, and Bio-Oss + hBMSCs (control group, 7 + C, 9 + C, and B + C). All animal experimental procedures were supported by Committee on Ethics of Biomedicine Research, Second Military Medical University. Each rat was anesthetized with 0.3% sodium pentobarbital via intraperitoneal injection, and the skin of the femoral surgery area was scraped off. A 1.5-cm incision was made in the distal femur, the skin and subcutaneous tissue were cut, the fascia and the muscle were separated, and the femoral condyle was exposed. A dental drill was used to drill a cylindrical defect with a diameter of 3 mm and a depth of 3 mm from the outside of the femoral condyle (Dong et al., 2020). Further, we implanted different materials (0.3 mg; 7, 9, and B) into the defect and then used a syringe to evenly instill hBMSCs (200 μl, 2 × 105) into the defect site. After the surgery was completed, the defect was covered with the muscle, the muscle layer and the skin were gently rinsed with normal saline, and the skin was sutured. Half of the rats in the group were randomly selected and sacrificed by the intraperitoneal injection of high-dose sodium pentobarbital 4 weeks after the surgery. The femurs were extracted. The remaining rats were sacrificed 8 weeks after the surgery, and the femurs were extracted.
2.3.2 Micro-Computed Tomography Analysis
The removed femurs were fixed overnight with 4% paraformaldehyde. Using a micro-computed tomography (CT) scanner (Skyscan 1275; Bruker, Germany), the fixed femurs were scanned under the conditions of a pixel size of 13 μm, a voltage of 50 kV, and a current of 60 µA. Then, CTAn and CTVox software were used to perform three-dimensional reconstruction analysis on the image. The bone volume/tissue volume (BV/TV) of the cortical bone area was calculated.
2.3.3 Histological Analysis
After being tested using micro-CT, the femurs were immersed in EDTA decalcification solution (Servicebio, China) and dehydrated with a series of ethanol solutions (50%–100%) of increasing concentration. After embedding in paraffin, the femurs were cut into 4-mm-thick sections. Then, hematoxylin–eosin staining was performed. Pannoramic DESK (P-MIDI, P250; 3D HISTECH, Hungary) was used to image the sections, and the new callus area was analyzed with ImageJ software.
2.4 Graphical Abstract
Figure 1A shows our general experimental procedure, including material modification, electron microscopic observation after in vitro loading of cells, Western blot analysis, and in vivo material and cell implantation and observation. Figure 1B shows the implantation of materials and cells in the rat femoral defect model.
3 Results
3.1 Characterization and Cell Adhesion
Figure 2A shows the SEM (x100k) images of the microstructure of Bio-Oss and butterfish bones (calcined at 700°C–1000°C), as well as the SEM (x250) images of the cell morphology and adhesion state of cells cultured on the functionally modified material for 14 days. Then, ImageJ software was used to analyze the grain size of the materials (Figure 2B). The grain size of the butterfish bone after calcination at 700°C was 30–110 nm, the grain size of the butterfish bone after calcination at 800°C was 45–150 nm, the grain size of the butterfish bone calcined at 900°C was 90–260 nm, and the grain size of the butterfish bone calcined at 1000°C was 110–300 nm. In addition, the natural pore structure of pomfret bone was shown, which demonstrated that the calcined butterfish bone has rich pore structure, including macropores and connecting pores connected between macropores. Bio-Oss grain size was relatively uniform, estimated as 50–70 nm. Besides, all materials had dense hBMSC adhesion. The hBMSCs grew well, with protruding pseudopods, and adhered to the materials in a star shape. The hBMSCs and their pseudopods were also observed in the pores of the materials.
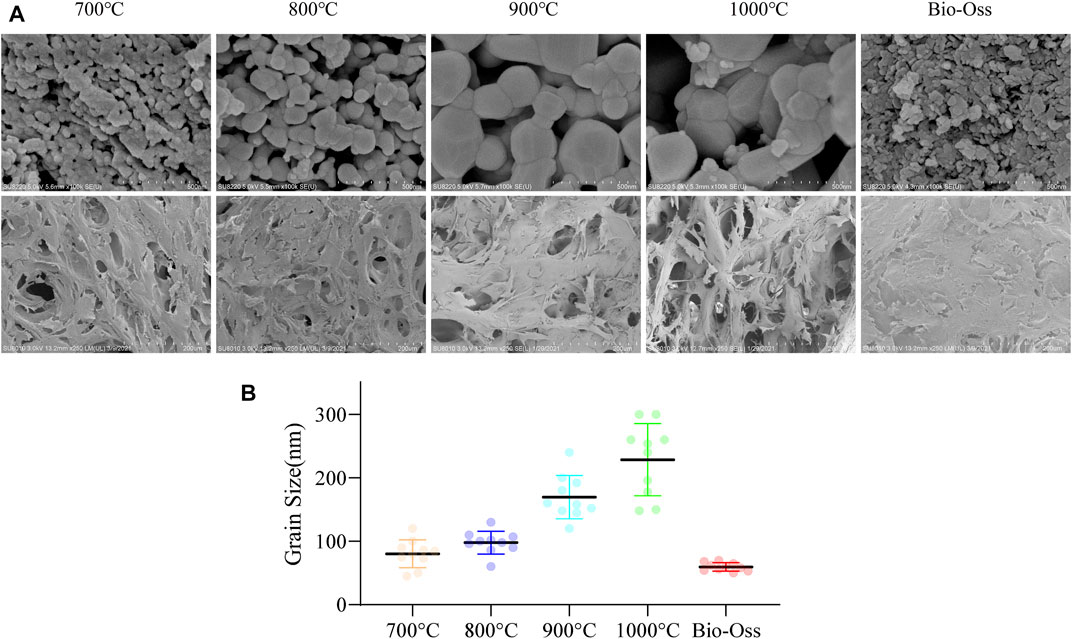
FIGURE 2. SEM observation of material microstructure and cell loading status (A). Quantitative statistics of particle size of materials (B). n = 10.
3.2 Osteogenesis-Related Marker Analysis
Vinculin was used as an internal control. As shown in Figure 3, after loading hBMSCs on the materials for 14 days, the expression level of early osteogenic marker ALP in groups 8, 9, 10, and B was significantly higher than that in group 7, while the expression levels in groups 8 and 9 were significantly higher than those in the other three groups. The early osteogenic marker Runx-2 was expressed in all groups; the expression in groups 8, 9, 10, and B was significantly higher than that in group 7, while the expression in group 9 was significantly higher than that in other groups.
3.3 Micro-Computed Tomography Three-Dimensional Reconstruction and Quantitative Analysis
Figure 4A shows the CT images and 3D reconstruction models of the cortical bone surface 4 weeks after the surgery. Still, defects were found in the cortical bone in each group. The 3D reconstruction models showed that the aperture caused by the surgery in the B + C group had a certain reduction. The CT images and 3D reconstruction models of the cortical bone surface 8 weeks after the surgery showed that despite some material on the outside of the bone cylinder, the cortical bone in each group was relatively well repaired. More residual materials were found inside the bone cylinder in the 7 + C and 9 + C groups. The degradation rate of the butterfish bones in vivo after calcination was lower than that of Bio-Oss (Figure 4B).
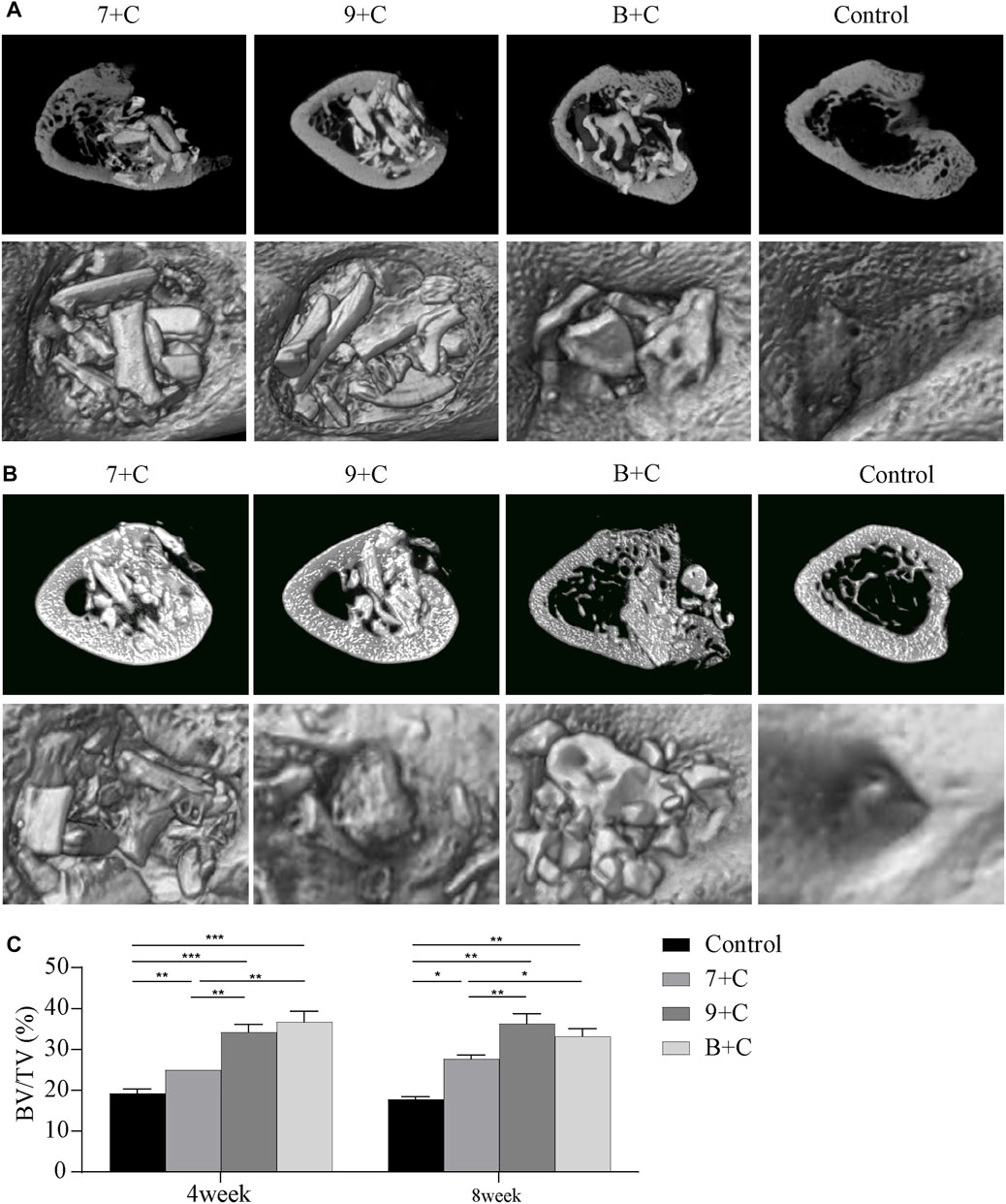
FIGURE 4. Micro-CT imaging images 4 weeks after surgery (A) and 8 weeks after surgery (B). Quantitative analysis of bone volume/tissue volume (BT/TV) in each group 4 weeks after surgery and 8 weeks after surgery (C). n = 3. *p < 0.05, **p < 0.005, ***p < 0.001.
Figure 4C shows the result of quantitative analysis of the BV/TV of the bone cylinder based on micro-CT images. Four weeks after the surgery, the BV/TV in each experimental group and the control group increased statistically significantly. The BV/TV in the 7 + C group was significantly lower than that in the B + C group, while BV/TV in the 7 + C group was significantly lower than that in the 9 + C group. No statistically significant difference was found between the 9 + C and B + C groups. Eight weeks after the surgery, no significant difference was found in BV/TV in each group compared with the 4-week groups. The BV/TV in each experimental group and the control group increased statistically significantly. The BV/TV in the 7 + C group was lower than that in the B + C group, the BV/TV in the 7 + C group was significantly lower than that in the 9 + C group, and no statistically significant difference was found between the 9 + C and B + C groups. The trend was basically the same 4 weeks after the surgery.
3.4 Histological Observation and Quantitative Analysis
Figure 5 shows that none of the implants in each group caused infection and severe inflammation. The single-layer cortical bone defects in the control group and each experimental group were more or less repaired 4 weeks after the surgery. However, obviously more new bone was formed around the materials and connected them into pieces in the bone marrow cavity in the 7 + C, 9 + C, and B + C groups compared with the control group; these three groups showed better osteoconductivity. Compared with Bio-Oss, the calcined butterfish bone had more pore structure and a large amount of new bone formed in the pores; however, basically no new bone was formed inside the Bio-Oss graft material (Figure 5A). Figure 4B shows the histological section 8 weeks after the surgery. It was seen that the single-layer cortical bone defects in each group were basically repaired. Consistent with the histological section results 4 weeks after the surgery, the 7 + C, 9 + C, and B + C groups also showed better osteoconductivity; more new bones were formed in the pores of the butterfish after calcination. However, the absorption rate of the graft material was higher in the B + C group than in the 7 + C and 9 + C groups.
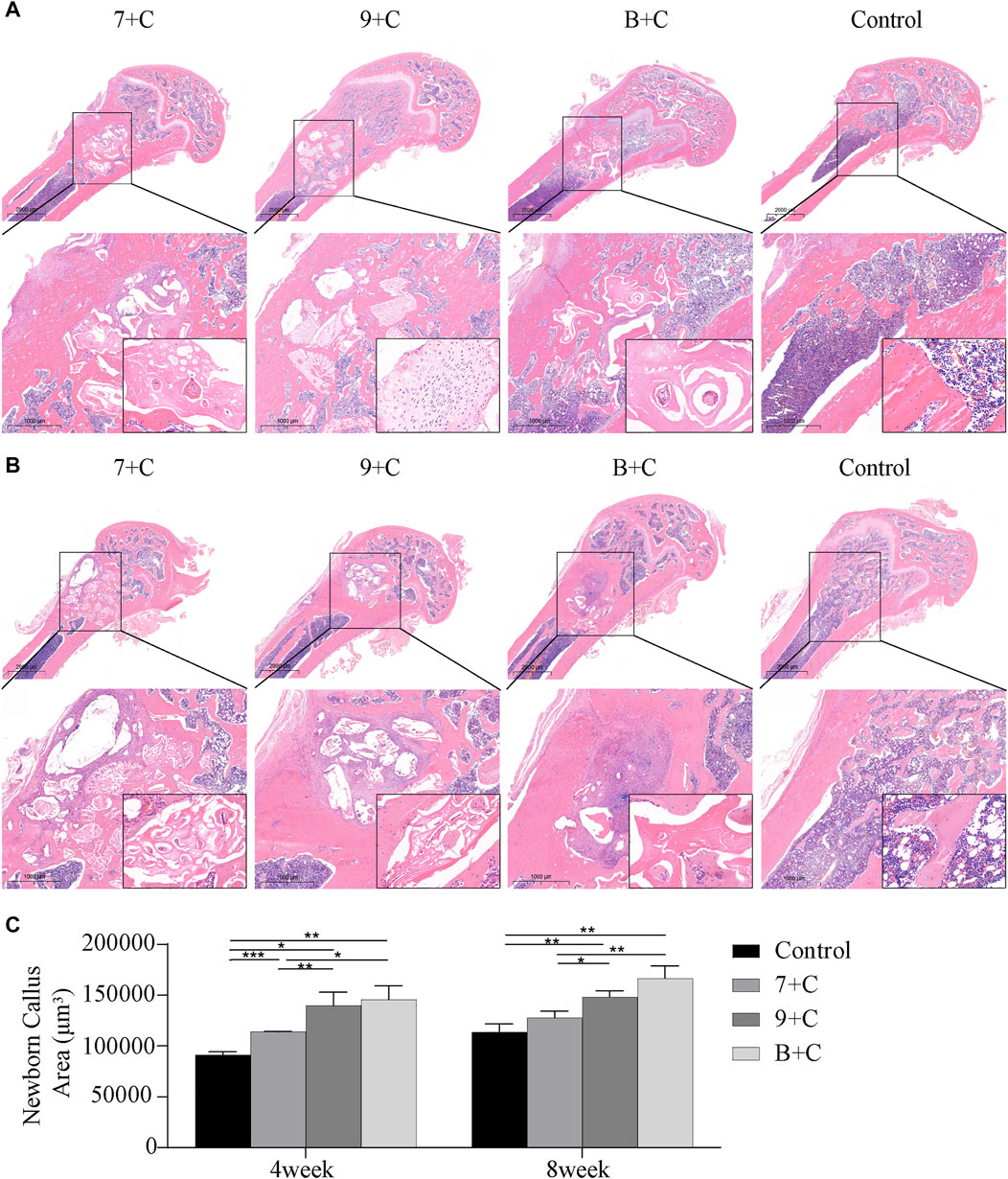
FIGURE 5. Hematoxylin-eosin staining images 4 weeks after surgery (A) and 8 weeks after surgery (B). Quantitative analysis of the area of new callus with ImageJ software (C). n = 3. *p < 0.05, **p < 0.005, ***p < 0.001.
ImageJ software was used to quantitatively calculate the area of new bone (Figure 5C). The amount of new bone statistically significantly increased in each experimental group compared with the control group 4 weeks after the surgery. The amount of new bone was significantly less in the 7 + C group than in the B + C group, and the amount of new bone was significantly less in the 7 + C group than in the 9 + C group. No statistically significant difference was found between the 9 + C and B + C groups. The amount of new bone in each group increased slightly in each group 8 weeks after the surgery compared with 4 weeks after the surgery, but it was not significant. Only the 9 + C and B + C groups had a statistically significant increase in new bone mass than the control group. The new bone mass in the 7 + C group was less than that in the B + C group, and the new bone mass in the 7 + C group was less than that in the 9 + C group. No statistically significant difference was found between the 9 + C and B + C groups 4 weeks after the surgery. Overall, the histological results were consistent with the micro-CT results.
4 Discussion
Previous studies have shown the application of calcium phosphate materials in many fields, especially in bone grafts (Ballouze et al., 2021; Dejob et al., 2021; Lodoso-Torrecilla et al., 2021). In an experiment conducted earlier, we considered extracting calcium phosphate materials from fishery waste. Then, we used the method of calcining butterfish bones at different temperatures to obtain materials containing only HA and also BCP materials containing HA and β-TCP. The results showed that the butterfish bone had the potential to be used as a raw material for further preparation of porous bioceramic scaffolds (Deng et al., 2021). Considering a wide range of sources and environmental protection, we believe that extracting calcium phosphate materials from fishery waste has broad prospects. In this study, we further explored the osteogenic effects of calcium phosphate derived from the butterfish bone in vivo and in vitro.
First, we observed the microstructure of the calcined butterfish bone and found that the crystal grains in each group of materials were at the nanometer level. Tiny pores were present between the crystal grains. The material crystal grains at the nanometer level were conducive to the adhesion, proliferation, and differentiation of stem cells. Also, the micropores could increase the surface area of the material, the attachment of cells, and the precipitation of biological HA (Rustom et al., 2016; Sethu et al., 2017). We observed that hBMSCs were firmly attached to the surface of each group of materials, with protruding pseudopods. The attachment state was good, indicating that the material had a good interaction with hBMSCs, and therefore it was a potential material for bone graft substitutes.
We used Western blot analysis to detect the bone formation–related markers ALP and Runx-2. The butterfish bones showed higher expression levels after calcining at 900°C, indicating that the bone formation ability in vitro was similar to that of the commonly used Bio-Oss in clinic. They were better than other groups, especially the butterfish bones calcined at 700°C. This might be because, in previous studies, only HA was contained in the butterfish bones after calcination at 700°C, β-TCP began to appear after the calcination temperature reached 800°C, and the ratio of the two-phase components after calcination at 900°C was more appropriate (Deng et al., 2021). As a bone graft substitute, HA had good biocompatibility and osteoconductivity. However, the lack of osteoinductive properties was a major disadvantage; BCP materials were proven to have better osteoinductive properties, which promoted the applications of these materials (Zhou et al., 2019; Zhao et al., 2020). Therefore, we believed that butterfish bones had better osteogenic properties after being calcined at 900°C in vitro.
In vivo, we used the calcined butterfish bones containing only HA (after calcination at 700°C), the calcined butterfish bones containing BCP (after calcination at 900°C), and Bio-Oss with hBMSCs and implanted them into a rat femoral defect model. Whether 4 weeks or 8 weeks after the surgery, micro-CT detection and quantitative analysis of BT/TV showed that the bone formation performance of butterfish bones containing BCP components calcined at 900°C was significantly better than that of butterfish bones calcined at 700°C, and comparable to that of the most commonly used commercial bone meal in clinical practice. The pore size of the butterfish bone calcined at 700°C was different from that of the butterfish bone calcined at 900°C. However, we believed that the presence of soluble β-TCP in the BCP material might have a more significant effect on bone healing (Habibovic et al., 2005; Li et al., 2017).
The conclusion of the histological staining results was almost the same as that of image detection. Compared with Bio-Oss, the calcined butterfish had certain defects. The micro-CT test and histological results showed that the calcined butterfish bone had a lower in vivo degradation rate. The low degradation rate of biological materials might increase the physical resistance to bone growth, affect the interaction with body fluids, and interfere with bone formation (da Silva Brum et al., 2019; Nazir and Iqbal, 2021; Prado et al., 2021). However, compared with Bio-Oss, the calcined butterfish bone also had certain advantages, such as its high porosity. The macroporosity of biomaterials provides a suitable environment for the formation of new bones and plays a key role in the expression of cell phenotype and the process of bone formation, while the microporosity dramatically increases the material’s adsorption of proteins (Toquet et al., 1999; Xie et al., 2004; Bouler et al., 2017). The histological staining results showed that bone tissue formation occurred in the pores of the butterfish bone after calcination, while Bio-Oss lacked the pores for bone tissue growth.
5 Conclusion
We proved that the BCP bioceramic material derived from butterfish bones had good biocompatibility and osteogenesis ability in vivo and in vitro. The biological material has a wide range of sources and a simple production method. Structurally, the material has a nanostructure and high porosity. Also, it has a two-phase composition. Further, it has certain osteoconductivity and osteoinductivity. Hence, it has a good development prospect as a bone replacement graft in the future.
Data Availability Statement
The original contributions presented in the study are included in the article/supplementary material, further inquiries can be directed to the corresponding authors.
Ethics Statement
The animal study was reviewed and approved by the committee on ethics of biomedicene research, second military medical university.
Author Contributions
SW and WM contributed to the concept and design of this study. ZL wrote the first draft. WD and QC wrote part of the manuscript. All authors participated in the revision of the manuscript, read and approved the submitted version.
Funding
The study was supported by the National Natural Science Foundation of China (Grant No: 81870808).
Conflict of Interest
The authors declare that the research was conducted in the absence of any commercial or financial relationships that could be construed as a potential conflict of interest.
Publisher’s Note
All claims expressed in this article are solely those of the authors and do not necessarily represent those of their affiliated organizations, or those of the publisher, the editors and the reviewers. Any product that may be evaluated in this article, or claim that may be made by its manufacturer, is not guaranteed or endorsed by the publisher.
References
Ballouze, R., Marahat, M. H., Mohamad, S., Saidin, N. A., Kasim, S. R., and Ooi, J. P. (2021). Biocompatible Magnesium‐Doped Biphasic Calcium Phosphate for Bone Regeneration. J. Biomed. Mater Res. 109, 1426–1435. doi:10.1002/jbm.b.34802
Bouler, J. M., Pilet, P., Gauthier, O., and Verron, E. (2017). Biphasic Calcium Phosphate Ceramics for Bone Reconstruction: A Review of Biological Response. Acta Biomater. 53, 1–12. doi:10.1016/j.actbio.2017.01.076
Boutinguiza, M., Pou, J., Comesaña, R., Lusquiños, F., de Carlos, A., and León, B. (2012). Biological Hydroxyapatite Obtained from Fish Bones. Mater. Sci. Eng. C 32, 478–486. doi:10.1016/j.msec.2011.11.021
da Silva Brum, I., de Carvalho, J. J., da Silva Pires, J. L., de Carvalho, M. A. A., Dos Santos, L. B. F., and Elias, C. N. (2019). Nanosized Hydroxyapatite and β-Tricalcium Phosphate Composite: Physico-Chemical, Cytotoxicity, Morphological Properties and In Vivo Trial. Sci. Rep. 9, 19602. doi:10.1038/s41598-019-56124-4
Dejob, L., Toury, B., Tadier, S., Grémillard, L., Gaillard, C., and Salles, V. (2021). Electrospinning of In Situ Synthesized Silica-Based and Calcium Phosphate Bioceramics for Applications in Bone Tissue Engineering: A Review. Acta Biomater. 123, 123–153. doi:10.1016/j.actbio.2020.12.032
Deng, K., Chen, H., Dou, W., Cai, Q., Wang, X., Wang, S., et al. (2021). Preparation and Characterization of Porous HA/β-TCP Biphasic Calcium Phosphate Derived from Butterfish Bone. Mater. Technol., 1–8. doi:10.1080/10667857.2021.1950886
Dong, R., Bai, Y., Dai, J., Deng, M., Zhao, C., Tian, Z., et al. (2020). Engineered Scaffolds Based on Mesenchymal Stem Cells/Preosteoclasts Extracellular Matrix Promote Bone Regeneration. J. Tissue Eng. 11, 2041731420926918. doi:10.1177/2041731420926918
Dorozhkin, S. V. (2016). Calcium Orthophosphates (CaPO4): Occurrence and Properties. Prog. Biomater. 5, 9–70. doi:10.1007/s40204-015-0045-z
Dorozhkin, S. V. (2013). Calcium Orthophosphates in Dentistry. J. Mater Sci. Mater Med. 24, 1335–1363. doi:10.1007/s10856-013-4898-1
Eppley, B. L., Pietrzak, W. S., and Blanton, M. W. (2005). Allograft and Alloplastic Bone Substitutes: A Review of Science and Technology for the Craniomaxillofacial Surgeon. J. Craniofac. Surg. 16, 981–989. doi:10.1097/01.scs.0000179662.38172.dd
Fernández-Arias, M., Álvarez-Olcina, I., Malvido-Fresnillo, P., Vázquez, J. A., Boutinguiza, M., Comesaña, R., et al. (2021). Biogenic Calcium Phosphate from Fish Discards and By-Products. Appl. Sci. 11, 3387. doi:10.3390/app11083387
Greenwald, A. S., Boden, S. D., Goldberg, V. M., Khan, Y., Laurencin, C. T., and Rosier, R. N. (2001). Bone-Graft Substitutes: Facts, Fictions, and Applications. J. Bone Jt. Surg. Am. 83 (Suppl. 2 Pt 2), 98–103. doi:10.2106/00004623-200100022-00007
Habibovic, P., Yuan, H., van der Valk, C. M., Meijer, G., van Blitterswijk, C. A., and de Groot, K. (2005). 3D Microenvironment as Essential Element for Osteoinduction by Biomaterials. Biomaterials 26, 3565–3575. doi:10.1016/j.biomaterials.2004.09.056
Jeong, J., Kim, J. H., Shim, J. H., Hwang, N. S., and Heo, C. Y. (2019). Bioactive Calcium Phosphate Materials and Applications in Bone Regeneration. Biomater. Res. 23, 4. doi:10.1186/s40824-018-0149-3
Lee, Y. C., Chan, Y. H., Hsieh, S. C., Lew, W. Z., and Feng, S. W. (2019). Comparing the Osteogenic Potentials and Bone Regeneration Capacities of Bone Marrow and Dental Pulp Mesenchymal Stem Cells in a Rabbit Calvarial Bone Defect Model. Int. J. Mol. Sci. 20, 5015. doi:10.3390/ijms20205015
Li, Y., Jiang, T., Zheng, L., and Zhao, J. (2017). Osteogenic Differentiation of Mesenchymal Stem Cells (MSCs) Induced by Three Calcium Phosphate Ceramic (CaP) Powders: A Comparative Study. Mater. Sci. Eng. C 80, 296–300. doi:10.1016/j.msec.2017.05.145
Lodoso-Torrecilla, I., van den Beucken, J., and Jansen, J. A. (2021). Calcium Phosphate Cements: Optimization toward Biodegradability. Acta Biomater. 119, 6985. doi:10.1016/j.actbio.2020.10.013
Matiyal, B., Sharma, H., Kumar, N., and Kaur, R. (2021). Microstructure and Biocompatibility of Biphasic Ceramic (HA/β-TCP) from Scales of Fresh Water Fish Common Carp (Cyprinus C). Mater. Lett. 304, 130669. doi:10.1016/j.matlet.2021.130669
Nazir, F., and Iqbal, M. (2021). Comparative Study of Crystallization, Mechanical Properties, and In Vitro Cytotoxicity of Nanocomposites at Low Filler Loadings of Hydroxyapatite for Bone-Tissue Engineering Based on Poly(l-Lactic acid)/Cyclo Olefin Copolymer. Polym. (Basel) 13, 841–845. doi:10.3390/polym13223865
Neto, A. S., Fonseca, A. C., Abrantes, J. C. C., Coelho, J. F. J., and Ferreira, J. M. F. (2019). Surface Functionalization of Cuttlefish Bone-Derived Biphasic Calcium Phosphate Scaffolds with Polymeric Coatings. Mater. Sci. Eng. C 105, 110014. doi:10.1016/j.msec.2019.110014
Piccirillo, C., Silva, M. F., Pullar, R. C., Braga da Cruz, I., Jorge, R., Pintado, M. M. E., et al. (2013). Extraction and Characterisation of Apatite- and Tricalcium Phosphate-Based Materials from Cod Fish Bones. Mater. Sci. Eng. C 33, 103–110. doi:10.1016/j.msec.2012.08.014
Prado, J. P. D. S., Yamamura, H., Magri, A. M. P., Ruiz, P. L. M., Prado, J. L. d. S., Rennó, A. C. M., et al. (2021). In Vitro and In Vivo Biological Performance of Hydroxyapatite from Fish Waste. J. Mater Sci. Mater Med. 32, 109. doi:10.1007/s10856-021-06591-x
Rustom, L. E., Boudou, T., Lou, S., Pignot-Paintrand, I., Nemke, B. W., Lu, Y., et al. (2016). Micropore-Induced Capillarity Enhances Bone Distribution In Vivo in Biphasic Calcium Phosphate Scaffolds. Acta Biomater. 44, 144–154. doi:10.1016/j.actbio.2016.08.025
Sethu, S. N., Namashivayam, S., Devendran, S., Nagarajan, S., Tsai, W.-B., Narashiman, S., et al. (2017). Nanoceramics on Osteoblast Proliferation and Differentiation in Bone Tissue Engineering. Int. J. Biol. Macromol. 98, 67–74. doi:10.1016/j.ijbiomac.2017.01.089
Terzioğlu, P., Öğüt, H., and Kalemtaş, A. (2018). Natural Calcium Phosphates from Fish Bones and Their Potential Biomedical Applications. Mater Sci. Eng. C Mater Biol. Appl. 91, 899–911. doi:10.1016/j.msec.2018.06.010
Toquet, J. l., Rohanizadeh, R., Guicheux, J. r., Couillaud, S. v., Passuti, N., Daculsi, G., et al. (1999). Osteogenic Potentialin Vitro of Human Bone Marrow Cells Cultured on Macroporous Biphasic Calcium Phosphate Ceramic. J. Biomed. Mat. Res. 44, 98–108. doi:10.1002/(sici)1097-4636(199901)44:1<98::aid-jbm11>3.0.co;2-p
Venkatesan, J., Qian, Z. J., Ryu, B., Thomas, N. V., and Kim, S. K. (2011). A Comparative Study of Thermal Calcination and an Alkaline Hydrolysis Method in the Isolation of Hydroxyapatite from Thunnus Obesus Bone. Biomed. Mat. 6, 035003. doi:10.1088/1748-6041/6/3/035003
Xie, J., Baumann, M. J., and McCabe, L. R. (2004). Osteoblasts Respond to Hydroxyapatite Surfaces with Immediate Changes in Gene Expression. J. Biomed. Mat. Res. 71A, 108–117. doi:10.1002/jbm.a.30140
Zamri, M. F. M. A., Bahru, R., Amin, R., Aslam Khan, M. U., Razak, S. I. A., Hassan, S. A., et al. (2021). Waste to Health: A Review of Waste Derived Materials for Tissue Engineering. J. Clean. Prod. 290, 125792. doi:10.1016/j.jclepro.2021.125792
Zhao, Q., Shi, M., Yin, C., Zhao, Z., Zhang, J., Wang, J., et al. (2020). Dual-Wavelength Photosensitive Nano-In-Micro Scaffold Regulates Innate and Adaptive Immune Responses for Osteogenesis. Nano-Micro Lett. 13, 28. doi:10.1007/s40820-020-00540-z
Zhou, K., Yu, P., Shi, X., Ling, T., Zeng, W., Chen, A., et al. (2019). Hierarchically Porous Hydroxyapatite Hybrid Scaffold Incorporated with Reduced Graphene Oxide for Rapid Bone Ingrowth and Repair. ACS Nano 13, 9595–9606. doi:10.1021/acsnano.9b04723
Keywords: biphasic calcium phosphate, bioceramic, fish bone, osteogenesis, osteoinductive
Citation: Deng K, Liu Z, Dou W, Cai Q, Ma W and Wang S (2022) HA/β-TCP Biphasic Calcium Phosphate Ceramics Derived From Butterfish Bones Loaded With Bone Marrow Mesenchymal Stem Cells Promote Osteogenesis. Front. Mater. 9:928075. doi: 10.3389/fmats.2022.928075
Received: 28 April 2022; Accepted: 17 June 2022;
Published: 11 July 2022.
Edited by:
Ashutosh Goel, Rutgers, The State University of New Jersey, United StatesReviewed by:
Sandra Pina, University of Minho, PortugalKannan Sanjeevi, Pondicherry University, India
Copyright © 2022 Deng, Liu, Dou, Cai, Ma and Wang. This is an open-access article distributed under the terms of the Creative Commons Attribution License (CC BY). The use, distribution or reproduction in other forums is permitted, provided the original author(s) and the copyright owner(s) are credited and that the original publication in this journal is cited, in accordance with accepted academic practice. No use, distribution or reproduction is permitted which does not comply with these terms.
*Correspondence: Shaohai Wang, shaohaiwang@smmu.edu.cn; Wei Ma, 4138088@qq.com
†These authors have contributed equally to this work