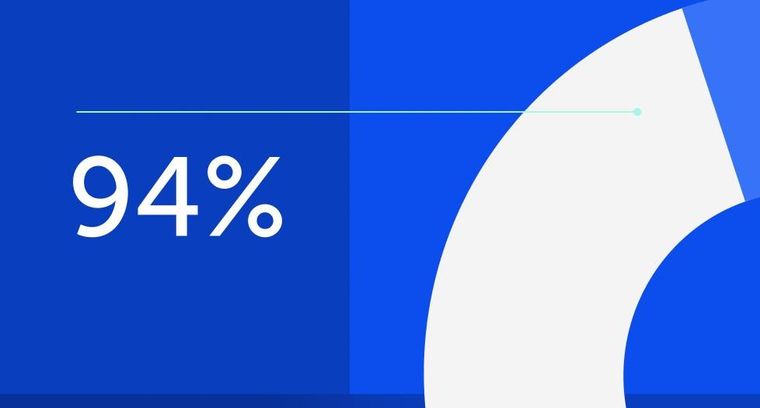
94% of researchers rate our articles as excellent or good
Learn more about the work of our research integrity team to safeguard the quality of each article we publish.
Find out more
MINI REVIEW article
Front. Mater., 22 July 2022
Sec. Biomaterials
Volume 9 - 2022 | https://doi.org/10.3389/fmats.2022.918890
This article is part of the Research TopicCelebrating 20 Years of CICECO – Aveiro Institute of Materials - Current and future perspectives in the use of Material Sciences, Chemistry, and Photonics for a more sustainable futureView all 20 articles
Glycine is the simplest natural amino acid, a basic building block for various biomaterials. Supramolecular packing of glycine molecules into three main crystalline polymorphs allows controlling their functional properties, such as piezoelectricity and ferroelectricity. Though piezoelectricity in glycine is well studied and reviewed, its ferroelectric properties were not summarized and analyzed until now. In this mini-review, we briefly discuss glycine polymorphs, their functional properties, and phase transitions, review recent findings on domain structure and polarization switching in β- and γ-glycine, and consider their possible applications in biocompatible photonic and piezoelectric devices.
Amino acid glycine is the simplest building block of various biomaterials and is often considered a symbol of life on our planet. Therefore, it has attracted considerable attention in different research fields, mainly in materials science, pharmacology, and medicine. Glycine plays a key role in many physiological processes, e.g., in cancer cell metabolism, (Jain et al., 2012) and is used to treat various diseases, including ischemic stroke, anxiety, insomnia, schizophrenia, benign prostatic hyperplasia, etc (Gusev et al., 2000; Babić and Babić, 2009). It also serves as a bulking agent in pharmaceutical protein formulations (Horn et al., 2018). On a completely different scale, glycine has been found in the interstellar medium (Kuan et al., 2003; Ioppolo et al., 2021) and in the comas of comets and meteorites, (Elsila et al., 2009; Altwegg et al., 2016) thus providing evidence of the panspermia hypothesis (Wesson, 2010). As for the functional physical properties of crystalline glycine, they largely depend on the supramolecular packing of its molecules into three main polymorphs (α, β, and γ), resulting in different crystallographic structures (Heredia et al., 2012; Guerin et al., 2017; Gleeson et al., 2020; Boldyreva, 2021). It has been a long time established that the α-phase is centrosymmetric (space group P21/n), in which only surface piezoelectricity and pyroelectricity may exist via doping or water incorporation (Piperno et al., 2013; Meirzadeh et al., 2018; Dishon et al., 2020). On the contrary, β and γ phases of glycine are non-centrosymmetric (space groups P21 and P31, respectively) (Iitaka, 1960; Iitaka, 1961) and exhibit technologically significant piezoelectric responses, as has been shown by Lemanov in 2000 (Lemanov, 2000).
It was like that until 2012, when another important functional property, ferroelectricity, was reported by Heredia et al. (2012). Using a novel Piezoresponse Force Microscopy (PFM) tool and molecular modeling, it has been shown, that switchable polarization domains exist in γ-glycine microcrystals grown from the solution. Polarization switching was performed by applying DC voltage to the PFM tip at the nanoscale and switchable domains were shown to persist for a long time. That work attracted a wide interest of the research community in different fields and has launched a new round of experimental and theoretical studies of glycine (Bystrov et al., 2014; Bystrov et al., 2015; Bystrov et al., 2016; Guerin et al., 2017; Seyedhosseini et al., 2017; Bai et al., 2018; Tasnim et al., 2018; Hu et al., 2019; Slabov et al., 2019; Bishara et al., 2020; Dishon et al., 2020; Gleeson et al., 2020; Hosseini et al., 2020; Kholkin et al., 2021). Several reviews reported piezoelectric properties of glycine (Tayi et al., 2015; Tofail and Bauer, 2016; Guerin et al., 2017; Maiti et al., 2019; Kim et al., 2020; Li et al., 2020; Boldyreva, 2021; Xu et al., 2021). However, until now, its ferroelectric properties have not been summarized and analyzed.
In this mini-review, we summarize 10 years of rigorous research on ferroelectricity and polarization switching in β-glycine, discuss undesirable phase transitions between polymorphs influencing the ferroelectricity, ways of stabilization of ferroelectric β-phase, and its possible applications in biocompatible photonic and piezoelectric devices.
Ferroelectricity is observed for a specific polymorphic state of the glycine—β-phase. Actually, glycine is a model material for studying polymorphism because crystalline glycine can be easily grown from the aqueous solution that, in principle, results in a mixture of different polymorphs—α, β, and γ. That is due to the Ostwald rule of stages, meaning that the less stable β-phase is formed first, (Seyedhosseini et al., 2014) and then more stable γ- and α-phases appear. Several papers discussed various possibilities to grow predominantly one phase or another (Boldyreva et al., 2003a; Lee et al., 2008; Poornachary et al., 2008). For instance, selective growth of single-phase piezoelectric γ-glycine can be achieved by the modification of the solution’s pH (Boldyreva et al., 2003a; Lee et al., 2008) or addition of trace amounts of chiral impurities into the aqueous solution, (Poornachary et al., 2008) whereas less stable β-phase can be preferentially grown from the solution in the presence of acetic acid (Drebushchak et al., 2002) or glucose (Gerasimov et al., 2022).
In general, polymorphic crystalline phases can be distinguished by various analytical techniques such as differential scanning calorimetry (DSC), thermogravimetric analysis (TGA), infrared, Raman, and solid-state nuclear magnetic resonance (NMR) spectroscopies, and powder X-ray diffraction (Brog et al., 2013). These integral methods allow detecting the presence of tiny amounts of phases in a mixture. However, for local polarization switching experiments by PFM, the polymorphic phase of an individual microcrystal should be determined. In this case, the choice of the methods is quite limited. Roughly, a phase of an individual crystal can be identified by observation of crystals’ habits by optical microscopy. Evaporation of the glycine aqueous solution droplet on a substrate leads to the formation of rhombohedral plate-like α-glycine crystals (Figure 1A), β-glycine forms elongated rectangular (needle-like) crystals (Figure 1B), and γ-glycine forms trigonal prisms (Figure 1C) (Iitaka, 1961; Bai et al., 2018). However, this is true for as-grown phases only. Due to polymorphic phase transitions, the crystal’s phase may change under various external factors (temperature, gases, mechanical treatment, etc.) (Boldyreva et al., 2003b) without habit modification (Isakov et al., 2014). Therefore, special methods for the phase determination of individual microcrystals should be applied prior to studies of ferroelectricity.
FIGURE 1. Morphology of the glycine microcrystals of different polymorphs: (A) α-phase, (B) β-phase, (C) γ-phase, and (D) microislands of β-glycine. The inset in (B) shows another common morphology of β-glycine crystals well suitable for studies of ferroelectricity. Types of as-grown domains in β-glycine (Vasileva et al., 2019): (E) stripe domains with charged domain walls, (F) quasi-periodic ensembles of needle-like domains, and (G) irregular shaped domains with segmental step-like domain walls. White arrows show the direction of spontaneous polarization. (H) Artificial domains created by the application of an external electric field to the PFM tip.
This problem can be partially resolved by PFM, which allows distinguishing the centrosymmetric α-phase (which does not possess any piezoelectric activity) from non-centrosymmetric β- and γ- phases (a priori piezoactive). But the distinction between β- and γ- phases is more difficult. PFM measurements in combination with crystal habit observation were used in (Heredia et al., 2012); however, they have led to an erroneous attribution of ferroelectricity to the γ-phase. Further studies (Isakov et al., 2014; Seyedhosseini et al., 2014; Seyedhosseini et al., 2015; Bystrov et al., 2016; Seyedhosseini et al., 2017; Slabov et al., 2019; Vasileva et al., 2019) showed that experimental results reported in (Heredia et al., 2012) could be actually done on β-phase.
The discrimination between β- and γ-phases by PFM can be done based on the determination of the components of the piezoelectric matrix, which are different for β- and γ-phases (Guerin et al., 2017). However, this method requires precise measurements at different crystal faces, which are not always accessible. Therefore, a more convenient method for the determination of the polymorphic phase of an individual microcrystal is confocal Raman microscopy (CRM). Application of this fast and non-destructible method just before the PFM measurements allows excluding possible artifacts with phase determination. CRM is based on the analysis of spectral fingerprints of each phase (Lee et al., 2008; Surovtsev et al., 2011; Seyedhosseini et al., 2014) and was successfully used for distinguishing phases in micro- and nanocrystals of glycine (Seyedhosseini et al., 2014; Zelenovskiy et al., 2016; Seyedhosseini et al., 2017; Slabov et al., 2019) and in situ studying an unusual solid-state phase transition (Isakov et al., 2014).
What is especially important is that CRM demonstrated preferable nucleation and stabilization of individual microcrystals of β-glycine and its quasi-regular arrays of nanocrystals formed via evaporative dewetting on a Pt substrate (Figure 1D) (Seyedhosseini et al., 2014; Seyedhosseini et al., 2017). This fact opens the way for studying ferroelectricity in β-glycine and its applications. Preferable β-phase crystallization can be also achieved in nanopores (Hamilton et al., 2008), microfluidic channels (Bhamidi et al., 2015), or under the action of standing surface acoustic waves (Bai et al., 2018). These are promising but more complicated and less studied ways for the ferroelectricity stabilization in glycine.
In 2005, the low-temperature calorimetric measurements on β-glycine revealed an anomaly of heat capacity at 252 K (Drebushchak et al., 2005), which was then confirmed by incoherent inelastic neutron scattering (Bordallo et al., 2008; Aree et al., 2013). Other polymorphs did not show such anomaly. The calculations of enthalpy, entropy, and Gibbs energy for all three polymorphs showed that their thermodynamic properties (and thus macroscopic physical properties) are affected by the arrangement of NH3+ tails of zwitterions rather than by the crystal structure (Drebushchak et al., 2005). Indeed, though, in the gas phase, the zwitterionic form of glycine molecule is less stable than the neutral one (Palla et al., 1980), it can be stabilized in a solution through the hydrogen bonds formation with water molecules (Basch and Stevens, 1990). In a solid phase, the zwitterionic structure of glycine molecules is also preserved and provides an additional interaction between the molecules (Drebushchak et al., 2005). Therefore, the anomaly in the heat capacity observed in β-glycine was attributed to a second-order ferroelectric-paraelectric phase transition (Drebushchak et al., 2005).
The detailed phenomenological analysis of this anomaly in terms of the compressible Ising model (Kiraci, 2021) confirmed that conclusion and also demonstrated that the critical exponents in “ferroelectric” (T < 252 K) and “paraelectric” (T > 252 K) phases are consistent with those predicted from the 3D Ising model and the 2D Potts model, respectively. However, as is shown in detail in the following section, the main features of ferroelectricity, such as domain structures and the ability to switch the polarization by an electric field, well persist in β-glycine at room temperature. Therefore, the observed anomaly at 252 K may be related not to the ferroelectric-paraelectric phase transition but, highly likely, to the transition between two ferroelectric phases. This question requires additional studies.
While piezoelectricity in γ- and β-phases originates from their non-centrosymmetric space groups, its attribution to the class of ferroelectrics can be done only experimentally (Lines and Glass, 1977). PFM can serve as a perfect tool to distinguish not only piezoelectric but also ferroelectric phases due to the domain structure visualization and the polarization switching in a point (Shvartsman et al., 2002; Kalinin et al., 2010; Soergel, 2011). A multitude of recent reports (Isakov et al., 2014; Seyedhosseini et al., 2014; Seyedhosseini et al., 2015; Bystrov et al., 2016; Hu et al., 2019; Vasileva et al., 2019) have proven that β-glycine is indeed ferroelectric with switchable polarization and diverse domain structures. The as-grown domain structure at nonpolar surfaces of β-glycine crystals, which are usually available for PFM study, consists of three types of domains (Vasileva et al., 2019): 1) stripe-like domains with flat charged domain walls (Figure 1E) appeared due to the variations of defects during the crystal growth; 2) quasi-periodic ensembles of needle-like domains (Figure 1F) appeared under the action of pyroelectric field occurred due to the cooling down of the evaporating droplet; and 3) irregular shaped domains with segmental step-like domain walls (Figure 1G).
Domains can also be created in β-glycine crystals by the application of an external electric field by the PFM tip (Figure 1H). Computer modeling based on density functional theory and molecular-dynamic simulations showed for β-glycine an average coercive field of about 1 V/nm (103 kV/mm) (Bystrov et al., 2015; Bystrov et al., 2016), which corresponds to 60 V applied to the PFM tip (Seyedhosseini et al., 2015). In principle, polarization switching in γ-glycine may also be possible, but it requires 4–8 times bigger electric fields (Heredia et al., 2012; Bystrov et al., 2015). Moreover, the maximum value of the electric field near the apex of the PFM tip with the applied voltage of 100 V is about 3 V/nm only (Seyedhosseini et al., 2015). Higher voltages lead to the electric breakdown of the crystal. Therefore, a single molecule switching in γ-glycine remains a theoretical possibility. Such a big difference in threshold fields for β- and γ-glycine is due to the mechanism of the polarization reversal. As computer modeling demonstrated, (Hu et al., 2019) the spontaneous polarization of β-glycine oriented along the polar crystallographic axis b stems from an ordered arrangement of the −NH3+ groups, whereas the polarization switching occurs via the change of a dihedral angle between the −NH3+ groups and the plane of the carboxyl (−COO−) groups (Figure 2A). At the same time, polarization reversal of γ-glycine requires the rotation of glycine molecules by 180° around an axis perpendicular to the polar c-axis (Hu et al., 2019) (Figure 2B), which makes the polarization reversal of γ-glycine much more difficult than that of β-phase.
FIGURE 2. Schemes of the polarization reversal in (A) β- and (B) γ-glycine. Green arrows show the direction of the spontaneous polarization. Molecular color scheme: carbon—grey, oxygen—red, nitrogen—blue. Hydrogen atoms are not shown for clarity.
The artificial needle-like domains created by the PFM tip propagate far outside the field area due to the kinks interaction (Vasileva et al., 2019), and their length depends on the applied voltage and pulse duration (Seyedhosseini et al., 2015). PFM tip movement during the voltage application allows the creation of tailored domain structures with different types of domain walls: neutral and charged head-to-head and tail-to-tail (Vasileva et al., 2019). Usually, tail-to-tail domain walls are flat and oriented normally to the polar axis, whereas head-to-head ones are rough and inclined (Vasileva et al., 2019). That allows suggesting that tail-to-tail domain walls possess higher conductivity, accelerating the screening of the depolarization fields created by the bound charges (Schröder et al., 2012; Schröder et al., 2014; Lu et al., 2015). This assumption is supported by the quantum chemical calculations that showed tail-to-tail walls are around seven times more stable than head-to-head ones (Bystrov et al., 2016).
As recently demonstrated (Seyedhosseini et al., 2015; Vasileva et al., 2019), the polarization switching and the evolution of the domain structures in β-glycine crystals are qualitatively similar to those found in uniaxial inorganic ferroelectrics. This observation opens up the possibility of applying the methods of domain engineering developed earlier for the creation of tailored micro- and nanodomain structures in inorganic ferroelectrics (Shur and Ye, 2008) for organic ones, which are highly demanded elements of biocompatible photonic devices. It is known that organic nonlinear optical materials have an advantage over their inorganic counterparts due to their big molecular polarizability and the ability to tune their properties by chemical modifications (Humar et al., 2017). In the case of crystalline organic ferroelectrics, such as β-glycine, the nonlinear optical properties can be additionally improved by the methods of domain engineering (Shur and Ye, 2008) by the creation of a periodical domain structure. Periodically poled β-glycine crystal can significantly enhance the effectiveness of the second harmonic generation and optical parametric oscillation, (Scrymgeour et al., 2014) thus allowing the usage of glycine in biocompatible photonic devices for quantitative tissue imaging and diseases diagnosis (Campagnola, 2011).
Stabilization of β-glycine crystals by Pt substrate (Seyedhosseini et al., 2014), strong piezoelectric activity exceeding that of classical perovskite piezoelectric ceramics (Heredia et al., 2012; Guerin et al., 2017), and nonlinear susceptibility much higher than that of γ-glycine (Gleeson et al., 2020), make β-glycine a promising functional material for various biomedical applications such as wearable and implantable sensors (Bishara et al., 2020; Hosseini et al., 2020; Li et al., 2020), energy harvesting, (Guerin et al., 2017) and nonlinear optical devices (Seyedhosseini et al., 2014; Gleeson et al., 2020). Recently, the combination of chitosan fibers with β-glycine allowed the creation of a new biodegradable sensor for sub-bandage pressure monitoring with quite high sensitivity in the range from 5 to 60 kPa (Hosseini et al., 2020). However, obtained spherulite-like β-glycine crystal structures demonstrate mainly shear piezoresponse that limits the applicability and sensitivity of the sensor. Another configuration of a sensor with β-glycine crystals confined in the cylindrical nanopores with the polar axis oriented along the pores provided pressure sensitivity as low as 1 Pa (Bishara et al., 2020). The application of inkjet printing suggests additional ways of designing the next-generation biosensors (Buanz and Gaisford, 2017; Slabov et al., 2019).
Though ferroelectricity in γ-glycine still remains a theoretical possibility, it also could be interesting for developing wearable and implantable bioelectronic devices even regardless of its slightly weaker piezoelectric response (Guerin et al., 2017). That is due to its phase stability and the pronounced positive therapeutic effect on cognitive functions and neural activity, as compared with α-glycine (Markel et al., 2011; Malakhin et al., 2012). As far as we know, the biological activity of the β-glycine was not studied yet. The combination of outstanding functional properties, inherent biocompatibility, stability, and direct therapeutic effect can endow γ-glycine-based sensors with additional multi-aspect functions. Recently, they have been grown as wafer-scale films in combination with PVDF (Yang et al., 2021) and demonstrated much higher sensitivity than PVDF polymer patches (Okosun et al., 2021).
Moreover, we suppose that humidity-controlled phase transitions (Isakov et al., 2014) allow, in principle, the creation of β-γ and β-α “chimera” crystals with the combination of two or even three polymorphic phases in one crystal. Such a combination of phases and thus functional properties may additionally expand possible applications of glycine and other amino acid crystals. At the same time, such devices would be able to disintegrate and resorb after completing their work cycle without any adverse long-term effects (Hosseini et al., 2021).
The idea of the control of functional properties via supramolecular packing (Guerin et al., 2017) can probably be also extended to β′, δ, ε, and ζ phases of glycine existing at high pressures (Boldyreva et al., 2005; Dawson et al., 2005; Goryainov et al., 2005; Goryainov et al., 2006). Thus, ε-glycine at about 4.3 GPa belongs to piezoelectric space group Pn, (Dawson et al., 2005) whereas δ-glycine belongs to the centrosymmetric space group P21/a (Dawson et al., 2005). Recent computer modeling (Guerra et al., 2020) revealed three more potentially piezoelectric phases of glycine: m, t, and o (space groups P21, P43, and P212121, respectively) that were not observed experimentally yet. These exotic glycine polymorphs are not stable under ambient conditions. However, the possibility of finding ways for their stabilization in further research (as it happened with metastable β-glycine) cannot be ruled out. The authors foresee that such a simple molecule as glycine will bring many more surprises in the future.
In this mini-review, we summarized the recent findings on ferroelectric properties and polarization switching of β- and γ-phases of glycine. We demonstrated that due to the transitions between polymorphic phases, the crystal habit is not a reliable method for choosing the appropriate crystals for the research, and additional phase confirmation by confocal or micro-Raman spectroscopy is highly recommended. Recent experimental and computer simulation studies of domain structures and polarization switching undoubtedly confirmed ferroelectricity in β-glycine, whereas a single molecule switching in γ-glycine still remains a theoretical possibility. Regardless of the metastability of the β-phase, its stabilization inside nanopores or microfluidic channels, as well as at the Pt substrates, allows considering its usage in various applications, whereas the outstanding nonlinear optical properties and the ability to create stable periodical domain structures make β-glycine a promising material for biocompatible photonic devices. We suppose that the combination of two or three polymorphic phases in one crystal is potentially achievable due to the humidity-controlled phase transitions, and searching the ways for effective stabilization of high-pressure polymorphic phases can open new ways for applications of crystals of this remarkable amino acid.
Conceptualization, AK; literature review, PZ, DV, and SV; writing—original draft preparation, PZ; writing—review and editing, SK and AK.
Portuguese Foundation for Science and Technology (UIDB/50011/2020, UIDP/50011/2020, LA/P/0006/2020, PTDC/CTM-CTM/4044/2020). This work was developed within the scope of the project CICECO-Aveiro Institute of Materials, UIDB/50011/2020, UIDP/50011/2020 and LA/P/0006/2020, financed by national funds through the FCT/MEC (PIDDAC). Part of this work was funded by national funds (OE), through FCT—Fundação para a Ciência e a Tecnologia, I.P., in the scope of the framework contract foreseen in the numbers 4, 5, and 6 of the article 23, of the Decree-Law 57/2016, of August 29, changed by Law 57/2017, of July 19. PZ and AK were supported by FCT through the project “Photomultiferro”- PTDC/CTM-CTM/4044/2020. SV was supported by Career-FIT received funding from the European Union’s Horizon 2020 research and innovation program under the Marie Skłodowska-Curie grant (agreement no. 713654).
The authors declare that the research was conducted in the absence of any commercial or financial relationships that could be construed as a potential conflict of interest.
All claims expressed in this article are solely those of the authors and do not necessarily represent those of their affiliated organizations, or those of the publisher, the editors and the reviewers. Any product that may be evaluated in this article, or claim that may be made by its manufacturer, is not guaranteed or endorsed by the publisher.
The authors are grateful for Alla S. Slautina and Vladimir Ya. Shur for their help in the manuscript preparation and fruitful discussions.
Altwegg, K., Balsiger, H., Bar-Nun, A., Berthelier, J. J., Bieler, A., Bochsler, P., et al. (2016). Prebiotic chemicals—Amino acid and phosphorus—In the coma of comet 67P/Churyumov-Gerasimenko. Sci. Adv. 2, e1600285. doi:10.1126/sciadv.1600285
Aree, T., Bürgi, H. B., Minkov, V. S., Boldyreva, E. V., Chernyshov, D., Törnroos, K. W., et al. (2013). Dynamics and thermodynamics of crystalline polymorphs. 2. β-Glycine, analysis of variable-temperature atomic displacement parameters. J. Phys. Chem. A 117, 8001–8009. doi:10.1021/jp404408h
Babić, D., and Babić, R. (2009). Complementary and alternative medicine in the treatment of schizophrenia. Psychiatr. Danub. 21, 376–381.
Bai, C., Wang, C., Zheng, T., and Hu, Q. (2018). Growth of β-glycine crystals promoted by standing surface acoustic waves (SSAWs). CrystEngComm 20, 1245–1251. doi:10.1039/C7CE02038D
Basch, H., and Stevens, W. J. (1990). The structure of glycine-water H- bonded complexes. Chem. Phys. Lett. 169, 275–280. doi:10.1016/0009-2614(90)85201-M
Bhamidi, V., Lee, S. H., He, G., Chow, P. S., Tan, R. B. H., Zukoski, C. F., et al. (2015). Antisolvent crystallization and polymorph screening of glycine in microfluidic channels using hydrodynamic focusing. Cryst. Growth Des. 15, 3299–3306. doi:10.1021/acs.cgd.5b00420
Bishara, H., Nagel, A., Levanon, M., and Berger, S. (2020). Amino acids nanocrystals for piezoelectric detection of ultra-low mechanical pressure. Mater. Sci. Eng. C 108, 110468. doi:10.1016/j.msec.2019.110468
Boldyreva, E. (2021). Glycine: The gift that keeps on giving. Isr. J. Chem. 61, 828–850. doi:10.1002/ijch.202100103
Boldyreva, E. V., Drebushchak, V. A., Drebushchak, T. N., Paukov, I. E., Kovalevskaya, Y. A., Shutova, E. S., et al. (2003). Polymorphism of glycine: Thermodynamic aspects. Part II. Polymorphic transitions. J. Therm. Anal. Calorim. 73, 419–428. doi:10.1023/A:1025457524874
Boldyreva, E. V., Drebushchak, V. A., Drebushchak, T. N., Paukov, I. E., Kovalevskaya, Y. A., Shutova, E. S., et al. (2003). Polymorphism of glycine: Thermodynamic aspects. Part I. Relative stability of the polymorphs. J. Therm. Anal. Calorim. 73, 409–418. doi:10.1023/A:1025405508035
Boldyreva, E. V., Ivashevskaya, S. N., Sowa, H., Ahsbahs, H., and Weber, H. P. (2005). Effect of hydrostatic pressure on the γ-polymorph of glycine. 1. A polymorphic transition into a new δ-form. Z. Krist. 220, 50–57. doi:10.1524/zkri.220.1.50.58886
Bordallo, H. N., Boldyreva, E. V., Buchsteiner, A., Koza, M. M., and Landsgesell, S. (2008). Structure-property relationships in the crystals of the smallest amino acid: An incoherent inelastic neutron scattering study of the glycine polymorphs. J. Phys. Chem. B 112, 8748–8759. doi:10.1021/jp8014723
Brog, J. P., Chanez, C. L., Crochet, A., and Fromm, K. M. (2013). Polymorphism, what it is and how to identify it: A systematic review. RSC Adv. 3, 16905. doi:10.1039/C3RA41559G
Buanz, A. B. M., and Gaisford, S. (2017). Formation of highly metastable β glycine by confinement in inkjet printed droplets. Cryst. Growth Des. 17, 1245–1250. doi:10.1021/acs.cgd.6b01633
Bystrov, V. S., Seyedhosseini, E., Bdikin, I., Kopyl, S., Neumayer, S. M., Coutinho, J., et al. (2015). Bioferroelectricity in nanostructured glycine and thymine: Molecular modeling and ferroelectric properties at the nanoscale. Ferroelectrics 475, 107–126. doi:10.1080/00150193.2015.995574
Bystrov, V. S., Seyedhosseini, E., Bdikin, I. K., Kopyl, S., Kholkin, A. L., Vasilev, S. G., et al. (2016). Glycine nanostructures and domains in beta-glycine: Computational modeling and PFM observations. Ferroelectrics 496, 28–45. doi:10.1080/00150193.2016.1157435
Bystrov, V. S., Seyedhosseini, E., Kopyl, S., Bdikin, I. K., and Kholkin, A. L. (2014). Piezoelectricity and ferroelectricity in biomaterials: Molecular modeling and piezoresponse force microscopy measurements. J. Appl. Phys. 116, 066803. doi:10.1063/1.4891443
Campagnola, P. (2011). Second harmonic generation imaging microscopy: Applications to diseases diagnostics. Anal. Chem. 83, 3224–3231. doi:10.1021/ac1032325
Dawson, A., Allan, D. R., Belmonte, S. A., Clark, S. J., David, W. I. F., McGregor, P. A., et al. (2005). Effect of high pressure on the crystal structures of polymorphs of glycine. Cryst. Growth Des. 5, 1415–1427. doi:10.1021/cg049716m
Dishon, S., Ushakov, A., Nuraeva, A., Ehre, D., Lahav, M., Shur, V., et al. (2020). Surface piezoelectricity and pyroelectricity in centrosymmetric materials: A case of α-glycine. Materials 13, 4663. doi:10.3390/ma13204663
Drebushchak, T. N., Boldyreva, E. V., and Shutova, E. S. (2002). β-Glycine. Acta Crystallogr. Sect. E Struct. Rep. Online E58, o634–o636. doi:10.1107/S160053680200836X
Drebushchak, V. A., Boldyreva, E. V., Kovalevskaya, Y. A., Paukov, I. E., and Drebushchak, T. N. (2005). Low-temperature heat capacity of β-glycine and a phase transition at 252 K. J. Therm. Anal. Calorim. 79, 65–70. doi:10.1007/s10973-004-0563-8
Elsila, J. E., Glavin, D. P., and Dworkin, J. P. (2009). Cometary glycine detected in samples returned by Stardust. Meteorit. Planet. Sci. 44, 1323–1330. doi:10.1111/j.1945-5100.2009.tb01224.x
Gerasimov, A. V., Zubaidullina, L. S., Safiullina, A. S., Nagrimanov, R. N., Ziganshin, M. A., Boldyrev, A. E., et al. (2022). Stabilization of metastable polymorphic form of glycine by glucose. AIP Conf. Proc. 2390, 030020. doi:10.1063/5.0069199
Gleeson, M., O’Dwyer, K., Guerin, S., Rice, D., Thompson, D., Tofail, S. A. M., et al. (2020). Quantitative polarization-resolved second-harmonic-generation microscopy of glycine microneedles. Adv. Mat. 32, 2002873. doi:10.1002/adma.202002873
Goryainov, S. V., Boldyreva, E. V., and Kolesnik, E. N. (2006). Raman observation of a new (ζ) polymorph of glycine? Chem. Phys. Lett. 419, 496–500. doi:10.1016/j.cplett.2005.11.123
Goryainov, S. V., Kolesnik, E. N., and Boldyreva, E. V. (2005). A reversible pressure-induced phase transition in β-glycine at 0.76GPa. Phys. B Condens. Matter 357, 340–347. doi:10.1016/j.physb.2004.11.089
Guerin, S., Stapleton, A., Chovan, D., Mouras, R., Gleeson, M., McKeown, C., et al. (2017). Control of piezoelectricity in amino acids by supramolecular packing. Nat. Mat. 17, 180–186. doi:10.1038/nmat5045
Guerra, D., Gómez, L. A., Restrepo, A., and David, J. (2020). New stable phases of glycine crystals. Chem. Phys. 530, 110645. doi:10.1016/j.chemphys.2019.110645
Gusev, E. I., Skvortsova, V. I., Dambinova, S. A., Raevskiy, K. S., Alekseev, A. A., Bashkatova, V. G., et al. (2000). Neuroprotective effects of glycine for therapy of acute ischaemic stroke. Cerebrovasc. Dis. 10, 49–60. doi:10.1159/000016025
Hamilton, B. D., Hillmyer, M. A., and Ward, M. D. (2008). Glycine polymorphism in nanoscale crystallization chambers. Cryst. Growth Des. 8, 3368–3375. doi:10.1021/cg800326a
Heredia, A., Meunier, V., Bdikin, I. K., Gracio, J., Balke, N., Jesse, S., et al. (2012). Nanoscale ferroelectricity in crystalline γ-glycine. Adv. Funct. Mat. 22, 2996–3003. doi:10.1002/adfm.201103011
Horn, J., Tolardo, E., Fissore, D., and Friess, W. (2018). Crystallizing amino acids as bulking agents in freeze-drying. Eur. J. Pharm. Biopharm. 132, 70–82. doi:10.1016/j.ejpb.2018.09.004
Hosseini, E. S., Dervin, S., Ganguly, P., and Dahiya, R. (2021). Biodegradable materials for sustainable health monitoring devices. ACS Appl. Bio Mat. 4, 163–194. doi:10.1021/acsabm.0c01139
Hosseini, E. S., Manjakkal, L., Shakthivel, D., and Dahiya, R. (2020). Glycine-chitosan-based flexible biodegradable piezoelectric pressure sensor. ACS Appl. Mat. Interfaces 12, 9008–9016. doi:10.1021/acsami.9b21052
Hu, P., Hu, S., Huang, Y., Reimers, J. R., Rappe, A. M., Li, Y., et al. (2019). Bioferroelectric properties of glycine crystals. J. Phys. Chem. Lett. 10, 1319–1324. doi:10.1021/acs.jpclett.8b03837
Humar, M., Kwok, S. J. J., Choi, M., Yetisen, A. K., Cho, S., Yun, S. H., et al. (2017). Toward biomaterial-based implantable photonic devices. Nanophotonics 6, 414–434. doi:10.1515/nanoph-2016-0003
Iitaka, Y. (1960). The crystal structure of β-glycine. Acta Cryst. 13, 35–45. doi:10.1107/S0365110X60000066
Iitaka, Y. (1961). The crystal structure of γ-glycine. Acta Crystallogr. 14, 1–10. doi:10.1107/S0365110X61000012
Ioppolo, S., Fedoseev, G., Chuang, K. J., Cuppen, H. M., Clements, A. R., Jin, M., et al. (2021). A non-energetic mechanism for glycine formation in the interstellar medium. Nat. Astron. 5, 197–205. doi:10.1038/s41550-020-01249-0
Isakov, D., Petukhova, D., Vasilev, S., Nuraeva, A., Khazamov, T., Seyedhosseini, E., et al. (2014). In situ observation of the humidity controlled polymorphic phase transformation in glycine microcrystals. Cryst. Growth Des. 14, 4138–4142. doi:10.1021/cg500747x
Jain, M., Nilsson, R., Sharma, S., Madhusudhan, N., Kitami, T., Souza, A. L., et al. (2012). Metabolite profiling identifies a key role for glycine in rapid cancer cell proliferation. Science 336, 1040–1044. doi:10.1126/science.1218595
Kalinin, S. V., Morozovska, A. N., Chen, L. Q., and Rodriguez, B. J. (2010). Local polarization dynamics in ferroelectric materials. Rep. Prog. Phys. 73, 056502. doi:10.1088/0034-4885/73/5/056502
Kholkin, A., Alikin, D., Shur, V., Dishon, S., Ehre, D., Lubomirsky, I., et al. (2021). Local piezoelectric properties of doped biomolecular crystals. Materials 14, 4922. doi:10.3390/ma14174922
Kim, D., Han, S. A., Kim, J. H., Lee, J. H., Kim, S. W., Lee, S. W., et al. (2020). Biomolecular piezoelectric materials: From amino acids to living tissues. Adv. Mat. 32, 1906989. doi:10.1002/adma.201906989
Kiraci, A. (2021). A phenomenological study on ferroelectric β-glycine. Ferroelectrics 572, 277–286. doi:10.1080/00150193.2020.1868888
Kuan, Y. J., Charnley, S. B., Huang, H. C., Tseng, W. L., and Kisiel, Z. (2003). Interstellar glycine. Astrophys. J. 593, 848–867. doi:10.1086/375637
Lee, I. S., Kim, K. T., Lee, A. Y., and Myerson, A. S. (2008). Concomitant crystallization of glycine on patterned substrates: The effect of pH on the polymorphic outcome. Cryst. Growth Des. 8, 108–113. doi:10.1021/cg700890m
Lemanov, V. V. (2000). Piezoelectric and pyroelectric properties of protein amino acids as basic materials of Soft State Physics. Ferroelectrics 238, 211–218. doi:10.1080/00150190008008786
Li, J., Long, Y., Yang, F., and Wang, X. (2020). Degradable piezoelectric biomaterials for wearable and implantable bioelectronics. Curr. Opin. Solid State Mat. Sci. 24, 100806. doi:10.1016/j.cossms.2020.100806
Lines, M. E., and Glass, A. M. (1977). Principles and applications of ferroelectrics and related materials. Oxford, UK: Clarendon Press, 680.
Lu, H., Li, T., Poddar, S., Goit, O., Lipatov, A., Sinitskii, A., et al. (2015). Statics and dynamics of ferroelectric domains in diisopropylammonium bromide. Adv. Mat. 27, 7832–7838. doi:10.1002/adma.201504019
Maiti, S., Karan, S. K., Kim, J. K., and Khatua, B. B. (2019). Nature driven bio-piezoelectric/triboelectric nanogenerator as next-generation green energy harvester for smart and pollution free society. Adv. Energy Mat. 9, 1803027. doi:10.1002/aenm.201803027
Malakhin, I. A., Achkasov, A. F., Ratushnyak, A. S., Zapara, T. A., Markel, A. L., Boldyreva, E. V., et al. (2012). Different effects of α- and γ-glycine on the aberrant activity of pyramidal neurons of hippocampal slices. Dokl. Biol. Sci. 444, 157–161. doi:10.1134/s0012496612030179
Markel, A. L., Achkasov, A. F., Alekhina, T. A., Prokudina, O. I., Ryazanova, M. A., Ukolova, T. N., et al. (2011). Effects of the alpha- and gamma-polymorphs of glycine on the behavior of catalepsy prone rats. Pharmacol. Biochem. Behav. 98, 234–240. doi:10.1016/j.pbb.2010.12.025
Meirzadeh, E., Weissbuch, I., Ehre, D., Lahav, M., and Lubomirsky, I. (2018). Polar imperfections in amino acid crystals: design, structure, and emerging functionalities. Acc. Chem. Res. 51, 1238–1248. doi:10.1021/acs.accounts.8b00054
Okosun, F., Guerin, S., Celikin, M., and Pakrashi, V. (2021). Flexible amino acid-based energy harvesting for structural health monitoring of water pipes. Cell Rep. Phys. Sci. 2, 100434. doi:10.1016/j.xcrp.2021.100434
Palla, P., Petrongolo, C., and Tomasi, J. (1980). Internal rotation potential energy for the glycine molecule in its zwitterionic and neutral forms: a comparison among several methods. J. Phys. Chem. 84, 435–442. doi:10.1021/j100441a019
Piperno, S., Mirzadeh, E., Mishuk, E., Ehre, D., Cohen, S., Eisenstein, M., et al. (2013). Water-induced pyroelectricity from nonpolar crystals of amino acids. Angew. Chem. Int. Ed. 52, 6513–6516. doi:10.1002/anie.201301836
Poornachary, S. K., Chow, P. S., and Tan, R. B. H. (2008). Influence of solution speciation of impurities on polymorphic nucleation in glycine. Cryst. Growth Des. 8, 179–185. doi:10.1021/cg060570w
Schröder, M., Chen, X., Haußmann, A., Thiessen, A., Poppe, J., Bonnell, D. A., et al. (2014). Nanoscale and macroscopic electrical ac transport along conductive domain walls in lithium niobate single crystals. Mat. Res. Express 1, 035012. doi:10.1088/2053-1591/1/3/035012
Schröder, M., Haußmann, A., Thiessen, A., Soergel, E., Woike, T., Eng, L. M., et al. (2012). Conducting domain walls in lithium niobate single crystals. Adv. Funct. Mat. 22, 3936–3944. doi:10.1002/adfm.201201174
Scrymgeour, D. A. (2014). “Applications of domain engineering in ferroelectrics for photonic applications,” in Ferroelectric crystals for photonic applications. Editors P. Ferraro, S. Grilli, and P. De Natale (Berlin, Heidelberg: Springer), 91, 385–399. Springer Series in Materials Science. doi:10.1007/978-3-642-41086-4_14
Seyedhosseini, E., Bdikin, I., Ivanov, M., Vasileva, D., Kudryavtsev, A., Rodriguez, B. J., et al. (2015). Tip-induced domain structures and polarization switching in ferroelectric amino acid glycine. J. Appl. Phys. 118, 072008. doi:10.1063/1.4927807
Seyedhosseini, E., Ivanov, M., Bystrov, V., Bdikin, I., Zelenovskiy, P., VYa, Shur, et al. (2014). Growth and nonlinear optical properties of β-glycine crystals grown on Pt substrates. Cryst. Growth Des. 14, 2831–2837. doi:10.1021/cg500111a
Seyedhosseini, E., Romanyuk, K., Vasileva, D., Vasilev, S., Nuraeva, A., Zelenovskiy, P., et al. (2017). Self-assembly of organic ferroelectrics by evaporative dewetting: aA case of β-glycine. ACS Appl. Mat. Interfaces 9, 20029–20037. doi:10.1021/acsami.7b02952
Shur, V. Y. (2008). “21 - Nano- and micro-domain engineering in normal and relaxor ferroelectrics,” in Handbook of advanced dielectric, piezoelectric and ferroelectric materials –synthesis, properties and applications. Editor Z. G. Ye (Cambridge, UK: Woodhead Publishing Ltd), 622–669. doi:10.1533/9781845694005.5.622
Shvartsman, V. V., AYu, Emelyanov, Kholkin, A. L., and Safari, A. (2002). Local hysteresis and grain size effect in Pb(Mg1/3Nb2/3)O3–PbTiO3 thin films. Appl. Phys. Lett. 81, 117–119. doi:10.1063/1.1490150
Slabov, V., Vasileva, D., Keller, K., Vasilev, S., Zelenovskiy, P., Kopyl, S., et al. (2019). Controlled growth of stable β-glycine via inkjet printing. Cryst. Growth Des. 19, 3869–3875. doi:10.1021/acs.cgd.9b00308
Soergel, E. (2011). Piezoresponse force microscopy (PFM). J. Phys. D. Appl. Phys. 44, 464003. doi:10.1088/0022-3727/44/46/464003
Surovtsev, N. V., Malinovsky, V. K., and Boldyreva, E. V. (2011). Raman study of low-frequency modes in three glycine polymorphs. J. Chem. Phys. 134, 045102. doi:10.1063/1.3524342
Tasnim, T., Goh, A., Gowayed, O., Hu, C. T., Chen, T. Y., Aber, J. E., et al. (2018). Dendritic growth of glycine from nonphotochemical laser-induced nucleation of supersaturated aqueous solutions in agarose gels. Cryst. Growth Des. 18, 5927–5933. doi:10.1021/acs.cgd.8b00688
Tayi, A. S., Kaeser, A., Matsumoto, M., Aida, T., and Stupp, S. I. (2015). Supramolecular ferroelectrics. Nat. Chem. 7, 281–294. doi:10.1038/nchem.2206
Tofail, S. A. M., and Bauer, J. (2016). Electrically polarized biomaterials. Adv. Mat. 28, 5470–5484. doi:10.1002/adma.201505403
Vasileva, D., Vasilev, S., Kholkin, A. L., and VYa, Shur (2019). Domain diversity and polarization switching in amino acid β-glycine. Materials 12, 1223. doi:10.3390/ma12081223
Wesson, P. S. (2010). Panspermia, past and present: astrophysical and biophysical conditions for the dissemination of life in space. Space Sci. Rev. 156, 239–252. doi:10.1007/s11214-010-9671-x
Xu, Q., Gao, X., Zhao, S., Liu, Y. N., Zhang, D., Zhou, K., et al. (2021). Construction of bio-piezoelectric platforms: From structures and synthesis to applications. Adv. Mat. 33, 2008452. doi:10.1002/adma.202008452
Yang, F., Li, J., Long, Y., Zhang, Z., Wang, L., Sui, J., et al. (2021). Wafer-scale heterostructured piezoelectric bio-organic thin films. Science 373, 337–342. doi:10.1126/science.abf2155
Keywords: organic piezoelectrics, ferroelectrics, domain structure, amino acids, glycine
Citation: Zelenovskii PS, Vasileva DS, Vasilev SG, Kopyl S and Kholkin A (2022) Ferroelectricity in glycine: A mini-review. Front. Mater. 9:918890. doi: 10.3389/fmats.2022.918890
Received: 12 April 2022; Accepted: 28 June 2022;
Published: 22 July 2022.
Edited by:
Vadim G. Kessler, Swedish University of Agricultural Sciences, SwedenReviewed by:
Dhiren Kumar Pradhan, Geophysical Laboratory (CIS), United StatesCopyright © 2022 Zelenovskii, Vasileva, Vasilev, Kopyl and Kholkin. This is an open-access article distributed under the terms of the Creative Commons Attribution License (CC BY). The use, distribution or reproduction in other forums is permitted, provided the original author(s) and the copyright owner(s) are credited and that the original publication in this journal is cited, in accordance with accepted academic practice. No use, distribution or reproduction is permitted which does not comply with these terms.
*Correspondence: Pavel S. Zelenovskii, emVsZW5vdnNraWlAdWEucHQ=; Andrei Kholkin, a2hvbGtpbkB1YS5wdA==
Disclaimer: All claims expressed in this article are solely those of the authors and do not necessarily represent those of their affiliated organizations, or those of the publisher, the editors and the reviewers. Any product that may be evaluated in this article or claim that may be made by its manufacturer is not guaranteed or endorsed by the publisher.
Research integrity at Frontiers
Learn more about the work of our research integrity team to safeguard the quality of each article we publish.