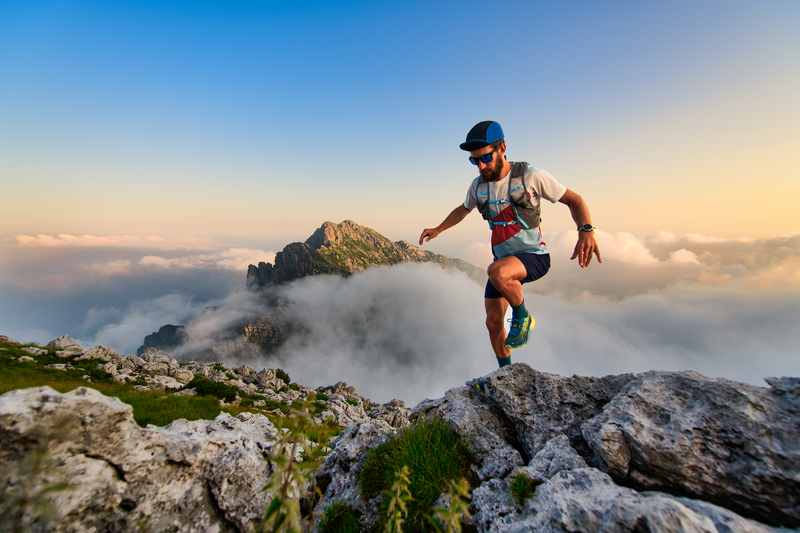
95% of researchers rate our articles as excellent or good
Learn more about the work of our research integrity team to safeguard the quality of each article we publish.
Find out more
REVIEW article
Front. Mater. , 08 June 2022
Sec. Biomaterials
Volume 9 - 2022 | https://doi.org/10.3389/fmats.2022.912713
The incidence of osteoporotic vertebral compression fractures (OVCFs) increases gradually with age, resulting in different degrees of pain for patients, even possible neurological damage and deformity, which can seriously affect their quality of life. Vertebral augmentation plays an important role in the surgical treatment of OVCFs. As the most widely used bone cement material, polymethyl methacrylate (PMMA) offers inherent advantages, such as injectability, ease of handling, and cost-effectiveness. However, with its application in the clinic, some disadvantages have been found, including cytotoxicity, high polymerization temperature, high elastic modulus, and high compressive strength. To improve the mechanical properties and the biological performance of conventional PMMA bone cement, several studies have modified it by adding bioceramics, bioglass, polymer materials, nanomaterials, and other materials, which have exhibited some advantages. In addition, other alternative materials, such as calcium phosphate, calcium sulfate, and calcium silicate cements—including their modifications—have also been explored. In this review, we examined the existing research on the side-effects of conventional PMMA bone cement, modified PMMA bone cement, and other alternative materials designed to improve properties in OVCFs. An overview of various modified bone cements can help further scientific research and clinical applications.
Osteoporosis is a systemic skeletal disease with low bone mass and microarchitectural deterioration of the bone tissue (Compston et al., 2019). Osteoporotic vertebral compression fractures (OVCFs) comprise the most common fracture associated with skeletal fragility and cause substantial morbidities, mortality, negatively impacting health-care costs (Kado et al., 2003; Burge et al., 2007; Si et al., 2015; Schousboe, 2016). The prevalence of osteoporosis among postmenopausal women is 32.1%, and among men 50 years or older is 6.9% (Linhong Wang et al., 2021). Besides, the prevalence of vertebral fractures among those aged 80 years or older, defined on the basis of radiographic findings, is 38.1% among women and 36.0% among men (Linhong Wang et al., 2021). Since OVCFs are often caused by low-energy injuries, less than half of them are clinically recognized (Ensrud and Schousboe, 2011; Ensrud et al., 2016; Linhong Wang et al., 2021). Treatment for OVCFs includes nonoperative treatment and surgical treatment, with percutaneous vertebroplasty (PVP) or percutaneous kyphoplasty (PKP) being preferred among surgical patients due to their advanced age (Buchbinder et al., 2009; Kallmes et al., 2009; Clark et al., 2016).
Polymethyl methacrylate (PMMA)—which is a polymer belonging to the category of acrylic resins—is currently the most widely used bone cement material in PVP or PKP procedures in the clinic (Bistolfi et al., 2019). PMMA bone cement is composed of two parts—that is, the solid phase is composed of methyl methacrylate (MMA) polymer particles, benzoyl peroxide (BPO), radiopaque reagents (such as tantalum powder, tungsten, barium sulfate, or zirconia dioxide), and the liquid phase includes MMA monomers, N,N-dimethyl-4-methylaniline (DMT) as an activator, and a small volume of inhibitors (usually hydroquinone). The polymerization reaction is rapid, and exothermic, and can be completed within 10–15 min (Milner, 2004). Moreover, there is a mechanical interlock between the bone and cement so that immediate fixation can be achieved (Mengran Wang et al., 2021). PMMA bone cement offers inherent advantages such as injectability, ease of handling, high biomechanical strength, and cost-effectiveness (Yimin et al., 2013). Consequently, it is widely used in the stability reconstruction of patients with OVCFs and bone metastases (Liu et al., 2010; Li et al., 2014; Qi et al., 2018). In addition, it can also be used as a good local drug delivery system to deliver antibiotics or antitumor drugs—such as cisplatin or methotrexate—to the local treatment site (Marks et al., 1976; Yan and Gemeinhart, 2005; Draenert and Draenert, 2008).
However, some deficiencies have been found in its clinical application. Given its shortcomings, researchers have made some effort to research and develop new bone cement materials. In this review, we examine the existing research on the side-effects of conventional PMMA bone cement, modified PMMA bone cement, and calcium-based alternative materials. We focus on the potential clinical application value in OVCFs, and summarize the modified methods and properties improvements, to provide reference for further scientific research and clinical applications.
First, in terms of cytotoxicity, studies have found that there are local toxic reactions, especially at the start of processing, some components having poor histocompatibility, which can cause serious local tissue changes (Kalteis et al., 2004). Second, several PMMA bone cements produce a strongly exothermic reaction when curing, studies having shown that the core temperature can be as high as 100°C or more during the curing process, while normal bone tissue can suffer necrosis at 50°C (Eriksson et al., 1984; Maffezzoli et al., 1997; Gundapaneni and Goswami, 2014). Third, previous studies have shown that the stiffness of bone cement material is a risk factor for adjacent vertebral fractures after vertebroplasty (Nouda et al., 2009). Most acrylic cements have a high elastic modulus (1700–3,700 MPa) and compressive strength (85–114 MPa), while the elastic modulus (10–900 MPa) and compressive strength (0.1–15 MPa) of cancellous bone are much smaller than those bone cements. This pronounced difference can easily lead to stress concentrations in unreinforced vertebral bodies, causing new fractures of the adjacent vertebral body (Helgason et al., 2008; Nazarian et al., 2008) (Figure 1). Fourth, the incidence of PMMA bone cement leakage during vertebroplasty is reported to be 17–88% (Chu et al., 2013; Alhashash et al., 2019; Li et al., 2021). Although most cases are asymptomatic, some research reports that bone cement leakage can cause severe complications such as a symptomatic pulmonary embolism (with an incidence of approximately 5.5%) or spinal cord injury (Chen et al., 2006; Duran et al., 2007; Kim et al., 2009). Even with effectively assistive procedure for injecting cement in percutaneous vertebroplasty, routine postoperative reviews revealed that 12% had minimal cement leakage (Chu et al., 2013).
FIGURE 1. New fracture of the adjacent vertebral body. This is an 89-year-old female with lower back pain and limited mobility. She developed these symptoms after accidentally falling 1 day ago. The pain can be relieved with bed rest and is aggravated when her position changes. The preoperative magnetic resonance imaging (A) suggests a fresh fracture of the L3 vertebral body. The patient underwent L2 vertebroplasty 3 years ago for an osteoporotic vertebral compression fracture. She underwent L3 vertebroplasty on this occasion because of a compression fracture of the adjacent vertebral body. Her pain is relieved after surgery, and postoperative fluoroscopy with anteroposterior (B) and lateral (C) positions indicate satisfactory bone cement filling.
To improve the mechanical properties and biological performance of existing PMMA bone cement, many studies have modified it by adding bioceramics, bioglass, polymer materials, nanomaterials, and other materials, and demonstrated some advantages (Tsukeoka et al., 2006; Boger et al., 2008; Abd Samad et al., 2011; Dall'Oca et al., 2014; Khandaker et al., 2014; Robo et al., 2018a; Robo et al., 2018b; De Mori et al., 2019; Phakatkar et al., 2020; Zapata et al., 2020; Zhu et al., 2020; Yingjie Wang et al., 2021) (Table 1) (Figure 2).
FIGURE 2. Modifications for PMMA bone cement. β-TCP, β-tricalcium phosphate; MgAl-LDH, MgAl-layered double hydroxide; HA, hydroxyapatite; γ-MPS, gamma-methacryloxypropyltrimethoxysilane.
The porous acrylic bone cement composed of PMMA and β-tricalcium phosphate (β-TCP) has a faster setting time (approximately 9 min), lower polymerization temperature (44°C), lower compressive strength (50 MPa), and lower flexural modulus (900 MPa) (Dall'Oca et al., 2014). Moreover, due to its microporous structure, it allows osteocytes and blood vessels to colonize on the surface of the bone space left after cement reabsorption, and also allows osteoblasts to uniformly rebuild bone tissue at the bone-cement interface (Dall'Oca et al., 2014). Bioglass can form strong chemical bonds with bone tissue and has good biocompatibility and osteogenic induction activity (Abd Samad et al., 2011). The peak temperature (39.1–47.2°C) during polymerization can be substantially reduced by adding glass-ceramic to the PMMA bone cement, an obvious apatite coating having been found on the surface of this modified bone cement (Abd Samad et al., 2011).
Chitosan, a linear semi-crystalline polysaccharide, is a direct derivative of chitin, which is the second most abundant natural polymer after cellulose (Friedman and Juneja, 2010). Structurally, it is the main component of the bone extracellular matrix (i.e., glycosaminoglycans), and due to its excellent biological properties, it can achieve more stable binding by improving the interlocking between bone and cement (Albanna et al., 2013). Studies have shown that adding 5–10% chitosan can improve the mechanical properties of bone cement and reduce the polymerization temperature (De Mori et al., 2019). Moreover, compared with acrylic bone cement without chitosan, the activity of osteoblasts is considerably increased (Khandaker et al., 2014). However, with an increase in the percentage of added chitosan, the mechanical properties of the modified acrylic bone cement decrease to varying degrees (Tunney et al., 2008).
Some researchers have surmised that the reason for osteoporotic fractures around PMMA bone cement is the higher stiffness of the filling material compared to the surrounding bone (Baroud et al., 2006; Pflugmacher et al., 2006; Chen et al., 2020). For example, the stiffness of the vertebral body increased by approximately 17% after the injection of conventional PMMA bone cement, resulting in high-stress concentrations in the adjacent endplates (Holub et al., 2015). In vitro model studies have found that low-modulus PMMA could better preserve the failure strength of augmented functional spinal units, prompting the concept of “low-modulus bone cement” (Boger et al., 2007). This property can be achieved by adding degradable polymer material in vivo to increase the porosity of the PMMA bone cement matrix, thereby reducing its modulus. In the work of Robo et al. (Robo et al., 2018b), a commercial low-modulus bone cement (Resilience) containing—in addition to the same components as conventional PMMA bone cement—polyamine acids (12.9 wt%), was used. By dissolving the cement in an aqueous solution, its elastic modulus was considerably reduced, and reached 1,067 ± 69 MPa after 4 weeks, only slightly higher than the highest value of healthy vertebral cancellous bone (Robo et al., 2018b; Ohman-Magi et al., 2021).
In another study by Robo et al., low-modulus bone cement was obtained by adding linoleic acid (LA) to conventional PMMA, an animal model showing that adding a small amount of LA to PMMA bone cement did not cause any additional cytotoxic effects on the tissue surrounding the implant site. Moreover, the modified bone cement did not differ significantly from conventional PMMA cement in terms of tissue response at the bone-implant interface, biocompatibility, and bone healing (Robo et al., 2018a). However, the study found that the amount of methyl methacrylate (MMA) released reached a peak after 24 h, which was significantly higher than that of traditional PMMA bone cement (Robo et al., 2018a). In addition, in another study of low-modulus bone cement (VS-LA) obtained by adding 12 wt% LA to commercial bone cement (V-Steady), the early release of MMA monomers from VS-LA was also observed, the peak of which was much higher than the monomer level released by VS over the same period (Robo et al., 2021). In other work, PMMA was mixed with sodium hyaluronate solutions of different volume fractions to prepare porous low-modulus bone cement (Boger et al., 2008). Its compressive strength and modulus were closer to human cancellous bone, and the addition of sodium hyaluronate did not dramatically change its injectability, viscosity, and MMA monomer release (Boger et al., 2008).
Graphene oxide (GO) is a nanomaterial composed of oxidized graphene (Bhattacharya et al., 2016). It is biocompatible, and the addition of 0.1 wt% GO or G powder to the PMMA matrix can improve the mechanical properties of the material under static and fatigue conditions (Paz et al., 2019). In addition, the existence of functional groups on the surface of GO powder can improve the dispersibility between GO and the PMMA matrix and enhance the interface bonding between them without obvious cytotoxicity (Paz et al., 2019). In other work, the maximum temperature during the polymerization process was shown to be reduced by approximately 19%, and the setting time was prolonged, which was related to the inhibitory and delaying effects of GO on the polymerization reaction (Zapata et al., 2020). At the same time, it was found that the acrylic bone cement with 0.3 wt% GO added had good antibacterial properties (Zapata et al., 2020).
In a study by Yingjie Wang et al. (2021), 15 wt% MgAl-layered double hydroxide (MgAl-LDH) microflakes were added to PMMA, and a bioactive PMMA-LDH composite bone cement was prepared. Due to the large size of LDH microplates, a certain number of pores are formed on the surface of the PMMA, which is conducive to bone formation. Moreover, in terms of mechanical properties, the compressive strength of the modified cement was 16.87% lower than that of PMMA, and its curing time was approximately 9.8 min. Due to the excellent thermal insulation properties of LDH, the maximum temperature of the polymerization reaction could be reduced by approximately 7°C compared with conventional PMMA. In addition, the modified bone cement exhibited good biocompatibility and promoted the differentiation of human bone marrow mesenchymal cells into the bone by moderately releasing magnesium ions, improving the interface bonding between the bone and the bone cement.
PMMA bone cement is one of the earliest and most widely used bone defect repair materials, and there has been much research on modifications based on it (Filippiadis et al., 2017). However, adding just a single material tends to focus on one aspect of modification, the effect being too one-dimensional, while by adding multiple materials simultaneously the overall performance can be improved. Tsukeoka et al. (Tsukeoka et al., 2006) found that by adding an appropriate amount of gamma-methacryloxypropyltrimethoxysilane (γ-MPS) and calcium acetate, after the modified PMMA bone cement had been implanted, it reacted with the surrounding body fluid to form an apatite layer, thereby enhancing the binding ability of the bone cement and the bone. Moreover, the temperature during polymerization was considerably reduced (to approximately 51°C), the curing time being approximately 18 min (Tsukeoka et al., 2006). However, it inevitably led to a decrease in compressive strength (Tsukeoka et al., 2006). Mineralized collagen (MC)—composed of organic type I collagen and nano-hydroxyapatite, which mimics the chemical composition and microstructure of natural bone matrix—can also be used for the modification of PMMA (Xu et al., 2016). MC-PMMA bone cement has the effect of promoting the proliferation and differentiation of bone marrow stromal cells (BMSCs) (Xu et al., 2016). By implanting MC-PMMA into a rabbit vertebral animal model, Zhu et al. (Zhu et al., 2020) found that after 4 weeks, there was obvious bone repair around the MC-PMMA, and after 8 weeks, the MC had been degraded, and the effects of remodeling lacunae and osteoblastic infiltration were evident. After 12 weeks, the borders and some interior areas of the MC were almost completely replaced by new bone. The research team conducted a small-scale clinical study and confirmed that MC-PMMA bone cement had indeed achieved good long-term efficacy (Zhu et al., 2020). Recent studies have found that adding hydroxyapatite (HA) nanofibers and two-dimensional magnesium phosphate (MGP) nanosheets to PMMA bone cement can improve the compressive strength of the bone cement, and that adding MGP nanosheets to PMMA can induce the formation of apatite on the material surface, which can improve the biological activity of the material (Phakatkar et al., 2020). Similarly, it has been established that adding HA nanofibers and MGP nanosheets to PMMA can improve cell viability (Phakatkar et al., 2020).
In addition to PMMA bone cement and other modifications based on it, many scholars have explored alternative materials, such as calcium phosphate cement (CPC), calcium sulfate cement (CSC), calcium silicate cement (CSiC), and other materials (Nilsson et al., 2004; Huan and Chang, 2007; Yu et al., 2013; El-Fiqi et al., 2015; Liu et al., 2015; Gong et al., 2016; Maenz et al., 2017; Shie et al., 2017; Xu et al., 2018; Yang et al., 2018; Zhang et al., 2018; Lu et al., 2019; Luo et al., 2019; de Lacerda Schickert et al., 2020; Miao et al., 2020; Wu et al., 2020; Yang et al., 2020; Ding et al., 2021) (Table 2).
Calcium phosphate cement is a paste-like mixture that can be arbitrarily shaped after mixing calcium phosphate powder (solid phase) with low-concentration phosphoric acid or phosphate solution (liquid phase) in a certain proportion (Canal and Ginebra, 2011). Depending on the Ca/P ratio, solubility, and pH of the initial calcium-phosphorus compound, two products—namely, hydroxyapatite or brushite—are finally formed (Ginebra et al., 2010). Although the composition formula is diverse, it can usually be divided into apatite bone cement and brushite bone cement based on the final product (Bohner et al., 2000). Moreover, because the structure is similar to natural bone, it has good biocompatibility compared with acrylic bone cement, having the characteristics of curing at low temperature and no exothermic effect during curing, thereby avoiding thermal damage to surrounding bone cells. This allows it to be used as a carrier to deliver drugs without destroying their activity (Ginebra et al., 2010; Ginebra et al., 2012).
Brushite bone cement has the characteristics of fast degradation, low strength—its highest compressive strength being 41.8–52 MPa—fast setting, and poor injectability (Hofmann et al., 2009; Engstrand et al., 2014). The main reason for its fast degradation rate is that it has greater solubility at the physiological pH, which enables degradation by passive dissolution in addition to active resorption. The degradation of brushite-based cement is also related to its disintegration (Grossardt et al., 2010). The degradation rate of brushite bone cement is most rapid at the initial stage of implantation, gradually stabilizing after a few weeks (Bohner et al., 2003). However, its injectability is poor. The solidification time of dicalcium phosphate (DCP) bone cement is about 7 min. Subsequently, the dehydrated DCP cement rapidly solidifies within 2–3 min, and cannot be discharged from the syringe after 2 min, restricting its application in surgical operations being unable to adequately fill irregular fractures or bone defects (Huan and Chang, 2009; Alshemary et al., 2021). Apatite bone cement is superior in compressive strength, tensile strength, and shear strength compared with brushite bone cement, reaching up to 80, 3.5, and 9.8 MPa, respectively (Charriere et al., 2001; Gbureck et al., 2005). However, its injectability is still not completely satisfactory, and the curing time cannot reach the clinically recommended 8–15 min (Alshemary et al., 2021). Using commercial apatite bone cement (HydroSet) to fill the bone defects of the femoral condyle of rabbits, the residual bone cement still amounted to 91.9 ± 4.9% after 26 weeks (An et al., 2016). In a clinical study, PKP was performed on 26 vertebral bodies in 21 patients with Calcibon apatite bone cement, the average bone cement absorption rate at a 10-year follow-up being approximately 22.9%, with just two vertebral bodies having absorption rates above 50% (Maestretti et al., 2014).
Given the insufficient performance of CPC, the modification is mainly carried out in the following ways.
1) Added fiber polymers—such as PLGA (poly (l-lactide-co-glycolide) acid)—can be used as degradable fibers to improve the performance of CPC. Studies have shown that PLGA fibers can promote new bone formation and reinforce the mechanical properties of CPC (Maenz et al., 2017).
2) Another study added low-degradable polyvinyl alcohol (PVA) fibers to α-tricalcium phosphate, which substantially improved the tensile strength of the bone cement (by approximately 9.2 MPa) (Luo et al., 2019). In addition to PVA, by adding carboxymethyl cellulose (CMC), the final setting time of CPC could be increased to more than 10 min, improving its injection performance and inherent brittleness (de Lacerda Schickert et al., 2020). At the same time, it was found that CMC acted as a viscosifying agent, which could keep the new bone cement within the confines of the bone defect without leaking into the surrounding bone (de Lacerda Schickert et al., 2020).
3) Lu et al. added gelatinized starch to calcium phosphate granules to form calcium phosphate-based nanocomposite (CPN) cement, which was found to be moderate in strength, injectable, and degradable in a vertebroplasty model, benefiting from its semi-solid and high viscosity characteristics, significantly reducing the extravasation of bone cement (Lu et al., 2019).
4) Bioactive glass (BG) is known for its unique binding activity and ability to promote bone growth (Jones, 2013). Studies have shown that after adding BG or mesoporous bioactive glass nanoparticles (mBGn) to CPC, the cement formulation has good biocompatibility, improved compressive strength (20 wt% CPC-BG compressive strength can reach 40 MPa, 10% mBGn/CPC can reach 26 MPa) and injectability. Moreover, it was found that its degradation rate and osteoinductive ability were significantly improved (Yu et al., 2013; El-Fiqi et al., 2015) (Figure 3).
FIGURE 3. Disadvantages of CPC and its modifications. CPC, calcium phosphate cement; CMC, carboxymethyl cellulose; PLGA, poly (l-lactide-co-glycolide) acid.
As a commonly used implant material, CSC is mainly composed of calcium sulfate hemihydrate (CSH)—which is finally converted into calcium sulfate dihydrate (CSD) through rehydration and recrystallization (Thomas and Puleo, 2009). It can be completely absorbed, has good compatibility with the surrounding physiological environment, and has better injectability than CPC (Dadkhah et al., 2017). The compressive strength of pure CSC is approximately 15.8–37.9 MPa, its final setting time being approximately 20–37 min (Dadkhah et al., 2017; Hall et al., 2021). By culturing BMSCs on CPC, the results have shown that after 2 days of culture, BMSCs could not only attach and spread on the bone cement but also bind well to the material surface through many prosthetic feet, proving that it could provide good cell adhesion, migration, and proliferation as a scaffold (Chen et al., 2013). At the same time, in an animal model of filling the mandibular defects of rabbits using CPC, it was established that CSH exhibited good bone repairability, making it beneficial in the repair of bone defects (Chen et al., 2013). However, its resistance to washout was poor, making it difficult to maintain its original form when soaked in solution (Zhang et al., 2018). Moreover, its degradation rate was rapid. Based on the literature, when calcium sulfate samples were soaked in Ringer’s solution, the degradation rate was found to be as high as 90% after 1 week (Huan and Chang, 2007). In a retrospective study that followed 28 patients who used CSC bone cement in vertebroplasty procedures, it was found that the CPC took approximately 43 days to completely resorb in the body, the rapid resorption of CSC bone cement potentially leading to the occupation of bone defects by vascular fibrous tissue and affecting new bone formation which could be associated with difficulties such as nonunion and “vacuum disc” complications (Bu et al., 2015). In addition, the fast degradation rate limits its role in weight-bearing. Consequently, it is used mainly for filling bone defects in non-weight-bearing areas or as a drug carrier (Luo et al., 2016).
The modification of CPC includes the following aspects:
1) After adding silk fibroin (SF) to CSH, the compressive strength can reach up to approximately 32.7 MPa under the optimal ratio, and the washout resistance of CPC is improved (Zhang et al., 2018).
2) In another study, the researchers prepared a bone cement composed of tricalcium silicate (C3S), glucono-delta-lactone (GDL), and CSD, which had a self-setting time of less than 15 min, the highest compressive strength after 1 h being approximately 5 MPa, and its self-degradation rate being up to approximately 58% after 12 weeks. Moreover, its degradation rate could be adjusted by adjusting the content of calcium sulfate (Ding et al., 2021). At the same time, it exhibited good osteogenic activity. In vitro cell tests demonstrated that it could effectively promote the proliferation and differentiation of bone marrow mesenchymal cells (Ding et al., 2021) (Figure 4).
FIGURE 4. Disadvantages of CSC and CSiC and their modifications. CSC, calcium sulfate cement; CSiC, calcium silicate cement; C3S, tricalcium silicate; GDL, glucono-delta-lactone.
CSiC—a bioactive material containing silicon—comprises primarily mineral trioxide aggregate (MTA) (Santiago et al., 2021), which is composed of C3S, dicalcium silicate (C2S), tricalcium aluminate (TCA), tetracalcium aluminoferrite, and uses bismuth oxide as a radiopaque additive (Roberts et al., 2008). When all components are mixed with water, they can form a paste that can be implanted in place and shaped. Several previous studies have shown that when CSiC is exposed to a simulated body fluid (SBF) solution, it forms calcium phosphate and apatite precipitate on its surface by releasing silicon ions, which exhibits good mineralization induction of bone-like apatite (Zhao et al., 2005). In a femoral defect model study in rabbits, it was found that C3S could be well bound by bone tissue, with new mineralized bone tissue being deposited on the binding surface without any signs of fibrosis and inflammation (Lin et al., 2021). Its compressive strength is related to the setting pressure, up to approximately 70 MPa (Nekoofar et al., 2007). It has a moderate degradation rate and lower heat release during hydration than CPC and has a certain promotion effect on cell proliferation (Zhao et al., 2005). However, its curing time can be long, with research showing its final setting time to be approximately 170 min (Gandolfi et al., 2009). Moreover, its washout resistance is poor. CSiC is prone to disintegration after being soaked in deionized water, which inevitably limits its application in orthopedics (Xu et al., 2018). More recently, a commercially available bone cement VK100 (BonWRX, Phoenix, Arizona, United States) was applied in vertebral augmentation. This dual-paste cement contains dimethyl methylvinyl siloxanes, barium sulfate powder, platinum catalyst, and methylhydrogen siloxane cross linker in the component. After setting, the structure is not completely rigid and provides a certain amount of compressibility, which is hypothesized that with this feature it could prevent the adjacent segment fractures commonly seen at PVP and PKP procedures (Neyisci et al., 2018).
The shortcomings of the low fracture strength and long curing time of CSiC materials can be resolved by preparing bioactive materials combined with organic polymers.
1) It was found that by adding sodium alginate (SA) to C3S, its washout resistance and compressive strength could be improved (1 wt% C3S/SA, its maximum compressive strength being approximately 54.05 MPa), having no obvious effect on biocompatibility (Xu et al., 2018). However, it could increase the curing time by varying degrees. When the mass percentage was 2%, the final setting time increased to 153 min (Xu et al., 2018).
2) By adding 5% gelatin, its washout resistance could be improved, its tensile strength increasing from 2.4 MPa to approximately 3.4 MPa. Moreover, it was found that the CSiC could inhibit osteoclasts more effectively than CPC, which may be related to the slow release of silicon ions (Wu et al., 2020).
3) After adding magnesium phosphate to the C3S powder, the mechanical properties of the bone cement could be significantly improved, the compressive strength reaching as much as 86 MPa (Liu et al., 2015). With different mass percentages of C3S, the curing time could be controlled within 3–29 min, all showing good biological activity (Liu et al., 2015).
4) Changing the composition ratio of the TCA in MTA could significantly shorten the setting time—that is, when the addition of TCA reached 15%, the final setting time was approximately 31 min (Liu et al., 2012). However, TCA affects its compressive strength. For example, the maximum strength of MTA without TCA was approximately 55 MPa, and when 15% TCA was added, the maximum strength was approximately 40 MPa (Liu et al., 2012) (Figure 4).
The ideal bone cement should be used as a non-toxic plastic scaffold to fill bone defects, provide stable mechanical support for the bone based on human biomechanics, and promote the growth of new bone—which will eventually be resorbed—to achieve the complete healing of bone defects. As a long-established standard material, PMMA is widely used in the repair of bone defects in vertebral body augmentation, although many deficiencies have been exposed with its use. Scholars have used various modification methods to improve the performance, more in line with the purpose and requirements of clinical treatment. However, existing modified bone cements remain unable to overcome all their inherent shortcomings. The research and development of new materials are gradually being explored, but their rapid production and perfecting is challenging. Moreover, the long-term efficacy of various materials needs to be observed and improved in the future. Currently, bone cement materials offering specific advantages can be selected based on a patient’s needs for individualized treatment.
Conceptualization, YG; writing—original draft preparation, software, YG and BZ; writing—review and editing, supervision, funding acquisition, LY. All authors have read and agreed to the published version of the manuscript.
This study was supported by the Key Project of Natural Science Basic Research Plan of Shaanxi Province (Grant No. 2022JZ-43).
The authors declare that the research was conducted in the absence of any commercial or financial relationships that could be construed as a potential conflict of interest.
All claims expressed in this article are solely those of the authors and do not necessarily represent those of their affiliated organizations, or those of the publisher, the editors and the reviewers. Any product that may be evaluated in this article, or claim that may be made by its manufacturer, is not guaranteed or endorsed by the publisher.
The authors would like to thank the Honghui Hospital, Xi’an Jiao Tong University for providing the facilities for this research. We would like to thank Editage for its English editing service.
Abd Samad, H., Jaafar, M., Othman, R., Kawashita, M., and Razak, N. H. A. (2011). New Bioactive Glass-Ceramic: Synthesis and Application in PMMA Bone Cement Composites. Biomed. Mater Eng. 21, 247–258. doi:10.3233/BME-2011-0673
Albanna, M. Z., Bou-Akl, T. H., Blowytsky, O., Walters, H. L., and Matthew, H. W. T. (2013). Chitosan Fibers with Improved Biological and Mechanical Properties for Tissue Engineering Applications. J. Mech. Behav. Biomed. Mater. 20, 217–226. doi:10.1016/j.jmbbm.2012.09.012
Alhashash, M., Shousha, M., Barakat, A. S., and Boehm, H. (2019). Effects of Polymethylmethacrylate Cement Viscosity and Bone Porosity on Cement Leakage and New Vertebral Fractures after Percutaneous Vertebroplasty: A Prospective Study. Glob. Spine J. 9, 754–760. doi:10.1177/2192568219830327
Alshemary, A. Z., Bilgin, S., Işık, G., Motameni, A., Tezcaner, A., and Evis, Z. (2021). Biomechanical Evaluation of an Injectable Alginate/Dicalcium Phosphate Cement Composites for Bone Tissue Engineering. J. Mech. Behav. Biomed. Mater. 118, 104439. doi:10.1016/j.jmbbm.2021.104439
An, J., Liao, H., Kucko, N. W., Herber, R.-P., Wolke, J. G. C., van den Beucken, J. J. J. P., et al. (2016). Long-term Evaluation of the Degradation Behavior of Three Apatite-Forming Calcium Phosphate Cements. J. Biomed. Mat. Res. 104, 1072–1081. doi:10.1002/jbm.a.35641
Baroud, G., Vant, C., and Wilcox, R. (2006). Long-term Effects of Vertebroplasty: Adjacent Vertebral Fractures. J. Long. Term. Eff. Med. Implants 16, 265–280. doi:10.1615/jlongtermeffmedimplants.v16.i4.10
Bhattacharya, K., Mukherjee, S. P., Gallud, A., Burkert, S. C., Bistarelli, S., Bellucci, S., et al. (2016). Biological Interactions of Carbon-Based Nanomaterials: From Coronation to Degradation. Nanomedicine Nanotechnol. Biol. Med. 12, 333–351. doi:10.1016/j.nano.2015.11.011
Bistolfi, A., Ferracini, R., Albanese, C., Vernè, E., and Miola, M. (2019). PMMA-based Bone Cements and the Problem of Joint Arthroplasty Infections: Status and New Perspectives. Materials 12, 4002. doi:10.3390/ma12234002
Boger, A., Heini, P., Windolf, M., and Schneider, E. (2007). Adjacent Vertebral Failure after Vertebroplasty: a Biomechanical Study of Low-Modulus PMMA Cement. Eur. Spine J. 16, 2118–2125. doi:10.1007/s00586-007-0473-0
Boger, A., Bohner, M., Heini, P., Verrier, S., and Schneider, E. (2008). Properties of an Injectable Low Modulus PMMA Bone Cement for Osteoporotic Bone. J. Biomed. Mat. Res. 86B, 474–482. doi:10.1002/jbm.b.31044
Bohner, M., Merkle, H. P., and Lemai^tre, J. (2000). In Vitro aging of a Calcium Phosphate Cement. J. Mater Sci. Mater Med. 11, 155–162. doi:10.1023/a:1008927624493
Bohner, M., Theiss, F., Apelt, D., Hirsiger, W., Houriet, R., Rizzoli, G., et al. (2003). Compositional Changes of a Dicalcium Phosphate Dihydrate Cement after Implantation in Sheep. Biomaterials 24, 3463–3474. doi:10.1016/s0142-9612(03)00234-5
Bu, B. X., Wang, M. J., Liu, W. F., Wang, Y. S., and Tan, H. L. (2015). Short-segment Posterior Instrumentation Combined with Calcium Sulfate Cement Vertebroplasty for Thoracolumbar Compression Fractures: Radiographic Outcomes Including Nonunion and Other Complications. Orthop. Traumatology Surg. Res. 101, 227–233. doi:10.1016/j.otsr.2014.11.019
Buchbinder, R., Osborne, R. H., Ebeling, P. R., Wark, J. D., Mitchell, P., Wriedt, C., et al. (2009). A Randomized Trial of Vertebroplasty for Painful Osteoporotic Vertebral Fractures. N. Engl. J. Med. 361, 557–568. doi:10.1056/NEJMoa0900429
Burge, R., Dawson-Hughes, B., Solomon, D. H., Wong, J. B., King, A., and Tosteson, A. (2007). Incidence and Economic Burden of Osteoporosis-Related Fractures in the United States, 2005-2025. J. Bone Min. Res. 22, 465–475. doi:10.1359/jbmr.061113
Canal, C., and Ginebra, M. P. (2011). Fibre-reinforced Calcium Phosphate Cements: a Review. J. Mech. Behav. Biomed. Mater. 4, 1658–1671. doi:10.1016/j.jmbbm.2011.06.023
Charrière, E., Terrazzoni, S., Pittet, C., Mordasini, P., Dutoit, M., Lemaître, J., et al. (2001). Mechanical Characterization of Brushite and Hydroxyapatite Cements. Biomaterials 22, 2937–2945. doi:10.1016/s0142-9612(01)00041-2
Chen, Y.-J., Tan, T.-S., Chen, W.-H., Chen, C. C.-C., and Lee, T.-S. (2006). Intradural Cement Leakage. Spine 31, E379–E382. doi:10.1097/01.brs.0000219495.57470.67
Chen, Z., Liu, H., Liu, X., Lian, X., Guo, Z., Jiang, H.-J., et al. (2013). Improved Workability of Injectable Calcium Sulfate Bone Cement by Regulation of Self-Setting Properties. Mater. Sci. Eng. C 33, 1048–1053. doi:10.1016/j.msec.2012.11.019
Chen, X.-s., Jiang, J.-m., Sun, P.-d., Zhang, Z.-f., and Ren, H.-l. (2020). How the Clinical Dosage of Bone Cement Biomechanically Affects Adjacent Vertebrae. J. Orthop. Surg. Res. 15, 370. doi:10.1186/s13018-020-01906-0
Chu, W., Tsuei, Y.-C., Liao, P.-H., Lin, J.-H., Chou, W.-H., Chu, W.-C., et al. (2013). Decompressed Percutaneous Vertebroplasty: a Secured Bone Cement Delivery Procedure for Vertebral Augmentation in Osteoporotic Compression Fractures. Injury 44, 813–818. doi:10.1016/j.injury.2012.10.017
Clark, W., Bird, P., Gonski, P., Diamond, T. H., Smerdely, P., McNeil, H. P., et al. (2016). Safety and Efficacy of Vertebroplasty for Acute Painful Osteoporotic Fractures (VAPOUR): a Multicentre, Randomised, Double-Blind, Placebo-Controlled Trial. Lancet 388, 1408–1416. doi:10.1016/S0140-6736(16)31341-1
Compston, J. E., McClung, M. R., and Leslie, W. D. (2019). Osteoporosis. Lancet 393, 364–376. doi:10.1016/s0140-6736(18)32112-3
Dadkhah, M., Pontiroli, L., Fiorilli, S., Manca, A., Tallia, F., Tcacencu, I., et al. (2017). Preparation and Characterisation of an Innovative Injectable Calcium Sulphate Based Bone Cement for Vertebroplasty Application. J. Mat. Chem. B 5, 102–115. doi:10.1039/c6tb02139e
Dall'Oca, C., Maluta, T., Cavani, F., Morbioli, G. P., Bernardi, P., Sbarbati, A., et al. (2014). The Biocompatibility of Porous vs Non-porous Bone Cements: a New Methodological Approach. Eur. J. Histochem 58, 2255. doi:10.4081/ejh.2014.2255
de Lacerda Schickert, S., Pinto, J. C., Jansen, J., Leeuwenburgh, S. C. G., and van den Beucken, J. J. J. P. (2020). Tough and Injectable Fiber Reinforced Calcium Phosphate Cement as an Alternative to Polymethylmethacrylate Cement for Vertebral Augmentation: a Biomechanical Study. Biomater. Sci. 8, 4239–4250. doi:10.1039/d0bm00413h
De Mori, A., Di Gregorio, E., Kao, A. P., Tozzi, G., Barbu, E., Sanghani-Kerai, A., et al. (2019). Antibacterial PMMA Composite Cements with Tunable Thermal and Mechanical Properties. ACS Omega 4, 19664–19675. doi:10.1021/acsomega.9b02290
Ding, Z., Xi, W., Ji, M., Chen, H., Zhang, Q., and Yan, Y. (2021). Developing a Biodegradable Tricalcium Silicate/glucono-Delta-Lactone/calcium Sulfate Dihydrate Composite Cement with High Preliminary Mechanical Property for Bone Filling. Mater. Sci. Eng. C 119, 111621. doi:10.1016/j.msec.2020.111621
Draenert, F. G., and Draenert, K. (2008). Methotrexate-loaded Polymethylmethacrylate Bone Cement for Local Bone Metastasis Therapy: Pilot Animal Study in the Rabbit Patellar Groove. Chemotherapy 54, 412–416. doi:10.1159/000153315
Duran, C., Sirvanci, M., Aydoğan, M., Ozturk, E., Ozturk, C., and Akman, C. (2007). Pulmonary Cement Embolism: a Complication of Percutaneous Vertebroplasty. Acta Radiol. 48, 854–859. doi:10.1080/02841850701422153
El-Fiqi, A., Kim, J.-H., Perez, R. A., and Kim, H.-W. (2015). Novel Bioactive Nanocomposite Cement Formulations with Potential Properties: Incorporation of the Nanoparticle Form of Mesoporous Bioactive Glass into Calcium Phosphate Cements. J. Mat. Chem. B 3, 1321–1334. doi:10.1039/c4tb01634c
Engstrand, J., Persson, C., and Engqvist, H. (2014). The Effect of Composition on Mechanical Properties of Brushite Cements. J. Mech. Behav. Biomed. Mater. 29, 81–90. doi:10.1016/j.jmbbm.2013.08.024
Ensrud, K. E., Blackwell, T. L., Fink, H. A., Zhang, J., Cauley, J. A., Cawthon, P. M., et al. (2016). What Proportion of Incident Radiographic Vertebral Fractures in Older Men Is Clinically Diagnosed and Vice Versa: A Prospective Study. J. Bone Min. Res. 31, 1500–1503. doi:10.1002/jbmr.2831
Ensrud, K. E., and Schousboe, J. T. (2011). Vertebral Fractures. N. Engl. J. Med. 364, 1634–1642. doi:10.1056/NEJMcp1009697
Eriksson, R. A., Albrektsson, T., and Magnusson, B. (1984). Assessment of Bone Viability after Heat Trauma: A Histological, Histochemical and Vital Microscopic Study in the Rabbit. Scand. J. Plastic Reconstr. Surg. 18, 261–268. doi:10.3109/02844318409052849
Fan, H., Yang, Q., Zheng, D., Gao, C., and Zhu, P. (2021). Synergistic Effects of Wheat Gluten and Poly (γ-Glutamic Acid) on Physicochemical Properties of Bioglass Modified Beta-Tricalcium Phosphate Bone Cement. Mater. Technol., 1–15. doi:10.1080/10667857.2021.2016294
Filippiadis, D. K., Marcia, S., Masala, S., Deschamps, F., and Kelekis, A. (2017). Percutaneous Vertebroplasty and Kyphoplasty: Current Status, New Developments and Old Controversies. Cardiovasc Interv. Radiol. 40, 1815–1823. doi:10.1007/s00270-017-1779-x
Friedman, M., and Juneja, V. K. (2010). Review of Antimicrobial and Antioxidative Activities of Chitosans in Food. J. Food Prot. 73, 1737–1761. doi:10.4315/0362-028x-73.9.1737
Gandolfi, M. G., Iacono, F., Agee, K., Siboni, F., Tay, F., Pashley, D. H., et al. (2009). Setting Time and Expansion in Different Soaking Media of Experimental Accelerated Calcium-Silicate Cements and ProRoot MTA. Oral Surg. Oral Med. Oral Pathology, Oral Radiology, Endodontology 108, e39–e45. doi:10.1016/j.tripleo.2009.07.039
Gbureck, U., Spatz, K., Thull, R., and Barralet, J. E. (2005). Rheological Enhancement of Mechanically Activated ?-tricalcium Phosphate Cements. J. Biomed. Mat. Res. 73B, 1–6. doi:10.1002/jbm.b.30148
Ginebra, M. P., Espanol, M., Montufar, E. B., Perez, R. A., and Mestres, G. (2010). New Processing Approaches in Calcium Phosphate Cements and Their Applications in Regenerative Medicine. Acta Biomater. 6, 2863–2873. doi:10.1016/j.actbio.2010.01.036
Ginebra, M.-P., Canal, C., Espanol, M., Pastorino, D., and Montufar, E. B. (2012). Calcium Phosphate Cements as Drug Delivery Materials. Adv. Drug Deliv. Rev. 64, 1090–1110. doi:10.1016/j.addr.2012.01.008
Gong, T., Wang, Z., Zhang, Y., Zhang, Y., Hou, M., Liu, X., et al. (2016). A Comprehensive Study of Osteogenic Calcium Phosphate Silicate Cement: Material Characterization and In Vitro/In Vivo Testing. Adv. Healthc. Mat. 5, 457–466. doi:10.1002/adhm.201500469
Grossardt, C., Ewald, A., Grover, L. M., Barralet, J. E., and Gbureck, U. (2010). Passive and Active In Vitro Resorption of Calcium and Magnesium Phosphate Cements by Osteoclastic Cells. Tissue Eng. Part A 16, 3687–3695. doi:10.1089/ten.TEA.2010.0281
Gundapaneni, D., and Goswami, T. (2014). Thermal Isotherms in PMMA and Cell Necrosis during Total Hip Arthroplasty. J. Appl. Biomaterials Funct. Mater. 12, 193–202. doi:10.5301/jabfm.5000196
Hall, T. J., Hughes, E. A. B., Sajjad, H., Kuehne, S. A., Grant, M. M., Grover, L. M., et al. (2021). Formulation of a Reactive Oxygen Producing Calcium Sulphate Cement as an Anti-bacterial Hard Tissue Scaffold. Sci. Rep. 11, 4491. doi:10.1038/s41598-021-84060-9
Hasan, M. L., Kim, B., Padalhin, A. R., Faruq, O., Sultana, T., and Lee, B.-T. (2019). In Vitro and In Vivo Evaluation of Bioglass Microspheres Incorporated Brushite Cement for Bone Regeneration. Mater. Sci. Eng. C 103, 109775. doi:10.1016/j.msec.2019.109775
Helgason, B., Perilli, E., Schileo, E., Taddei, F., Brynjólfsson, S., and Viceconti, M. (2008). Mathematical Relationships between Bone Density and Mechanical Properties: a Literature Review. Clin. Biomech. 23, 135–146. doi:10.1016/j.clinbiomech.2007.08.024
Hofmann, M., Mohammed, A., Perrie, Y., Gbureck, U., and Barralet, J. (2009). High-strength Resorbable Brushite Bone Cement with Controlled Drug-Releasing Capabilities. Acta Biomater. 5, 43–49. doi:10.1016/j.actbio.2008.08.005
Holub, O., López, A., Borse, V., Engqvist, H., Kapur, N., Hall, R. M., et al. (2015). Biomechanics of Low-Modulus and Standard Acrylic Bone Cements in Simulated Vertebroplasty: A Human Ex Vivo Study. J. Biomechanics 48, 3258–3266. doi:10.1016/j.jbiomech.2015.06.026
Huan, Z., and Chang, J. (2007). Self-setting Properties and In Vitro Bioactivity of Calcium Sulfate Hemihydrate-Tricalcium Silicate Composite Bone Cements. Acta Biomater. 3, 952–960. doi:10.1016/j.actbio.2007.05.003
Huan, Z., and Chang, J. (2009). Novel Bioactive Composite Bone Cements Based on the β-tricalcium Phosphate-Monocalcium Phosphate Monohydrate Composite Cement System. Acta Biomater. 5, 1253–1264. doi:10.1016/j.actbio.2008.10.006
Huang, T.-H., Kao, C.-T., Shen, Y.-F., Lin, Y.-T., Liu, Y.-T., Yen, S.-Y., et al. (2019). Substitutions of Strontium in Bioactive Calcium Silicate Bone Cements Stimulate Osteogenic Differentiation in Human Mesenchymal Stem Cells. J. Mater Sci. Mater Med. 30, 68. doi:10.1007/s10856-019-6274-2
Ji, M., Ding, Z., Chen, H., Peng, H., and Yan, Y. (2019). Design of Novel Organic-Inorganic Composite Bone Cements with High Compressive Strength, In Vitro Bioactivity and Cytocompatibility. J. Biomed. Mater Res. B 107, 2365–2377. doi:10.1002/jbm.b.34330
Jones, J. R. (2013). Review of Bioactive Glass: from Hench to Hybrids. Acta Biomater. 9, 4457–4486. doi:10.1016/j.actbio.2012.08.023
Kado, D. M., Duong, T., Nevitt, M. C., Greendale, G. A., Cummings, S. R., Stone, K. L., et al. (2003). Incident Vertebral Fractures and Mortality in Older Women: a Prospective Study. Osteoporos. Int. 14, 589–594. doi:10.1007/s00198-003-1412-5
Kallmes, D. F., Comstock, B. A., Heagerty, P. J., Turner, J. A., Wilson, D. J., Diamond, T. H., et al. (2009). A Randomized Trial of Vertebroplasty for Osteoporotic Spinal Fractures. N. Engl. J. Med. 361, 569–579. doi:10.1056/NEJMoa0900563
Kalteis, T., Lüring, C., Gugler, G., Zysk, S., Caro, W., Handel, M., et al. (2004). Akute Gewebetoxizität von PMMA-Knochenzementen. Z Orthop. Ihre Grenzgeb 142, 666–672. doi:10.1055/s-2004-832317
Khandaker, M., Vaughan, M., Morris, T., White, J., and Meng, Z. (2014). Effect of Additive Particles on Mechanical, Thermal, and Cell Functioning Properties of Poly(methyl Methacrylate) Cement. Int. J. Nanomedicine 9, 2699–2712. doi:10.2147/IJN.S61964
Kim, Y. J., Lee, J. W., Park, K. W., Yeom, J.-S., Jeong, H. S., Park, J. M., et al. (2009). Pulmonary Cement Embolism after Percutaneous Vertebroplasty in Osteoporotic Vertebral Compression Fractures: Incidence, Characteristics, and Risk Factors. Radiology 251, 250–259. doi:10.1148/radiol.2511080854
Knabe, C., Adel-Khattab, D., Hübner, W.-D., Peters, F., Knauf, T., Peleska, B., et al. (2019). Effect of Silicon-Doped Calcium Phosphate Bone Grafting Materials on Bone Regeneration and Osteogenic Marker Expression after Implantation in the Ovine Scapula. J. Biomed. Mat. Res. 107, 594–614. doi:10.1002/jbm.b.34153
Kucko, N. W., Li, W., García Martinez, M. A., Rehman, I. u., Ulset, A.-S. T., Christensen, B. E., et al. (2019). Sterilization Effects on the Handling and Degradation Properties of Calcium Phosphate Cements Containing Poly (D,L -Lactic-Co-Glycolic Acid) Porogens and Carboxymethyl Cellulose. J. Biomed. Mater Res. B 107, 2216–2228. doi:10.1002/jbm.b.34306
Li, Z., Ni, C., Chen, L., Sun, Z., Yang, C., Zhao, X., et al. (2014). Kyphoplasty Versus Vertebroplasty for the Treatment of Malignant Vertebral Compression Fractures Caused by Metastases: a Retrospective Study. Chin. Med. J. Engl. 127, 1493–1496. doi:10.3760/cma.j.issn.0366-6999.20132435
Li, K., Ji, C., Luo, D., Zhang, W., Feng, H., Yang, K., et al. (2021). Role of Percutaneous Vertebroplasty with High-Viscosity Cement in the Treatment of Severe Osteoporotic Vertebral Compression Fractures. Sci. Rep. 11, 4602. doi:10.1038/s41598-021-84314-6
Lin, Q., Zhang, X., Liang, D., Li, J., Wang, W., Wang, Z., et al. (2021). The In Vivo Dissolution of Tricalcium Silicate Bone Cement. J. Biomed. Mater Res. 109, 2527–2535. doi:10.1002/jbm.a.37247
Linhong Wang, L., Yu, W., Yin, X., Cui, L., Tang, S., Jiang, N., et al. (2021). Prevalence of Osteoporosis and Fracture in China. JAMA Netw. Open 4, e2121106. doi:10.1001/jamanetworkopen.2021.21106
Liu, J. T., Liao, W. J., Tan, W. C., Lee, J. K., Liu, C. H., Chen, Y. H., et al. (2010). Balloon Kyphoplasty versus Vertebroplasty for Treatment of Osteoporotic Vertebral Compression Fracture: a Prospective, Comparative, and Randomized Clinical Study. Osteoporos. Int. 21, 359–364. doi:10.1007/s00198-009-0952-8
Liu, W., Peng, W., Zhu, Y., and Chang, J. (2012). Physicochemical Properties and In Vitro Biocompatibility of a Hydraulic Calcium Silicate/tricalcium Aluminate Cement for Endodontic Use. J. Biomed. Mat. Res. 100B, 1257–1263. doi:10.1002/jbm.b.32690
Liu, W., Zhai, D., Huan, Z., Wu, C., and Chang, J. (2015). Novel Tricalcium Silicate/magnesium Phosphate Composite Bone Cement Having High Compressive Strength, In Vitro Bioactivity and Cytocompatibility. Acta Biomater. 21, 217–227. doi:10.1016/j.actbio.2015.04.012
Lu, Q., Liu, C., Wang, D., Liu, H., Yang, H., and Yang, L. (2019). Biomechanical Evaluation of Calcium Phosphate-Based Nanocomposite versus Polymethylmethacrylate Cement for Percutaneous Kyphoplasty. Spine J. 19, 1871–1884. doi:10.1016/j.spinee.2019.06.007
Luo, S., Jiang, T., Yang, Y., Yang, X., and Zhao, J. (2016). Combination Therapy with Vancomycin-Loaded Calcium Sulfate and Vancomycin-Loaded PMMA in the Treatment of Chronic Osteomyelitis. BMC Musculoskelet. Disord. 17, 502. doi:10.1186/s12891-016-1352-9
Luo, J., Faivre, J., Engqvist, H., and Persson, C. (2019). The Addition of Poly(Vinyl Alcohol) Fibers to Apatitic Calcium Phosphate Cement Can Improve its Toughness. Materials 12, 1531. doi:10.3390/ma12091531
Maenz, S., Brinkmann, O., Kunisch, E., Horbert, V., Gunnella, F., Bischoff, S., et al. (2017). Enhanced Bone Formation in Sheep Vertebral Bodies after Minimally Invasive Treatment with a Novel, PLGA Fiber-Reinforced Brushite Cement. Spine J. 17, 709–719. doi:10.1016/j.spinee.2016.11.006
Maestretti, G., Sutter, P., Monnard, E., Ciarpaglini, R., Wahl, P., Hoogewoud, H., et al. (2014). A Prospective Study of Percutaneous Balloon Kyphoplasty with Calcium Phosphate Cement in Traumatic Vertebral Fractures: 10-year Results. Eur. Spine J. 23, 1354–1360. doi:10.1007/s00586-014-3206-1
Maffezzoli, A., Ronca, D., Guida, G., Pochini, I., and Nicolais, L. (1997). In-situ Polymerization Behaviour of Bone Cements. J. Mater Sci. Mater Med. 8, 75–83. doi:10.1023/a:1018502731892
Marks, K., Nelson, C., and Lautenschlager, E. (1976). Antibiotic-impregnated Acrylic Bone Cement. J. Bone & Jt. Surg. 58, 358–364. doi:10.2106/00004623-197658030-00011
Mengran Wang, M., Zhang, L., Fu, Z., Wang, H., and Wu, Y. (2021). Selections of Bone Cement Viscosity and Volume in Percutaneous Vertebroplasty: A Retrospective Cohort Study. World Neurosurg. 150, e218–e227. doi:10.1016/j.wneu.2021.02.133
Miao, Q., Yang, S., Ding, H., and Liu, J. (2020). Controlled Degradation of Chitosan-Coated Strontium-Doped Calcium Sulfate Hemihydrate Composite Cement Promotes Bone Defect Repair in Osteoporosis Rats. Biomed. Mat. 15, 055039. doi:10.1088/1748-605X/ab9fcf
Milner, R. (2004). The Development of Theoretical Relationships between Some Handling Parameters (Setting Time and Setting Temperature), Composition (Relative Amounts of Initiator and Activator) and Ambient Temperature for Acrylic Bone Cement. J. Biomed. Mat. Res. 68B, 180–185. doi:10.1002/jbm.b.20019
Nazarian, A., von Stechow, D., Zurakowski, D., Müller, R., and Snyder, B. D. (2008). Bone Volume Fraction Explains the Variation in Strength and Stiffness of Cancellous Bone Affected by Metastatic Cancer and Osteoporosis. Calcif. Tissue Int. 83, 368–379. doi:10.1007/s00223-008-9174-x
Nekoofar, M. H., Adusei, G., Sheykhrezae, M. S., Hayes, S. J., Bryant, S. T., and Dummer, P. M. H. (2007). The Effect of Condensation Pressure on Selected Physical Properties of Mineral Trioxide Aggregate. Int. Endod. J. 40, 453–461. doi:10.1111/j.1365-2591.2007.01236.x
Neyisci, C., Erdem, Y., Bilekli, A. B., Demiralp, B., Kose, O., Bek, D., et al. (2018). Treatment of Implant-Related Methicillin-Resistant Staphylococcus aureus Osteomyelitis with Vancomycin-Loaded VK100 Silicone Cement: An Experimental Study in Rats. J. Orthop. Surg. 26, 230949901775409. doi:10.1177/2309499017754093
Nilsson, M., Wang, J.-S., Wielanek, L., Tanner, K. E., and Lidgren, L. (2004). Biodegradation and Biocompatability of a Calcium Sulphate-Hydroxyapatite Bone Substitute. J. Bone Jt. Surg. Br. volume 86-B, 120–125. doi:10.1302/0301-620x.86b1.14040
Nouda, S., Tomita, S., Kin, A., Kawahara, K., and Kinoshita, M. (2009). Adjacent Vertebral Body Fracture Following Vertebroplasty with Polymethylmethacrylate or Calcium Phosphate Cement. Spine 34, 2613–2618. doi:10.1097/BRS.0b013e3181abc150
Öhman‐Mägi, C., Holub, O., Wu, D., Hall, R. M., and Persson, C. (2021). Density and Mechanical Properties of Vertebral Trabecular Bone-A Review. JOR Spine 4, e1176. doi:10.1002/jsp2.1176
Paz, E., Ballesteros, Y., Abenojar, J., Del Real, J. C., and Dunne, N. J. (2019). Graphene Oxide and Graphene Reinforced PMMA Bone Cements: Evaluation of Thermal Properties and Biocompatibility. Materials 12, 3146. doi:10.3390/ma12193146
Pflugmacher, R., Schroeder, R.-J., and Klostermann, C. K. (2006). Incidence of Adjacent Vertebral Fractures in Patients Treated with Balloon Kyphoplasty: Two Years' Prospective Follow-Up. Acta Radiol. 47, 830–840. doi:10.1080/02841850600854928
Phakatkar, A. H., Shirdar, M. R., Qi, M.-l., Taheri, M. M., Narayanan, S., Foroozan, T., et al. (2020). Novel PMMA Bone Cement Nanocomposites Containing Magnesium Phosphate Nanosheets and Hydroxyapatite Nanofibers. Mater. Sci. Eng. C 109, 110497. doi:10.1016/j.msec.2019.110497
Qi, L., Li, C., Wang, N., Lian, H., Lian, M., He, B., et al. (2018). Efficacy of Percutaneous Vertebroplasty Treatment of Spinal Tumors. Med. Baltim. 97, e9575. doi:10.1097/MD.0000000000009575
Roberts, H., Toth, J., Berzins, D., and Charlton, D. (2008). Mineral Trioxide Aggregate Material Use in Endodontic Treatment: A Review of the Literature☆. Dent. Mater. 24, 149–164. doi:10.1016/j.dental.2007.04.007
Robo, C., Hulsart-Billström, G., Nilsson, M., and Persson, C. (2018a). In Vivo response to a Low-Modulus PMMA Bone Cement in an Ovine Model. Acta Biomater. 72, 362–370. doi:10.1016/j.actbio.2018.03.014
Robo, C., Öhman-Mägi, C., and Persson, C. (2018b). Compressive Fatigue Properties of Commercially Available Standard and Low-Modulus Acrylic Bone Cements Intended for Vertebroplasty. J. Mech. Behav. Biomed. Mater. 82, 70–76. doi:10.1016/j.jmbbm.2018.03.001
Robo, C., Öhman-Mägi, C., and Persson, C. (2021). Long-term Mechanical Properties of a Novel Low-Modulus Bone Cement for the Treatment of Osteoporotic Vertebral Compression Fractures. J. Mech. Behav. Biomed. Mater. 118, 104437. doi:10.1016/j.jmbbm.2021.104437
Santiago, M. C., Gomes-Cornélio, A. L., de Oliveira, L. A., Tanomaru-Filho, M., and Salles, L. P. (2021). Calcium Silicate-Based Cements Cause Environmental Stiffness and Show Diverse Potential to Induce Osteogenesis in Human Osteoblastic Cells. Sci. Rep. 11, 16784. doi:10.1038/s41598-021-96353-0
Schousboe, J. T. (2016). Epidemiology of Vertebral Fractures. J. Clin. Densitom. 19, 8–22. doi:10.1016/j.jocd.2015.08.004
Shie, M.-Y., Chiang, W.-H., Chen, I.-W. P., Liu, W.-Y., and Chen, Y.-W. (2017). Synergistic Acceleration in the Osteogenic and Angiogenic Differentiation of Human Mesenchymal Stem Cells by Calcium Silicate-Graphene Composites. Mater. Sci. Eng. C 73, 726–735. doi:10.1016/j.msec.2016.12.071
Si, L., Winzenberg, T. M., Jiang, Q., Chen, M., and Palmer, A. J. (2015). Projection of Osteoporosis-Related Fractures and Costs in China: 2010-2050. Osteoporos. Int. 26, 1929–1937. doi:10.1007/s00198-015-3093-2
Thomas, M. V., and Puleo, D. A. (2009). Calcium Sulfate: Properties and Clinical Applications. J. Biomed. Mat. Res. 88B, 597–610. doi:10.1002/jbm.b.31269
Tsukeoka, T., Suzuki, M., Ohtsuki, C., Sugino, A., Tsuneizumi, Y., Miyagi, J., et al. (2006). Mechanical and Histological Evaluation of a PMMA-Based Bone Cement Modified with γ-methacryloxypropyltrimethoxysilane and Calcium Acetate. Biomaterials 27, 3897–3903. doi:10.1016/j.biomaterials.2006.03.002
Tunney, M. M., Brady, A. J., Buchanan, F., Newe, C., and Dunne, N. J. (2008). Incorporation of Chitosan in Acrylic Bone Cement: Effect on Antibiotic Release, Bacterial Biofilm Formation and Mechanical Properties. J. Mater Sci. Mater Med. 19, 1609–1615. doi:10.1007/s10856-008-3394-5
Wu, I.-T., Kao, P.-F., Huang, Y.-R., and Ding, S.-J. (2020). In Vitro and In Vivo Osteogenesis of Gelatin-Modified Calcium Silicate Cement with Washout Resistance. Mater. Sci. Eng. C 117, 111297. doi:10.1016/j.msec.2020.111297
Xu, S.-J., Qiu, Z.-Y., Wu, J.-J., Kong, X.-D., Weng, X.-S., Cui, F.-Z., et al. (2016). Osteogenic Differentiation Gene Expression Profiling of hMSCs on Hydroxyapatite and Mineralized Collagen. Tissue Eng. Part A 22, 170–181. doi:10.1089/ten.TEA.2015.0237
Xu, C., Wang, X., Zhou, J., Huan, Z., and Chang, J. (2018). Bioactive Tricalcium Silicate/alginate Composite Bone Cements with Enhanced Physicochemical Properties. J. Biomed. Mat. Res. 106, 237–244. doi:10.1002/jbm.b.33848
Xu, B., Sun, H., Jia, H., Li, Z., Sang, L., Wu, Y., et al. (2021). Application of New Bone Cement Biomaterials in Osteoporotic Compression Fractures. Sci. Adv. mater 13, 662–671. doi:10.1166/sam.2021.3959
Yan, X., and Gemeinhart, R. A. (2005). Cisplatin Delivery from Poly(acrylic Acid-Co-Methyl Methacrylate) Microparticles. J. Control. Release 106, 198–208. doi:10.1016/j.jconrel.2005.05.005
Yang, D., Yan, Y., Liu, X., Wang, P., Huang, G., Xu, G., et al. (2018). Characterization of an α-Calcium Sulfate Hemihydrates/α-Tricalcium Phosphate Combined Injectable Bone Cement. ACS Appl. Bio Mat. 1, 768–776. doi:10.1021/acsabm.8b00221
Yang, B.-C., Lee, J.-W., Ju, C.-P., and Chern Lin, J.-H. (2020). Physical/Chemical Properties and Resorption Behavior of a Newly Developed Ca/P/S-Based Bone Substitute Material. Materials 13, 3458. doi:10.3390/ma13163458
Yimin, Y., Zhiwei, R., Wei, M., and Jha, R. (2013). Current Status of Percutaneous Vertebroplasty and Percutaneous Kyphoplasty - a Review. Med. Sci. Monit. 19, 826–836. doi:10.12659/MSM.889479
Yingjie Wang, Y., Shen, S., Hu, T., Williams, G. R., Bian, Y., Feng, B., et al. (2021). Layered Double Hydroxide Modified Bone Cement Promoting Osseointegration via Multiple Osteogenic Signal Pathways. ACS Nano 15, 9732–9745. doi:10.1021/acsnano.1c00461
Yu, L., Li, Y., Zhao, K., Tang, Y., Cheng, Z., Chen, J., et al. (2013). A Novel Injectable Calcium Phosphate Cement-Bioactive Glass Composite for Bone Regeneration. PLoS One 8, e62570. doi:10.1371/journal.pone.0062570
Yuan, Z., Bi, J., Wang, W., Sun, X., Wang, L., Mao, J., et al. (2021). Synthesis and Properties of Sr2+ Doping α-tricalcium Phosphate at Low Temperature. J. Appl. Biomaterials Funct. Mater. 19, 228080002199699. doi:10.1177/2280800021996999
Zapata, M. E. V., Ruiz Rojas, L. M., Mina Hernández, J. H., Delgado-Ospina, J., and Tovar, C. D. G. (2020). Acrylic Bone Cements Modified with Graphene Oxide: Mechanical, Physical, and Antibacterial Properties. Polymers 12, 1773. doi:10.3390/polym12081773
Zhang, F., Zhu, H., Wang, G., Xie, J., Tao, Y., Xia, W., et al. (2018). Preparation and Characterization of a Silk Fibroin/calcium Sulfate Bone Cement. J. Biomed. Mat. Res. 106, 512–519. doi:10.1002/jbm.b.33855
Zhang, R., Hu, J., Chen, H., Ding, Z., Ouyang, Y., Zhang, Q., et al. (2021). A Novel Degradable Tricalcium Silicate/calcium Polyphosphate/polyvinyl Alcohol Organic-Inorganic Composite Cement for Bone Filling. J. Biomater. Appl. 36, 772–788. doi:10.1177/08853282211020399
Zhao, W., Wang, J., Zhai, W., Wang, Z., and Chang, J. (2005). The Self-Setting Properties and In Vitro Bioactivity of Tricalcium Silicate. Biomaterials 26, 6113–6121. doi:10.1016/j.biomaterials.2005.04.025
Keywords: bone cement, polymethyl methacrylate, modified, alternative materials, osteoporotic vertebral compression fracture
Citation: Gong Y, Zhang B and Yan L (2022) A Preliminary Review of Modified Polymethyl Methacrylate and Calcium-Based Bone Cement for Improving Properties in Osteoporotic Vertebral Compression Fractures. Front. Mater. 9:912713. doi: 10.3389/fmats.2022.912713
Received: 04 April 2022; Accepted: 24 May 2022;
Published: 08 June 2022.
Edited by:
Masoud Mozafari, University of Toronto, CanadaReviewed by:
Panagiotis Korovessis, Olympion Medical Center, GreeceCopyright © 2022 Gong, Zhang and Yan. This is an open-access article distributed under the terms of the Creative Commons Attribution License (CC BY). The use, distribution or reproduction in other forums is permitted, provided the original author(s) and the copyright owner(s) are credited and that the original publication in this journal is cited, in accordance with accepted academic practice. No use, distribution or reproduction is permitted which does not comply with these terms.
*Correspondence: Liang Yan, eWFubGlhbmdkcjU1ODNAMTYzLmNvbQ==
†These authors have contributed equally to this work
Disclaimer: All claims expressed in this article are solely those of the authors and do not necessarily represent those of their affiliated organizations, or those of the publisher, the editors and the reviewers. Any product that may be evaluated in this article or claim that may be made by its manufacturer is not guaranteed or endorsed by the publisher.
Research integrity at Frontiers
Learn more about the work of our research integrity team to safeguard the quality of each article we publish.