- 1Department of Ultrasound, Fudan University Shanghai Cancer Center, Department of Oncology, Shanghai Medical College, Fudan University, Shanghai, China
- 2Department of Ultrasound, Huashan Hospital, Fudan University, Shanghai, China
Inspired by the distinct metabolic pathway of tumor cells, here a new anti-cancer therapy (i.e., Cu-TCPP(Fe) metal-organic frameworks (MOFs) with sonosensitizer for sonodynamic therapy (SDT) and tumor microenvironment (TME)-specific release of copper/iron for chemical catalysis) is introduced and verified. Upon exposure to ultrasound, the porphyrin structure in the MOFs yields reactive oxygen species (ROS), and the copper/iron induces the Fenton reaction in the TME existing H2O2 and acid. Thus, highly toxic ROS are generated through these TME-specific reactions to trigger the apoptosis and death of tumor cells. The current work paves a new avenue to design SDT with catalytic nanomedicine for the selective and effective treatment of tumors.
1 Introduction
Malignant tumors are a global public health issue (Siegel et al., 2021). Although striking progress has been made in the area of targeted cancer therapy, the anticancer effect of molecular targeted treatment is still inadequate (Cheng et al., 2014; Chu and Dupuy, 2014; Liu et al., 2015a; Zhang et al., 2015; Long et al., 2016; Pelaz et al., 2017). To better understand the tumor microenvironment (TME), tumor cellular metabolism, biosynthetic intermediates, and the physical environment have been broadly studied (Carmeliet and Jain, 2000; Coussens and Werb, 2002; Hanahan and Weinberg, 2011; Wang et al., 2017). Among all TME signals, reactive oxygen species (ROS) (Trachootham et al., 2009; DeBerardinis and Chandel, 2016; Hornsveld and Dansen, 2016)—chemically reactive molecules containing oxygen, including hydroxyl radical (•OH), superoxide anion (O2•-), singlet oxygen (1O2), and hydrogen peroxide (H2O2)—were found to be important messengers in the cell. Changes in the levels of ROS influence cell proliferation, differentiation, apoptosis, or necrosis. Low levels of ROS promote cell proliferation and survival. However, excess production of ROS can induce cell senescence and death by causing oxidative damage to intracellular biomacromolecules, such as proteins, lipids, RNA, and DNA (Landry and Cotter, 2014; Galadari et al., 2017; Zou et al., 2017). Therefore, ROS-mediated cancer therapy has gained increasing attention (Dolmans et al., 2003; Castano et al., 2006; Allison and Sibata, 2010; Firczuk et al., 2011). It should be noted that increasing ROS levels via molecular-targeted drugs is a novel therapeutic regimen for treating cancer patients with drug-resistant tumors.
As an emerging non-invasive management technique for malignant tumors, sonodynamic therapy (SDT) has received extensive attention in recent years (Deepagan et al., 2016; McEwan et al., 2016; Huang et al., 2017a; Kou et al., 2017). Similar to photodynamic therapy, SDT uses therapeutic ultrasound (US) waves to activate a sonosensitizer (e.g., porphyrins) to generate ROS, which directly induces cancer cell death (Harada et al., 2011; Li et al., 2016). Moreover, SDT has been considered as a potential complementary treatment to conventional cancer chemotherapy because of its deep-tissue penetration, high spatial accuracy, and biosafety features. Additionally, advancements in novel nanomaterials have enhanced the accuracy of SDT and mitigated its side effects. Metal-organic frameworks (MOFs) have been receiving substantial consideration for various applications due to their tunable structure and function, large surface area, and highly ordered pores for substrate molecules (Strickland et al., 2015; Huang et al., 2017b; Guo et al., 2017; Simon-Yarza et al., 2017).
It is plausible that approaches that increase production ROS in a controlled manner may serve as alternative strategies to SDT. For example, the Fenton reaction has been used for the production of •OH—one of the strongest oxidants in nature (Burkitt and Gilbert, 1990; Portnow et al., 2009; Rahim Pouran et al., 2014; Hofmans et al., 2016). Therefore, in this work (Figure 1), we report a sonodynamic agent based on MOFs that possess enhanced sonodynamic effects in combination with the Fenton reaction, which highlights the potential clinical application of SDT combined with chemical catalysis in anti-cancer management.
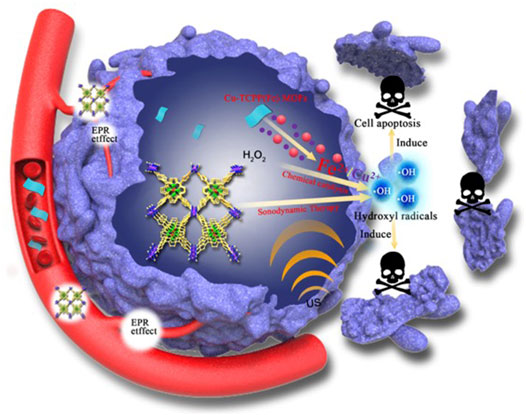
FIGURE 1. Schematic illustration of the synergistic SDT/chemical catalytic-therapeutic mechanism of Cu-TCPP(Fe) MOFs against cancer.
2 Results and Discussion
2.1 Design, Synthesis, and Characterization of Cu-TCPP(Fe)
The application of MOFs, a new type of 2-dimensional (2D) nanomaterial with unique physical and chemical properties (Novoselov et al., 2004; Chhowalla et al., 2013; Pakdel et al., 2014; Ma and Sasaki, 2015; Tan and Zhang, 2015; Liu et al., 2016; Huang et al., 2017b), has been widely explored in many fields, such as hydrogen storage and theranostic nanomedicine (Peng et al., 2014; Rodenas et al., 2015). In this work, water-stable, 2D, metalloporphyrinic MOFs, labeled as Cu-TCPP(Fe), were fabricated by TCPP(Fe) [TCPP = tetrakis (4-carboxyphenyl) porphyrin] as ligands and Cu2(COO)4 paddle-wheel clusters as metal nodes. The four Cu2(COO)4 paddle-wheels were used as the linkage of the 2D “checkboard” motif of TCPP(Fe) for each layered sheet, and the layered sheets were stacked in an AB-packing pattern (Figure 2A). The as-prepared Cu-TCPP(Fe) MOFs were dispersed in water for several weeks in a tightly sealed bottle. Digital photographs of Cu-TCPP(Fe) MOFs dispersed in water with typical Tyndall effects indicated their excellent hydrophilicity and dispersity (Figure 2B). Transmission electron microscopy (TEM) image showed low contrast of the obtained nanosheets with sizes ranging from hundreds of nanometers to a few micrometers (Figure 2C), confirming the ultrathin nature of the Cu-TCPP(Fe) MOFs. The TEM images also displayed typical Cu-TCPP(Fe) MOFs (Figure 2D). The corresponding selected area electron diffraction (SAED) pattern (Figure 2E) also confirmed the basal plane’s hexagonal symmetry structure, demonstrating its highly crystallized structure. The quantitative element analysis was determined by the inductively-coupled plasma optical emission spectroscopy (ICP-OES) (Cu: Fe = 2:1). X-ray diffraction (XRD) patterns exhibited characteristic diffraction peaks of 8.8°, 10.6°, and 19.2°, which were assigned to (110), (001), and (002) (Makiura et al., 2010; Xu et al., 2012), further verifying the crystalline nature of the 2D Cu-TCPP(Fe) nanosheets (Figure 2F). X-ray photoelectron spectroscopy (XPS) analysis was carried out to verify the oxidation states of copper/iron in the Cu-TCPP(Fe) nanosheet. The XPS peaks for the Cu-TCPP(Fe) MOFs showed FeII and its satellite peaks at 707.23 eV and 718.44 eV, and the FeIII peaks at 711.51 eV and 725.76 eV, demonstrating the coexistence of FeII and FeIII in the MOFs (Figure 2G). Deconvolution of the MOFs signal was used to determine the portion of FeII (59.55% and 12.34%) and FeIII (23.76% and 4.35%) in the sample.
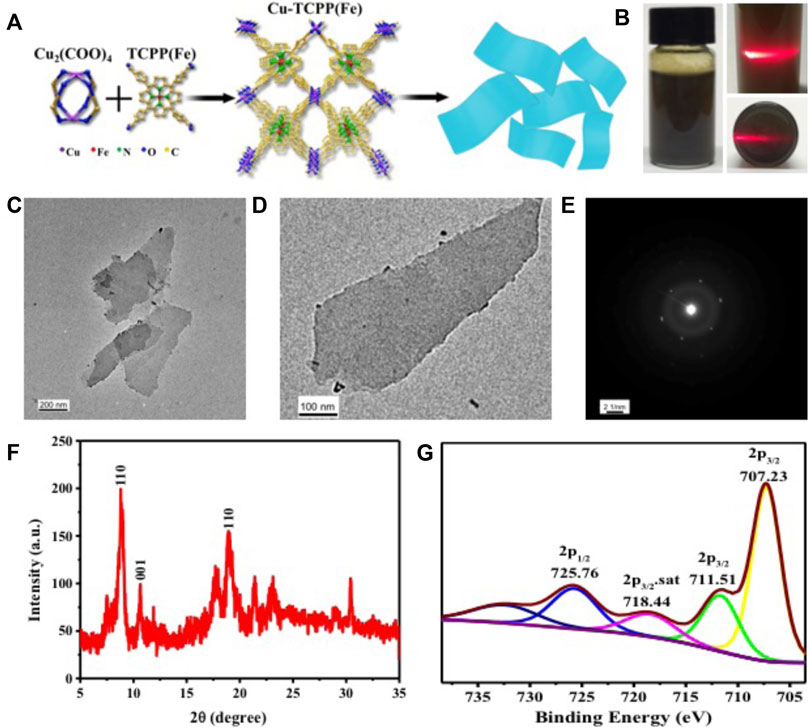
FIGURE 2. Characterization of Cu-TCPP(Fe) MOFs. (A) A schematic showing the synthetic procedure for Cu-TCPP(Fe) MOFs. (B) A digital photograph and (C) TEM image of Cu-TCPP(Fe) MOFs. (D) A TEM image and (E) SAED pattern of a typical Cu-TCPP(Fe) MOF. (F) XRD patterns, and (G) XPS spectra of Cu-TCPP(Fe) MOFs.
To enhance the stability of the Cu-TCPP(Fe) nanosheets in the physiological environment of the human body and lengthen the internal circulation time, their surface was grafted with a biocompatible soybean phospholipid (SP). These surface-modified Cu-TCPP(Fe) nanosheets featured high colloidal stability in physiological solutions, such as Dulbecco’s modified Eagle’s medium (DMEM) and phosphate buffer saline (PBS).
2.2 In vitro Reactive Oxygen Species Generation and Cytotoxicity by Fenton Reaction
We used 3,3′,5,5′-tetramethylbenzidine (TMB), a widely-used substrate for the assay of enzyme mimic catalytic performance (Gao et al., 2007; Chen et al., 2012; Su et al., 2012; An et al., 2013; Liu et al., 2015b; Kwon et al., 2016; Zhang et al., 2016), to assess the peroxidase-like catalytic activity of Cu-TCPP(Fe) and the enhancement of SDT (Figure 3A). The Cu/Fe in Cu-TCPP(Fe) initially catalyzed H2O2 to produce highly toxic hydroxyl radicals (•OH) in the acidic environment (Equation 1), which then oxidized the colorless TMB to form chromogenic oxidized TMB (ox-TMB) with a λmax = 650 nm. The catalytic functionalities and performances of Cu-TCPP(Fe) were explored by plotting typical Michaelis-Menten steady-state kinetics. H2O2 (40, 20, 10, and 5 mM) served as the reactants in the assays of 20 μg/ml Cu-TCPP(Fe) and the trigger of US (1.0 MHz, 1.5 W/cm2, 50% duty cycle, 1 min) implied that SDT could enhance enzyme mimic catalytic performance (Figure 3B). The time-course absorbance upon the addition of H2O2 into the Cu-TCPP(Fe) in sodium citrate buffer solution (pH = 6.0) was plotted, and the corresponding average initial velocities were calculated. Michaelis-Menten steady-state kinetics of Cu-TCPP(Fe) was plotted (Equation 2). All average initial velocities of absorbance changes were then converted as initial velocities (v0) of cation-free radical production or hydroxyl radical formation via the Beer-Lambert law (Equation 3), and then plotted against the corresponding concentration and fitted with Michaelis-Menten curves (Figure 3D). The ox-TMB resulted in the blue color in the solutions (inset in Figure 3C), which showed a maximum absorption peak at 650 nm (Figure 3C), demonstrating that the cascade reaction occurred. A linear double-reciprocal plot (Lineweaver-Burk plot, Equation 4 and Figure 3E) was obtained to determine the Michaelis-Menten constant (KM) and maximum velocity (Vmax). The KM and Vmax values were calculated to be 33.60 mM and 1.2 × 10−3 M/s for Cu-TCPP(Fe).
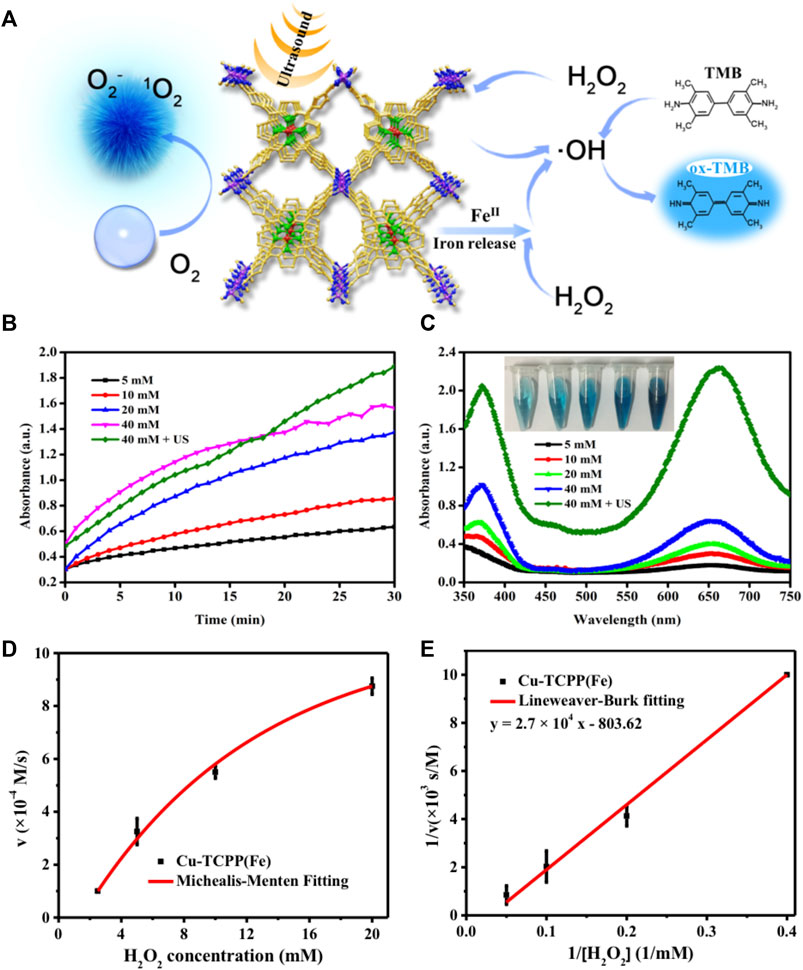
FIGURE 3. In vitro characterizations of the catalytic performance of Cu-TCPP(Fe). (A) Schematic illustration of the mechanism of ROS generation by employing Cu-TCPP(Fe) as sonosensitizer for SDT (left) and chemical catalyze (right). (B) The absorbance of Cu-TCPP(Fe) over time after the addition of varied concentrations of H2O2 (40, 20, 10, and 5 mM) and the trigger of US (1.0 MHz, 1.5 W/cm2, 50% duty cycle, 1 min) at pH = 6.0, with 0.8 mg/ml TMB and 20 μg/ml Cu-TCPP(Fe). (C) Absorbance spectra changes (λmax = 650 nm) at the end of the reaction (inset shows the photographs of ox-TMB). (D) Michaelis-Menten kinetics and (E) Lineweaver-Burk plotting of Cu-TCPP(Fe).
The methylene blue (MB) dye test (Figure 5A) was performed to determine the presence of hydroxyl radicals generated by the Fenton reaction (Satoh et al., 2007; Zhang et al., 2015). •OH radicals are the primary active substances for MB bleaching in the traditional Fe2+/H2O2 system. Importantly, the added H2O2 did not cause obvious MB bleaching (Figure 4C) as compared to the MB group (Figure 4B), whereas the MB with the H2O2 group was homogeneously dark blue. The introduction of Cu-TCPP(Fe) cause obvious MB bleaching (Figure 4D) and adding US (1.0 MHz, 1.5 W/cm2, 50% duty cycle, 1 min) implied that SDT could enhance the Fenton reaction (Figure 4E). The bleaching of MB in the Cu2+/Fe2+/H2O2 system can be divided into two stages: the short initial stage and the long deceleration stage. In the short initial stage, the •OH generation rate was rapid, which led to a rapid drop in MB concentration (Figure 4F). However, after H2O2 and Cu2+/Fe2+ were rapidly consumed during the short initial stage, the bleaching reaction entered the long deceleration stage. The bleaching of MB resulted in the pale blue color in the solutions (inset in Figure 4F), which showed a maximum absorption peak at 664 nm.
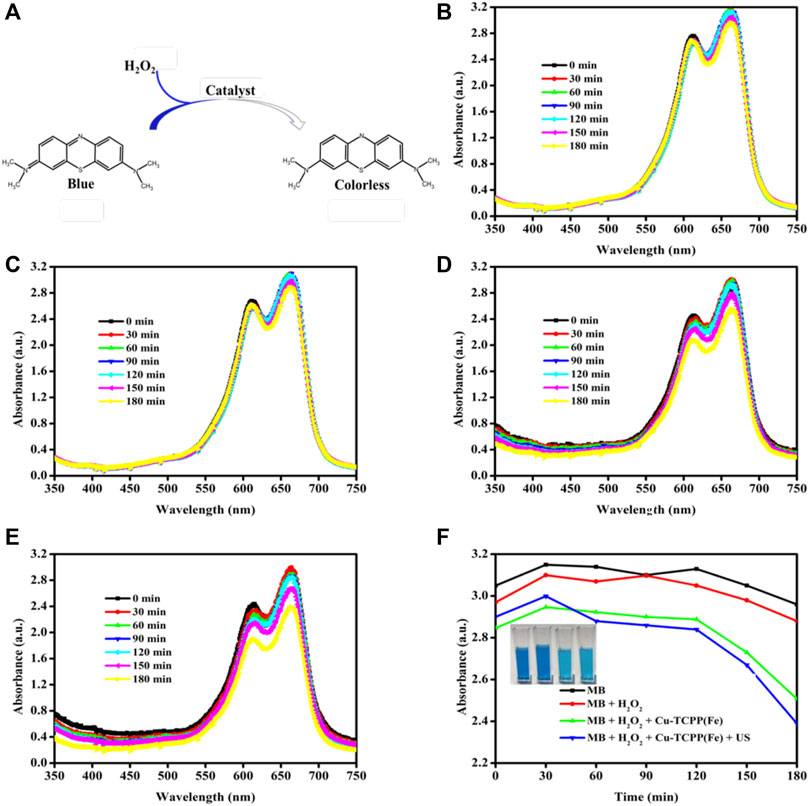
FIGURE 4. MB bleaching test. (A) The sketch map of MB bleaching. MB absorbance spectra changes (λmax = 664 nm) at different time points (0, 30, 60, 90, 120, 150, and 180 min) after initiation of the Fenton reaction at (B) pH = 6.0, (C) pH = 6.0 + 40 mM H2O2, (D) pH = 6.0 + 40 mM H2O2 + 40 μg/ml Cu-TCPP(Fe) and (E) pH = 6.0 + 40 mM H2O2 + 40 μg/ml Cu-TCPP(Fe) + US (1.0 MHz, 1.5 W/cm2, 50% duty cycle, 1 min). (F) Comparison of ROS production in different treatments as determined by MB absorption.
2.3 Intracellular Biodegradation of Cu-TCPP(Fe)
Bio-TEM was employed to perceive the underlying evolution of Cu-TCPP(Fe) after co-culture with 4T1 cells over time [(Chu and Dupuy, 2014; Liu et al., 2015a; Pelaz et al., 2017; Siegel et al., 2021) d] (Figure 5A). The Cu-TCPP(Fe) was endocytosed well after 1 d co-incubation. Subsequently, some nanoparticles were degraded and excreted from the cells. As co-incubation continued, an increasing amount of nanoparticles were degraded and barely any nanoparticles were observed after 7 d.
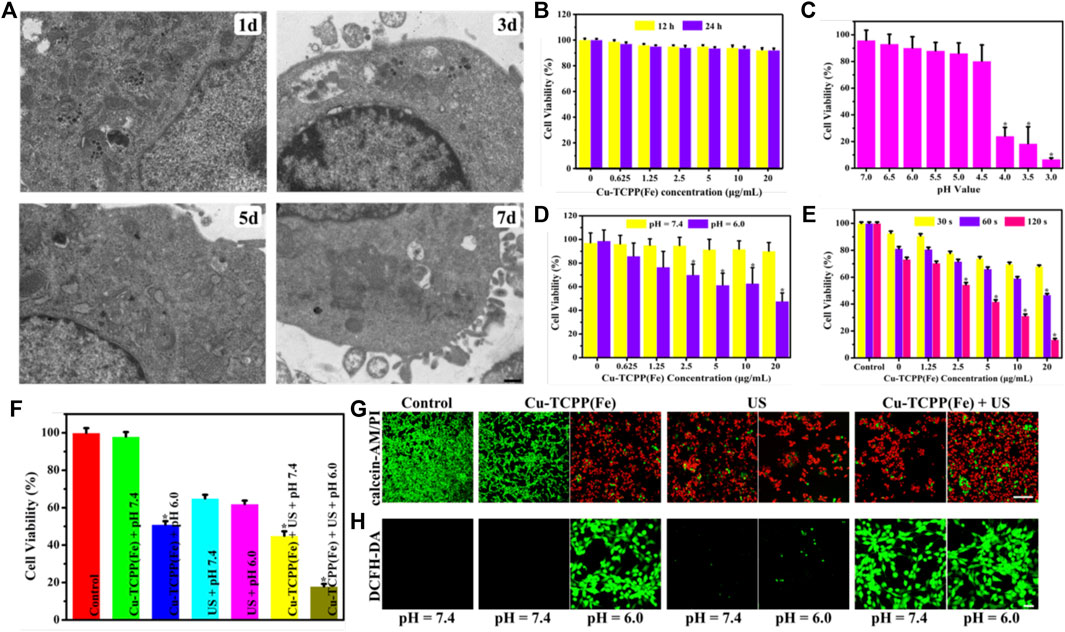
FIGURE 5. In vitro SDT/chemical catalysis therapy for cancer. (A) Bio-TEM images for the intracellular biodegradation behavior of Cu-TCPP(Fe) in 4T1 cells after co-incubation over time (Chu and Dupuy, 2014;Liu et al., 2015a; Pelaz et al., 2017; Siegel et al., 2021) (D). (B) Viability of 4T1 cells after incubation with various concentrations of Cu-TCPP(Fe) for 12 and 24 h. (C) Viability of 4T1 cells after incubation at various pH values (7.0, 6.5, 6.0, 5.5, 5.0, 4.5, 4.0, 3.5 and 3.0) for 12 h. (D) Cytotoxicity profiles of 4T1 cells treated with Cu-TCPP(Fe) under neutral (pH = 7.4) and acidic (pH = 6.0) conditions. (E) Viability of 4T1 cells after exposure to US irradiation (1.0 MHz, 1.5 W/cm2, 50% duty cycle) over time (30, 60, and 120 s) at various Cu-TCPP(Fe) concentrations (20, 10, 5, 2.5, 1.25 μg/ml). (F) Relative viabilities of 4T1 cells after different treatments, including control (without any treatment), Cu-TCPP(Fe) at pH = 7.4/6.0, US irradiation at pH = 7.4/6.0, and Cu-TCPP(Fe) combined with US irradiation at pH = 7.4/6.0. CLSM images of 4T1 cells stained with calcein AM/PI solution (G) and the ROS fluorescent probe DCFH-DA (H) after different treatments (scale bar: 50 μm). *p < 0.05, calculated by one-way ANOVA test.
2.4 In vitro Sonodynamic Therapy/Chemical Catalysis Therapy for Cancer
The above-mentioned in vitro research was shown to yield a substantial amount of hydroxyl radicals by Cu-TCPP(Fe). The CCK-8 assay was used to define the inherent toxicity of Cu-TCPP(Fe) by using 4T1 cells. Furthermore, 4T1 cells were initially co-cultured with Cu-TCPP(Fe) at various concentrations (20, 10, 5, 2.5, 1.25, and 0.625 μg/ml) for 12 and 24 h. No obvious cytotoxicity of Cu-TCPP(Fe) nanocomposites was observed (Figure 5B). 4T1 cells were then incubated at different pH values (7.0, 6.5, 6.0, 5.5, 5.0, 4.5, 4.0, 3.5, and 3.0) for 12 h. No obvious cytotoxicity was observed at pH values between 5.0–7.0 (Figure 5C). To observe the cytotoxicity of the Fenton reaction of Cu-TCPP(Fe), 4T1 cells were then incubated with Cu-TCPP(Fe) at various concentrations (20, 10, 5, 2.5, 1.25, and 0.625 μg/ml) under acidic (pH = 6.0) and neutral (pH = 7.4) culture mediums for 4 h. The Cu-TCPP(Fe) present 47.76, 62.87, 61.46, 70.12, 76.66, 85.94, 91.91, 91.55, 94.94, 95.21, 96.25% cell viabilities at descendent concentrations under the acidic (pH = 6.0) and neutral (pH = 7.4) condition, respectively. This confirmed that the cell viability is dependent upon the quantity of Cu-TCPP(Fe) and the pH value (Figure 5D). Concurrently, a CCK-8 assay was also used to define the toxicity of Cu-TCPP(Fe) as a sonosensitizer. Cu-TCPP(Fe) alone exhibits negligible cytotoxicity, whereas Cu-TCPP(Fe) combined with SDT significantly improved the treatment efficiency, inhibited cell proliferation, and induced cell death. These effects were Cu-TCPP(Fe) concentration- and US irradiation time (30, 60, and 120 s)-dependent (Figure 5E).
To validate the synergistic effect induced by SDT and the Fenton reaction of Cu-TCPP(Fe), a CCK-8 assay, calcein acetoxymethyl ester (calcein-AM)/propidium iodide (PI) solution stain test, and 2′-7′-dichlorofluorescein diacetate (DCFH-DA) test were conducted. For the CCK-8 assay, 4T1 cells were incubated with Cu-TCPP(Fe) at 20 μg/ml for 4 h at a pH = 7.4 or pH = 6.0, with or without US (1.0 MHz, 1.5 W/cm2, 50% duty cycle, 1 min) irradiation. As revealed in Figure 5F, the cells were largely eradicated by US irradiation after co-incubation with Cu-TCPP(Fe) under acid conditions (pH = 6.0). The viability of cancer cells by Cu-TCPP(Fe) combined with US irradiation under acidic conditions decreased to nearly 18%, which was much lower as compared to neutral conditions at the same concentration (20 μg/ml) under the same US irradiation conditions. The drastically enhanced sonotoxicity of Cu-TCPP(Fe) under acidic conditions was ascribed to the immense production of ROS enhanced by the Fenton reaction. To visually observe the cells, 4T1 cells were stained with calcein-AM/PI solution after co-culturing with 20 μg/ml Cu-TCPP(Fe) at pH = 7.4 or pH = 6.0 conditions for 4 h. The viable cells emitted green fluorescence, while the dead cells emitted red fluorescence as witnessed by confocal laser scanning microscope (CLSM) (Figure 5G). The CLSM showed that the 4T1 cells were impaired at pH = 7.4. A vast amount of dead cells were observed at pH = 6.0. These results were consistent with the cytotoxicity profiles of Cu-TCPP(Fe), which was assumed to be initiated by the toxic hydroxyl radicals produced in situ by Cu-TCPP(Fe).
To unveil the intracellular mechanism of Cu-TCPP(Fe) as sonosensitizers that kill tumor cells, DCFH-DA was used to show that the ROS levels within the cell (Figure 5H). Cu-TCPP(Fe) + US irradiation (1.0 MHz, 1.5 W/cm2, 50% duty cycle, 60 s) induced substantial intracellular ROS production, as manifested by the production of considerable green fluorescence in tumor cells. The control, as well as the US and Cu-TCPP(Fe) pH = 7.4 group, displayed a low level of green fluorescence, indicating the increased production of ROS through the sonodynamic method combined with Cu-TCPP(Fe).
2.5 The in vivo Synergistic Effects of Sonodynamic Therapy and Chemical Catalysis of Cu-TCPP(Fe)
Encouraged by the excellent synergy of SDT and the chemical catalysis of Cu-TCPP(Fe), the in vivo synergistic effects of this combination were further assessed by using intravenous administration of the Cu-TCPP(Fe) into 4T1 tumor-bearing mice followed by various treatments. Several evaluations were carried out to estimate the in vivo biocompatibility of Cu-TCPP(Fe) before subsequent in vivo therapeutic evaluation. Healthy Kunming mice (n = 5) were administrated with Cu-TCPP(Fe) (0, 5, 10, and 20 mg/kg) via tail vein injections. No obvious major organ damage was detected one-month post-injection, as confirmed by body weight (Figure 6A), hematology (Figures 7A–D), serum biochemistry (Figures 7E,F), and histological analyses (Figure 6C). Then 20 tumor-bearing mice were randomly divided into four groups (n = 5 per group), including the control group (treated with saline), US group, Cu-TCPP(Fe) group, and Cu-TCPP(Fe) + US group. The US irradiation groups were treated with US irradiation on the first and fifth day. The body weight and tumor volume of the four groups were recorded every 2 d (Figures 6B,D). The tumor-bearing mice in the control and US group experienced rapid tumor growth, while the tumor growth was significantly inhibited in the Cu-TCPP(Fe) and Cu-TCPP(Fe) + US group, ultimately demonstrating the therapeutic efficacy of SDT and its synergetic effect with the chemical catalysis (Figure 6E). The tumor inhibition rate in the Cu-TCPP(Fe) group and Cu-TCPP(Fe) + US group reached 52.2% and 58.8%, respectively (Figure 6F). At the end of the therapeutic treatment, the tumors in the mice of all groups were imaged, dissected, and compared (Figure 6G). We found that 4T1 tumor growth was effectively inhibited after the intravenous administration of Cu-TCPP(Fe). To further study the synergistic effect of various treatments, H&E, TUNEL, and Ki-67 antibody staining were performed on tumor sections from all groups of mice at 24 h post-treatment (Figure 6H). Additionally, H&E and TUNEL results showed that a large number of dead cells were observed in the tumor tissue. The Cu-TCPP(Fe) alone and combined with US exhibited greater necrosis and apoptosis rates as compared to the control and US group. The Ki-67 results of each group were in agreement with the H&E and TUNEL staining results, confirming the efficacy of SDT and its synergistic effects with chemical catalysis. Furthermore, there was no noticeable adverse effects or organ damage to the heart, liver, spleen, lung, and kidney in the treatment groups (Figure 8).
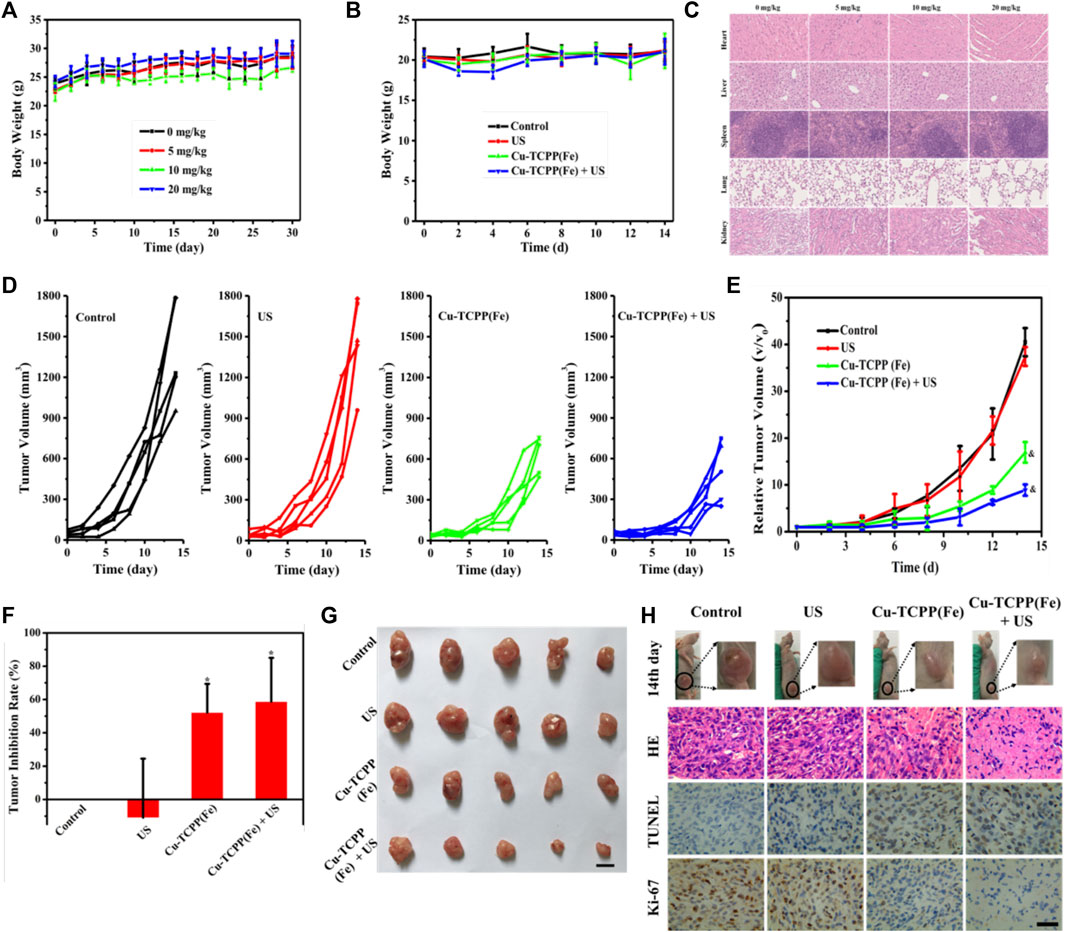
FIGURE 6. In vivo synergistic SDT/chemical catalysis for tumor suppression. (A) The bodyweight of mouse changes in the biocompatibility evaluation. (B) Bodyweight changes of 4T1 tumor-bearing mice in different groups. (C) H&E-stained histological images of organs in the biocompatibility evaluation; scale bar: 50 μm. Time-dependent tumor-growth curves (D), relative tumor volume (E), and tumor inhibition rate (F) of 4T1 tumor-bearing mice in four experimental groups (n = 5), including the control group, US group, Cu-TCPP(Fe) group, and Cu-TCPP(Fe) + US group. (G) Photographs of the dissected tumors from four groups after 2 weeks of treatment (scale bar: 10 mm). (H) H&E staining, TUNEL staining, and Ki-67 immunohistochemical staining of tumor tissue from each group after different treatments (scale bar: 50 μm). &p < 0.05, calculated by repeated measure ANOVA test. *p < 0.05, calculated by one-way ANOVA test.
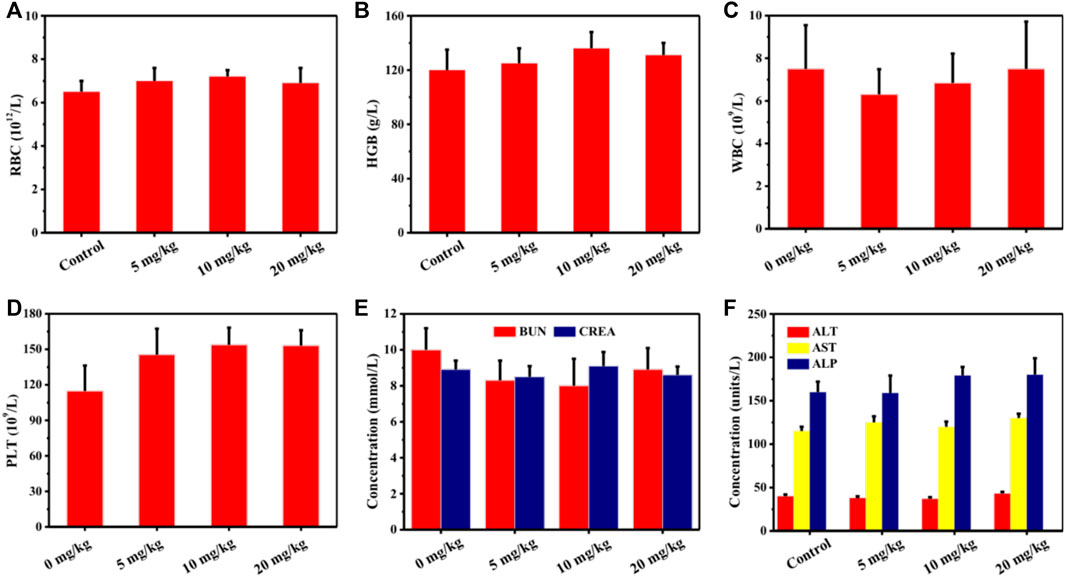
FIGURE 7. Blood routine examination and serum biochemical levels of mice treated with 0 mg/kg, 5 mg/kg, 10 mg/kg, and 20 mg/kg of Cu-TCPP(Fe). (A) Red blood cell (RBC), (B) Hemoglobin (HGB), (C) White blood cell (WBC), (D) Platelet (PLT), (E) Blood urea nitrogen (BUN) and creatinine (CREA), (F) Alanine transaminase (ALT), aspartate aminotransferase (AST) and alkaline phosphatase (ALP) levels of mice in all groups.
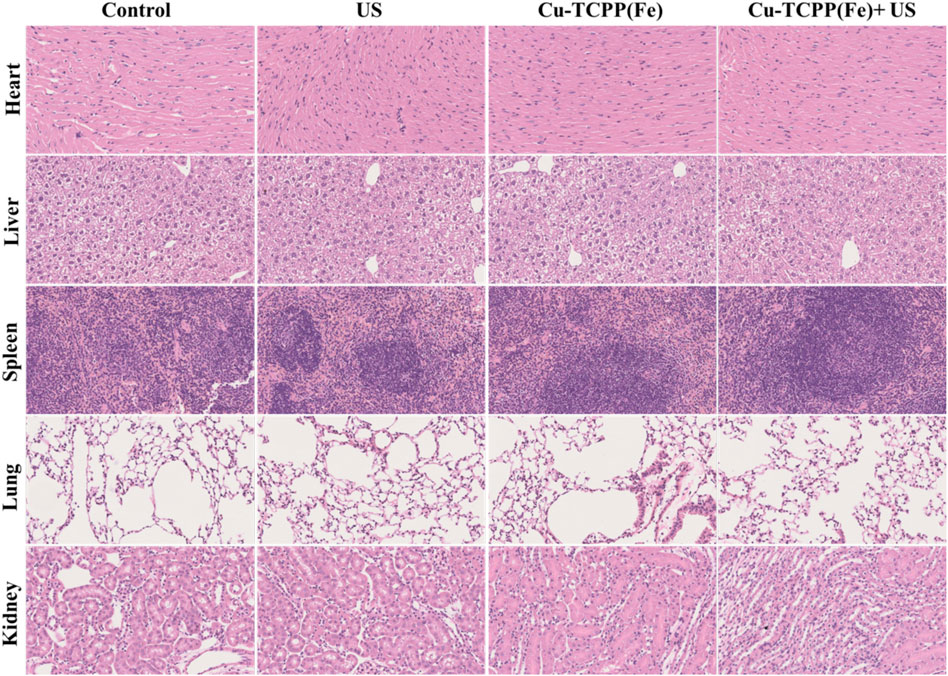
FIGURE 8. Histopathological examinations of major organs (heart, liver, spleen, lung, and kidney) from mice after different treatments. Scale bar: 50 μm.
3 Experimental Section
3.1 Materials and Reagents
Copper nitrate trihydrate [Cu(NO3)2·3H2O, 99%], N,N-dimethylformamide (DMF, 99.8%), hydrogen peroxide (H2O2, 30 wt%), soybean phospholipid (SP), and 3,3′,5,5′-tetramethyl-benzidine (TMB, 99%) were purchased from Sigma-Aldrich (Shanghai, China). 3,7-bis(dimethylamino)-phenothiazin-5-ium chloride (methylene blue dye, MB, 97%) was purchased from Fluka (Buchs, Switzerland). Fe(III) meso-tetra(4-carboxyphenyl)porphine chloride [TCPP(Fe), 97%] was purchased from J&K Chemical Ltd. (Shanghai, China). Ethanol (99.9%) was purchased from Merck (Nordic European Centre, Singapore). Trifluoroacetic acid (CF3COOH, 99%) was purchased from Alfa Aesar (Haverhill, Massachusetts, United States). 2′-7′-dichlorofluorescein diacetate (DCFH-DA) was bought from Beyotime Institute of Biotechnology. Calcein acetoxymethyl ester (calcein-AM) and propidium iodide (PI) were purchased from Thermo Fisher Scientific Inc. Dulbecco’s Modified Eagle Medium (DMEM), penicillin-streptomycin solution, fetal bovine serum (FBS), trypsin-EDTA solution, and phosphate buffer saline (PBS) were purchased from Gibco (AG, United States). All chemicals were used as received without further purification, and their aqueous solutions were prepared using deionized water.
3.2 Synthesis of 2D Cu-TCPP(Fe) Metal-Organic Frameworks
A mixture of Cu(NO3)2·3H2O (4.8 mg, 0.02 mmol), TCPP(Fe) (8.8 mg, 0.01 mmol), CF3COOH (20 μL, 1.0 M), DMF (1.5 ml), and ethanol (0.5 ml) was added into a small, capped vial, which was then heated to 80°C and kept for 24 h. The resulting dark-brown crystals were washed with ethanol and re-dispersed in ethanol.
The as-prepared Cu-TCPP(Fe) MOFs are hydrophilic and cannot be used for future biological applications. To solve this problem, a “thin-film” approach was used to produce SP-encapsulated Cu-TCPP(Fe) MOFs [Cu-TCPP(Fe)-SP]. Typically, 2 ml of Cu-TCPP(Fe) MOFs in ethanol solution (1 mg/ml) was added into 10 ml of SP in chloroform solution (1 mg/ml) and sonicated for 5 min. The mixture was incubated in a vacuum rotary evaporator at 60°C for 30 min to evaporate the solvent. Subsequently, 10 ml of PBS was added into the lipidic film and then sonicated for 10 min.
3.3 Characterization
Transmission electron microscopy (TEM) images and energy-dispersive X-ray spectra (EDS) were acquired using a JEM-2100F transmission electron microscope operated at 200 kV. X-ray diffraction (XRD) was performed on a Rigaku D/MAX-2200 PC XRD system with Cu Kα radiation (λ = 1.54 Å) at 40 kV and 40 mA. Scanning electron microscopy (SEM) images and element mapping were captured by FEI Magellan 400 scanning electron microscope (FEI Company, United States). XPS spectra were recorded by ESCAlab250 (Thermal Scientific). The CLSM images were measured on an FV1000 (Olympus Company, Japan). The optical absorbance spectra were conducted on a Molecular Device SpectraMax M2. The concentrations of copper and iron were measured by using inductively coupled plasma optical emission spectrometry (ICP-OES, OPTIMA 7000DV).
3.4 Cell Culture and Animals
Mouse breast cancer cell 4T1 cells were used in all of these experiments, which were cultured in high glucose DMEM media supplemented with 10% FBS and 1% penicillin-streptomycin. The cells were maintained at 37°C in a humidified incubator with 5% CO2.
Healthy female Kunming mice (18–22 g, 6–8 weeks) and female BALB/c nude mice (18–22 g, 6–8 weeks) were obtained from Beijing Vital River Laboratory Animal Technology Co., Ltd. and raised at the Laboratory Animal Center at Shanghai Medical College of Fudan University. All animal experiments were conducted in agreement with the guidelines of the Regional Ethics Committee for Animal Experiments, and the care regulations were approved by the Administrative Committee of Laboratory Animals of Fudan University.
3.5 In vitro Reactive Oxygen Species Generation by Sonodynamic Therapy
The cells were co-incubated with Cu-TCPP(Fe) (1 ml, 20 μg/ml in DMEM) at 37°C for 4 h. Then, the cells were incubated for another 4 h after being treated by US irradiation (1.0 MHz, 1.5 W/cm2, 50% duty cycle, 1 min). The cells were washed with PBS three times after staining for 15 min with DCFH-DA (100 μL, 1 μL/9 μL in DMEM), and finally observed by CLSM.
3.6 CLSM Evaluation of the Sonotoxicity of Cu-TCPP(Fe)
The cell processing procedures were similar to those in ROS generation mentioned above; however, the culture medium was replaced with Calcein-AM (100 μL, 20 μM) and PI (100 μL, 20 μM) to stain the live and dead cells, respectively.
3.7 In vitro Sonodynamic Therapy Efficacy of Cu-TCPP(Fe) Against Tumor Cells
4T1 cells were cultured and seeded in 96-well plates at a density of 1 × 104 cells per well for 12 h to allow them to stick to the plate. Then, Cu-TCPP(Fe) (20, 10, 5, 2.5, 1.25, and 0.625 μg/ml) was added to the well and co-incubated for another 4 h. Then the cells were treated with US irradiation (1.0 MHz, 1.5 W/cm2, 50% duty cycle) for 30, 90, and 120 s. CCK-8 (100 μL, VCCK-8: VDMEM = 1: 9) was then used to examine the cell inhibition rate on a microplate reader at the wavelength 450 nm after 90 min.
3.8 Michaelis-Menten Kinetics
TMB (0.8 mg/ml) was applied to monitor the chromogenic reaction (λ = 650 nm) of 20 μg/ml Cu-TCPP(Fe) upon addition of various concentrations of H2O2 (40, 20, 10, and 5 mM). The trigger of US (1.0 MHz, 1.5 W/cm2, 50% duty cycle, 1 min) was applied to verify the enhancement of SDT. Sodium citrate buffer solution (pH = 6.0) was used to prepare the above reagents. The Michaelis-Menten kinetic curve of Cu-TCPP(Fe) was acquired by plotting the respective initial velocities against H2O2 concentrations. The Michaelis-Menten constant (KM) and maximal velocity (Vmax) were calculated via the Lineweaver-Burk plotting.
3.9 Bleaching of MB by Reactive Oxygen Species
MB (200 μL) was used to monitor the chromogenic reaction (λ = 664 nm) of 40 μg/ml Cu-TCPP(Fe) upon addition of 40 mM H2O2. Sodium citrate buffer solution (pH = 6.0) was used to bring the final volume to 3 ml. Further, 200 μL MB and 40 mM H2O2 were added into 3 ml of sodium citrate buffer solution (pH = 6.0) to observe the bleaching ability of H2O2. The trigger of US (1.0 MHz, 1.5 W/cm2, 50% duty cycle, 1 min) was applied to verify the enhancement of SDT. MB (200 μL) in 3 ml of sodium citrate buffer solution (pH = 6.0) was set as the control. The spectrum of MB in the different groups was acquired at different time points (5, 15, 30, 60, 90, 120, 150, and 180 min).
3.10 In vivo Sonodynamic Therapy Efficiency of Cu-TCPP(Fe) Against Tumor Growth
A total of 20 female BALB/c nude mice were subcutaneously injected with 4T1 cells (1 × 106 cells/mouse) to establish the tumor model. When the tumor volume reached 40 mm3, the mice were divided into 4 groups (n = 5 in each group): 1) control group, 2) US group, 3) Cu-TCPP(Fe) group, and 4) Cu-TCPP(Fe) + US group. The mice in the Cu-TCPP(Fe) + US group and Cu-TCPP(Fe) group were intravenously injected with Cu-TCPP(Fe) (10 mg/kg). The mice in the Cu-TCPP(Fe) + US group and US group were treated with US irradiation (1.0 MHz, 2.3 W/cm2, 50% duty cycle, 5 min) after injection of Cu-TCPP(Fe) for days 1 and 5. The tumor volume was calculated as follows, V = (ab2)/2, where a and b refer to the largest length and width of the tumor, respectively. The tumor volume was recorded and photographed every 2 d. At the end of the treatment, the tumor was cut and stained by H&E, TUNEL, and Ki-67 for histological analysis.
4 Conclusion
A concept of SDT combined with chemical catalysis has been introduced into the field of nanomedicine to effectively inhibit tumor growth by Cu-TCPP(Fe) MOFs with excellent biodegradability and biocompatibility. The porphyrin structure in Cu-TCPP(Fe) is effectively triggered by US, which results in a microenvironment of H2O2 and acid within the tumor tissue. This microenvironment is subsequently used in Fe-based chemo-Fenton reactions, which results in increased levels of toxic hydroxyl radicals that induce tumor cell apoptosis and death. This work showed that introducing well-designed nanocatalysts into tumor tissue could trigger a specific sequence of specific TME responses that inhibit tumor growth. Thus, we provided here a promising strategy for tumor therapy that could greatly enhance tumor specificity and reduce side effects on normal tissue.
Data Availability Statement
The original contributions presented in the study are included in the article/Supplementary Material, further inquiries can be directed to the corresponding author.
Ethics Statement
The animal study was reviewed and approved by Administrative Committee of Laboratory Animals of Fudan University.
Author Contributions
HZ, YL, and CC conceived the study and participated in the experiment design. HZ and YL performed the experiment. JL provided experimental technical guidance. HZ and YL completed figures construction and manuscript writing. CC checked and revised the manuscript. All authors discussed the results and approved the final version.
Funding
This work was supported by the National Nature Science Foundation of China (Grant No. 81901749, 81602262 and 81801701) and the Shanghai Sailing Program (Grant No. 19YF1410000).
Conflict of Interest
The authors declare that the research was conducted in the absence of any commercial or financial relationships that could be construed as a potential conflict of interest.
Publisher’s Note
All claims expressed in this article are solely those of the authors and do not necessarily represent those of their affiliated organizations, or those of the publisher, the editors and the reviewers. Any product that may be evaluated in this article, or claim that may be made by its manufacturer, is not guaranteed or endorsed by the publisher.
Supplementary Material
The Supplementary Material for this article can be found online at: https://www.frontiersin.org/articles/10.3389/fmats.2022.908789/full#supplementary-material
References
Allison, R. R., and Sibata, C. H. (2010). Oncologic Photodynamic Therapy Photosensitizers: a Clinical Review. Photodiagnosis Photodyn. Ther. 7 (2), 61–75. doi:10.1016/j.pdpdt.2010.02.001
An, Q., Sun, C., Li, D., Xu, K., Guo, J., and Wang, C. (2013). Peroxidase-like Activity of Fe3O4@carbon Nanoparticles Enhances Ascorbic Acid-Induced Oxidative Stress and Selective Damage to PC-3 Prostate Cancer Cells. ACS Appl. Mat. Interfaces 5 (24), 13248–13257. doi:10.1021/am4042367
Burkitt, M. J., and Gilbert, B. C. (1990). Model Studies of the Iron-Catalysed Haber-Weiss Cycle and the Ascorbate-Driven Fenton Reaction. Free Radic. Res. Commun. 10 (4-5), 265–280. doi:10.3109/10715769009149895
Carmeliet, P., and Jain, R. K. (2000). Angiogenesis in Cancer and Other Diseases. Nature 407 (6801), 249–257. doi:10.1038/35025220
Castano, A. P., Mroz, P., and Hamblin, M. R. (2006). Photodynamic Therapy and Anti-tumour Immunity. Nat. Rev. Cancer 6 (7), 535–545. doi:10.1038/nrc1894
Chen, Z., Yin, J.-J., Zhou, Y.-T., Zhang, Y., Song, L., Song, M., et al. (2012). Dual Enzyme-like Activities of Iron Oxide Nanoparticles and Their Implication for Diminishing Cytotoxicity. ACS Nano 6 (5), 4001–4012. doi:10.1021/nn300291r
Cheng, L., Liu, J., Gu, X., Gong, H., Shi, X., Liu, T., et al. (2014). PEGylated WS2Nanosheets as a Multifunctional Theranostic Agent for In Vivo Dual-Modal CT/Photoacoustic Imaging Guided Photothermal Therapy. Adv. Mat. 26 (12), 1886–1893. doi:10.1002/adma.201304497
Chhowalla, M., Shin, H. S., Eda, G., Li, L.-J., Loh, K. P., and Zhang, H. (2013). The Chemistry of Two-Dimensional Layered Transition Metal Dichalcogenide Nanosheets. Nat. Chem. 5 (4), 263–275. doi:10.1038/nchem.1589
Chu, K. F., and Dupuy, D. E. (2014). Thermal Ablation of Tumours: Biological Mechanisms and Advances in Therapy. Nat. Rev. Cancer 14 (3), 199–208. doi:10.1038/nrc3672
Coussens, L. M., and Werb, Z. (2002). Inflammation and Cancer. Nature 420 (6917), 860–867. doi:10.1038/nature01322
DeBerardinis, R. J., and Chandel, N. S. (2016). Fundamentals of Cancer Metabolism. Sci. Adv. 2 (5), e1600200. doi:10.1126/sciadv.1600200
Deepagan, V. G., You, D. G., Um, W., Ko, H., Kwon, S., Choi, K. Y., et al. (2016). Long-Circulating Au-TiO2 Nanocomposite as a Sonosensitizer for ROS-Mediated Eradication of Cancer. Nano Lett. 16, 6257–6264. doi:10.1021/acs.nanolett.6b02547
Dolmans, D. E. J. G. J., Fukumura, D., and Jain, R. K. (2003). Photodynamic Therapy for Cancer. Nat. Rev. Cancer 3 (5), 380–387. doi:10.1038/nrc1071
Firczuk, M., Nowis, D., and Gołąb, J. (2011). PDT-induced Inflammatory and Host Responses. Photochem. Photobiol. Sci. 10 (5), 653–663. doi:10.1039/c0pp00308e
Galadari, S., Rahman, A., Pallichankandy, S., and Thayyullathil, F. (2017). Reactive Oxygen Species and Cancer Paradox: To Promote or to Suppress? Free Radic. Biol. Med. 104, 144–164. doi:10.1016/j.freeradbiomed.2017.01.004
Gao, L., Zhuang, J., Nie, L., Zhang, J., Zhang, Y., Gu, N., et al. (2007). Intrinsic Peroxidase-like Activity of Ferromagnetic Nanoparticles. Nat. Nanotech 2 (9), 577–583. doi:10.1038/nnano.2007.260
Guo, W., Xia, W., Cai, K., Wu, Y., Qiu, B., Liang, Z., et al. (2017). Kinetic-Controlled Formation of Bimetallic Metal-Organic Framework Hybrid Structures. Small 13 (41), 2049. doi:10.1002/smll.201702049
Hanahan, D., and Weinberg, R. A. (2011). Hallmarks of Cancer: the Next Generation. Cell 144 (5), 646–674. doi:10.1016/j.cell.2011.02.013
Harada, Y., Ogawa, K., Irie, Y., Endo, H., Feril, L. B., Uemura, T., et al. (2011). Ultrasound Activation of TiO2 in Melanoma Tumors. J. Control. Release 149 (2), 190–195. doi:10.1016/j.jconrel.2010.10.012
Hofmans, S., Berghe, T. V., Devisscher, L., Hassannia, B., Lyssens, S., Joossens, J., et al. (2016). Novel Ferroptosis Inhibitors with Improved Potency and ADME Properties. J. Med. Chem. 59 (5), 2041–2053. doi:10.1021/acs.jmedchem.5b01641
Hornsveld, M., and Dansen, T. B. (2016). The Hallmarks of Cancer from a Redox Perspective. Antioxidants Redox Signal. 25 (6), 300–325. doi:10.1089/ars.2015.6580
Huang, P., Qian, X., Chen, Y., Yu, L., Lin, H., Wang, L., et al. (2017). Metalloporphyrin-Encapsulated Biodegradable Nanosystems for Highly Efficient Magnetic Resonance Imaging-Guided Sonodynamic Cancer Therapy. J. Am. Chem. Soc. 139 (3), 1275–1284. doi:10.1021/jacs.6b11846
Huang, Y., Zhao, M., Han, S., Lai, Z., Yang, J., Tan, C., et al. (2017). Growth of Au Nanoparticles on 2D Metalloporphyrinic Metal-Organic Framework Nanosheets Used as Biomimetic Catalysts for Cascade Reactions. Adv. Mater 29 (32), 102. doi:10.1002/adma.201700102
Kou, J. Y., Li, Y., Zhong, Z. Y., Jiang, Y. Q., Li, X. S., Han, X. B., et al. (2017). Berberine-sonodynamic Therapy Induces Autophagy and Lipid Unloading in Macrophage. Cell Death Dis. 8 (1), e2558. doi:10.1038/cddis.2016.354
Kwon, B., Han, E., Yang, W., Cho, W., Yoo, W., Hwang, J., et al. (2016). Nano-Fenton Reactors as a New Class of Oxidative Stress Amplifying Anticancer Therapeutic Agents. ACS Appl. Mat. Interfaces 8 (9), 5887–5897. doi:10.1021/acsami.5b12523
Landry, W. D., and Cotter, T. G. (2014). ROS Signalling, NADPH Oxidases and Cancer. Biochem. Soc. Trans. 42 (4), 934–938. doi:10.1042/bst20140060
Li, W.-P., Su, C.-H., Chang, Y.-C., Lin, Y.-J., and Yeh, C.-S. (2016). Ultrasound-Induced Reactive Oxygen Species Mediated Therapy and Imaging Using a Fenton Reaction Activable Polymersome. ACS Nano 10 (2), 2017–2027. doi:10.1021/acsnano.5b06175
Liu, J., Wang, H., and Antonietti, M. (2016). Graphitic Carbon Nitride "reloaded": Emerging Applications beyond (Photo)catalysis. Chem. Soc. Rev. 45 (8), 2308–2326. doi:10.1039/c5cs00767d
Liu, Y., He, J., Yang, K., Yi, C., Liu, Y., Nie, L., et al. (2015). Folding up of Gold Nanoparticle Strings into Plasmonic Vesicles for Enhanced Photoacoustic Imaging. Angew. Chem. Int. Ed. 54 (52), 15809–15812. doi:10.1002/anie.201508616
Liu, Y., Purich, D. L., Wu, C., Wu, Y., Chen, T., Cui, C., et al. (2015). Ionic Functionalization of Hydrophobic Colloidal Nanoparticles to Form Ionic Nanoparticles with Enzymelike Properties. J. Am. Chem. Soc. 137 (47), 14952–14958. doi:10.1021/jacs.5b08533
Long, D., Liu, T., Tan, L., Shi, H., Liang, P., Tang, S., et al. (2016). Multisynergistic Platform for Tumor Therapy by Mild Microwave Irradiation-Activated Chemotherapy and Enhanced Ablation. ACS Nano 10, 9516–9528. doi:10.1021/acsnano.6b04749
Ma, R., and Sasaki, T. (2015). Two-dimensional Oxide and Hydroxide Nanosheets: Controllable High-Quality Exfoliation, Molecular Assembly, and Exploration of Functionality. Acc. Chem. Res. 48 (1), 136–143. doi:10.1021/ar500311w
Makiura, R., Motoyama, S., Umemura, Y., Yamanaka, H., Sakata, O., and Kitagawa, H. (2010). Surface Nano-Architecture of a Metal-Organic Framework. Nat. Mater 9 (7), 565–571. doi:10.1038/nmat2769
McEwan, C., Kamila, S., Owen, J., Nesbitt, H., Callan, B., Borden, M., et al. (2016). Combined Sonodynamic and Antimetabolite Therapy for the Improved Treatment of Pancreatic Cancer Using Oxygen Loaded Microbubbles as a Delivery Vehicle. Biomaterials 80, 20–32. doi:10.1016/j.biomaterials.2015.11.033
Novoselov, K. S., Geim, A. K., Morozov, S. V., Jiang, D., Zhang, Y., Dubonos, S. V., et al. (2004). Electric Field Effect in Atomically Thin Carbon Films. Science 306 (5696), 666–669. doi:10.1126/science.1102896
Pakdel, A., Bando, Y., and Golberg, D. (2014). Nano Boron Nitride Flatland. Chem. Soc. Rev. 43 (3), 934–959. doi:10.1039/c3cs60260e
Pelaz, B., Alexiou, C., Alvarez-Puebla, R. A., Alves, F., Andrews, A. M., Ashraf, S., et al. (2017). Diverse Applications of Nanomedicine. ACS Nano 11 (3), 2313–2381. doi:10.1021/acsnano.6b06040
Peng, Y., Li, Y., Ban, Y., Jin, H., Jiao, W., Liu, X., et al. (2014). Metal-organic Framework Nanosheets as Building Blocks for Molecular Sieving Membranes. Science 346 (6215), 1356–1359. doi:10.1126/science.1254227
Portnow, J., Badie, B., Chen, M., Liu, A., Blanchard, S., and Synold, T. W. (2009). The Neuropharmacokinetics of Temozolomide in Patients with Resectable Brain Tumors: Potential Implications for the Current Approach to Chemoradiation. Clin. Cancer Res. 15 (22), 7092–7098. doi:10.1158/1078-0432.ccr-09-1349
Rahim Pouran, S., Abdul Raman, A. A., and Wan Daud, W. M. A. (2014). Review on the Application of Modified Iron Oxides as Heterogeneous Catalysts in Fenton Reactions. J. Clean. Prod. 64, 24–35. doi:10.1016/j.jclepro.2013.09.013
Rodenas, T., Luz, I., Prieto, G., Seoane, B., Miro, H., Corma, A., et al. (2015). Metal-organic Framework Nanosheets in Polymer Composite Materials for Gas Separation. Nat. Mater 14 (1), 48–55. doi:10.1038/nmat4113
Satoh, A. Y., Trosko, J. E., and Masten, S. J. (2007). Methylene Blue Dye Test for Rapid Qualitative Detection of Hydroxyl Radicals Formed in a Fenton's Reaction Aqueous Solution. Environ. Sci. Technol. 41 (8), 2881–2887. doi:10.1021/es0617800
Siegel, R. L., Miller, K. D., Fuchs, H. E., and Jemal, A. (2021). Cancer Statistics, 2021. CA A Cancer J. Clin. 71 (1), 7–33. doi:10.3322/caac.21654
Simon-Yarza, T., Giménez-Marqués, M., Mrimi, R., Mielcarek, A., Gref, R., Horcajada, P., et al. (2017). A Smart Metal-Organic Framework Nanomaterial for Lung Targeting. Angew. Chem. Int. Ed. 56 (49), 15565–15569. doi:10.1002/anie.201707346
Strickland, K., Miner, E., Jia, Q., Tylus, U., Ramaswamy, N., Liang, W., et al. (2015). Highly Active Oxygen Reduction Non-platinum Group Metal Electrocatalyst without Direct Metal-Nitrogen Coordination. Nat. Commun. 6, 7343. doi:10.1038/ncomms8343
Su, L., Feng, J., Zhou, X., Ren, C., Li, H., and Chen, X. (2012). Colorimetric Detection of Urine Glucose Based ZnFe2O4 Magnetic Nanoparticles. Anal. Chem. 84 (13), 5753–5758. doi:10.1021/ac300939z
Tan, C., and Zhang, H. (2015). Two-dimensional Transition Metal Dichalcogenide Nanosheet-Based Composites. Chem. Soc. Rev. 44 (9), 2713–2731. doi:10.1039/c4cs00182f
Trachootham, D., Alexandre, J., and Huang, P. (2009). Targeting Cancer Cells by ROS-Mediated Mechanisms: a Radical Therapeutic Approach? Nat. Rev. Drug Discov. 8 (7), 579–591. doi:10.1038/nrd2803
Wang, Q., Hu, B., Hu, X., Kim, H., Squatrito, M., Scarpace, L., et al. (2017). Tumor Evolution of Glioma-Intrinsic Gene Expression Subtypes Associates with Immunological Changes in the Microenvironment. Cancer Cell 32 (1), 42–56. doi:10.1016/j.ccell.2017.06.003
Xu, G., Yamada, T., Otsubo, K., Sakaida, S., and Kitagawa, H. (2012). Facile "modular Assembly" for Fast Construction of a Highly Oriented Crystalline MOF Nanofilm. J. Am. Chem. Soc. 134 (40), 16524–16527. doi:10.1021/ja307953m
Zhang, C., Zhao, K., Bu, W., Ni, D., Liu, Y., Feng, J., et al. (2015). Marriage of Scintillator and Semiconductor for Synchronous Radiotherapy and Deep Photodynamic Therapy with Diminished Oxygen Dependence. Angew. Chem. Int. Ed. 54 (6), 1770–1774. doi:10.1002/anie.201408472
Zhang, C., Bu, W., Ni, D., Zhang, S., Li, Q., Yao, Z., et al. (2016). Synthesis of Iron Nanometallic Glasses and Their Application in Cancer Therapy by a Localized Fenton Reaction. Angew. Chem. Int. Ed. 55 (6), 2101–2106. doi:10.1002/anie.201510031
Keywords: metal-organic frameworks, Cu-TCPP(Fe), sonodynamic therapy, Fenton reaction, catalytic
Citation: Zhang H, Li Y, Liu J and Chang C (2022) Nano-Sonosensitized Sonodynamic Nanomedicine Augments Tumor-Selective Catalytic Tumor Eradication. Front. Mater. 9:908789. doi: 10.3389/fmats.2022.908789
Received: 31 March 2022; Accepted: 26 May 2022;
Published: 08 June 2022.
Edited by:
Mingqiang Li, Third Affiliated Hospital of Sun Yat-sen University, ChinaCopyright © 2022 Zhang, Li, Liu and Chang. This is an open-access article distributed under the terms of the Creative Commons Attribution License (CC BY). The use, distribution or reproduction in other forums is permitted, provided the original author(s) and the copyright owner(s) are credited and that the original publication in this journal is cited, in accordance with accepted academic practice. No use, distribution or reproduction is permitted which does not comply with these terms.
*Correspondence: Cai Chang, Y2hhbmdjNjFAMTYzLmNvbQ==
†These authors have contributed equally to this work