- 1Petroleum Engineering Department, College of Petroleum Engineering and Geosciences, King Fahd University of Petroleum and Minerals, Saudi Arabia
- 2Center for Integrative Petroleum Research (CIPR), College of Petroleum Engineering and Geosciences, King Fahd University of Petroleum and Minerals, Saudi Arabia
- 3Department of Petroleum Engineering, Khalifa University, Abu Dhabi, United Arab Emirates
The reservoir rock ismade up of differentminerals which contribute to the overall formation wettability. These minerals in their natural state differ in chemistry and structure, and thus behave differently in an environment of varying composition and salinity. These have direct implications for enhanced oil recovery due to water flooding, or wettability alteration due to long-term exposure to brine. With the reservoir rock being a complex system of multiple minerals, the control of wettability alterations becomes difficult to manage. One of the dominant mechanisms responsible for wettability alteration is the mineral surface charge, which is dependent on pH, and fluid composition (salt type and salinity). For the first time, the surface charge development of barite, dolomite, and feldspar minerals in their native reservoir environments (accounting for the formation brine complexity) is presented. Also, the effect of oilfield operations (induced pH change) on minerals’ surface charge development is studied. This was achieved by using the zeta potential measurements. The zeta potential results show that barite and dolomite minerals possess positively charge surfaces in formation water and seawater, with feldspar having a near-zero surface charge. Furthermore, the surface charge development is controlled by the H+/OH− (pH), electrical double-layer effect, as well as ion adsorption on the mineral’s surfaces. These findings provide key insights into the role of fluid environment (pH, composition) and oilfield operations on mineral surface charge development. In addition, the results show that careful tuning of pH with seawater injection could serve as an operational strategy to control the mineral surface charge. This is important as negatively charged surfaces negate wettability alteration due to polar crude oil components. Also, the design of an ion-engineered fluid to control the surface charge of minerals was implemented, and the results show that reduction in the Ca2+ concentration holds the key to the surface charge modifications. Surface charge modifications as evidenced in this study play a critical role in the control of wettability alteration to enhance production.
1 Introduction
The world’s economy is driven by crude oil, even in the wake of renewable energies, and with the continuous growth of the world’s population and energy demands, there is no better time to increase the production to meet the demand than now. Crude oil which is a major source of revenue to many producing nations is found in carbonate and sandstone formations, with carbonate formations holding about 60% of the world’s proven reserves (Burchette, 2012). These formations are made up of different minerals, with sandstone made up of quartz and varying proportions of clay and feldspar (aluminosilicate minerals with sodium, calcium, or potassium). Carbonate formations are made up of calcite and dolomite (Cui et al., 2014; Derkani et al., 2019). Production from these formations is controlled by pressure, mineralogy, wettability, and operational strategy, with these factors, intertwined (Mohammed et al., 2021b). The formation wettability is a major factor that controls fluid production and distribution (Celik and Somasundaran, 1980; Kim et al., 1990; Standnes and Austad, 2003; Sayyad Amin et al., 2010; Darabi et al., 2012; Shiran and Skauge, 2012; Liang et al., 2019; Rezaei et al., 2021). It is affected by several factors, one of which is the formation mineralogy. This is because the formations are made of multiple minerals which control the rock–fluid interactions (Muhammed et al., 2022). In this context, sandstone is typically water-wet, but a large spread is observed in the contact angle dataset under a broad range of operating conditions such as in CO2/rock/brine systems (Arif et al., 2019). In the case of carbonate, a more diverse wetting behavior ranging from water-wet to oil-wet or even mixed-wet is observed (Deng et al., 2021). Thus, understanding the surface chemistry and the surface charge of the associated constituent minerals of these rocks is crucial.
Rock–fluid interactions which affect the rock wettability include ion adsorption, ion exchange, and mineral dissolutions (Afagwu et al., 2021). Also, these interactions are driven by the individual’s mineral surface chemistry which includes their surface charge and morphology. For example, barite which is added to the drilling mud could interact with brine to cause sulfate scale in the formation owing to fluid interaction. So, since the reservoir formations have multiple crude oil contacting minerals that contribute to the total formation wettability, it has become a necessity to understand the behavior of the different constituent minerals to ascertain their propensity to cause problems, or wettability alterations.
Several works of literature have been reported about these minerals (Syunyaev et al., 2009; Xue et al., 2019; Mady et al., 2021; Sanati et al., 2021) and their interactions with molecules like asphaltenes, surfactants, and polymers which are said to be responsible for the rock wettability alterations. Sanati and Malayeri (2021) reported Cetrimonium bromide (CTAB) adsorption and the use of imidazolium-based ionic liquids to reduce its adsorption on dolomite rock surfaces. The findings revealed that the interaction was an acid-base type. Tang et al. (2021) reported the adsorption of potassium cetyl phosphate (PCP) on magnesite and dolomite surfaces stating the effect of PCP on the flotation process. The use of scale inhibitors to retard gypsum precipitation in a brine–dolomite system has also been reported with a surface energy approach explored to provide insight into the controlling mechanism (Nikoo et al., 2020). In the same vein, Bai et al. (2021) reported the propensity of MgSO4 to revert the dolomite rock wettability compared to CaCl2, MgCl2, and Na2SO4. The author attributed to its ability to attract sulfate, which disengages the carboxylic groups from the surface (wettability alteration to water-wet), and thus, Ca2+, Mg2+, and SO42- are potential determining ions (PDIs).
Although numerous studies (Hiorth et al., 2010; Wolthers et al., 2012; Sedghi et al., 2016; Prabhakar and Melnik, 2017; Awolayo et al., 2018; Azari et al., 2018; Abdel-Azeim et al., 2021; Zallaghi and Khaz’ali, 2021; Collini and Jackson, 2022; Mehraban et al., 2022; Mokhtari et al., 2022) have been conducted to understand the fluid interaction with these minerals, a critical aspect is missing. This we believe is the fundamental understanding of the behavior of the minerals in native reservoir fluids. Thus, a detailed study aimed at understanding these minerals’ behavior in formation brines of varying compositions and salinity is sought. For the first time, a detailed study aimed at providing insights into the behavior of barite, dolomite, and feldspar in different environments is presented. Furthermore, the effect of ion-engineered fluid on the mineral surface charge behavior and the effect of salinity gradient are reported. The findings from this study would provide insights into the design of oilfield operations to achieve a favorable wettability condition to improve recovery.
2 Materials and Methods
2.1 Materials
The materials used in this study are pure as reported by Mohammed et al. (2021a). The mineral particles used are of average particle sizes of 7, 3, and 3 µM for barite, dolomite, and feldspar, respectively. All chemicals used are of the American Chemical Society (ACS) reagent grade. Synthetic Arabian Gulf seawater (SW) and formation water (FW) with compositions as listed in Table 1 were used. Salts used to prepare the brine solutions included sodium chloride (NaCl), sodium sulfate (Na2SO4), sodium bicarbonate (NaCO3), magnesium chloride (MgCl2), and calcium chloride (CaCl2) purchased from Sigma-Aldrich® (Saint Louis, MO, United States).
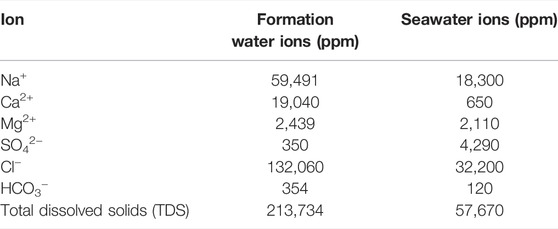
TABLE 1. Ionic composition of formation water and Arabian seawater (Mohammed et al., 2022).
2.2 Zeta Potential Sample Preparation
The mineral particles were conditioned in different fluids to mimic oilfield operations and a realistic reservoir environment. Throughout this study, 10 mg of sample particles were conditioned in 10 ml of fluid, with the mixture sonicated to ensure maximum interactions. Thereafter, the mixture was allowed to stand undisturbed for 24 h s before the measurements were conducted. For the ion concentration studies, different proportions of FW and SW were mixed to achieve the desired ionic composition. Direct dilution with deionized (DI) water was not used in this study as it does not reflect the operations conducted in the field; thus, seawater is used for dilution and ion modification to achieve the target ion concentrations.
2.3 Zeta Potential Measurement
Zeta potential measurements using electrophoresis were conducted using Anton Paar Litesizer 500 (Graz, Austria) with a size range of 3.8 nm to about 100 µM. Before every zeta potential measurement, the sample mixtures were centrifuged using the Hermle Z326K® centrifuge (Gosheim, Germany) equipped with a refrigeration system. Samples were centrifuged at 2000 rpm and 298 K for 2 min before the measurement sample is taken from the clear supernatant. All zeta potential measurements in this study are within ±2 mV standard deviation and were conducted at 298 K.
2.4 Contact Angle Measurement
The mineral (barite, dolomite, and feldspar) wettability was evaluated using contact angle measurement with the minerals conditioned in the respective fluids (DI, FW, and SW) 24 h before measurements. The contact angle was measured between the mineral chip/fluid interface in the presence of crude oil whose composition is shown in Supplementary Table S1. The measurement was conducted at 298 K and the ambient pressure using the Attension theta optical tensiometer® (Phoenix, AZ, United States).
3 Results and Discussions
3.1 Reservoir Environment
The pH values of the freshly prepared synthetic brine solutions, as well as the DI water used in this study, are 6.33, 5.94, and 8.06 for DI, FW, and SW, respectively. This serves as a benchmark for pH changes reported in this study and helps in understanding the governing mechanisms responsible for the observed changes. As a first step, the mineral particles were conditioned in DI, FW, and SW to mimic the different environments possible in the oil reservoir. The result of each mineral conditioned in the different fluids for 24 h s is discussed in the subsequent sections.
3.1.1 Barite
The surface charge of barite particles in DI, FW, and SW is shown in Figure 1, with the particle surface charge observed to be positive in all fluids. In the case of DI water, the slight reduction in the pH value (from 6.33 to 5.80) signifies the participation of the OHwater in the surface charge development. This interaction would be a hydrogen bond formation between the OHwater and the Obarite, resulting in a slightly acidic system, thus resulting in the reduction in pH. But to understand the surface charge of barite, a good understanding of its structure is critical. Barite has several surfaces, but the most stable surfaces are the (001) and the (210) (Stack et al., 2016; Hamid, 2019), although more frequently referred to as the (0 0 1) surface. When a crystal structure is cleaved, it consists of repeating units of atoms arranged orderly. Barium atoms are coordinated (12-fold) in bulk; however, cleavage to the (001) surface breaks the Ba–O bonds into Bahigh and Balow (Figure 2). Sulfate is coordinated by three oxygen atoms and five barium atoms; however, as depicted in Figure 2B, sites with missing atoms exist due to crystal structure cleaving.
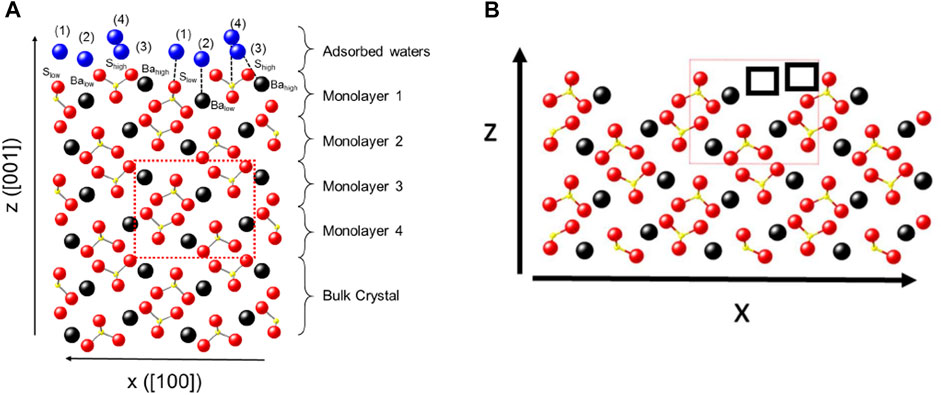
FIGURE 2. (A) Barite (001) surface with adsorbed water (blue), barium (black), and sulfur (yellow). Labels 1–4 are four different water molecules. (B) Barite surface terminated by sulfate ion with the location of missing barium and sulfate ions in high positions represented by the black square box (Bracco et al., 2017).
Hydration of the barite mineral requires about four water molecules which complete some coordination sites in the missing atom positions. The first and fourth water molecules get adsorbed at the positions of the missing barium atoms at low and high positions (Figure 2), with the same projected behavior for oxygen atoms by water molecules. When water adsorption occurs, it does so on the Balow, completing its coordinating shell leaving the Bahigh with two fewer bonds than the bulk barium ion. In solution, the barium atom is coordinated by about eight oxygen atoms. With the water adsorption resulting in fewer bonds than its bulk, the surface becomes positively charged (Figure 1). So the observed positive surface charge in the case of DI water is attributed to the incomplete coordination of the barium atom owing to steric hindrance, which prevents water access to the sites (Stack et al., 2016).
In the case of FW, barite particles possess a positive surface charge, however lower than in DI water, with no observable pH change. This indicates that the observed positive charge in FW is not due to the H+/OH− interactions but the brine compositions. The most probable reason for the observed surface charge is the incomplete coordination of barium ions although sodium ions have the propensity to adsorb on the surface. A similar behavior is observed in the case of SW, with no observable pH change. However, in the case of SW, the dominance of the sulfate and chloride ions is manifested via a decreased positive charge magnitude. This is because SW contains over 10 times the sulfate ion concentration compared to the FW. This does imply that an increased anion (sulfate) concentration would impact the barite mineral surface charge and possibly result in charge reversal.
3.1.2 Dolomite
The surface charge of dolomite particles in DI, FW, and SW is shown in Figure 3, with an all-positive surface charge. In the case of DI, an increase in the pH value (from 6.33 to 8.36) is observed, which indicates the interaction of Hwater with the dolomite surface, resulting in an excess OHwater in the system. This interaction can be said to be due to hydrogen bonding and electrostatic interaction. This agrees well with the reports of Escamilla-Roa et al. (2013) who studied the adsorption of water and organic molecules on dolomite surfaces using density functional theory (DFT) calculations. Their results showed that the water molecule interaction with the dolomite surface has higher adsorption energy than the organic molecules. This interaction was also attributed to hydrogen bond formation.
On the other hand, in the case of FW, the observed positive surface charge is not due to H+/OH− interactions as no observable pH changes were recorded (Figure 3). The observed surface charge can be attributed to the adsorption/interaction of Na, Mg, and HNaHCO3, and its reduced magnitude can be attributed to the competitive interaction of the chloride ion with the cations. In the case of SW, an increased positive surface charge magnitude is recorded with no pH change as in FW. Given that the SW has fewer cations than the FW and more sulfate ions, one would expect the surface to be negatively charged. However, the reverse is the case. This observed charge can be attributed to the increased sulfate ion concentration in SW, which enhances Na+ adsorption on the surface. However, unlike in the FW case, which has a significant amount of chloride ions to interact with the surface and reduce the magnitude of the positive charge, SW has less amount of Cl−, thus resulting in increased interaction of the Na ion with the surface.
3.1.3 Feldspar
The surface charge of feldspar particles in DI water, FW, and SW is shown in Figure 4. From this figure, there is no change in the pH in cases of the FW and SW with a slight change in the case of DI. This suggests that the H+/OH− are not PDIs in the cases of SW and FW, whereas they are PDIs in the case of DI. In the case of DI water, the feldspar mineral behavior is like that of other phyllosilicate minerals with the dominant interaction via H atoms existing as H+ and OH−, however with no pH change. More so, the negative surface charge observed in the case of FW shows the dominance of the water interaction in controlling the ion interactions with the surface. However, due to the presence of the ions in both FW and SW, a reduction in the magnitude of the negative charge is observed. In the case of SW, which contains more sulfate ions than FW, a charge reversal is observed. This is accompanied by no pH change, thus depicting the sensitivity of the feldspar surface charge in both brine conditions. The near-zero surface charge of feldspar in both FW and SW could result in surfaces being prone to polar crude oil adsorption. At near-zero surface charge conditions, a slight change in the fluid composition or oilfield operations can result in a positively charged surface condition that serves as a precursor to polar crude oil compounds adsorption. However, it can easily be reversed using ion-engineered fluids or chelating agents.
3.2 Effect of Oilfield Operations
Several oilfield operations which include, drilling, stimulation, water injection, alkaline, and surfactant flooding are implemented during the life of a field (Muhammed et al., 2020, 2022; Adjei et al., 2022; Afagwu et al., 2022). These operations could be during the primary production (FW) or secondary production stage with seawater (SW) injection (Mohammed et al., 2021a). These operations induce pH change around the wellbore, affecting the surface chemistry of the minerals within those environments (Schoonen et al., 2000; Saka and Güler, 2006; Mahani et al., 2017). These effects have dire consequences as they change the surface condition of the minerals, thus altering the nature of interaction with the reservoir fluids (Mohammed et al., 2021b). The effect of these operations was mimicked by the pH change which they induce in the field. Thus, the surface charge behavior of barite, dolomite, and feldspar due to oilfield-induced pH is reported.
3.2.1 Barite
The effect of oilfield operations on barite particles is shown in Figure 5. In the case of FW, the trend of the surface charge across all pH shows the dominance of the electrical double-layer effect (due to increased ionic strength). For example, in the acidic pH range (1–4), the surface charge decreases, and a subsequent increase at pH 2 and 3 shows the effect of the double layer. This is because as we move from pH 3 to 2, there is an increased concentration of H+, which should increase the magnitude of the positive surface charge. However, a reduction is observed because of the double-layer compression. On the other hand, at pH 5, the charge reversal is attributed to the double-layer collapse. The trend of the surface charge magnitude shows that in the FW environment, barite mineral surface charge is significantly affected by the oilfield operations. In the case of SW, which depicts the secondary production stage where seawater injection has commenced, an all-negative surface charge except at pH 2 is observed. Similarly in the case of FW, the electrical double-layer effect is observed across all pH values. At the alkaline pH values, high negative surface charges are recorded owing to the increased pH. So, to achieve a barite mineral surface charge condition inhibitive of polar crude oil compound adsorption, an extreme alkaline pH environment is necessary.
3.2.2 Dolomite
The effect of the oilfield operations on dolomite particles’ surface charge is shown in Figure 6. Oilfield-induced pH effect on dolomite particles in the primary production stage (FW) shows an all-positive surface charge behavior. This can be attributed to the effect of the electrical double layer. On the other hand, in the secondary production stage (SW), an all-negative surface charge is observed, except at an extreme alkaline pH value of 13. Like in the case of FW, the electrical double layer effect is observed. However, a double-layer collapse only occurred at the extreme alkaline pH (pH 13). From both trends (FW and SW), it can be deduced that the injection of SW into a dolomite formation promotes the surface charge conditions that inhibit polar crude oil compound adsorption. Thus, a careful design of oilfield operations especially after seawater injection has commenced is critical, as it can serve as an operation-dependent mitigation strategy for wettability control.
3.2.3 Feldspar
The behavior of the feldspar particles due to oilfield-induced pH change is shown in Figure 7. Unlike barite and dolomite, the feldspar particles across pH values from 1 to 7 in the FW environment depict a negative surface charge with charge reversal observed at pH 8 and subsequently at pH 12. This can be attributed to the double-layer collapse. This is caused by increased OH− density with the pH increase at the alkaline pH range. However, in the SW environment, all-negative surface charge conditions were recorded although the values are low at the extreme pH of the acidic and alkaline range. From the discussions thus far about the effect of oilfield operations on the mineral particles’ surface charge, it can be said that the effect of the induced pH is significant and should be factored into the design of field operations to avoid flow assurance problems that would result in wettability alterations.
3.3 Effect of Ion Engineering
The injection of ion-engineered, or composition-tuned, water into the formations for enhanced recovery is widely recognized in the industry. This is seen in numerous publications (Lee et al., 2010; Shiran and Skauge, 2012; Myint and Firoozabadi, 2015; Derkani et al., 2018; Alfazazi et al., 2019; Darvish Sarvestani et al., 2021; Kashiri et al., 2021; Mokhtari et al., 2022) on recovery improvement due to ion-tuned water injection with several mechanisms identified to be responsible for the observed recovery. This section examines the effect of ion-tuned water on the surface charge development of the particles under study. To this effect, the particles were conditioned in ion-tuned water with their surface charge determined. The concentration of different ions present in the brines was reduced by 25, 50, and 75% to identify their effect on the mineral particles’ surface charge development. This is to provide critical insights into ions that are PDIs and should be controlled to achieve a target surface charge condition.
3.3.1 Barite
The effect of ion-tuned water on the barite particle surface charge is shown in Figure 8. To achieve the target ion concentration reduction, SW was used to dilute the formation water at varying ratios carefully designed. From Figure 8A, it can be observed that the reduction of the ion concentrations resulted in an all-positive surface charge with varying magnitude. The positive charge in all cases would imply that one specific ion is not responsible for the mineral particle surface charge development; however, its contributions are not equal. For example, 25 and 50 % reduction in Na+ concentration results in an increased magnitude of positive surface charge, whereas, in the case of Mg ion, an increase is observed only at 75% ion concentration reduction. This implies that several ions interact with the surface competitively, which makes it difficult to control (cost of ion-specific treatment), except with the use of chelating agents which can chelate the cations out of the solution. Furthermore, the reduction of the anion concentration reduces the resistance to the cation’s interaction with the surface; thus, there is observed increase in the surface charge magnitude with the reduction in the concentration of anions like chloride. On the other hand, increasing the sulfate ion concentration does not reverse the positive surface charge. Instead, a reduction is achieved only at a 50% sulfate ion increase (Figure 8B). This means that for barite mineral surface charge control, carefully designed ion concentration optimization is required first to complete the coordination of the barite crystal structure and then to control the surface charge vis-à-vis ion adsorption.
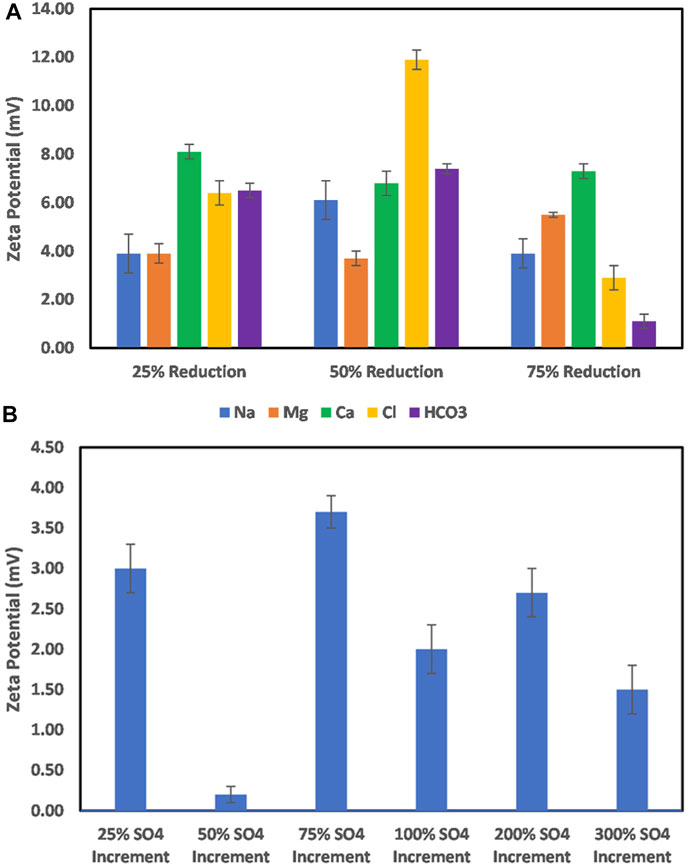
FIGURE 8. (A) Effect of Na, Mg, and Ca, and HCO3 ion concentration reduction on barite mineral surface charge. (B) Effect of increase in the sulfate ion concentration on barite mineral surface charge.
3.3.2 Dolomite
The effect of ion-tuned water on dolomite particle surface charge is shown in Figure 9. From Figure 9A, which shows the effect of Na+, Mg2+, Ca2+, Cl−, and HCO3− ion concentration reduction, an all-positive surface charge is observed. The reduction of Na+ concentration shows no significant effect until at 75% reduction. This implies that Na+ may not be the most dominant cation responsible for the dolomite particles’ surface charge. For Mg ion, a seemingly small effect is observed, which also points to the fact that it might not be the dominant cation responsible for the observed charge. However, in the case of Ca2+, a 75% reduction reverses the surface charge to negative. This implies that Ca2+ is the most dominant cation responsible for the dolomite particle surface charge. This also explains the reason reduction in the Na+ and Mg2+ concentration did not affect the nature of the surface charge. With regard to the anions, a reduction in ion concentration resulted in an increased surface charge magnitude, implying a reduction in the hindrance of the cations from interacting with the surface. On the other hand, increased SO42− concentration resulted in an all-positive surface charge with the least magnitude recorded at 75% sulfate ion increase (Figure 9B). These trends point to the fact that the reversal of the dolomite mineral surface charge can only be achieved through Ca2+ concentration reduction.
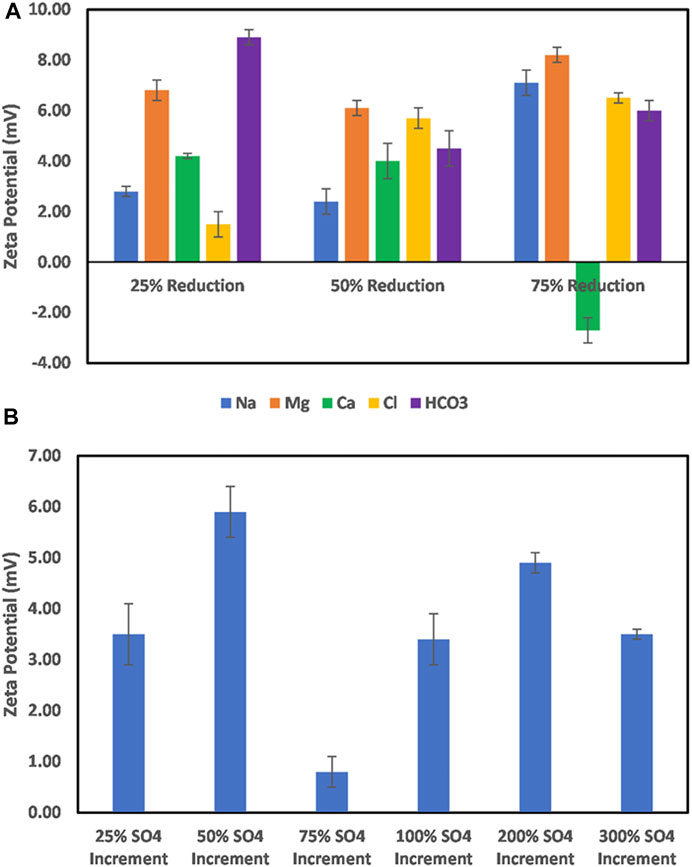
FIGURE 9. (A) Effect of Na, Mg, and Ca, and HCO3 ion concentration reduction on dolomite mineral surface charge. (B) Effect of increase in the sulfate ion concentration on dolomite mineral surface charge.
3.3.3 Feldspar
The effect of ion-engineered water on the feldspar particles’ surface charge is shown in Figure 10. From Figure 10A, it is observed that cation concentration reduction significantly impacts the surface charge of feldspar particles, with Ca2+ having the highest effect. For Na+ and Mg2+, a 50% ion concentration reduction is required to reverse the surface charge, whereas for Ca2+, only a 25% ion concentration reduction reversed the charge. A continuous decrease in the ion concentration (Na and Mg) increased the negative surface charge magnitude, but the reverse is the case in the case of Ca2+. This implies that Ca2+ is the most dominant ion responsible for the surface charge; however, with Ca2+ reduction, other ions, especially Na+, can interact more with the surface, thus reducing the negative surface charge magnitude. On the other hand, an increase in SO42− concentration does not result in a negatively charged feldspar surface (Figure 10B) but results in a positive charge surface. Even so, the effect of the electrical double layer is evident from the trends as the sulfate ion concentration increases.
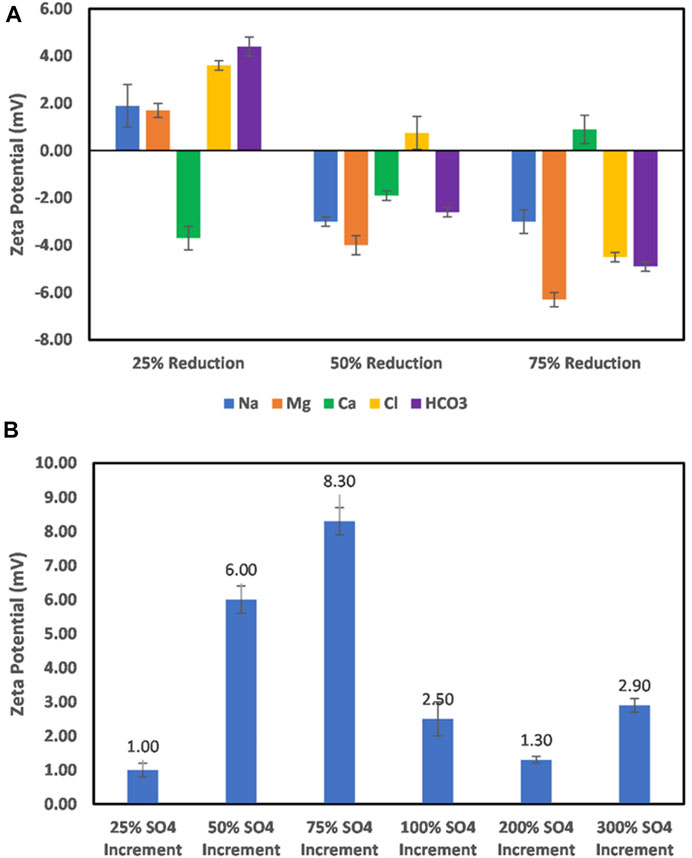
FIGURE 10. (A) Effect of Na, Mg, and Ca, and HCO3 ion concentration reduction on feldspar mineral surface charge. (B) Effect of increase in the sulfate ion concentration on feldspar mineral surface charge.
3.4 Effect of Salinity Gradient
The injection of water into the formations for pressure maintenance, or flooding processes, introduces fluids of different salinity into the reservoir. These fluids of varying composition and salinity have a significant effect on the mineral surface charge as the injected fluids flow through the reservoir from the injection well to the production wells. With the mineral surface charge being sensitive to the fluid composition and salinity changes, it becomes critical to understand the effect of the injected fluids on the mineral surface charge development. The effect of the salinity gradient on the barite particle surface charge is shown in Figure 11A. From the figure, as the SW mixes with the FW, an increase in the magnitude of the positive surface charge is observed due to a higher concentration of cations in the FW. Across the field from the injector to the producer well, it is observed that the barite mineral is positively charged. In the case of dolomite (Figure 11B), an all-positive surface charge is recorded until a mix ratio of 75% SW and 25% FW, where a charge reversal to negative is observed. This supports our earlier explanations of the effect of SW on the mineral charge development of dolomite. This means that after the 4:1 mix ratio of the SW and FW and upon the seawater breakthrough into the production well, the dolomite mineral becomes negatively charged. This also means that the dolomite formations’ wettability can be controlled by seawater injection at the stated mix ratio to mitigate polar crude oil compound adsorption. Like in the case of dolomite (Figure 11B), feldspar (Figure 12) exhibits positively charged surface conditions; however, charge reversal is observed close to the production well. This means that the injection of seawater can be explored as a surface modifying strategy to mitigate the polar compound adsorption; however, an optimal injection condition must be established for each formation.
3.5 Contact Angle
The contact angle is a measure of the wettability of a rock with its values ranging between 0 and 180°. A contact angle of zero indicates complete water wetness, and a value of 180° indicates complete oil wetness. A correlation between the zeta potential values and contact angle has been reported in the literature (Faria et al., 2012; Hassan et al., 2012; Gomari et al., 2020); however, caution must be exercised when attempting to establish a correlation. This is because the zeta potential value as a wettability alteration indicator is dependent on what the wetting phase (cationic or anionic) is. Also, the explanation of the observed changes must acknowledge the reference state before alterations. In the study by El-Din Mahmoud (El-Din Mahmoud, 2018), the effect of clay minerals on recovery was evaluated. A similar approach to that of El-Din Mahmoud (El-Din Mahmoud, 2018) is adopted here, but this time, pure mineral samples (barite, dolomite, and feldspar) are used instead of core samples. Figure 13 and Figure 14 show the contact angles of barite, dolomite, and feldspar in DI, FW, and SW, respectively. Concerning the contact angle of the minerals in DI, SW, and FW, there is no direct correlation between the observed contact angle and the increase in salinity. However, in the cases of SW and FW for all minerals, increased salinity corresponds to a decrease in the contact angle. This implies that increased salinity results in more water wetness of the surfaces.
4 Conclusion
The surface charge development of barite, dolomite, and feldspar in fluids mimicking the native reservoir environment was investigated. The results show that the dominant mechanisms responsible for the surface charge development are crystal structure coordination, ion adsorption, and the electrical double-layer effect due to increased ionic strength. Also, barite and dolomite minerals demonstrate a positive charge in DI-water, FW, and SW at all pH investigated, while feldspar remains negatively charged. The results further show that oilfield operations significantly affect the minerals’ surface charge and that Ca2+ has the most significant effect on the mineral surface charge reversal for dolomite and feldspar minerals. Last, contact angle measurements show no direct correlation between the contact angle and increase in salinity.
Data Availability Statement
The original contributions presented in the study are included in the article/Supplementary Material, further inquiries can be directed to the corresponding authors.
Author Contributions
IM: conceptualization, methodology, writing—original draft, and writing—reviewing and editing. MM: conceptualization, writing—reviewing and editing, and supervision. DS: conceptualization, writing—reviewing and editing, and supervision. MK: methodology and writing—reviewing and editing. OA: methodology and writing—reviewing and editing. MA: methodology and writing—reviewing and editing. SP: writing—reviewing and editing, and supervision.
Funding
This work was funded by the KFUPM-KU Joint Research Program, under the grants # KU-201004 and award # KU-KFUPM-2020–28.
Conflict of Interest
The authors declare that the research was conducted in the absence of any commercial or financial relationships that could be construed as a potential conflict of interest.
Publisher’s Note
All claims expressed in this article are solely those of the authors and do not necessarily represent those of their affiliated organizations, or those of the publisher, the editors, and the reviewers. Any product that may be evaluated in this article, or claim that may be made by its manufacturer, is not guaranteed or endorsed by the publisher.
Acknowledgments
The College of Petroleum and Geoscience, at King Fahd University of Petroleum and Minerals, is acknowledged for the support and permission to publish this work.
Supplementary Material
The Supplementary Material for this article can be found online at: https://www.frontiersin.org/articles/10.3389/fmats.2022.891455/full#supplementary-material
References
Abdel-Azeim, S., Sakthivel, S., Kandiel, T. A., and Kanj, M. Y. (2021). Specificity and Synergy at the Oil-Brine Interface: New Insights from Experiments and Molecular Dynamics Simulations. Energy Fuels 35, 14647–14657. doi:10.1021/acs.energyfuels.1c02133
Adewunmi, A. A., and Kamal, M. S. (2019). Demulsification of Water-In-Oil Emulsions Using Ionic Liquids: Effects of Counterion and Water Type. J. Mol. Liquids 279, 411–419. doi:10.1016/j.molliq.2019.02.008
Adjei, S., Elkatatny, S., Aggrey, W. N., and Abdelraouf, Y. (2022). Geopolymer as the Future Oil-Well Cement: A Review. J. Pet. Sci. Eng. 208, 109485. doi:10.1016/j.petrol.2021.109485
Afagwu, C., Al-Afnan, S., Patil, S., Aljaberi, J., Mahmoud, M. A., and Li, J. (2021). The Impact of Pore Structure and Adsorption Behavior on Kerogen Tortuosity. Fuel 303, 121261. doi:10.1016/j.fuel.2021.121261
Afagwu, C., Mahmoud, M. A., Alafnan, S., and Patil, S. (2022). Multiscale Storage and Transport Modeling in Unconventional Shale Gas: A Review. J. Pet. Sci. Eng. 208, 109518. doi:10.1016/j.petrol.2021.109518
Alfazazi, U., AlAmeri, W., and Hashmet, M. R. (2019). Experimental Investigation of Polymer Flooding with Low-Salinity Preconditioning of High Temperature-High-Salinity Carbonate Reservoir. J. Petrol. Explor Prod. Technol. 9, 1517–1530. doi:10.1007/s13202-018-0563-z
Arif, M., Abu-Khamsin, S. A., and Iglauer, S. (2019). Wettability of rock/CO2/brine and rock/oil/CO2-enriched-brine systems:Critical Parametric Analysis and Future Outlook. Adv. Colloid Interf. Sci. 268, 91–113. doi:10.1016/j.cis.2019.03.009
Awolayo, A., Sarma, H., and Nghiem, L. (2018). Brine-Dependent Recovery Processes in Carbonate and Sandstone Petroleum Reservoirs: Review of Laboratory-Field Studies, Interfacial Mechanisms and Modeling Attempts. Energies 11, 3020. doi:10.3390/en11113020
Azari, V., Abolghasemi, E., Hosseini, A., Ayatollahi, S., and Dehghani, F. (2018). Electrokinetic Properties of Asphaltene Colloidal Particles: Determining the Electric Charge Using Micro Electrophoresis Technique. Colloids Surf. A: Physicochemical Eng. Aspects 541, 68–77. doi:10.1016/j.colsurfa.2018.01.029
Bai, S., Kubelka, J., and Piri, M. (2021). Wettability Reversal on Dolomite Surfaces by Divalent Ions and Surfactants: An Experimental and Molecular Dynamics Simulation Study. Langmuir 37, 6641–6649. doi:10.1021/acs.langmuir.1c00415
Bracco, J. N., Lee, S. S., Stubbs, J. E., Eng, P. J., Heberling, F., Fenter, P., et al. (2017). Hydration Structure of the Barite (001)-Water Interface: Comparison of X-ray Reflectivity with Molecular Dynamics Simulations. J. Phys. Chem. C 121, 12236–12248. doi:10.1021/acs.jpcc.7b02943
Burchette, T. P. (2012). Carbonate Rocks and Petroleum Reservoirs: a Geological Perspective from the Industry. Geol. Soc. Lond. Spec. Publications 370, 17–37. doi:10.1144/SP370.14
Celik, M. S., and Somasundaran, P. (1980). “Wettability of Reservoir Minerals by Flotation and Correlation with Surfactant Adsorption,” in SPE Oilfield and Geothermal Chemistry Symposium, Stanford, California (Society of Petroleum Engineers). doi:10.2118/9002-MS
Collini, H., and Jackson, M. D. (2022). Relationship between Zeta Potential and Wettability in Porous Media: Insights from a Simple Bundle of Capillary Tubes Model. J. Colloid Interf. Sci. 608, 605–621. doi:10.1016/j.jcis.2021.09.100
Cui, L., Ma, K., Abdala, A. A., Lu, L. J., Tanakov, I., Biswal, S. L., et al. (2014). “Adsorption of a Switchable Cationic Surfactant on Natural Carbonate Minerals,” in SPE Improved Oil Recovery Symposium (Society of Petroleum Engineers), 1–16. doi:10.2118/169040-MS
Darabi, H., Sepehrnoori, K., and Kalaei, M. H. (2012). “Modeling of Wettability Alteration Due to Asphaltene Deposition in Oil Reservoirs,” in SPE Annual Technical Conference and Exhibition, San Antonio, TX (Society of Petroleum Engineers), 2551–2563. doi:10.2118/159554-MS
Darvish Sarvestani, A., Rostami, B., and Mahani, H. (2021). Polymer-Enhanced Low-Salinity Brine to Control In Situ Mixing and Salt Dispersion in Low-Salinity Waterflooding. Energy Fuels 35, 10540–10550. doi:10.1021/acs.energyfuels.1c00871
Deng, X., Shahzad Kamal, M., Patil, S., Muhammad Shakil Hussain, S., Zhou, X., and Mahmoud, M. (2021). Wettability Alteration of Locally Synthesized Cationic Gemini Surfactants on Carbonate Rock. J. Mol. Liquids 344, 117817. doi:10.1016/j.molliq.2021.117817
Derkani, M., Fletcher, A., Abdallah, W., Sauerer, B., Anderson, J., and Zhang, Z. (2018). Low Salinity Waterflooding in Carbonate Reservoirs: Review of Interfacial Mechanisms. Colloids Inter. 2, 20. doi:10.3390/colloids2020020
Derkani, M. H., Fletcher, A. J., Fedorov, M., Abdallah, W., Sauerer, B., Anderson, J., et al. (2019). Mechanisms of Surface Charge Modification of Carbonates in Aqueous Electrolyte Solutions. Colloids Inter. 3, 62. doi:10.3390/colloids3040062
El-Din Mahmoud, M. A. (2018). Effect of Chlorite Clay-Mineral Dissolution on the Improved Oil Recovery from Sandstone Rocks during Diethylenetriaminepentaacetic Acid Chelating-Agent Flooding. SPE J. 23, 1880–1898. doi:10.2118/189988-PA
Escamilla-Roa, E., Sainz-Díaz, C. I., Huertas, F. J., and Hernández-Laguna, A. (2013). Adsorption of Molecules onto (101̅4) Dolomite Surface: An Application of Computational Studies for Microcalorimetry. J. Phys. Chem. C 117, 17583–17590. doi:10.1021/jp404529e
Faria, M., Geraldes, V., and De Pinho, M. N. (2012). Surface Characterization of Asymmetric Bi-soft Segment Poly(ester Urethane Urea) Membranes for Blood-Oxygenation Medical Devices. Int. J. Biomater. 2012, 1–9. doi:10.1155/2012/376321
Hassan, B. A. R., Yusoff, Z. B. M., Othman, M. A. H., and Bin, S.information is available at the end of the Chapter, A (2012). We Are IntechOpen , the World ’ S Leading Publisher of Open Access Books Built by Scientists , for Scientists TOP 1 %. Intech 13. Available at: https://www.intechopen.com/books/advanced-biometric-technologies/liveness-detection-in-biometrics.
Hiorth, A., Cathles, L. M., and Madland, M. V. (2010). The Impact of Pore Water Chemistry on Carbonate Surface Charge and Oil Wettability. Transp Porous Med. 85, 1–21. doi:10.1007/s11242-010-9543-6
Kashiri, R., Kalantariasl, A., Parsaei, R., and Zeinijahromi, A. (2021). Experimental Study of the Effect of Clay and Oil Polarity on Oil Recovery by Low Salinity Water Flooding Using Glass Micromodel. Nat. Resour. Res. 30, 3695–3716. doi:10.1007/s11053-021-09877-7
Kim, S. T., Boudh-Hir, M.-E., and Mansoori, G. A. (1990). “The Role of Asphaltene in Wettability Reversal,” in Proc. - SPE Annu. Tech. Conf. Exhib. Pi, 799–809. doi:10.2118/20700-MS
Lee, S. Y., Webb, K. J., Collins, I. R., Lager, A., Clarke, S. M., O’Sullivan, M., et al. (2010). Low Salinity Oil Recovery-Increasing Understanding of the Underlying Mechanisms. SPE - DOE Improv. Oil Recover. Symp. Proc. 1, 534–544. doi:10.2118/129722-ms
Liang, C., Xiao, L., Zhou, C., Wang, H., Hu, F., Liao, G., et al. (2019). Wettability Characterization of Low-Permeability Reservoirs Using Nuclear Magnetic Resonance: An Experimental Study. J. Pet. Sci. Eng. 178, 121–132. doi:10.1016/j.petrol.2019.03.014
Mady, M. F., Abdel-Azeim, S., and Kelland, M. A. (20210244). Antiscaling Evaluation and Quantum Chemical Studies of Nitrogen-free Organophosphorus Compounds for Oilfield Scale Management. Ind. Eng. Chem. Res. 60, 12175–12188. doi:10.1021/acs.iecr.1c02441
Mahani, H., Keya, A. L., Berg, S., and Nasralla, R. (2017). Electrokinetics of Carbonate/Brine Interface in Low-Salinity Waterflooding: Effect of Brine Salinity, Composition, Rock Type, and pH on ?-Potential and a Surface-Complexation Model. SPE J. 22, 53–68. doi:10.2118/181745-pa
Mehraban, M. F., Farzaneh, S. A., Sohrabi, M., and Sisson, A. (2022). Fluid-Fluid Interactions Inducing Additional Oil Recovery during Low Salinity Water Injection in Inefficacious Presence of Clay Minerals. Fuel 308, 121922. doi:10.1016/j.fuel.2021.121922
Mohammed, I., Al Shehri, D. A., Mahmoud, M., Kamal, M. S., and Alade, O. (2021a). Surface Charge Investigation of Reservoir Rock Minerals. Energy Fuels 35, 6003–6021. doi:10.1021/acs.energyfuels.1c00459
Mohammed, I., Mahmoud, M., El-Husseiny, A., Al Shehri, D., Al-Garadi, K., Kamal, M. S., et al. (2021b). Impact of Asphaltene Precipitation and Deposition on Wettability and Permeability. ACS Omega 6, 20091–20102. doi:10.1021/acsomega.1c03198
Mohammed, I., Mahmoud, M., Al Shehri, D., Kamal, M. S., and Alade, O. S. (2022). Effects of the Reservoir Environment and Oilfield Operations on the Iron Mineral Surface Charge Development: An Insight into Their Role in Wettability Alteration. Energy Fuels 36, 1676–1687. doi:10.1021/acs.energyfuels.1c03909
Mokhtari, R., Anabaraonye, B. U., Afrough, A., Mohammadkhani, S., and Feilberg, K. L. (2022). Experimental Investigation of Low Salinity Water-Flooding in Tight Chalk Oil Reservoirs. J. Pet. Sci. Eng. 208, 109282. doi:10.1016/j.petrol.2021.109282
Muhammed, N. S., Haq, M. B., Al-Shehri, D., Rahaman, M. M., Keshavarz, A., and Hossain, S. M. Z. (2020). Comparative Study of green and Synthetic Polymers for Enhanced Oil Recovery. Polymers 12, 2429–2432. doi:10.3390/polym12102429
Muhammed, N. S., Haq, B., Al Shehri, D., Al-Ahmed, A., Rahman, M. M., and Zaman, E. (2022). A Review on Underground Hydrogen Storage: Insight into Geological Sites, Influencing Factors and Future Outlook. Energ. Rep. 8, 461–499. doi:10.1016/j.egyr.2021.12.002
Myint, P. C., and Firoozabadi, A. (2015). Thin Liquid Films in Improved Oil Recovery from Low-Salinity Brine. Curr. Opin. Colloid Interf. Sci. 20, 105–114. doi:10.1016/j.cocis.2015.03.002
Nikoo, A. H., Mahmoodi, L., Malayeri, M. R., and Kalantariasl, A. (2020). Gypsum-brine-dolomite Interfacial Interactions in the Presence of Scale Inhibitor. Chem. Eng. Sci. 222, 115718. doi:10.1016/j.ces.2020.115718
Prabhakar, S., and Melnik, R. (2017). Wettability Alteration of Calcite Oil wells: Influence of Smart Water Ions. Sci. Rep. 7, 17365. doi:10.1038/s41598-017-17547-z
Rezaei, A., Khodabakhshi, A., Esmaeili, A., and Razavifar, M. (2021). Effects of Initial Wettability and Different Surfactant-Silica Nanoparticles Flooding Scenarios on Oil-Recovery from Carbonate Rocks. Petroleum. doi:10.1016/j.petlm.2021.03.009
Rezaei Gomari, S., Amrouche, F., Santos, R. G., Greenwell, H. C., and Cubillas, P. (2020). A New Framework to Quantify the Wetting Behaviour of Carbonate Rock Surfaces Based on the Relationship between Zeta Potential and Contact Angle. Energies 13, 993. doi:10.3390/en13040993
Saka, E. E., and Güler, C. (2006). The Effects of Electrolyte Concentration, Ion Species and pH on the Zeta Potential and Electrokinetic Charge Density of Montmorillonite. Clay miner. 41, 853–861. doi:10.1180/0009855064140224
Sanati, A., Malayeri, M. R., Busse, O., and Weigand, J. J. (2021). Inhibition of Asphaltene Precipitation Using Hydrophobic Deep Eutectic Solvents and Ionic Liquid. J. Mol. Liquids 334, 116100. doi:10.1016/j.molliq.2021.116100
Sanati, A., and Malayeri, M. R. (2021). CTAB Adsorption onto Dolomite in the Presence of Ionic Liquid and Deep Eutectic Solvent: Experimental and Theoretical Studies. J. Mol. Liquids 325, 115176. doi:10.1016/j.molliq.2020.115176
Sayyad Amin, J., Nikooee, E., Ayatollahi, S., and Alamdari, A. (2010). Investigating Wettability Alteration Due to Asphaltene Precipitation: Imprints in Surface Multifractal Characteristics. Appl. Surf. Sci. 256, 6466–6472. doi:10.1016/j.apsusc.2010.04.036
Schoonen, M., Elsetinow, A., Borda, M., and Strongin, D. (2000). Effect of Temperature and Illumination on Pyrite Oxidation between pH 2 and 6. Geochem. Trans. 1, 23. doi:10.1039/b004044o10.1186/1467-4866-1-23
Sedghi, M., Piri, M., and Goual, L. (2016). Atomistic Molecular Dynamics Simulations of Crude Oil/Brine Displacement in Calcite Mesopores. Langmuir 32, 3375–3384. doi:10.1021/acs.langmuir.5b04713
Shiran, B. S., and Skauge, A. (2012). Wettability and Oil Recovery by Low Salinity Injection. Towar. Sustain. Growth 2, 887–901. Soc. Pet. Eng. - SPE EOR Conf. Oil Gas West Asia 2012, OGWA - EOR Build. doi:10.2118/155651-ms
Stack, A. G., Borreguero, J. M., Prisk, T. R., Mamontov, E., Wang, H.-W., Vlcek, L., et al. (2016). Precise Determination of Water Exchanges on a mineral Surface. Phys. Chem. Chem. Phys. 18, 28819–28828. doi:10.1039/C6CP05836A
Standnes, D. C., and Austad, T. (2003). Wettability Alteration in Carbonates. Colloids Surf. A: Physicochemical Eng. Aspects 216, 243–259. doi:10.1016/S0927-7757(02)00580-0
Syunyaev, R. Z., Balabin, R. M., Akhatov, I. S., and Safieva, J. O. (2009). Adsorption of Petroleum Asphaltenes onto Reservoir Rock Sands Studied by Near-Infrared (NIR) Spectroscopy. Energy Fuels 23, 1230–1236. doi:10.1021/ef8006068
Tang, Y., Yin, W., and Kelebek, S. (2021). Molecular Dynamics Simulation of Magnesite and Dolomite in Relation to Flotation with Cetyl Phosphate. Colloids Surf. A: Physicochemical Eng. Aspects 610, 125928. doi:10.1016/j.colsurfa.2020.125928
Wolthers, M., Di Tommaso, D., Du, Z., and De Leeuw, N. H. (2012). Calcite Surface Structure and Reactivity: Molecular Dynamics Simulations and Macroscopic Surface Modelling of the Calcite-Water Interface. Phys. Chem. Chem. Phys. 14, 15145–15157. doi:10.1039/c2cp42290e
Xue, X., Wang, W., Fan, H., Xu, Z., Pedruzzi, I., Li, P., et al. (2019). Adsorption Behavior of Oxalic Acid at Water-Feldspar Interface: Experiments and Molecular Simulation. Adsorption 25, 1191–1204. doi:10.1007/s10450-019-00111-8
Keywords: wettability alteration, low salinity water, reservoir minerals, adsorption, double-layer effects
Citation: Mohammed I, Al Shehri D, Mahmoud M, Kamal MS, Arif M, Alade OS and Patil S (2022) Investigation of Surface Charge at the Mineral/Brine Interface: Implications for Wettability Alteration. Front. Mater. 9:891455. doi: 10.3389/fmats.2022.891455
Received: 07 March 2022; Accepted: 28 March 2022;
Published: 27 April 2022.
Edited by:
Zhiyong Gao, Central South University, ChinaReviewed by:
Youguo Yan, China University of Petroleum, ChinaYuping Fan, Taiyuan University of Technology, China
Copyright © 2022 Mohammed, Al Shehri, Mahmoud, Kamal, Arif, Alade and Patil. This is an open-access article distributed under the terms of the Creative Commons Attribution License (CC BY). The use, distribution or reproduction in other forums is permitted, provided the original author(s) and the copyright owner(s) are credited and that the original publication in this journal is cited, in accordance with accepted academic practice. No use, distribution or reproduction is permitted which does not comply with these terms.
*Correspondence: Dhafer Al Shehri, YWxzaGVocmlkYUBrZnVwbS5lZHUuc2E=; Mohamed Mahmoud, bW1haG1vdWRAa2Z1cG0uZWR1LnNh