- 1College of Science, Jiangsu University of Science and Technology, Zhenjiang, China
- 2College of Materials Science and Engineering, Jiangsu University, Zhenjiang, China
All-inorganic perovskite quantum dots (QDs) (CsPbX3, X = Cl, Br, I) become promising candidate materials for the new generation of light-emitting diodes for their narrow emission spectrum, high photoluminescence quantum yield, and adjustable emission wavelength. However, the perovskite QDs materials still face instability against moisture, high-temperature, and UV-light. Many strategies have been reported to improve the photoluminescence (PL) performance of QDs while increasing their stability. These strategies can be divided into three main categories: doping engineering, surface ligand modification, coating strategies. This paper reviews the recent research progress of surface ligands, inorganic and polymer coating, and metal ions doping of CsPbX3 QDs. Partial substitution of Pb2+ with non-toxic or low-toxic metal ions can improve the formation energy of the perovskite lattice and reduce its toxicity. The surface polymer modification can use their ligands to bond with the uncoordinated lead and halogen ions on perovskite QDs surface to reduce surface defects, thereby improving the PL intensity and stability. In addition, the organic or inorganic coating materials on perovskite QDs can effectively avoid their contact with the external environment, thereby improving the stability of the perovskite. The optical properties of the modified QDs, including transient absorption spectra, temperature-dependent PL spectra, time-resolved photoluminescence (TRPL) spectra properties, etc. were discussed to explain the physical mechanism. The potential applications of all-inorganic perovskite QDs as down-conversion fluorescent materials in light-emitting diodes are presented. Finally, we provide some possible methods to further improve the PL performance of the all-inorganic perovskite QDs.
Introduction
Recently, all-inorganic perovskite materials have attracted much attention for their high carrier mobility, high radiation recombination efficiency, high color purity, and tunable bandgap, which are considered promising materials for next-generation light-emitting devices (LED) (Akkerman et al., 2018; Fu et al., 2019; Xu et al., 2020a).
The perovskite materials have the formula of ABX3, where A generally represents a monovalent organic or metal cation (such as Cs+, MA+, FA+), B often represents a divalent metal cation (typically Pb2+, Sn2+), and X is a halide anion (typically Cl−, Br−, I−). Figure 1 shows the regular octahedral perovskite structure. The B site ion is located at the center of the octahedron, and the X site ion is located on the six vertices of the octahedron. The BX6− octahedral units are interconnected to form the basic framework of perovskite crystal structure (Abdi-Jalebi et al., 2018). In 2009, perovskite solar cells were reported for the first time, demonstrating that perovskite semiconductor has potential applications in optoelectronic devices. Now power conversion efficiency of the perovskite solar cell has increased to 25.2% in the last years (Kojima et al., 2009; Roy et al., 2020). Since Protesescu et al. (2015) reported all-inorganic metal halide perovskite quantum dots (QDs) in 2015, inorganic perovskite materials have aroused researchers’ enthusiasm for their excellent optical properties and low synthesis cost. As a new type of direct bandgap semiconductor material, the all-inorganic metal halide perovskites (CsPbX3, X = Cl, Br, I) present some unique advantages as high quantum efficiency, large optical absorption coefficient, narrow emission peak half-width, and tunable luminescence wavelength (Li et al., 2017; Su et al., 2017; Quan et al., 2019). It has broad applications in light-emitting diodes, solar cells, and photodetectors (Wang et al., 2018; Bi et al., 2019a; Huang et al., 2019; Keqiang Chen et al., 2020). Dong et al. (2020) reported that the surface reconstruction of perovskite QDs realized an internal anion bipolar shell and an external composite materials shell with cations and polar solvent molecules. The negatively charged inner layer electrostatically attracts the outer shell, this method not only improved the carrier mobility but also reduced the defect density. In this way, the improved carrier mobility expands the recombination zone, and an active layer that is thinner than the recombination zone width will limit the carrier recombination rate. Stoumpos et al. (2013) used the CsPbBr3 single-crystal for high-energy radiation detection. Wang et al. (2017) fabricated blue, green, and red VCSELs (vertical cavity surface emitting lasers) by using CsPb(Br/Cl)3, CsPbBr3, and CsPb(I/Br)3 as active materials, which exhibited low threshold, directional output, and good stability. Yi-Xin Chen et al. (2020) also found that the CsPbBr3 halide perovskite photocatalyst significantly affected the CO2 reduction performance, achieving a higher reaction rate and long-term stability. Liu et al. (2021) used strained perovskite QDs as nucleation centers to make the film crystallization more uniform and stable. This method reduced gradient crystallization and suppressed halide segregation, leading to the efficient transport of charge carriers, the red CsPbI3 QDs films based LED showed external quantum efficiency (EQE) of 18% and remained half of the initial luminance even after 2,400 h working. Fang et al. (2021) achieved a high-performance perovskite green QLED through modulating the charge injection balance with the incorporation of a bilayered electron transport structure, while improving efficiency and operating life, a champion external quantum efficiency (EQE) of 21.63%, representing one of the most efficient perovskite QLEDs so far. All these reports indicate that the all-inorganic perovskite semiconducting material is ideal for optoelectronic device application.
Despite the excellent properties of the perovskite materials, the high sensitivity of CsPbX3 QDs to the external environment leads to their poor stability, which restricts the commercial application of perovskite (Niu et al., 2015). Hence, it still needs investigation to improve the stability of perovskite QDs. The main strategies for improving the stability of all-inorganic perovskite QDs include doping engineering, surface ligand modification engineering, and surface coating strategies. Ion-doping aims to replace some elements at the A and B sites with equivalent ions (Zou et al., 2017). The A-site doping is helpful to increase the tolerance factor of perovskite material, which thereby improves the stability. The B-site doping method mainly uses the ions with smaller ion radius to substitute Pb2+, which causes a change in the length of the B-X bond, thereby improving its phase stability and also decreasing the amount of toxic Pb2+ ions. Surface ligand modification aims to introduce ligands that bind firmly to the uncoordinated lead and halide ions on the QDs surfaces (Noel et al., 2014; Bodnarchuk et al., 2018; Krieg et al., 2018), passivating dangling bonds and defect sites, thus reducing non-radiative channels and enhancing the optical performance. The surface coating method mainly uses transparent wide-bandgap materials to encapsulate and protect the perovskite QDs from the external environment (Liu et al., 2018; Pan et al., 2018; Yajing Chang et al., 2018; Zhang et al., 2018), which dramatically reduces the degradation of QDs, thereby effectively improving their water and oxygen resistances. Meanwhile, it also avoids the reduction of quantum efficiency caused by aggregation and ion exchange effects. After modification, the stability of perovskite QDs is prominently improved, and the photoluminescence quantum yield (PLQY) is also enhanced.
This review focuses on the stability and fluorescence enhancement strategies of all-inorganic CsPbX3 QDs, primarily describing the aspects of doping engineering, surface ligand modification, and surface coating strategies. In the final section, we summarize the recent research advances and make an outlook about the future development of the perovskite materials.
Synthetic Methods
For CsPbX3 (X = Cl, Br, I) QDs, the most commonly used methods are the hot-injection method, room temperature anti-solvent method, and ion exchange method. The hot injection method can synthesize high-performance perovskite nanomaterials. Protesescu et al. (2015) injected the pre-prepared cesium oleate (Cs-oleate) precursor into dissolved oleic acid (OA), oleylamine (OAm), and octadecene (ODE) under high temperature and nitrogen flow conditions in the PbX2 solution, and then, the temperature of the reaction was rapidly cooled to room temperature, and the reaction solution was centrifuged to obtain the original perovskite solution. The hot-injection method introduces OA and OAm ligands, which provides the possibility for the subsequent study of ligand modification. In addition, this method facilitates the introduction of ions into the perovskite lattice. Since perovskite QDs materials are ionic crystals, ion migration can take place in the solution. By adding different halogen elements to the perovskite QDs solution prepared by thermal injection and other methods, perovskite QDs with different photoluminescence wavelength ranges can be prepared. Nedelcu et al. (2015) achieved the preparation of perovskite QDs in the entire visible light region by partial or full anion exchange at room temperature. However, mixing different halogens will lead to the phase separation of QDs and reduce photostability.
The room-temperature anti-solvent method mainly uses the principle that the solubility of materials in different solutions is quite different. At room temperature, PbX2 and CsX are used as material sources and they are dissolved into dimethylformamide (DMF), and then injected into the toluene solution, the solvent is in a highly supersaturated, and a large number of perovskite crystals are precipitated (Pan et al., 2016). This process still requires OA or OAm as a surface ligand to passivate surface defects and enhance the stability of QDs materials. Since the whole experimental process is carried out at room temperature, the preparation method is very simple and low-cost. However, the uniformity of the perovskite QDs obtained by this method is not very good.
Doping Engineering
The typical formula of all-inorganic perovskite QDs material is ABX3. Doping with different elements can adjust the phase transition temperature, optical bandgap, and luminescence performance, reduce the nonradiative recombination rate, and improve the PLQY and LED performance (Zhou et al., 2015; Xu et al., 2019).
The phase structure of the perovskite QDs ABX3 can change among cubic, tetragonal and orthorhombic phases (Wasylishen et al., 1985) through various doping methods. In the ABX3 structure, the radii of the A, B, and X-site ions determine the Goldschmidt tolerance factor (t) (Travis et al., 2016):
where rA, rB, and rX are the ionic radii for the components A, B, and X atoms. For most three-dimensional perovskite materials, a more stable perovskite phase structure can be formed when the t value is between 0.8 and 1. Other cations can be doped in the perovskite if their radii are similar to those of the A sites and B sites cation elements. The photoluminescence (PL) intensity and stability of perovskite QDs can be improved effectively by doping a small amount of cations at the A and B sites.
A-Site Doping
Generally, the A-site element of perovskite QDs materials is Cs+. Cs+ ion has similar properties to other alkali metal ions. A strategy for improving the perovskite QDs performance and stability is to replace a small part of Cs+ in CsPbX3 with other alkali metal cations.
Liu et al. (2020) doped Na+ into CsSnxPb1-xI3 QDs. The Na+ doping can effectively inhibit the formation of I− vacancy defects. The result of XPS demonstrated that the change of binding energy caused by Na+ doping is noticeable for Cs+ and I−. With the incorporation of Na+, the chemical bond between Sn2+ and I− ions is enhanced, the diffusion and dissociation of Sn2+ and I− ions are reduced, and the formation of harmful Sn4+ and I− vacancy defects is reduced. Figure 2A shows the PL spectra of the perovskite QDs without and with Na doping, Na+ doping causes the band-edge recombination of perovskite QDs to be significantly enhanced, and the increase of Na concentration leads to stronger near-infrared PL emission. When the Na content is 0.5%, the PLQY can reach 28%. As shown in Figures 2B,C, the transient absorption (TA) spectrum and time-resolved photoluminescence (TRPL) spectrum show that the PL lifetime of Na-doped perovskite QDs is significantly longer. Wu et al. (2021) prepared Li+-doped CsPbBr3 and CsPbI3 QDs and explored their effects on the nonradiative and radiative recombination processes. Doping with the proper concentration of Li+ could passivate halide vacancies and defects to reduce the nonradiative recombination and improve the phase stability caused by halide migration. Todorović et al. (2019) synthesized stable blue perovskite QDs by incorporating Rb+ into the A-site of CsPbBr3 nanocrystals. The Rb+ can lead to tilting of [PbX6]4− octahedral structure, reduce the overall overlap of orbits and widen the bandgap of CsPbBr3, so that QDs exhibit high PLQY (>60%) and tunable stable PL performance. A possible photocarrier recombination process model is shown in Figure 2D. The free electrons from Li+ can passivate the traps, so the trapping of the excited electrons is suppressed, leading to enhanced radiative recombination.
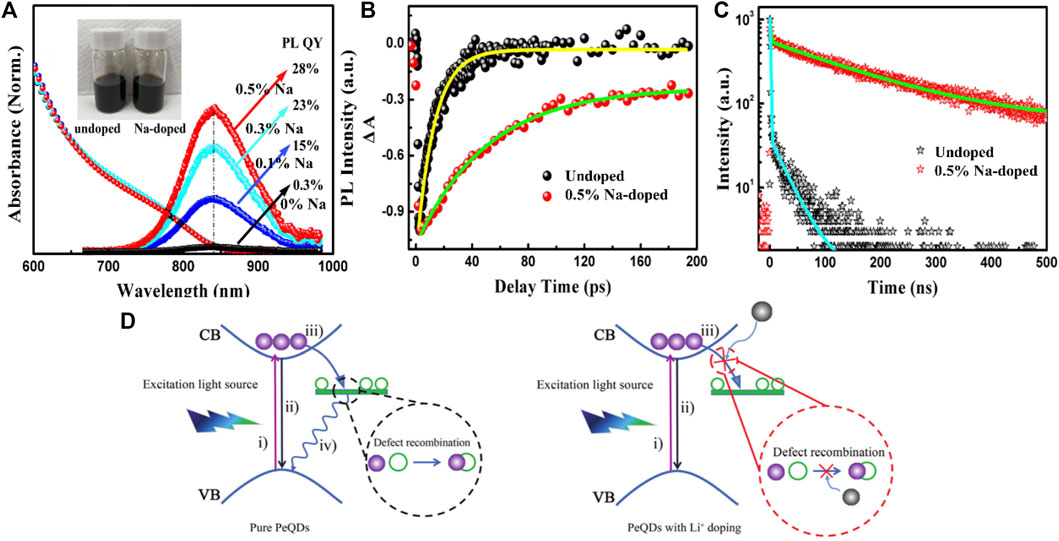
FIGURE 2. (A) UV-vis and PL spectra of the QDs with and without Na+ doping; (B) Normalized TA kinetic profiles and (C) TRPL decay of the QDs (Liu et al., 2020); (D) The schematic diagram of photocarrier recombination mechanism (Wu et al., 2021).
B-Site Doping
For CsPbX3, B-site doping is a widely accepted effective method, enhancing the QDs’ stability and inducing some novel optical properties (Ming Liu et al., 2017; Swarnkar et al., 2018; Yang et al., 2018). Sn2+, Zn2+, Mn2+ and lanthanide ions (e.g., Ce3+, Tb3+, Eu3+) have been reported as B-site doping elements in the CsPbX3 QDs. Among these B-site doping elements, the Mn-doped perovskite attracts more attention for its effective bright yellow-orange emission for the white light emission device. Zou et al. (2017) developed a thermal injection Mn2+ doping strategy to improve the stability and PL intensity. After 120-days exposure of CsPbBr3:Mn QDs to the air, 60% of the initial fluorescence intensity can be maintained. Zou et al. (2017) synthesized CsPbCl3:Mn QDs with controllable size and shape by adjusting the ratio between Mn and Pb via a one-step ultrasonic approach. As shown in Figures 3A,B, With the increase of Mn2+ doping concentration, the intensity of Mn2+ ions broadband emission band at 600 nm increases gradually, which is obviously different from the exciton emission behavior of CsPbCl3:Mn QDs (404 nm). The intensity of exciton emission peak from CsPbCl3:Mn QDs increases firstly and then decreases. This change produces an overall color output change from blue to orange. Due to surface defects’ passivation, the quantum yield can be up to 78% (Parobek et al., 2016) theoretically explained the reason why Mn-doped can improve perovskite PL performance. Under the excitation of CsPbX3 band-edge exciton recombination emission, the d–d transition of Mn2+ ions generated strong luminescence originating from the strong exchange coupling between the host charge carriers and the d electrons in the doped Mn2+ ions.
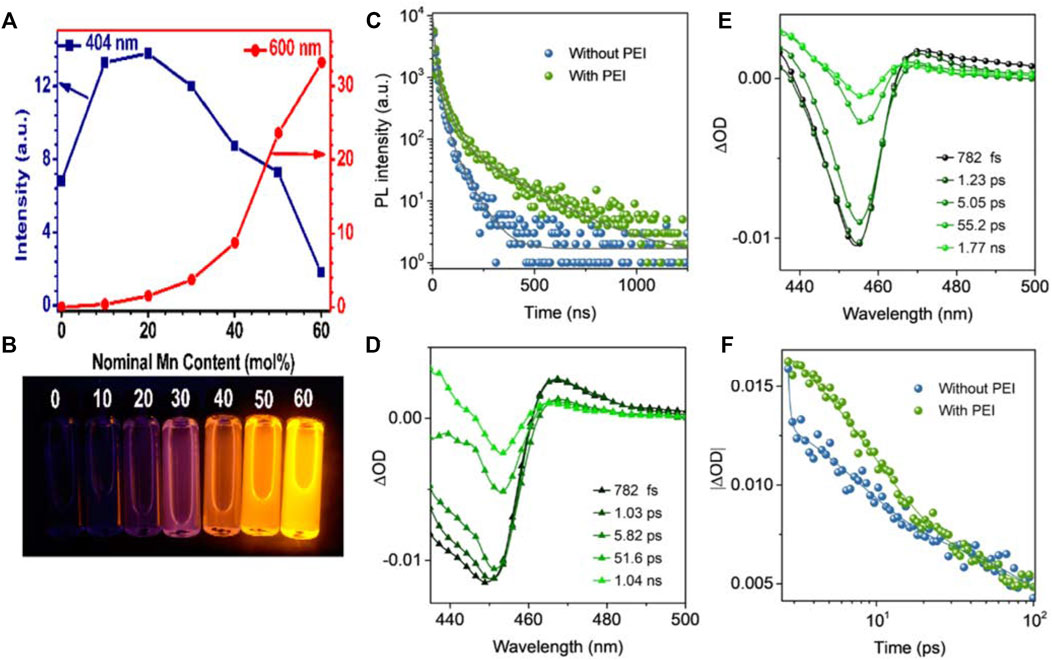
FIGURE 3. (A) PL spectra and (B) Luminescence photos of different Mn-doped ratios under UV (Zou et al., 2017). (C) PL decay of CsPbBr3 QDs; (D,E) TA spectra of CsPbBr3 QDs with and without PEI; (F) Comparisons of TA bleach recovery kinetics (Yin et al., 2021).
Song’s group found that B-site Zn2+ doping in the CsPbCl3 QDs can increase the PLQY to 120% (Li et al., 2020). The EQE of prepared CsPbCl3:Zn QLED devices can be doubled. Deng et al. (2020) fabricated CsPb1-xSnxBr3 QDs with the PLQY of 91% at room temperature due to the improvement of QDs lattices. The PL intensity increased obviously in CsPb0.9Sn0.1Br3 QDs, and with the increase of Sn-doped, the average PL lifetimes decreased rapidly. Bi et al. (2019b) doped Cu2+ into the perovskite lattices to enhance the intrinsic blue exciton emission of CsPb1−xCux (Cl/Br)3 QDs, the smaller Cu2+ ions caused lattice shrinkage and thus can eliminate the halide vacancy, which led to increased lattice formation energy and improved short-range order of doping, so that the stability of QDs were improved. A certain proportion of Cu-doped could significantly improve PLQYs, which is associated with the improvement of short-range order and the decrease of lattice vacancies. Through in-situ lanthanide cations doping, Shi et al. (2019) synthesized CsPbI3:Yb3+ where the surface defects and lattice trap states can be reduced. The CsPbCl3 QDs with La3+ and F− co-doping were reported with a high PLQY (36.5%) (Zhai et al., 2019), which showed the PL intensity is much higher than undoped pure CsPbCl3 QDs. Partial cationic doping can effectively improve the PL stability of CsPbX3 QDs, which is also a hotspot of current research.
Advantages and Disadvantages of Ion Doping
Doping engineering introduces impurity atoms into targeted lattices for efficient modulation of semiconductor optoelectronic properties. The A-site atom has a great influence on the structure and stability of QDs. By changing the size of the A-site doping elements, chemical pressure can be applied to the perovskite octahedral framework structure, and this chemical pressure will cause the octahedral structure to tilt, the central cation shift, etc., and induces some novel optoelectronic properties (Lin et al., 2016). Considering the valence state distribution of the perovskite lattice, the A-site doping ions mostly use monovalent cations, because alkali metals (Li+, Na+, K+, Rb+) have strong oxidation resistance, they are regarded as the ideal A-site doping ions. Doping some ions with smaller radii may cause lattice shrinkage, thereby reducing the perovskite QDs formation energy and greatly improving the stability and optical intensity. The B-site has a large contribution to the conduction band of QDs (Li et al., 2016), which mainly affects the optoelectronic properties of the material. The doping strategy of the B-site can reduce the lead content to a certain extent, which is very important for QDs. Commonly used doping ions are Eu3+, Bi3+, Zn2+, Fe2+, Sn2+, Mn2+, and so on. B-site doping is of great significance for reducing the lead content of lead-halide perovskite QDs, providing new energy levels, and reducing surface defects, and has become one of the important feasible schemes for the preparation of efficient and stable perovskite devices.
However, the ion doping method has a relatively limited improvement in the stability of QDs, and the distribution of doped elements into the perovskite structure is difficult to precisely adjust. Excessive or too few doping elements will cause the degradation of optical performance. How to precisely control the quantitative doping of elements is a problem to be solved.
Surface Ligand Modification
Perovskite QDs’ fluorescence is generated by exciton recombination. However, the intrinsic large surface-volume ratio of the perovskite QDs leads to many atomic dangling bonds and substantial defects on their surface. These defects will trap the electrons from the conduction band, causing nonradiative recombination and decreasing the PLQY. During the synthesis reaction of QDs, some ligands can be introduced to bond with the uncoordinated lead and halogen ions on the QDs surfaces to reduce surface defects, thereby improving the PL intensity and stability. Hence, the ligand modification of QDs is also widespread for improving the photoelectric performance of perovskite QDs.
OA and OAm Ligands
In 2015, Kovalenko’s group used a pair of oleic acid (OA) and oleylamine (OAm) ligands to form the coordination bond between Pb2+ and carboxylate, as well as the hydrogen bond between halogen and protonated amine to modify the QDs surfaces and enhance their stability (Protesescu et al., 2015). Since then, the OA and OAm ligands have been widely used to synthesize CsPbX3 QDs. However, OA and OAm are prone to protons exchange and detachments during purification and storage, causing aggregation and destabilization of QDs. Besides, excessive OAm also disaggregates the CsPbX3 QDs into PbX2, thereby quenching their fluorescence (Zeke Liu et al., 2017; Yangning Zhang et al., 2019). To achieve better passivation, short-chain ligands were found to bind more firmly to the QDs surfaces during the synthesis reaction to achieve better passivation. Common ligand alternatives can be classified into Lewis acids, Lewis bases, and multidentate ligands depending on covalent bonds.
Lewis Base Ligands
The Lewis base can provide a pair of non-bonding electrons to bond with Pb2+, thereby eliminating the defects on the CsPbX3 QD surfaces. Using tri-n-octyl phosphine oxide (TOPO) as ligands, Wu et al. (2017) synthesized CsPbBr3-TOPO QDs with adjustable size, whose fluorescence intensity decreased by only 5% after a 2-h treatment with a polar solvent, suggesting that the incorporation of TOPO ligands could remarkably improve the stability of perovskite QDs. After treatment with polar solvent ethanol, the PL intensity of CsPbX3 was significantly reduced, in contrast, the PL intensity of CsPbX3-TOPO was basically unchanged after treatment with ethanol at different times. Tan et al. (2018) obtained stable CsPbBr3-OPA QDs, exploiting the strong interaction between n-octyl phosphonic acid (OPA) and Pb2+, which not only maintained a considerably high PLQY (>90%), but also achieved high-quality dispersion in the solvent after multiple purifications, and its LEDs devices present an EQE of 6.5%.
Lewis Acids Ligands
The Lewis acids can bond with the uncoordinated halides on QDs surfaces, thereby decreasing the surface defect state of CsPbX3 QDs. Yang et al. (2019) used Dodecyl benzene sulfonic acid (DBSA) to eliminate halogen vacancy defects on the QDs surfaces. In contrast, the QDs prepared with either OAm or OA exhibited a severe decrease in the PLQY with increased washing time in ethanol. In addition, the TRPL lifetime under various washing times is almost the same for the CsPbX3 QDs with DBSA ligands, indicating a strong interaction between sulfonate groups and lead ions. Feng Liu et al. (2017) used Tri-n-octyl phosphine (TOP) ligands to obtain CsPbI3-TOP QDs, whose quantum efficiency is approximately 100% and still can be 85% after 1 month. The chemical stability of CsPbI3 QDs has been tremendously enhanced.
Multidentate Ligands
Multidentate ligands have at least two coordinating atoms in a ligand, with a stronger binding force with CsPbX3 QDs. Pan et al. (2017) passivated CsPbI3 QDs using 2, 2′-iminodibenzoic acid (IDA), a short-chain bidentate ligand that bound tightly to the PbI2-rich surfaces through dicarboxylic groups, thereby reducing surface defects while injecting additional electrons into QDs. Compared to the conventional long-chain OAm and OA ligands, they featured stronger conductivity and carrier transport capacity. Additionally, the CsPbI3-IDA QDs retained 90% of the initial luminescence peak after 15 days, exhibiting tremendously improved stability than the QDs without IDA treatment. Yin et al. (2021) used polyethyleneimine (PEI), a multidentate ligand rich in amine-based polymers on the branches, to stabilize and enhance the blue light emission of CsPbBr3 nanosheets. PEI effectively prevented the aggregation of CsPbBr3 nanosheets and reduced the vacancy defect density of bromide ions through the interaction between the electrons in its alkylamine group and the PbBr6 octahedrons. CsPbBr3 nanosheets show short-term transient absorption on the low energy side (Figure 3D), which is not as strong as the CsPbBr3with PEI (Figure 3E), and the bleach minimum of CsPbBr3 nanosheets lies to a higher energy than the CsPbBr3 with PEI. In addition, the bleaching recovery kinetics and TRPL spectra of CsPbBr3 nanosheets with PEI are much slower (Figures 3C–F), which indicates the elimination speed of excitons is much slower for the CsPbBr3 with PEI ligands.
Ligand Exchange Strategies
Except for the above three types of surface ligand modifications, researchers have proposed ligand exchange strategies to improve the stability of CsPbX3 QDs. Zhu et al. (2021) were exchanged the OAm and OA ligands with the Dodecyl dimethyl ammonium bromide (DDAB) to synthesize CsPbBr3 QDs. The shorter-chain ligand DDAB replaced the OA and OAm and then anchored onto the surfaces of CsPbX3 QDs. DDAB had a stronger binding force with the QD surfaces than the long-chain ligands as a short-chain ligand. Owing to the DDAB large branched structure and a strong affinity with X site elements (Br−) in the perovskite QDs, DDA+ cations could effectively passivate the surface defect state of CsPbX3 QDs, resulting in narrow FWHM and high PLQY. As shown in Figures 4A,B, the thermal stability of DDAB-CsPbX3 QDs was significantly improved, which was attributed to the synergistic effect between the large steric hindrance of DDAB and the SiO2 core-shell protection. Bin-Bin Zhang et al. (2019) used thionyl halides (SOCl2, SOBr2) as the passivator of CsPbCl3 instead of OAm and OA ligands. The thionyl halides take a well-controlled mild reaction with the carboxyl and amine groups on the QDs surfaces, which could easily achieve ligand exchange. After modification, a blue LED with an FWHM of only 14.6 nm was obtained, whose EQE was 1.35%.
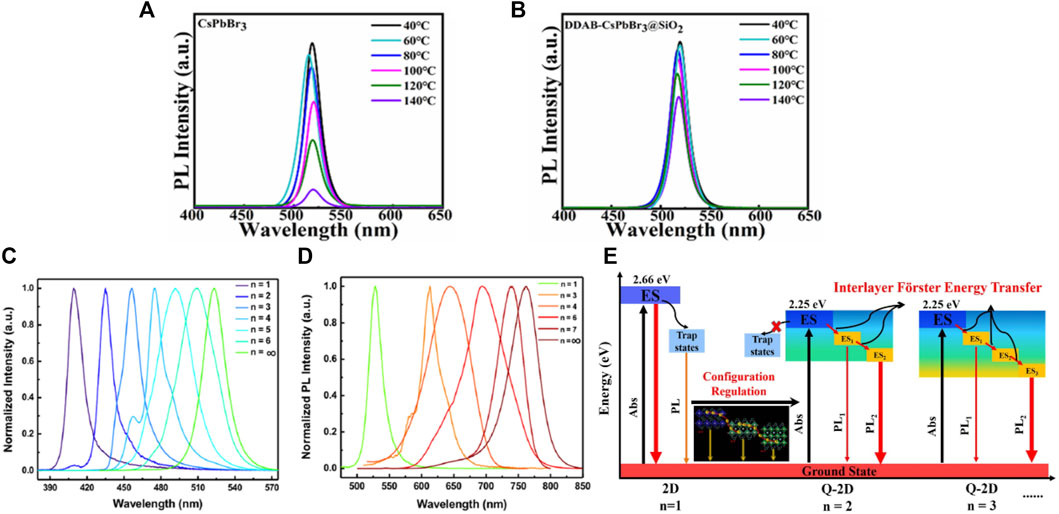
FIGURE 4. (A,B) PL spectra of CsPbBr3 and DDAB-CsPbBr3@SiO2 QD films annealed at different temperatures for 10 min (Zhu et al., 2021); (C,D) PL emission spectra of 2D QDs with for Brand I based perovskite (Yi-Hsuan Chang et al., 2018); (E) The schematic diagram of the photophysical processes (Ma et al., 2021).
Two-Dimensional Perovskite Ligands
In addition, two-dimensional (2D) Ruddlesden-Popper perovskite materials can be formed by inserting different kinds of large organic cations into the A sites of three-dimensional perovskite ABX3. The general chemical formula of this layered perovskite structure is L2 (APbX3)n-1PbX4, where L is the large organic cation (phenylethylamine (PEA+), butylammonium (BA+)) that acts as a barrier spacer between different layers, and n represents the layer number of [BX6]4−octahedron sandwiched between the organic spacer layers. In the synthesis process, the n value is generally controlled by adjusting the L-site and A-site cations ratio. Compared with three-dimensional perovskite, due to the strong dielectric and quantum confinement effects, quasi-2D perovskite materials have excellent optical properties, including large exciton binding energy and better stability against light and moisture. The corresponding colloidal perovskite nanocrystals show great potential for LEDs and other optoelectronic applications.
By introducing large cation BA+, Yi-Hsuan Chang et al. (2018) synthesized quasi-2D (BA)2 (MA)n-1PbnX3n + 1 QDs using ligand-assisted reprecipitation method and obtained a PL quantum yield as high as 48.6% by adjusting the n value (Figures 4C,D). The introduction of BA+ improves the optical stability of QDs, thereby reducing non-radiative recombination decay originating from electron-phonon coupling and increasing PL lifetime, promoting the application of durable solution-processed perovskite materials in the photoelectric field. Ma et al. (2021) introduced PEA+ cations to regulate the dimension of perovskite and obtained n = 1–3 quasi-2D perovskite (PEA)2Csn-1PbnBr3n + 1 nanocrystals with different thicknesses. The results of the transient absorption spectrum show that most of the free excitons in quasi-2D perovskite nanocrystals can be transferred from the highly-excited state to the lowest excited state through interlayer Förster energy transfer, and then the occurrence of radiative recombination results in the redshift. As shown in Figure 4E, for Quasi-2D QDs, most free excitons can be transferred from the excited state (ES) to the lowest ES at higher-dimensionality perovskites by interlayer Förster energy transfer (ES → ES1→ ES2→ ES3), and then realize radiative recombination, which could cause the electronic spectral redshift during the energy transfer process. In addition, the n = 2–3 nanocrystal solution has excellent UV light stability and great environmental stability. With the decrease of n value, the grain size decreases continuously, and the effect of quantum confinement effect becomes more significant, which increases the bandgap and makes the PL spectrum and absorption spectrum blueshift. Deng et al. (2019) synthesized ultrathin single-crystalline (PEA)2PbBr4 nanoplates by completely replacing A cation with PEA+ cation. The nanoplate-based films synthesized by an electric-field-deposition method exhibit PL emission at 410 nm with a narrow FWHM of 10.6 nm and high stability in high humidity (60%) environment. Temperature-dependent PL spectroscopy showed that (PEA)2PbBr4 nanoplates exhibited ultrahigh exciton binding energy (398.7 meV), which significantly reduced the probability of non-radiative recombination. Finally, the deep-blue LED devices were prepared based on (PEA)2PbBr4 nanoplates, which have bright luminescence and high stability under continuous operation. It can be used as an excitation light source for the construction of white light LEDs, showing great application potential in the fields of optical communication and display lighting.
The surface ligand modifications for improving the stability of perovskite QDs mainly inhibit the shedding of their surface ligands, so that their non-radiative transitions and surface defects could be reduced. More importantly, short-chain ligands would not excessively impact the electrical conductivity of QDs, thus allowing more comprehensive application of the perovskite QDs in optoelectronic devices.
Surface Coating
Although the all-inorganic lead halide perovskite QDs exhibited unique advantages in the luminescence field, their poor stability and easy dissolution in polar solvents are unavoidable shortcomings. So, the surface coating strategy for improving their stability is widely investigated. Surface coating of CsPbX3 QDs with appropriate materials can avoid direct contact with moisture, light, and oxygen and reduce the Pb diffusion to the environment. The coating materials can be inorganic or polymer materials (Loiudice et al., 2017; Zhang et al., 2017; Park et al., 2018; Bhattacharyya et al., 2019; Gao et al., 2021).
Inorganic Material Coating
Silica is a nontoxic, transparent optical material and can protect the perovskite QDs from the external environment without changing their original luminous properties. Tang’s group encapsulated the CsPbBr3 QDs with silica and effectively increased the humidity and thermal stability (Tang et al., 2019). Researchers found that the silicon shells could terminate the anion exchange when the silica-coated perovskite QDs with different halide elements (Sun et al., 2016).
Currently, tetraethyl orthosilicate (TEOS) is the most common silicon source. Gao et al. (2021) prepared CsPbBr3@SiO2 QDs using TEOS as the silicon source and hydrophobic TOPO as the inhibitor, whose PLQY reached 87% compared to the pristine QDs, thereby prominently improving the stability. However, coating with the TEOS silicon source required a harsh alkaline environment, and the QDs were prone to quenching. Hence, the particle powder silicon sources were tried. Wang et al. (2016) utilized mesoporous silica as the silicon source and fabricated mesoporous perovskite QDs nanocomposites. In the presence of water vapor, (3-aminopropyl) triethoxysilane (APTES) can be hydrolyzed into SiO2, which can also be used as a silicon source. Cao et al. (2020) prepared QDs@SiO2 composite using APTES as the silicon source instead of TEOS, which can maintain about 95% initial PL intensity after 30 days of storage in the air or 96 h of exposure to ultraviolet light. Additionally, tetramethoxysilane (TMOS) is also an excellent precursor alternative. Further, He et al. (2018) reported SiO2/Al2O3-coated perovskite QDs to realize highly-luminescent and ultra-stable Mn-doped CsPbCl3 QDs, where nonpolar hexane solvent was used to prevent the polymer solvent effect. Compared to uncoated CsPbCl3 QDs, SiO2/Al2O3-coated samples exhibited stronger photostability under blue light radiation. This was attributed to the effective protection of dense SiO2 and Al2O3 layers, which prevent erosion by oxygen and moisture.
Zhang et al. (2020) comparatively analyzed the optical properties between CsPbBr3@SiO2 and CsPbBr3 QDs, found that the exciton binding energy of CsPbBr3 QDs after silica coating is higher than that of uncoated CsPbBr3 QDs. As shown in Figures 5A,B, although the optical band gaps of both QDs were continuously broadened from 10 to 300 K, the fitting results of the temperature-dependent spectra indicate that the electron-phonon coupling in the CsPbBr3@SiO2 QDs is smaller. The encapsulation layer outside the QDs has a great influence on the strength of the electron-phonon coupling effect on the dielectric constant. SiO2 is an insulator, which can insulate the influence of the substrate and solution on the QDs, resulting in the reduction of the electron-phonon coupling effect of CsPbBr3@SiO2 QDs. According to the temperature-dependent TRPL results (Figures 5C,D), the PL lifetime increased with rising temperature, showing a longer PL lifetime than CsPbBr3 QDs when the temperature increased from 110 K to room temperature. The less phonon effect and longer photoluminescence lifetime of the CsPbBr3@SiO2 QDs indicate the silica-coated perovskite materials are more suitable for the down-conversion luminescent materials. During the 16 days PL test, the results show that CsPbBr3@SiO2 QDs exhibited better stability than CsPbBr3 QDs in the air environment.
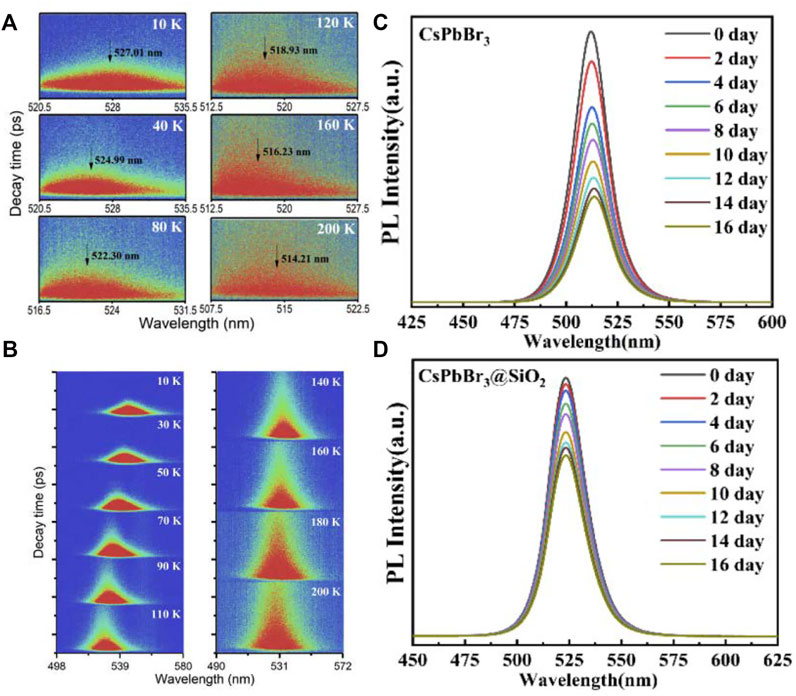
FIGURE 5. TRPLs of (A) CsPbBr3 QDs and (B) CsPbBr3@SiO2 QDs at different temperatures; PL spectra of (C) CsPbBr3 QDs and (D) CsPbBr3@SiO2 QDs measured at different times (Zhang et al., 2020).
Wu et al. (2020) synthesized SiO2-coated CsPbX3 QDs doped with Mn2+ at room temperature, which had high PLQYs. The orange-yellow PL of the CsPb0.7Mn0.3Br0.75Cl2.25@SiO2 QDs exhibited strong thermal and moisture stabilities, and their PLQY was also relatively high. As shown in Figures 6A,B, by coating the orange-yellow QDs on the UV LED chip (365 nm), a high-quality WLED device was fabricated. The luminous intensity did not change evidently after 16 h of continuous operation.
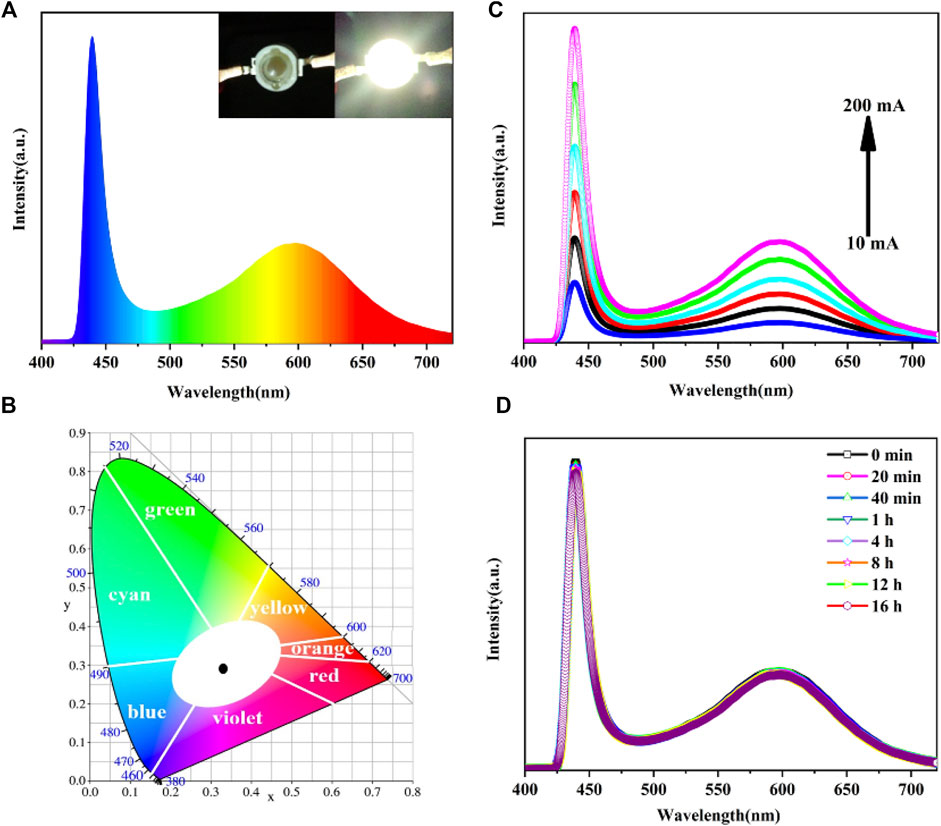
FIGURE 6. (A) White emission spectrum under a 20 mA drive current; (B) CIE chromaticity coordinates of the device; (C) Relationship between the WLED emission spectrum and the working current; (D) PL spectra of WLED measured at different times (Wu et al., 2020).
Metal oxides with dense structure and outstanding stability, such as TiO2 and Al2O3, are also reported to protect the CsPbX3 QDs. Loiudice et al. deposited alumina by ALD to coat CsPbBr3 QDs (Loiudice et al., 2017). The composite had uniform morphology and composition, with an Al2O3 coating thickness of about 10 nm. It retained over 50% of PL intensity after exposure to air or heating up to 200°C. Zhi-Jun Li et al. (2018) synthesized CsPbBr3@TiO2 composite by calcination at 300°C with TiO2 precursor. Compared to the pristine CsPbBr3 QDs, the UV spectrum absorbance of CsPbBr3@TiO2 increased slightly, which was attributed to the UV activity of the core/shell TiO2 surface layer. After encapsulating the TiO2 shell, the PL intensity of CsPbBr3@TiO2 decreased, indicating that a new nonradiative path was formed in the core/shell NCs, probably caused by the transfer of electrons from the conduction band of CsPbBr3 to the TiO2. The formed TiO2 shell layer kept the perovskite QDs undegraded in water for at least 3 months.
Polymers Coating
Polymers have the advantages of high flexibility, easy processing, and low photo-absorption coefficient. Polymers can wrap CsPbX3 QDs via their dense polymer chains. By strengthening the binding between the polymer ligand and QDs, the surrounding medium can be an effective block, thus improving the water and oxygen resistances of the perovskite QDs. Meanwhile, fluorescence quenching, toxic Pb2+ contamination, and QDs aggregation can be avoided. The thermal and moisture stabilities of QDs are significantly improved after polymer coating.
Zhang et al. (2017) developed a method for coating CsPbX3 QDs with synthetic polyvinylpyrrolidone (PVP). The PVP formed a protective layer on the CsPbBr3 surface, which also acted as an interface layer to embed QDs into polystyrene microspheres. By the protection and modification effects of PVP polymers, the quantum efficiency was increased to 27%. Raja et al. explored the combination of hydrophobic bulk polymer and CsPbBr3 to enhance the light and water stabilities, and the high fluorescence intensity can be maintained even after several months of storage (Raja et al., 2016). The metal-organic framework (MOF) was constituted by an inorganic metal center and an organic connecting substrate through coordination bonds, which enabled effective encapsulation of all-inorganic perovskite QDs. Li et al. (2019) wrapped CsPbX3 QDs with MOF material. Owing to the highly uniform microporous structure of MOF, the QDs could be prevented from contacting the external environment. Yang Li et al. (2018) employed the one-step method to prepare CsPbBr3/ethylene vinyl acetate (EVA) composite films. The EVA coated CsPbBr3 exhibited long-term stable luminescence performance in air and water, whose fluorescence intensity remained unchanged after keeping in the air for 192 and 240 h in the water, the prepared CsPbBr3 PQDs/EVA films had sufficient flexibility to be repeatedly bent 1,000 times without causing changes of PL intensity.
When the polymer structure contains polyamino, carboxyl, hydroxyl, and other groups to form a multidentate polymer, the stability of perovskite QDs can be improved due to the strong binding between the ligand and the perovskite QDs. Meyns et al. (2016) introduced poly (PMA) to coat perovskite QDs and improve its stability, where the diamine polymerization can further enhance the combination of OA and OAm ligands with the perovskite QD surfaces, thereby effectively improving the QD stability. Xu et al. (2020b) used poly maleic anhydride-alt-1-octadicene (PMAO) to coat the CsPbX3 QDs. The PMAO could act as a protective layer by combining its anhydride group with the surface ligands of the perovskite QDs. The TRPL results revealed a prolonged PL lifetime of CsPbX3/PMAO. According to the transient absorption spectroscopy results displayed in Figures 7A–D, the PMAO could slow down the intra-band hot exciton relaxation and the exciton recombination of CsPbX3 QDs. After PMAO coating, the PLQYs of green CsPbBr3 QDs and red CsPbBr0.6I2.4 QDs increased. WLED device was fabricated by integrating the green CsPbBr3/PMAO QDs and the red CsPbBr0.6I2.4/PMAO QDs on a blue GaN chip, whose stability was greatly improved as compared to that pristine perovskite QDs.
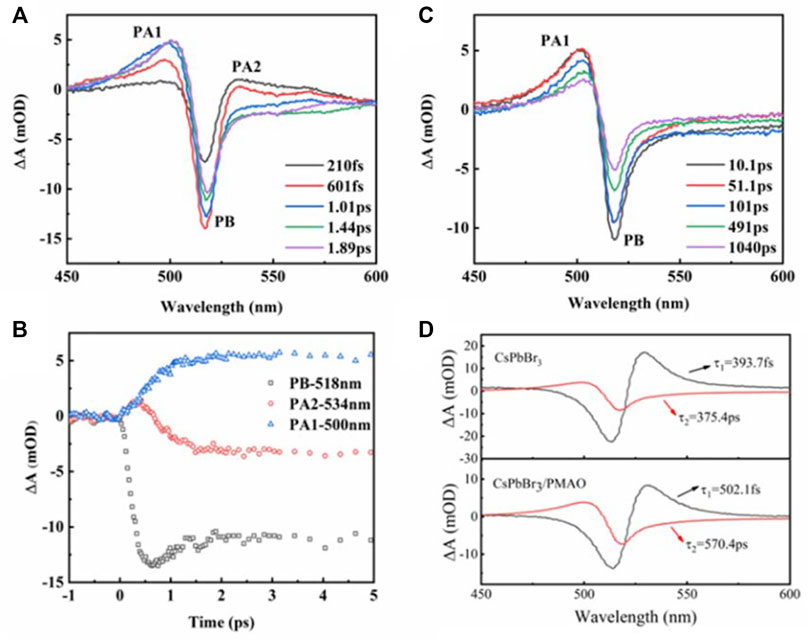
FIGURE 7. (A–D) TA spectra of CsPbBr3/PMAO QDs (Xu et al., 2020b).
The polymer materials coated CsPbX3 QDs present the advantages of simple preparation, good compatibility, and anion exchange blockage. However, most polymers have high oxygen diffusion coefficients which result in low stability and PLQY of perovskite QDs. The charge transport performance of the polymers is relatively poor. These polymer-encapsulated CsPbX3 QDs still face many problems to be solved urgently.
Conclusion
All-inorganic perovskite QDs, as a novel material, exhibit wide color tunability, narrowband PL emission, and high quantum yield. All of these properties make halide perovskite QDs an ideal candidate for light-emitting and display devices. Tremendous work has been done to improve the luminescence intensity and thermal stability of CsPbX3 QDs by strategies such as doping engineering, surface passivation, and surface coating. Nonetheless, there are still some aspects of metal halide perovskite materials that need to be further studied:
1) Although doping perovskite materials can improve their optical properties to a certain extent, excessive or too little doping will hinder the optical strength and stability of QDs. For metal ion-doped perovskites, especially non-divalent metal ions, the actual amount of doping into the lattice is hard to be controlled due to the mismatch with the valence state of Pb2+. In addition, the effects of dopants on the crystal and electronic structures of CsPbX3 QDs also require more detailed studies.
2) Ion migration occurs at defect sites in perovskites. Ion migration is one of the main reasons for the spectral instability and short worktime in perovskite QDs-based LED devices. Strategies such as the incorporation of passivation in perovskite films and interfacial layers to reduce defects may suppress ion migration in perovskite films.
3) Although doping and coating strategies can improve the air stability of perovskite materials, stability is still an inevitable problem due to the inherent structural properties of perovskite materials. Perovskite QDs with either doping or surface passivation may be a feasible way to further improve their stability.
4) Another major problem for lead halide perovskite QDs-based LEDs is the toxicity of lead. At present, researchers mainly use non-toxic cations to partially replace Pb2+ or use suitable packing materials to encapsulate QDs and suppress lead leakage. Materials with hydroxyl, carboxyl, amino, and sulfonic acid groups are expected to act as surface passivation materials, which have good complexing ability to Pb2+ and can prevent the diffusion of heavy metal ions to the environment.
In summary, inorganic perovskite materials demonstrate great potential in luminescent devices. With the progress of the research, more new semiconductor perovskite materials were found, and more effective methods will be designed to increase the stability and performance of the perovskite materials. With the tremendous efforts in the perovskite optoelectronic device research, we believe the perovskite can be the ideal candidate for the next-generation semiconductor material.
Author Contributions
YW, RJ, and JX have equally contributed to the paper writting. LS, YL, YZ, SU helped collected the references and revised the manuscript. JD provided project motivation, guidance and corrected the manuscript. All authors contributed to the article and approved the submitted version.
Funding
This work was supported by the National Science Foundation of China (11874185).
Conflict of Interest
The authors declare that the research was conducted in the absence of any commercial or financial relationships that could be construed as a potential conflict of interest.
Publisher’s Note
All claims expressed in this article are solely those of the authors and do not necessarily represent those of their affiliated organizations, or those of the publisher, the editors and the reviewers. Any product that may be evaluated in this article, or claim that may be made by its manufacturer, is not guaranteed or endorsed by the publisher.
References
Abdi-Jalebi, M., Pazoki, M., Philippe, B., Dar, M. I., Alsari, M., Sadhanala, A., et al. (2018). Dedoping of lead Halide Perovskites Incorporating Monovalent Cations. ACS Nano 12 (7), 7301–7311. doi:10.1021/acsnano.8b03586
Akkerman, Q. A., Rainò, G., Kovalenko, M. V., and Manna, L. (2018). Genesis, Challenges and Opportunities for Colloidal lead Halide Perovskite Nanocrystals. Nat. Mater 17 (5), 394–405. doi:10.1038/s41563-018-0018-4
Bhattacharyya, S., Rambabu, D., and Maji, T. K. (2019). Mechanochemical Synthesis of a Processable Halide Perovskite Quantum Dot-MOF Composite by post-synthetic Metalation. J. Mater. Chem. A 7 (37), 21106–21111. doi:10.1039/C9TA05977F
Bi, C., Kershaw, S. V., Rogach, A. L., and Tian, J. (2019a). Improved Stability and Photodetector Performance of CsPbI 3 Perovskite Quantum Dots by Ligand Exchange with Aminoethanethiol. Adv. Funct. Mater. 29 (29), 1902446. doi:10.1002/adfm.201902446
Bi, C., Wang, S., Li, Q., Kershaw, S. V., Tian, J., and Rogach, A. L. (2019b). Thermally Stable Copper(II)-Doped Cesium Lead Halide Perovskite Quantum Dots with Strong Blue Emission. J. Phys. Chem. Lett. 10 (5), 943–952. doi:10.1021/acs.jpclett.9b00290
Zhang, B.-B., Yuan, S., Ma, J.-P., Zhou, Y., Hou, J., Chen, X., et al. (2019). General Mild Reaction Creates Highly Luminescent Organic-Ligand-Lacking Halide Perovskite Nanocrystals for Efficient Light-Emitting Diodes. J. Am. Chem. Soc. 141 (38), 15423–15432. doi:10.1021/jacs.9b08140
Bodnarchuk, M. I., Boehme, S. C., Ten Brinck, S., Bernasconi, C., Shynkarenko, Y., Krieg, F., et al. (2018). Rationalizing and Controlling the Surface Structure and Electronic Passivation of Cesium lead Halide Nanocrystals. ACS Energ. Lett. 4 (1), 63–74. doi:10.1021/acsenergylett.8b01669
Cao, P., Yang, B., Zheng, F., Wang, L., and Zou, J. (2020). High Stability of Silica-Wrapped CsPbBr3 Perovskite Quantum Dots for Light Emitting Application. Ceramics Int. 46 (3), 3882–3888. doi:10.1016/j.ceramint.2019.10.114
Deng, W., Jin, X., Lv, Y., Zhang, X., Zhang, X., and Jie, J. (2019). 2D Ruddlesden-Popper Perovskite Nanoplate Based Deep‐Blue Light‐Emitting Diodes for Light Communication. Adv. Funct. Mater. 29 (40), 1903861. doi:10.1002/adfm.201903861
Deng, J., Wang, H., Xun, J., Wang, J., Yang, X., Shen, W., et al. (2020). Room-temperature Synthesis of Excellent-Performance CsPb1-Sn Br3 Perovskite Quantum Dots and Application in Light Emitting Diodes. Mater. Des. 185, 108246. doi:10.1016/j.matdes.2019.108246
Dong, Y., Wang, Y.-K., Yuan, F., Johnston, A., Liu, Y., Ma, D., et al. (2020). Bipolar-shell Resurfacing for Blue LEDs Based on Strongly Confined Perovskite Quantum Dots. Nat. Nanotechnol. 15 (8), 668–674. doi:10.1038/s41565-020-0714-5
Fang, T., Wang, T., Li, X., Dong, Y., Bai, S., and Song, J. (2021). Perovskite QLED with an External Quantum Efficiency of over 21% by Modulating Electronic Transport. Sci. Bull. 66 (1), 36–43. doi:10.1016/j.scib.2020.08.025
Liu, F., Zhang, Y., Ding, C., Kobayashi, S., Izuishi, T., Nakazawa, N., et al. (2017). Highly Luminescent Phase-Stable CsPbI3 Perovskite Quantum Dots Achieving Near 100% Absolute Photoluminescence Quantum Yield. ACS Nano 11 (10), 10373–10383. doi:10.1021/acsnano.7b05442
Fu, Y., Zhu, H., Chen, J., Hautzinger, M. P., Zhu, X.-Y., and Jin, S. (2019). Metal Halide Perovskite Nanostructures for Optoelectronic Applications and the Study of Physical Properties. Nat. Rev. Mater. 4 (3), 169–188. doi:10.1038/s41578-019-0080-9
Gao, F., Yang, W., Liu, X., Li, Y., Liu, W., Xu, H., et al. (2021). Highly Stable and Luminescent Silica-Coated Perovskite Quantum Dots at Nanoscale-Particle Level via Nonpolar Solvent Synthesis. Chem. Eng. J. 407, 128001. doi:10.1016/j.cej.2020.128001
He, M., Cheng, Y., Shen, L., Shen, C., Zhang, H., Xiang, W., et al. (2018). Mn-doped CsPbCl3 Perovskite Quantum Dots (PQDs) Incorporated into Silica/alumina Particles Used for WLEDs. Appl. Surf. Sci. 448, 400–406. doi:10.1016/j.apsusc.2018.04.098
Huang, H., Li, Y., Tong, Y., Yao, E. P., Feil, M. W., Richter, A. F., et al. (2019). Spontaneous Crystallization of Perovskite Nanocrystals in Nonpolar Organic Solvents: A Versatile Approach for Their Shape‐Controlled Synthesis. Angew. Chem. Int. Ed. 58 (46), 16558–16562. doi:10.1002/anie.201906862
Chen, K., Jin, W., Zhang, Y., Yang, T., Reiss, P., Zhong, Q., et al. (2020). High Efficiency Mesoscopic Solar Cells Using CsPbI3 Perovskite Quantum Dots Enabled by Chemical Interface Engineering. J. Am. Chem. Soc. 142 (8), 3775–3783. doi:10.1021/jacs.9b10700
Kojima, A., Teshima, K., Shirai, Y., and Miyasaka, T. (2009). Organometal Halide Perovskites as Visible-Light Sensitizers for Photovoltaic Cells. J. Am. Chem. Soc. 131 (17), 6050–6051. doi:10.1021/ja809598r
Krieg, F., Ochsenbein, S. T., Yakunin, S., Ten Brinck, S., Aellen, P., Süess, A., et al. (2018). Colloidal CsPbX3 (X = Cl, Br, I) Nanocrystals 2.0: Zwitterionic Capping Ligands for Improved Durability and Stability. ACS Energ. Lett. 3 (3), 641–646. doi:10.1021/acsenergylett.8b00035
Li, X., Wu, Y., Zhang, S., Cai, B., Gu, Y., Song, J., et al. (2016). Quantum Dots: CsPbX3Quantum Dots for Lighting and Displays: Room-Temperature Synthesis, Photoluminescence Superiorities, Underlying Origins and White Light-Emitting Diodes (Adv. Funct. Mater. 15/2016). Adv. Funct. Mater. 26 (15), 2584. doi:10.1002/adfm.201670096
Li, J., Xu, L., Wang, T., Song, J., Chen, J., Xue, J., et al. (2017). 50‐Fold EQE Improvement up to 6.27% of Solution‐processed All‐inorganic Perovskite CsPbBr3 QLEDs via Surface Ligand Density Control. Adv. Mater. 29 (5), 1603885. doi:10.1002/adma.201603885
Li, Z., Yu, C., Wen, Y., Wei, Z., Chu, J., Xing, X., et al. (2019). MOF-confined Sub-2 Nm Stable CsPbX3 Perovskite Quantum Dots. Nanomaterials 9 (8), 1147. doi:10.3390/nano9081147
Li, J., Chen, J., Xu, L., Liu, S., Lan, S., Li, X., et al. (2020). A Zinc Non-halide Dopant Strategy Enables Efficient Perovskite CsPbI3 Quantum Dot-Based Light-Emitting Diodes. Mater. Chem. Front. 4 (5), 1444–1453. doi:10.1039/C9QM00734B
Lin, J., Gomez, L., de Weerd, C., Fujiwara, Y., Gregorkiewicz, T., and Suenaga, K. (2016). Direct Observation of Band Structure Modifications in Nanocrystals of CsPbBr3 Perovskite. Nano Lett. 16 (11), 7198–7202. doi:10.1021/acs.nanolett.6b03552
Liu, Y., Li, F., Liu, Q., and Xia, Z. (2018). Synergetic Effect of Postsynthetic Water Treatment on the Enhanced Photoluminescence and Stability of CsPbX3 (X = Cl, Br, I) Perovskite Nanocrystals. Chem. Mater. 30 (19), 6922–6929. doi:10.1021/acs.chemmater.8b03330
Liu, F., Jiang, J., Zhang, Y., Ding, C., Toyoda, T., Hayase, S., et al. (2020). Near‐Infrared Emission from Tin-Lead (Sn-Pb) Alloyed Perovskite Quantum Dots by Sodium Doping. Angew. Chem. Int. Ed. 59 (22), 8421–8424. doi:10.1002/anie.201916020
Liu, Y., Dong, Y., Zhu, T., Ma, D., Proppe, A., Chen, B., et al. (2021). Bright and Stable Light-Emitting Diodes Based on Perovskite Quantum Dots in Perovskite Matrix. J. Am. Chem. Soc. 143 (38), 15606–15615. doi:10.1021/jacs.1c02148
Loiudice, A., Saris, S., Oveisi, E., Alexander, D. T. L., and Buonsanti, R. (2017). CsPbBr3 QD/AlO X Inorganic Nanocomposites with Exceptional Stability in Water, Light, and Heat. Angew. Chem. Int. Ed. 56 (36), 10696–10701. doi:10.1002/anie.201703703
Ma, L., Wang, C., Chu, Y., Guo, Y., Feng, X., Yu, Z., et al. (2021). Highly Efficient Photoluminescence of 2D Perovskites Enabled by Dimensional Increasing. 2D Mater. 8 (2), 021003. doi:10.1088/2053-1583/abdb96
Meyns, M., Perálvarez, M., Heuer-Jungemann, A., Hertog, W., Ibáñez, M., Nafria, R., et al. (2016). Polymer-enhanced Stability of Inorganic Perovskite Nanocrystals and Their Application in Color Conversion LEDs. ACS Appl. Mater. Inter. 8 (30), 19579–19586. doi:10.1021/acsami.6b02529
Liu, M., Zhong, G., Yin, Y., Miao, J., Li, K., Wang, C., et al. (2017). Aluminum-Doped Cesium Lead Bromide Perovskite Nanocrystals with Stable Blue Photoluminescence Used for Display Backlight. Adv. Sci. 4 (11), 1700335. doi:10.1002/advs.201700335
Nedelcu, G., Protesescu, L., Yakunin, S., Bodnarchuk, M. I., Grotevent, M. J., and Kovalenko, M. V. (2015). Fast Anion-Exchange in Highly Luminescent Nanocrystals of Cesium Lead Halide Perovskites (CsPbX3, X = Cl, Br, I). Nano Lett. 15 (8), 5635–5640. doi:10.1021/acs.nanolett.5b02404
Niu, G., Guo, X., and Wang, L. (2015). Review of Recent Progress in Chemical Stability of Perovskite Solar Cells. J. Mater. Chem. A 3 (17), 8970–8980. doi:10.1039/C4TA04994B
Noel, N. K., Abate, A., Stranks, S. D., Parrott, E. S., Burlakov, V. M., Goriely, A., et al. (2014). Enhanced Photoluminescence and Solar Cell Performance via Lewis Base Passivation of Organic-Inorganic Lead Halide Perovskites. ACS Nano 8 (10), 9815–9821. doi:10.1021/nn5036476
Pan, J., Quan, L. N., Zhao, Y., Peng, W., Murali, B., Sarmah, S. P., et al. (2016). Highly Efficient Perovskite-Quantum-Dot Light-Emitting Diodes by Surface Engineering. Adv. Mater. 28 (39), 8718–8725. doi:10.1002/adma.201600784
Pan, J., Shang, Y., Yin, J., De Bastiani, M., Peng, W., Dursun, I., et al. (2017). Bidentate Ligand-Passivated CsPbI3 Perovskite Nanocrystals for Stable Near-Unity Photoluminescence Quantum Yield and Efficient Red Light-Emitting Diodes. J. Am. Chem. Soc. 140 (2), 562–565. doi:10.1021/jacs.7b10647
Pan, A., Wang, J., Jurow, M. J., Jia, M., Liu, Y., Wu, Y., et al. (2018). General Strategy for the Preparation of Stable Luminous Nanocomposite Inks Using Chemically Addressable CsPbX3 Peroskite Nanocrystals. Chem. Mater. 30 (8), 2771–2780. doi:10.1021/acs.chemmater.8b00587
Park, D. H., Han, J. S., Kim, W., and Jang, H. S. (2018). Facile Synthesis of Thermally Stable CsPbBr3 Perovskite Quantum Dot-Inorganic SiO2 Composites and Their Application to white Light-Emitting Diodes with Wide Color Gamut. Dyes Pigm. 149, 246–252. doi:10.1016/j.dyepig.2017.10.003
Parobek, D., Roman, B. J., Dong, Y., Jin, H., Lee, E., Sheldon, M., et al. (2016). Exciton-to-dopant Energy Transfer in Mn-Doped Cesium lead Halide Perovskite Nanocrystals. Nano Lett. 16 (12), 7376–7380. doi:10.1021/acs.nanolett.6b02772
Protesescu, L., Yakunin, S., Bodnarchuk, M. I., Krieg, F., Caputo, R., Hendon, C. H., et al. (2015). Nanocrystals of Cesium Lead Halide Perovskites (CsPbX3, X = Cl, Br, and I): Novel Optoelectronic Materials Showing Bright Emission with Wide Color Gamut. Nano Lett. 15 (6), 3692–3696. doi:10.1021/nl5048779
Quan, L. N., Rand, B. P., Friend, R. H., Mhaisalkar, S. G., Lee, T.-W., and Sargent, E. H. (2019). Perovskites for Next-Generation Optical Sources. Chem. Rev. 119 (12), 7444–7477. doi:10.1021/acs.chemrev.9b00107
Raja, S. N., Bekenstein, Y., Koc, M. A., Fischer, S., Zhang, D., Lin, L., et al. (2016). Encapsulation of Perovskite Nanocrystals into Macroscale Polymer Matrices: Enhanced Stability and Polarization. ACS Appl. Mater. Inter. 8 (51), 35523–35533. doi:10.1021/acsami.6b09443
Roy, P., Kumar Sinha, N., Tiwari, S., and Khare, A. (2020). A Review on Perovskite Solar Cells: Evolution of Architecture, Fabrication Techniques, Commercialization Issues and Status. Solar Energy 198, 665–688. doi:10.1016/j.solener.2020.01.080
Shi, J., Li, F., Yuan, J., Ling, X., Zhou, S., Qian, Y., et al. (2019). Efficient and Stable CsPbI3 Perovskite Quantum Dots Enabled by In Situ Ytterbium Doping for Photovoltaic Applications. J. Mater. Chem. A 7 (36), 20936–20944. doi:10.1039/C9TA07143A
Stoumpos, C. C., Malliakas, C. D., Peters, J. A., Liu, Z., Sebastian, M., Im, J., et al. (2013). Crystal Growth of the Perovskite Semiconductor CsPbBr3: A New Material for High-Energy Radiation Detection. Cryst. Growth Des. 13 (7), 2722–2727. doi:10.1021/cg400645t
Su, Y., Chen, X., Ji, W., Zeng, Q., Ren, Z., Su, Z., et al. (2017). Highly Controllable and Efficient Synthesis of Mixed-Halide CsPbX3 (X = Cl, Br, I) Perovskite QDs toward the Tunability of Entire Visible Light. ACS Appl. Mater. Inter. 9 (38), 33020–33028. doi:10.1021/acsami.7b10612
Sun, C., Zhang, Y., Ruan, C., Yin, C., Wang, X., Wang, Y., et al. (2016). Efficient and Stable White LEDs with Silica-Coated Inorganic Perovskite Quantum Dots. Adv. Mater. 28 (45), 10088–10094. doi:10.1002/adma.201603081
Swarnkar, A., Mir, W. J., and Nag, A. (2018). Can B-Site Doping or Alloying Improve Thermal- and Phase-Stability of All-Inorganic CsPbX3 (X = Cl, Br, I) Perovskites? ACS Energ. Lett. 3 (2), 286–289. doi:10.1021/acsenergylett.7b01197
Tan, Y., Zou, Y., Wu, L., Huang, Q., Yang, D., Chen, M., et al. (2018). Highly Luminescent and Stable Perovskite Nanocrystals with Octylphosphonic Acid as a Ligand for Efficient Light-Emitting Diodes. ACS Appl. Mater. Inter. 10 (4), 3784–3792. doi:10.1021/acsami.7b17166
Tang, X., Chen, W., Liu, Z., Du, J., Yao, Z., Huang, Y., et al. (2019). Ultrathin, Core-Shell Structured SiO 2 Coated Mn 2+ ‐Doped Perovskite Quantum Dots for Bright White Light‐Emitting Diodes. Small 15 (19), 1900484. doi:10.1002/smll.201900484
Todorović, P., Ma, D., Chen, B., Quintero‐Bermudez, R., Saidaminov, M. I., Dong, Y., et al. (2019). Spectrally Tunable and Stable Electroluminescence Enabled by Rubidium Doping of CsPbBr3 Nanocrystals. Adv. Opt. Mater. 7 (24), 1901440. doi:10.1002/smll.201900484
Travis, W., Glover, E. N. K., Bronstein, H., Scanlon, D. O., and Palgrave, R. G. (2016). On the Application of the Tolerance Factor to Inorganic and Hybrid Halide Perovskites: a Revised System. Chem. Sci. 7 (7), 4548–4556. doi:10.1039/C5SC04845A
Wang, H.-C., Lin, S.-Y., Tang, A.-C., Singh, B. P., Tong, H.-C., Chen, C.-Y., et al. (2016). Mesoporous Silica Particles Integrated with All-Inorganic CsPbBr3 Perovskite Quantum-Dot Nanocomposites (MP-PQDs) with High Stability and Wide Color Gamut Used for Backlight Display. Angew. Chem. Int. Ed. 55 (28), 7924–7929. doi:10.1002/anie.201603698
Wang, Y., Li, X., Nalla, V., Zeng, H., and Sun, H. (2017). Solution-Processed Low Threshold Vertical Cavity Surface Emitting Lasers from All-Inorganic Perovskite Nanocrystals. Adv. Funct. Mater. 27 (13), 1605088. doi:10.1002/adfm.201605088
Wang, H.-C., Bao, Z., Tsai, H.-Y., Tang, A.-C., and Liu, R.-S. (2018). Perovskite Quantum Dots and Their Application in Light-Emitting Diodes. Small 14 (1), 1702433. doi:10.1002/smll.201702433
Wasylishen, R. E., Knop, O., and Macdonald, J. B. (1985). Cation Rotation in Methylammonium lead Halides. Solid State. Commun. 56 (7), 581–582. doi:10.1016/0038-1098(85)90959-7
Wu, L., Zhong, Q., Yang, D., Chen, M., Hu, H., Pan, Q., et al. (2017). Improving the Stability and Size Tunability of Cesium lead Halide Perovskite Nanocrystals Using Trioctylphosphine Oxide as the Capping Ligand. Langmuir 33 (44), 12689–12696. doi:10.1021/acs.langmuir.7b02963
Wu, C., Zhang, H., Wang, S., Wang, W., and Dai, J. (2020). Room-temperature One-Pot Synthesis of Highly Stable SiO2-Coated Mn-Doped All-Inorganic Perovskite CsPb0.7Mn0.3Br0.75Cl2.25 Quantum Dots for Bright white Light-Emitting Diodes. J. Lumin. 223, 117236. doi:10.1016/j.jlumin.2020.117236
Wu, H., Qiu, J., Wang, J., Wen, Y., Wang, Q., Long, Z., et al. (2021). The Dual-Defect Passivation Role of Lithium Bromide Doping in Reducing the Nonradiative Loss in CsPbX3 (X = Br and I) Quantum Dots. Inorg. Chem. Front. 8 (3), 658–668. doi:10.1039/D0QI01262A
Xu, L., Yuan, S., Zeng, H., and Song, J. (2019). A Comprehensive Review of Doping in Perovskite Nanocrystals/quantum Dots: Evolution of Structure, Electronics, Optics, and Light-Emitting Diodes. Mater. Today Nano 6, 100036. doi:10.1016/j.mtnano.2019.100036
Xu, J., Zhang, H., Wu, C., and Dai, J. (2020a). Stable CsPbX3@SiO2 Perovskite Quantum Dots for White-Light Emitting Diodes. J. Nanoelectron. Optoelectron. 15 (5), 599–606. doi:10.1166/jno.2020.2824
Xu, J., Zhu, L., Chen, J., Riaz, S., Sun, L., Wang, Y., et al. (2020b). Transient Optical Properties of CsPbX 3/Poly(maleic Anhydride‐ Alt ‐1‐octadecene) Perovskite Quantum Dots for White Light‐Emitting Diodes. Phys. Status Solidi RRL 15 (1), 2000498. doi:10.1002/pssr.202000498
Chang, Y., Yoon, Y. J., Li, G., Xu, E., Yu, S., Lu, C.-H., et al. (2018). All-inorganic Perovskite Nanocrystals with a Stellar Set of Stabilities and Their Use in white Light-Emitting Diodes. ACS Appl. Mater. Inter. 10 (43), 37267–37276. doi:10.1021/acsami.8b13553
Yang, F., Hirotani, D., Kapil, G., Kamarudin, M. A., Ng, C. H., Zhang, Y., et al. (2018). All-Inorganic CsPb1−x Ge X I2 Br Perovskite with Enhanced Phase Stability and Photovoltaic Performance. Angew. Chem. 130 (39), 12927–12931. doi:10.1002/ange.201807270
Yang, D., Li, X., Zhou, W., Zhang, S., Meng, C., Wu, Y., et al. (2019). CsPbBr 3 Quantum Dots 2.0: Benzenesulfonic Acid Equivalent Ligand Awakens Complete Purification. Adv. Mater. 31 (30), 1900767. doi:10.1002/adma.201900767
Li, Y., Lv, Y., Guo, Z., Dong, L., Zheng, J., Chai, C., et al. (2018). One-Step Preparation of Long-Term Stable and Flexible CsPbBr3 Perovskite Quantum Dots/Ethylene Vinyl Acetate Copolymer Composite Films for White Light-Emitting Diodes. ACS Appl. Mater. Inter. 10 (18), 15888–15894. doi:10.1021/acsami.8b02857
Zhang, Y., Shah, T., Deepak, F. L., and Korgel, B. A. (2019). Surface Science and Colloidal Stability of Double-Perovskite Cs2AgBiBr6 Nanocrystals and Their Superlattices. Chem. Mater. 31 (19), 7962–7969. doi:10.1021/acs.chemmater.9b02149
Chang, Y.-H., Lin, J.-C., Chen, Y.-C., Kuo, T.-R., and Wang, D.-Y. (2018). Facile Synthesis of Two-Dimensional Ruddlesden-Popper Perovskite Quantum Dots with fine-tunable Optical Properties. Nanoscale Res. Lett. 13 (1), 1–7. doi:10.1186/s11671-018-2664-5
Yin, W., Li, M., Dong, W., Luo, Z., Li, Y., Qian, J., et al. (2021). Multidentate Ligand Polyethylenimine Enables Bright Color-Saturated Blue Light-Emitting Diodes Based on CsPbBr3 Nanoplatelets. ACS Energ. Lett. 6 (2), 477–484. doi:10.1021/acsenergylett.0c02651
Chen, Y.-X., Xu, Y.-F., Wang, X.-D., Chen, H.-Y., and Kuang, D.-B. (2020). Solvent Selection and Pt Decoration towards Enhanced Photocatalytic CO2 Reduction over CsPbBr3 Perovskite Single Crystals. Sustain. Energ. Fuels 4 (5), 2249–2255. doi:10.1039/c9se01218d
Liu, Z., Bekenstein, Y., Ye, X., Nguyen, S. C., Swabeck, J., Zhang, D., et al. (2017). Ligand Mediated Transformation of Cesium Lead Bromide Perovskite Nanocrystals to Lead Depleted Cs4PbBr6 Nanocrystals. J. Am. Chem. Soc. 139 (15), 5309–5312. doi:10.1021/jacs.7b01409
Zhai, Y., Bai, X., Pan, G., Zhu, J., Shao, H., Dong, B., et al. (2019). Effective Blue-Violet Photoluminescence through Lanthanum and Fluorine Ions Co-doping for CsPbCl3 Perovskite Quantum Dots. Nanoscale 11 (5), 2484–2491. doi:10.1039/C8NR09794A
Zhang, H., Wang, X., Liao, Q., Xu, Z., Li, H., Zheng, L., et al. (2017). Embedding Perovskite Nanocrystals into a Polymer Matrix for Tunable Luminescence Probes in Cell Imaging. Adv. Funct. Mater. 27 (7), 1604382. doi:10.1002/adfm.201604382
Zhang, F., Shi, Z.-F., Ma, Z.-Z., Li, Y., Li, S., Wu, D., et al. (2018). Silica Coating Enhances the Stability of Inorganic Perovskite Nanocrystals for Efficient and Stable Down-Conversion in white Light-Emitting Devices. Nanoscale 10 (43), 20131–20139. doi:10.1039/C8NR07022A
Zhang, C., Zhang, H., Wang, R., You, D., Wang, W., Xu, C., et al. (2020). Exciton Photoluminescence of CsPbBr3@SiO2 Quantum Dots and its Application as a Phosphor Material in Light-Emitting Devices. Opt. Mater. Express 10 (4), 1007. doi:10.1364/ome.389847
Li, Z. J., Hofman, E., Li, J., Davis, A. H., Tung, C. H., Wu, L. Z., et al. (2018). Photoelectrochemically Active and Environmentally Stable CsPbBr 3/TiO 2 Core/Shell Nanocrystals. Adv. Funct. Mater. 28 (1), 1704288. doi:10.1002/adfm.201704288
Zhou, B., Shi, B., Jin, D., and Liu, X. (2015). Controlling Upconversion Nanocrystals for Emerging Applications. Nat. Nanotech. 10 (11), 924–936. doi:10.1038/nnano.2015.251
Zhu, L., Wu, C., Riaz, S., and Dai, J. (2021). Stable Silica Coated DDAB-CsPbX3 Quantum Dots and Their Application for white Light-Emitting Diodes. J. Lumin. 233, 117884. doi:10.1016/j.jlumin.2021.117884
Keywords: perovskite, quantum dots, doping, stability, photoluminescence, white light-emitting diodes
Citation: Wu Y, Jia R, Xu J, Song L, Liu Y, Zhang Y, Ullah S and Dai J (2022) Strategies of Improving CsPbX3 Perovskite Quantum Dots Optical Performance. Front. Mater. 9:845977. doi: 10.3389/fmats.2022.845977
Received: 30 December 2021; Accepted: 10 February 2022;
Published: 01 March 2022.
Edited by:
Mingming Jiang, Nanjing University of Aeronautics and Astronautics, ChinaReviewed by:
Jizhong Song, Nanjing University of Science and Technology, ChinaGangyi Zhu, Nanjing University of Posts and Telecommunications, China
Qiannan Cui, Southeast University, China
Hongyu Chen, South China Normal University, China
Copyright © 2022 Wu, Jia, Xu, Song, Liu, Zhang, Ullah and Dai. This is an open-access article distributed under the terms of the Creative Commons Attribution License (CC BY). The use, distribution or reproduction in other forums is permitted, provided the original author(s) and the copyright owner(s) are credited and that the original publication in this journal is cited, in accordance with accepted academic practice. No use, distribution or reproduction is permitted which does not comply with these terms.
*Correspondence: Jun Dai, ZGFpanVuQGp1c3QuZWR1LmNu
†These authors have contributed equally to this work and share first authorship