- 1Institute of Environment and Health, South China Hospital, Health Science Center, Shenzhen University, Shenzhen, China
- 2College of Public Health, Zhengzhou University, Zhengzhou, China
- 3Institute of Chronic Disease Risks Assessment, School of Nursing and Health, Henan University, Kaifeng, China
- 4Department of Clinical Laboratory, The Air Force Hospital of Southern Theater Command of PLA, Guangzhou, China
Magnetically induced self-assembling is considered a novel method to form photonic crystals (PCs) by the directive arrangement of nanoparticles (NPs) under a magnetic field. Magnetically responsive PCs (MRPCs) have become one of the most promising materials due to their adjustable bandgap along with the field intensity and direction, and rapid and reversible response. In this paper, we review the basic principles of MRPCs, the research progress of magnetically induced self-assembling PCs including synthesis and modification of magnetically induced NPs, the formation of an ordered structure of MRPCs, the non-spherical materials self-assemble into PC structure, and the non-magnetic materials self-assembling into PC structure. And then we also summarize the regulatory factors of the physical and chemical responses under magnetic field, and give an outlook as to the applications of MRPCs.
Introduction
Photonic Crystals (PCs) are one of the most popular nanomaterials in recent years (John, 1987; Yablonovitch, 1987) which are regular multi-dimensional periodic structures that are composed of two or more materials with different refractive indexes. As a unique photonic metamaterial, it has found many important applications in dynamic color displays (Liao, et al., 2019; Lai, et al., 2022), optical sensing (Wang, et al., 2021), and anticounterfeiting (Wang, et al., 2020). At present, they are mainly prepared by self-assembly methods, among which vertical self-assembly (Hong et al., 2014), centrifugal self-assembly (Hu et al., 2013), electrophoretic deposition self-assembly (Katagiri et al., 2017), electrostatic and capillary action self-assembly (Lee et al., 2014) and microfluidic synthesis (Yin et al., 2016)are commonly used. These self-assembly methods mainly rely on an internal driving force, such as hydrogen bond, van der Waals force, electrostatic force, capillary force, etc. (Chen et al., 2014) to achieve the spontaneous aggregation and assembly of nano-material structure unit. However, these methods are generally lacking efficiency, and usually take hours or even days to complete, and it is difficult to meet the requirements of practical application. In addition, the traditional self-assembly methods tend to be unable to produce precise thickness, orientation, grain-size, and controllable PCs, which are more difficult subsequent fabrication processing.
As a new self-assembly power source, magnetic or non-magnetic nanomaterials can be assembled under an external magnetic field (EMF) instantly. Magnetically responsive PCs (MRPCs) have attracted wide attention and developed rapidly due to their special assembly methods. Magnetically induced self-assembling can contactless transmit energy by using the interaction between MFs and magnetic nanoparticles (MNPs) and non-MNPs, which leads to change of some molecules, atoms, colloids, and particles with micro/nano scales regarding orientation, migration, and arrangement, and aggregate to form the ordered PCs structure at the micro-nano scale. Unlike the gravitational field, the size and direction of MF generated by a permanent magnet or electromagnet could be controlled artificially and directionally (Gu et al., 2014; He et al., 2015); the response is rapid and reversible, which can be well controlled by the structure. Besides, the obtained MRPCs are visible to the naked eye and can be tailor-made in different colors. Magnetically induced self-assembling offers fast, efficient, and low-cost advantages compared with the traditional self-assembly methods. Whereby, the development of this novel self-assembly technology could expand the scope of the application of PCs, and MRPC is considered as a new intelligent material with great scientific and application value in anti-counterfeiting, physical and biological sensors, detection and monitoring of chemical pollutants, etc. In this review, the synthesis and modification methods, related theories, and research status of using magnetically induced self-assembling to product PCs ordered structural material are reviewed.
Basic Principles of MRPCS
In order to enable prepared colloidal nanomaterials to form MRPCs under EMFs, the MNPs have to be equipped with homogenous particle size and monodispersing in solution. Nonetheless, the aggregation caused by the inherent mutual attraction between magnetic particles (MPs) could be the obstacle to monodispersing. In this regard, similar to the traditional self-assembling methods, MRPCs are required to be prepared in suitable particle sizes for visible spectral segments and narrow particle size distribution. Accordingly, recent studies have found that surface-active agents, such as polyacrylic acid (PAA) (Ge et al., 2007) (Figures 1A,B), polyvinylpyrrolidone (PVP) (Ma et al., 2017) (Figures 1C,D) and oleic acid (OA) (You et al., 2017) (Figures 1E,F) were usually coated on MNPs or a carbon nanolayer with a large number of carboxyl groups (Wang et al., 2010) (Figures 1G,H) so as to introduce a large amount of charge on the MNPs surface with a large amount of charge to balance the attraction between MNPs so that it could disperse evenly in the solvent. Basically, in the absence of EMF, these synthesized SMNs are randomly dispersed and scattered distribution in an aqueous solution which is known as Brownian motion. However, when an EMF is applied, the forces between the particles are mainly the electrostatic force or steric repulsion force provided by the surface coating and the magnetic dipole force between EMF and MNPs (Liu H. et al., 2019). The magnetic dipole force (He et al., 2010) was described as Eq. 1:
Where μ and θ is the magnetic moment and the angle between the center of the particle and the magnetic field; while d is the distance from the center of the adjacent particle.
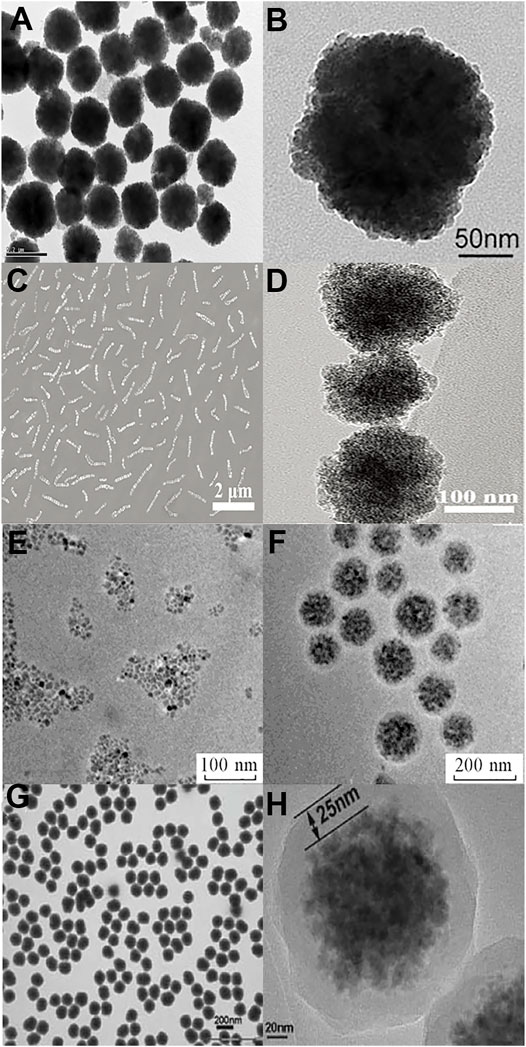
FIGURE 1. (A) Representative TEM image and (B) High-magnification TEM image of MNPs which use PAA as surfactant. Reprinted with permission from Ge et al. (2007). Copyright© 2007 John Wiley and Sons; (C) SEM image and (D) TEM image of Fe3O4@PVP@SiO2 photonic nanorods. Reprinted with permission from Ma et al. (2017). Copyright© 2017 Royal Society of Chemistry; (E) TEM image of OA-Fe3O4, which shows that the mean size of OA-Fe3O4 nanoparticles was 10 nm; (F) TEM image of MCNPs which was prepared from OA-Fe3O4 nanoparticles by employing three-step miniemulsion polymerization. Reprinted with permission from You et al. (2017). Copyright 2017© Springer Nature; and (G) TEM image and (H) HRTEM image of core/shell structure magnetite/carbon superparamagnetic CNPs. Reprinted with permission from Wang et al. (2010). Copyright© 2010 Royal Society of Chemistry.
Therefore, the dipole-dipole interaction of two adjacent particles along the MF is gravity, which is perpendicular to the direction of the MF is the repulsion force. The magnetic dipole repulsion along the MF is in balance with the electrostatic force (or long-range gravitational force), and MNPs will move in the direction of the weakening of the MF, which allows NPs to form a nano-chain structure along the MF direction. In this case, the magnetic dipole repulsion and electrostatic repulsion (or long-range repulsion) between the nano-chains can be separated from each other; thereby eventually forming a periodic configuration of the PCs on a plane perpendicular to the MF. This periodic structure has a specific photonic bandgap, where the transmission of light in the photonic bandgap could be restrained and reflected. It can be visible as bright color by naked eyes, and the optical fiber spectrometer could be used to capture its specific reflection peak. In the magnetic field, the diffraction of one-dimensional nano-chain accords with the Bragg diffraction law (Ma et al., 2017) can be expressed by Eq. 2:
Where λ is the spectral peak, n is the refractive index of the solvent, d is the distance between two adjacent particles, namely, the PCs cycle, and θ is the angle of incidence. Therefore, the factors affecting the structural color of PCs mainly include the refractive index of the solvent, periodicity, and the angle of incidence. Under a condition of constant refractive index and the incident angle, the PCs period d of the nano-chain will become small, and along with the blueshift of the diffraction peak, if the strength of the MF is increased, and the moving range is related to the particle size.
He’s research group (Yang et al., 2016) proved that Fe3O4@PAA colloidal aqueous solution with a diameter of 123 nm has 310 nm reflection peak movement in the MF including most of the visible spectrum. However, Fe3O4@PAA colloidal aqueous solutions with a larger diameter and smaller diameter have only about 200 nm reflection peak movement because the size of the MNPs limits the variation of the PCs cycle under varying MFs (Figure 2).
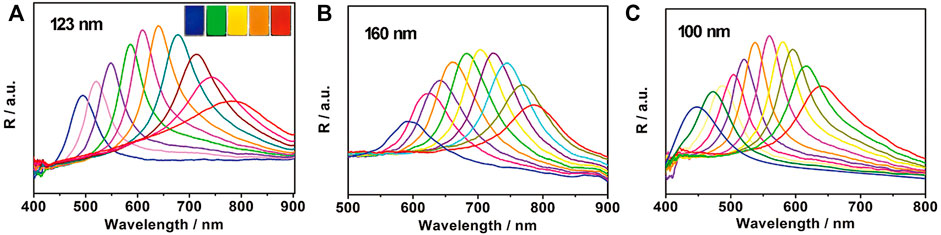
FIGURE 2. Reflection spectra of Fe3O4@PAA colloidal aqueous solutions with different particle sizes. (A) 123 nm; (B) 160 nm; and (B) 100 nm, under different MFs achieved by changing the sample-magnet distance from 7 to 3 cm for (A), from 8 to 3 cm for (B), and from 6 to 1 cm for (C) with a step of 0.5 cm. Reprinted with permission from Yang et al. (2016). Copyright 2016© Royal Society of Chemistry.
The random-stated and assembly SMNs can be formed into ordered chain-like 1D structures and then evolve into the multi-chains and the long-range 2D planar/sheet structures (Liu J. et al., 2019). Further increasing the SMNs concentration and the interparticle potential may lead to coalescence of the 2D planar structures and eventually assemble to 3D crystals (Wang and Yin, 2016). Originally, in the absence of EMF, these SMNs were of randomly dispersed and scattered distribution (Brownian motion) in aqueous solution by the strong electrostatic repulsive interactions and the color in the visual field turned to almost brown (left panel of Figure 3A). The forces imposed on SMNs were illustrated in the right panel of Figure 3A. The MRPCs diffraction was correspondingly red-shift from left to right as blue, green, and red with the SMNs-magnet distance gradually increased (Figure 3B). The key point for the assembling of MRPCs in an aqueous solution is to establish a balance among the dipole-dipole attractive force, exclusion force, and dipole-dipole electrostatic repulsive forces (Wang and Yin, 2016).
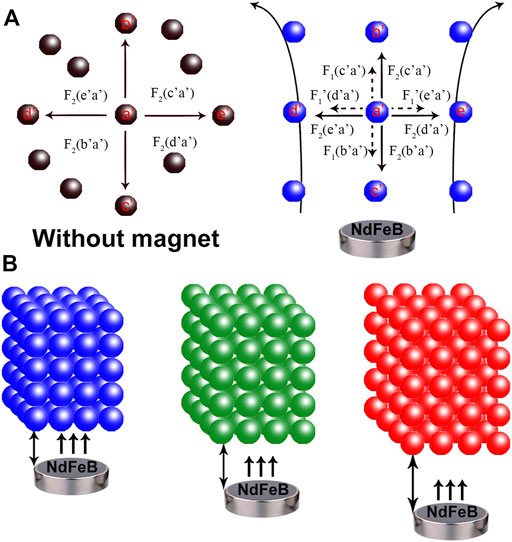
FIGURE 3. (A) Schematic illustration of the forces on Fe3O4@C@SiO2 SMNs in the absence (left) and presence of the EMF (right). F1: dipole-dipole attractive force (the small dotted arrow), F1′: dipole-dipole repulsive force (the small dotted arrow), F2: electrostatic repulsive force (the solid arrow). a′ to e′ refers to the different directions; and (B) Assembling a 3D orderly array under the EMF. The MRPCs diffraction was correspondingly red-shift from left to right as blue, green, and red with the SMNs-magnet distance gradually increased. Reprinted with permission from Liu et al. (2019) Copyright© 2019, Frontiers Media S.A.
Research Progress of Magnetically Induced SELF-ASSEMBLING PCS
Synthesis and Modification of Magnetically Induced NPs
Similar to the traditional magnetic materials, the synthetic methods of MRPCs are including co-precipitation, hot-injection, and one-step method, etc. Asher’s group employed coprecipitation of ferric and ferrous chloride in ammonium hydroxide solution to synthesize MNPs with a particle size of about 10 nm and then uses emulsion polymerization method to coat the non-magnetic material polystyrene (PS) on the surface of MNPs. A magnetic field self-assembles the particles and controls the diffraction wavelength and crystal parameters of the array. In this case, the particle size was about 134 nm, the ferromagnetic content was 17.1wt.-%, and the polymer MNPs, which has no coercivity and remanence at room temperature (RT).
The method used by Yin’s group (Ge et al., 2007) was called hot-injection, which is based on the high-temperature reaction, including three samples, two changes of reaction temperature, inlets nitrogen under the condition of 220°C for nearly 3 h to get Fe3O4 NPs with a large number of carboxyl groups on the surface. Also, the addition of NaOH (25 M) in the system could regulate the particle size of MNPs in the range of 30–180 nm (Figures 4A–F). The MRPCs from this method are homogeneous, the monodisperse is satisfied with the accurate and controllable particle size which presents superparamagnetic and high saturation of the magnetic intensity at RT. However, the synthesis conditions are relatively difficult to control, and the yield is not high, which limits its further application.
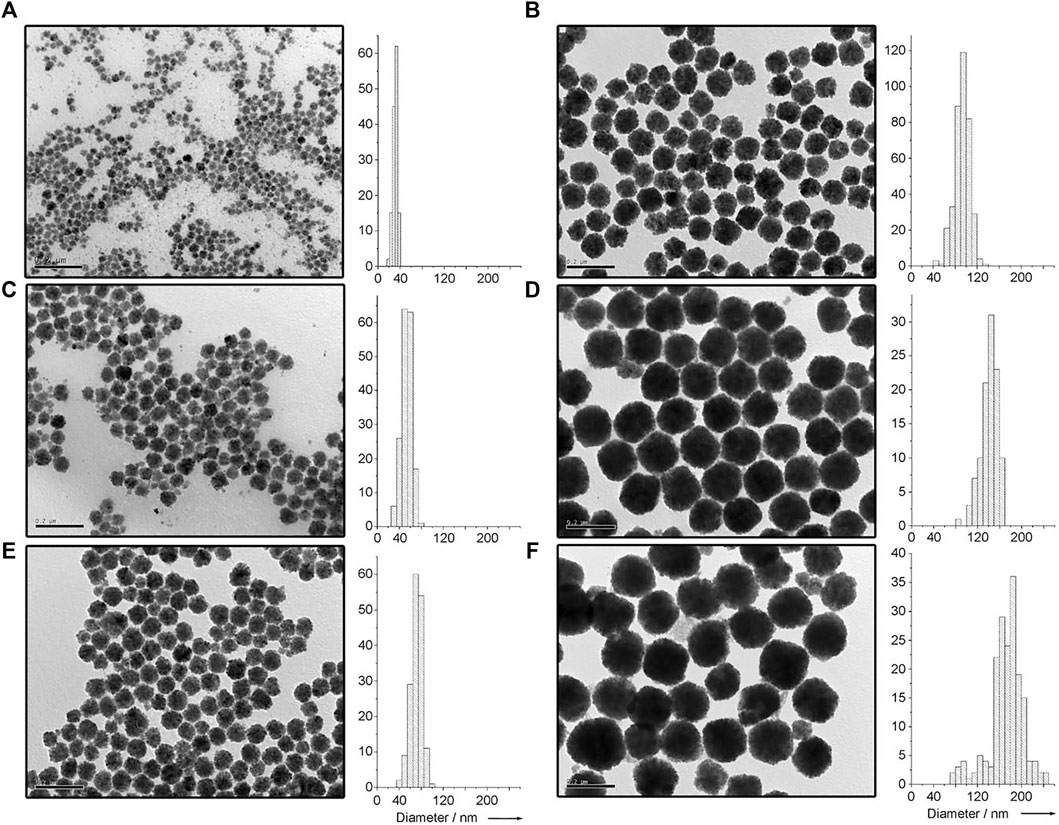
FIGURE 4. TEM images of MNPs. The average diameters of the MNPs can be controlled from 30 to 180 nm (A–F) by increasing the amount of NaOH (25 M) in the system. Reprinted with permission from Ge et al. (2007). Copyright© 2007 John Wiley and Sons.
In recent years, the one-step method has gradually become mainstream for the synthesis of MNPs. It has become increasingly popular to use simple and one-step methods for MNPs. This kind of method is generally used to combine the reaction precursor and pour it into the hydrothermal reaction kettle with a Teflon inner gallbladder at a certain temperature. Because of its simple operation, controllable conditions, and good repeatability, it is an ideal method to synthesize MRPCs MNPs. He’s group (Yang et al., 2016) and Yin’s group (Ge et al., 2007) used nearly the same raw materials to produce the high-stability MNPs (Figure 5A) through the simple thermal solvent “one-step method.” Because more PAA could be bound to the particle surface and crevices under the condition of a hot solvent and the prepared Fe3O4@PAA colloidal aqueous solution can maintain a high surface charge (−30 mV) in a longer period (30 days) (Figure 5B) while maintaining a relatively wide moving range of diffraction peaks after 3 months of storage (Figure 5C). In this case, at the same time, under the condition that the consistency of the product quality is assured, it could increase the reaction system by nearly 20 times and 2 g products could be obtained at most once (Figure 5D), which might increase the possibility for the industrialization and wider application of MRPCs.
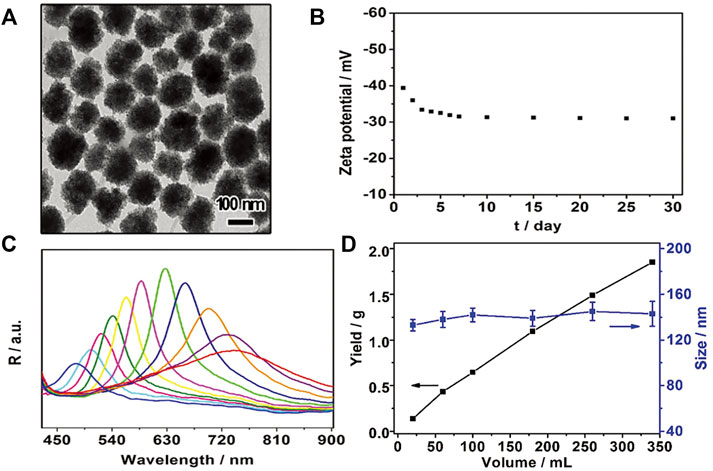
FIGURE 5. (A) TEM image of a 138 nm Fe3O4 CNC sample; (B) Evolution of zeta potential values of Fe3O4 CNCs dispersed in deionized water during storage for 30 days; (C) Reflection spectra of the 123 nm Fe3O4 CNC sample in aqueous suspension after storage for 3 months under different MFs achieved by changing the sample–magnet distance from 7 to 3 cm with a step of 0.5 cm; and (D) The mass yield and particle size of Fe3O4 CNCs obtained by hydrothermal reactions with different amounts of EG. Reprinted with permission from Yang et al. (2016). Copyright© 2016 Royal Society of Chemistry.
In another one-step method, Chen et al. (Wang et al., 2010) used ferrocene as the iron source to obtain the Fe3O4@C after the reduction of hydrogen peroxide (Figure 6A). The particle size of this method was homogeneous. Carbon nanostructures rich in carboxyl groups were uniformly coated on MNPs surface in the process of synthesizing MNPs, and this inert carbon coating greatly enhanced the stability of NPs colloids. After 8 months of storage in ethanol, the colloidal suspension still had a clear color response in the MF (Figures 6B–E). However, the temperature reaction time of this method was up to 72 h, which would reduce productivity.
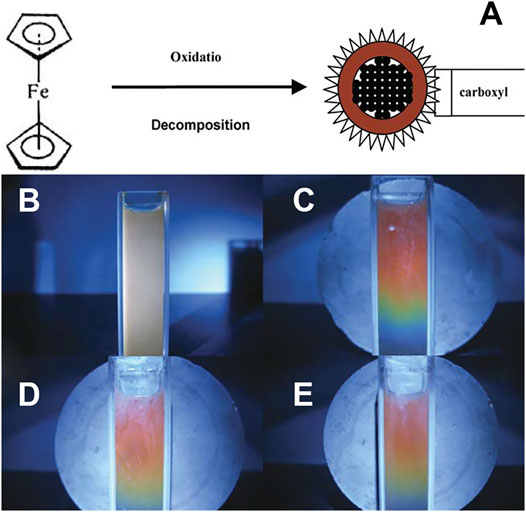
FIGURE 6. (A) Schematic of synthesis of carboxyl-functionalized magnetite/carbon core/shell nanoparticles; Black dot represents magnetite nanocrystals; red ring symbolizes the carbon; (B) Reflection photographs of the CNPs solution without MF; and (C–E) Reflection photographs of the CNPs solution under an external MF (0.20 T) with different stored time: (B,C) 0 m; (D) 4 m; (E) 8 m. Reprinted with permission from Wang et al. (2010). Copyright© 2010 Royal Society of Chemistry.
Another simple “one-step method” for synthesizing of MRPCs is the employment of hexahydrate ferric chloride as an iron source (Luo et al., 2014), sodium acetate to provide alkaline conditions, and PVP as a surfactant, the mixture is poured into the hydrothermal reaction kettle for 10 h after forming homogeneous solution in glycol. This method is simple, rapid, and the obtained particle size was easy to control. Dong (Dong et al., 2016) used the conical bottle, which was commonly used in the laboratory and instead of the inner gallbladder of the hydrothermal reaction kettle, directly as a container for high-temperature reactions; and it could effectively rule out the possibility of pollution caused by repeated use of the reaction kettle and greatly reduces the cost of the experiment. However, the feasibility and safety of this method is required to be further verified.
At present, most of the synthetic temperature of MNPs is around 200°C. Almost all of the solvents used to dissolve the precursors are organic solvents with high boiling points, such as ethylene glycol (EG), diethylene glycol (Wang W. et al., 2015), and acetone (Jia et al., 2015). Whereas, Liu (Liu et al., 2015) prepared the water-dispersible monodisperse hollow Fe3O4 microspheres via a one-pot hydrothermal process at RT with high saturation magnetization value of about 76.7 emu g−1. Due to its hollow properties, the magnetically induced assembly of NPs whose maximum particle size of 380 nm in aqueous solution could be realized and it exhibits great application potential in biomedicine, MRI agents, and color display areas. Besides, You (You et al., 2017) synthesized MRPCs by a three-step method. Firstly, MNPs were prepared by co-precipitation method, and then sodium lauryl sulfate was used as a surfactant to separate the MPs from each well. However, these particles did have the properties of PCs, and they needed to copolymerize with monomers and crosslinking agents and to form the polymers in multiple MNPs. With the increase of surfactant concentration, the magnetic saturation intensity would therefore be increased. Although the three-step method was relatively complicated, the generated MNPs could quickly respond to some compounds in an aqueous solution, which allowed it a great application for potential in optical sensors.
In the present period, as more attention on new materials, (i.e., MRPCs) is gradually being paid by researchers, higher demands are required for the synthesis method and efficiency of the basic materials. Therefore, it is imperative to develop synthesis methods that are simple, easy prepare, have easily-controllable conditions and particle size, and with high production efficiency.
The Formation of an Ordered Structure of MRPCs Under EMF
Specific structural colors could be generated by the periodic arrangement of MRPCs under MFs, even of an ordinary magnet. The induced color could be randomly controlled by MF size, particle size, and solvent; and the structural color does not easily fade. These unique properties grant MRPCs good application prospects in the fields of anti-counterfeiting, secret information storage, real-time MF detection, etc. Asher’s group (Xu et al., 2001a) set an important precedent by reporting Fe3O4@PS ferromagnetic fluid as early as 2001 (Figure 7). Under the MF, a regular PCs structure is formed under the drive of the kernel MNPs. Because of the low saturation magnetization of the colloidal MNPs, the response to EMF is relatively slow, which would take a few minutes or even 1 h, so that the wavelength of MRPCs prepared is narrow and the response time was relatively longer.
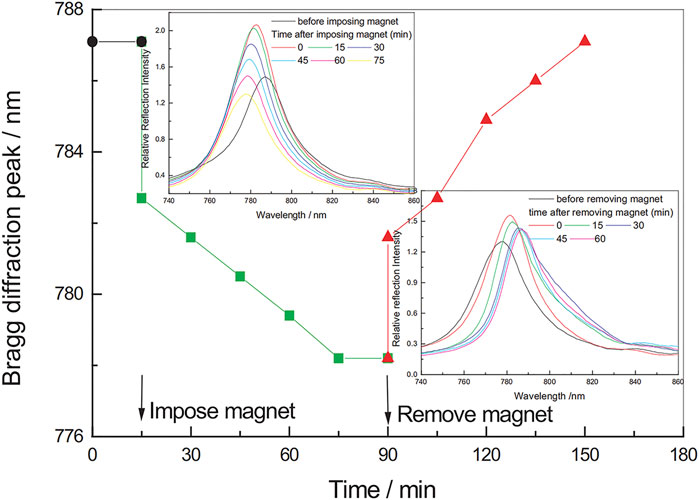
FIGURE 7. Response of superparamagnetic PCCA film to a 2.4 kOe MF with a 3.2 kOe/cm gradient. Reprinted with permission from Xu et al. (2001). Copyright© 2001 John Wiley and Sons.
Yin’s group (Ge et al., 2007) has advanced a big step in the research of MRPCs. The electrostatic repulsion provided by a large amount of charge on the surface of the Fe3O4 MNPs coated with PAA could separate MNPs from each other. In this way, a paramagnetic chain structure is formed rapidly (<1 s) under the MF. After coating a silicon ioxide shell (SiO2) on the surface, a large number of silica oxygen bonds can be well dispersed into organic solvents by controlling the thickness of the silicon shell, and the diameter of Fe3O4@SiO2 could be adjusted randomly. The ethanol solutions of Fe3O4 and Fe3O4@SiO2 with different particle sizes have different structural colors and diffraction peaks; therefore, the increase of particle size could result in the redshift of the diffraction peak.
Similarly, due to the different refractive indices in different solvents, MNPs with the same particle size in different solvents also showed different structural colors and diffraction peaks in different solvents under the equivalent EMF. However, the charge on the surface of NPs is difficult in the long-term storage in the water, and the surface charge will be reduced due to the separation of PAA on its surface, resulting in a narrow diffraction range of the PCs response. In the subsequent studies, it could be solved by hot reflux closure polyelectrolytes. Subsequently, the Yin’s group (Ge et al., 2009) combined the microfluidic technology with MRPCs, using microfluidic channels to polymerize the nano-level Fe3O4@SiO2 into tens of micron-sized photon microspheres (Figure 8A), and the color changes were clearly observed under the light microscope when the MF was applied (Figures 8B–G). The micron grade of material is very stable, well compatible with dispersion medium, and capable of rapid color change with the MF applied, which could be very suitable for color display, signal identification, biological and chemical detection, and MF sensor.
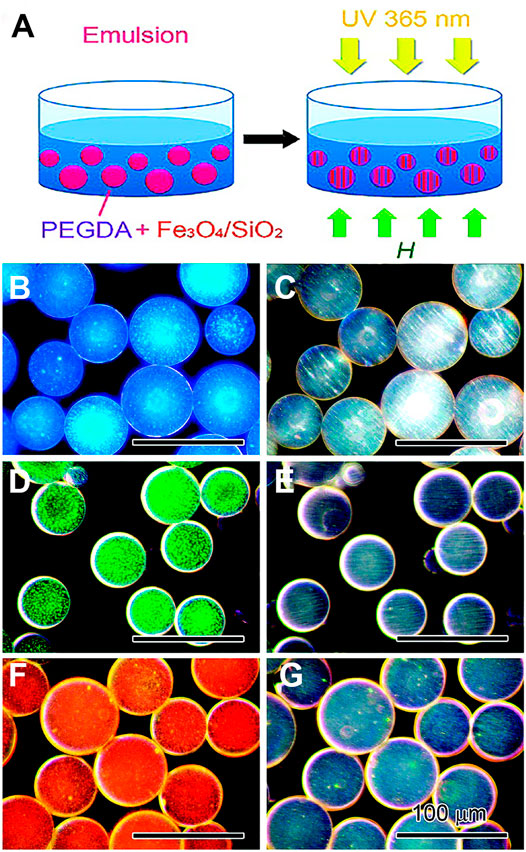
FIGURE 8. (A) Synthetic procedures for the magnetochromatic microspheres. When dispersed as emulsion droplets, superparamagnetic Fe3O4@SiO2 core/shell particles self-organize under the balanced interaction of repulsive and attractive forces to form one-dimensional chains, each of which contains periodically arranged particles diffracting visible light and displaying field tunable colors. UV initiated polymerization of the oligomers in emulsion droplets fixes the periodic structures inside the microspheres and retains the diffraction property; (B–G) Optical microscopy images (500×) of magnetochromatic microspheres with diffractions switched between “on” (B,D,F) and “off” (C,E,G) states by using EMFs. These microspheres are prepared using (B,C) 127, (D,E) 154, and (F,G) 197 nm Fe3O4@SiO2 colloids. Reprinted with permission from Ge et al. (2009). Copyright© 2009 American Chemical Society.
Liu’s group (Tang et al., 2018) fabricated a hydrophilic and size-controllable Fe3O4@poly (4-styrenesulfonic acid-co-maleic acid) (PSSMA)@SiO2 MRPC by orderly assembling of the core-shell colloidal nanocrystal clusters (CNC) via a two-step facile synthesis approach under EMF in aqueous solution. Figure 9A demonstrates a rainbow-like color effect within 1 s when the prepared MRPC was placed in a circular shaped disk-like NdFeB magnet. Figure 9B displays the intensity distribution of the EMF simulated by COMSOL Multiphysics 5.3. This was the verification of MRPC formation.
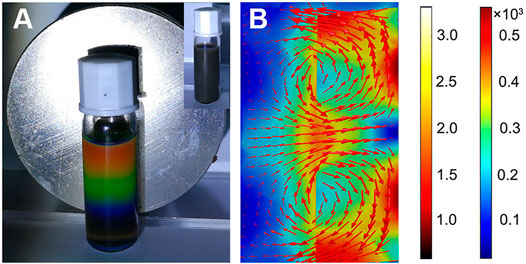
FIGURE 9. (A) Photo of the rainbow-like colors effect displayed with a NdFeB magnet with circular disk shape. Inset: A bottle of Fe3O4@PSSMA@SiO2 CNCs without magnet placed (5 mg/ml in aqueous solution and the size is 220 nm). (B) The EMF strength distribution simulated by COMSOL Multiphysics 5.3. Copyright© 2018 American Chemical Society.
MRPCs relying on the long-range steric hindrance effect were firstly reported by Guan’s group (Luo et al., 2014). The force is provided by the macromolecular PVP which wraps the surface of MNPs. In the organic solvent dimethylformamide, with the increase of the MF, the reflection peak was blue-shifted with a width of 280 nm which has been considered as the widest diffraction peak displacement of the MRPCs reported in the organic phase. Whereas the diffraction peaks of NPs with the same particle size only shifted 238 and 200 nm in ethanol and dichloromethane. Both the electric field and MF are similar referring to the regulation of MNPs arrangement. Joo (Joo et al., 2014) reported PCs that can be either induced by MF and electric field, the hydrophobic group, and the positive charge modified Fe2O3@SiO2, which could be well distributed in polar and non-polar solvents. The electrostatic repulsive force can be balanced with the magnetic dipole attraction between particles, and a bright color response would occur under EMF. When the same magnetic colloid solution was injected into the conductive glass interlayer, the structure colors were similar to those in EMF which could be assembled under the electric field due to the repulsive force of the electric field. As same as the MF, along with the increasing of the electric field, the diffraction peak also takes a blueshift.
Induced by MF, the MNPs of MRPCs with different particle sizes are characterized as periodic permutations of dielectric constants. Chen’s group (Hu et al., 2012) mixed Fe3O4@C MNPs with two different particle sizes in EG solution, and then sealed the result in polydimethylsiloxane gel film in the form of micron droplets. After self-assembling by magnetic inducing, MRPCs containing a two-photon bandgap structure were prepared and successfully achieved photon toning, which is difficult for ordinary pigments or chemical dyes to imitate. It could be used as a new type of anti-counterfeiting identification, and also provides the idea of multi-channel detection for sensing technology.
Compared with the general magnetic materials, MRPCs not only have good magnetic response effect but are also characterized as a monodispersing and periodic arrangement under MF as well as having good application prospects when combined with other technology. It was reported liquid fluorescence enhanced substrates consisting of suspensions of Fe3O4 NPs could assemble 3D PCs under EMF (Hu et al., 2016). Combining the dye molecules whose incident wavelength overlapping with the PCs photonic bandgap on the surface of MRPCs, the periodic PCs can provide a continuous and highly ordered substrate, whereby enhancing the collection efficiency of fluorescence incident, and reaching each up to 12.3-fold fluorescence enhancement without the utilization of metal particles (Hu et al., 2016). They also emphasized that the dynamic modulation and precise control of the photonic bandgap of the liquid Fe3O4 PC system may significantly contribute to more accurate and flexible applications of fluorescence enhancement. Kwon’s group (Kim et al., 2012) modified amino on the surface of Fe3O4 wrapped in silicon shell and further coated the shell structure of Au NPs. It also reported that, under EMF, the magnetic core could cause the promotion of Au NPs to form a periodic one-dimensional chain structure, and the introduction of the Au NPs has expanded the application of MRPCs in localized surface plasmon resonance, such as the surface-enhanced Raman matrix construction (Liu et al., 2017), whereby the orderly structure makes the MRPCs difference from general magnetic materials, and can significantly improve the sensing Raman signal by the coupling of the core-shell structure owing to the high surface to volume ratio of PC beads as well.
The Non-Spherical Materials Self-Assemble Into PC Structure Under EMF
Besides the spherical magnetic materials, the non-spherical materials, such as elliptic and fusiform, also can be quickly assembled into PCs under magnetic induction. Clays’s group (Ding et al., 2009) prepared the elliptical and densely packed Fe2O3@SiO2 by direct convective self-assembly with the aid of EMF (Figures 10A–E). The PCs of this cube lattice have a bright structural color, and the color takes a blueshift when the axial proportion of ellipsoid is larger. Ellipsoidal NPs have special properties under MF due to their unique structure which could respond to the direction of the MF. Accordingly, Fe@SiO2 nano-ellipsoids as anisotropic building blocks. Wang (Wang M. et al., 2015) employed the ellipsoidal FeOOH@SiO2 colloidal solution to respond to the direction of applying the MF. When the direction of the MF which used to be perpendicular to the incident angle becomes parallel to the incident angle, the diffraction peak takes a blueshift up to 210 nm. Compared with the spherical magnetic colloidal solution which only has two colors on the Halbach magnet array (Wang et al., 2013), due to its response to the different MF directions of ellipsoidal special structure, three different color responses could occur on the Halbach array (Figure 10F).
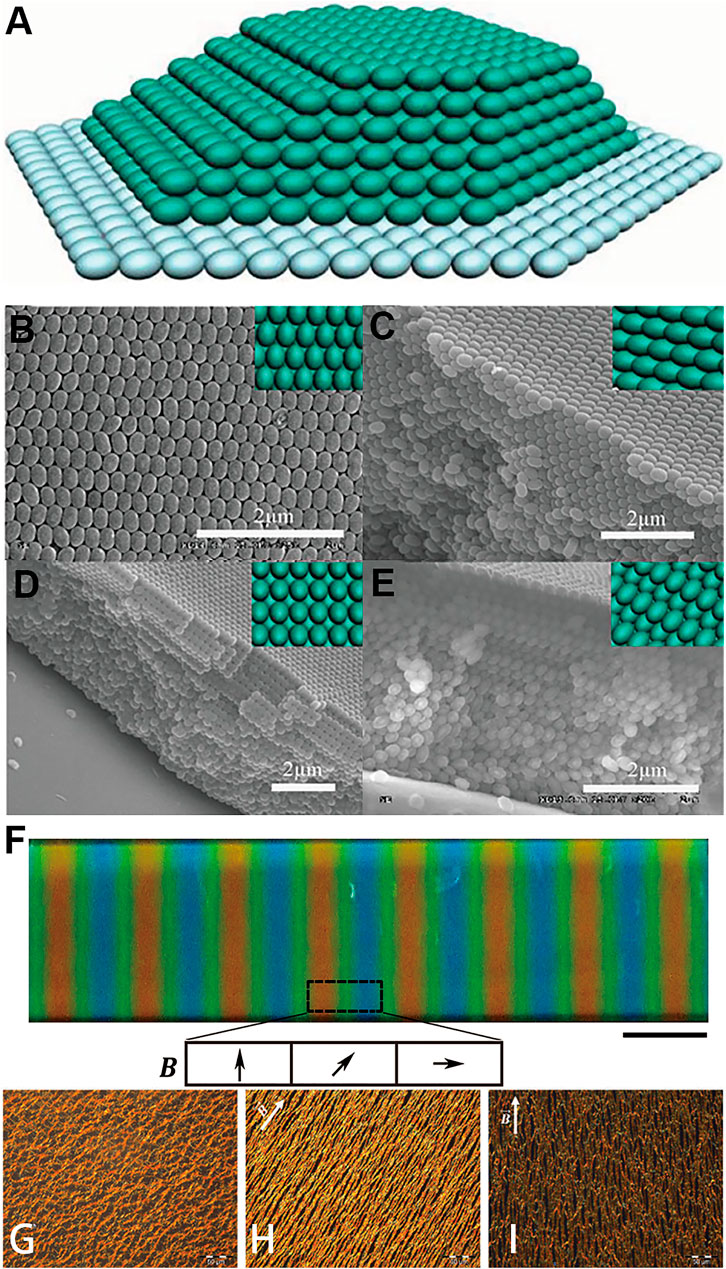
FIGURE 10. (A) 3D model of an assembled colloidal crystal of ellipsoidal building blocks with the aspect ratio of 1.5; (B–E) SEM images of different crystalline planes. Scale bars: 2 mm. The figure insets show the models of the corresponding crystalline planes. Reprinted with permission from Ding et al. (2009). Copyright© 2009 John Wiley and Sons; (F) Digital photo showing the photonic response of nano ellipsoids encapsulated in a flat glass tube under a non-ideal linear Halbach array. The scale bar corresponds to 5 mm. Reprinted with permission from Wang M. et al. (2015). Copyright© 2015 John Wiley and Sons; (G–I) Polarized optical microscope images with crossed polarizers showing (G) dispersions of NRs functionalized with PDEGMEMA in excess P(DEGMEMA-b-DOPA), (H) its alignment under an applied external MF; and (I) the rotation of the external MF by 45°. The scale bar in all images is 50 mm. Reprinted with permission from Klöckner et al. (2017). Copyright© 2017 Royal Society of Chemistry.
Klöckner (Klöckner et al., 2017) modified the surfactant outside the fusiform Fe3O4 nano-rod to enable a large amount of charge on its surface. The space resistance repulsion generated by the outer wrapping polymer could effectively reduce the aggregation of NPs and show good dispersibility so that it can be very well dispersed in any solvent. With the incident angle of 45°, the “meteor” shape could be observed in an optical microscope under the MF, which intuitively shows that the fusiform magnetic sphere could also be arranged in the direction of MF so as to form a periodic PCs structure (Figures 10G,H).
He’s group developed, tailored, and assembled ellipsoidal particles into PC supraparticles (PCSs) with unusual non-close-packed structures with a spatially confined magnetic assembly strategy (Liu J. et al., 2019) which exhibits anisotropic optical properties and multiple magneto-optical responses. Based on the above-mentioned, the periodic structure of PCs is not confined to the spheres, and the formation of PCs by non-spherical magnetic material under the MF also further have validated the regulation of MNPs by the MF. This non-spherical material has shape-dependent physical and chemical properties, which could provide new ideas for the composition and display of non-spherical MRPCs photons, such as a color display that can be used to detect the direction of MFs.
Yin’s group (Li et al., 2021) reported the construction of magnetite nanorods by magnetic assembly into tetragonal colloidal crystals and demonstrated that the assembly method caused the nanorods to be assembled along a size-dependent critical angle rather than the simple end-on attachment. The unconventional assembly manner was determined by the coupled shape and magnetic anisotropy and it led to the non-close-packed and hard-contact phase. The novelty of the work lies in preparing the body-centered tetragonal colloidal crystals by manipulating magnetic interactions of various anisotropically shaped nanostructures which are actively tuned by changing the magnetic field direction.
The Non-magnetic Materials Self-Assembling Into PC Structure Under EMF
Non-magnetic materials in magnetic fluids have similar properties to magnetic materials under MFs, therefore not only magnetic materials but also non-magnetic materials could be arranged and assembled into ordered structures under EMF so as to form PCs structures. Non-magnetic liquid mixed with magnetic colloids could become anti-magnetic liquids, this concept was first proposed by Skjeltorp (Skjeltorp, 1983). It was proposed that the non-MPs incorporated into the magnetic fluid were named magnetic holes forming a variety of different lattices. He (He et al., 2010) investigated the phase transitions of the diamagnetic liquid containing the PS microspheres using EMF. Non-magnetic polymer beads measuring 185 nm were assembled into PC structures, from 1D chains to 3D assemblies as determined by the interplay of magnetic dipole force and packing force.
The structural color could be significantly blue-shifted with increasing MF (Figures 11A,B). Also, the intensity of the diffraction peak continuously keeps rising within 3 min and 30 s after the application of a strong MF (2500 Gs), and even continued to 6 min after the removal of MF, could be up to a maximum of 83%, which is far beyond the maximum of magnetically induced material and then slowly decreases within 20 min (Figures 11C,D). In short, this is the entire process of magnetization and demagnetization of PS microspheres with negative charges on the surface of the magnetic fluid. They also demonstrate the magnetic assembly of Janus PCSs with increased complexity from a colloidal mixture of nonmagnetic spheres and magnetic ellipsoids (Xiao et al., 2021). According to these studies, PS microspheres can also respond well under EMF, which provides new technical means for the magnetic control of non-MPs.
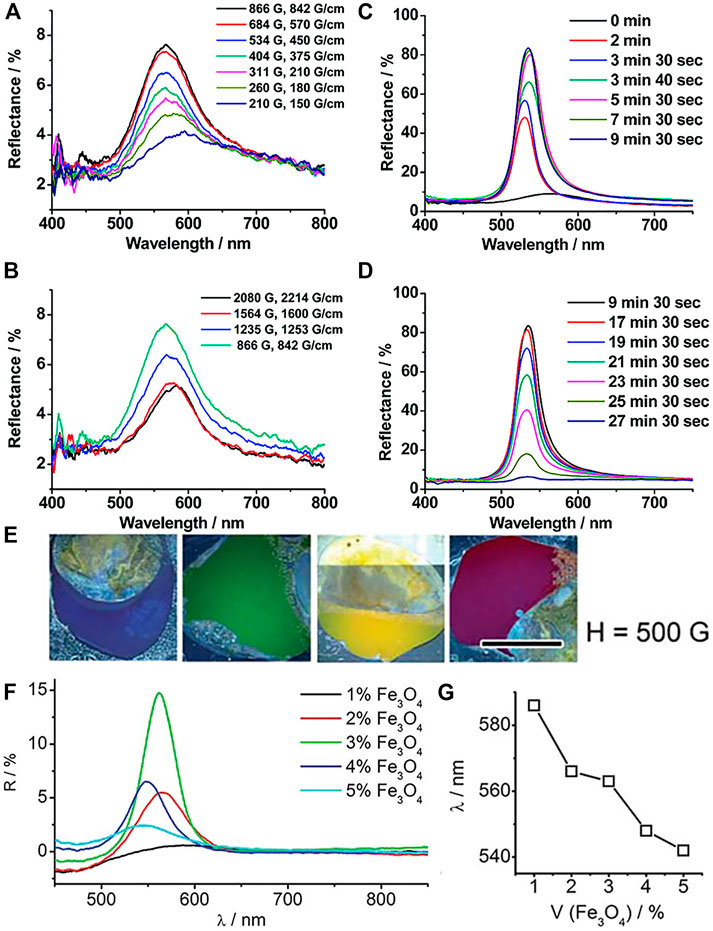
FIGURE 11. (A,B) Reflection spectra of the 1 mm thick film of mixed PS beads and ferrofluid solution in response to an external MF with varying strengths. The volume fractions are 4% for both PS and Fe3O4; (C,D) Time-dependent reflection spectra of the 1 mm thick liquid film of mixed PS and ferrofluid solution in response to an MF of 2530 G with a gradient of 2500 G/cm. The MF was removed at 3 min 30 s. The volume fractions are 4% for both PS and Fe3O4. Reprinted with permission from He et al. (2010). Copyright© 2010 American Chemical Society. (E) The brown mixture of the PS colloids and the ferrofluid shows a blue, green, yellow, and red color in an MF of 650 Gauss, where the PS size is 311, 348, 397, and 470 nm, respectively. (F) The reflection spectra of the colloidal assemblies in ferrofluids with various magnetite volume fractions (Fe3O4%); (G) The influence of the Fe3O4% on the reflection wavelength. Reprinted with permission from Ge et al. (2012). Copyright© 2012 Royal Society of Chemistry.
Asher’s group (Xu et al., 2002) employed a 134 nm PS sphere and a mixed colloidal solution of Fe3O4 or Co3O4 to form a PC under MF, but the assembly time was as long as 60 min due to its low contents of magnetic material. Besides, Deng (Deng and Li, 2013) observed the dynamics of the formation of different structures by assembly of non-magnetic spheres, i.e., micro-sized PS microspheres and nano-sized Fe3O4 magnetic colloid under an EMF. When MF is parallel to the direction of the incident light, PS microspheres were arranged from the original random distribution to the chain-type arrangement under MF. Along with the repulsion shown by the adjacent chains this structure has a certain threshold value for the MF strength; when applying a vertical MF, PS microspheres attracted each other along the direction of the MF to form a short chain structure. Subsequently, Ge (Liu et al., 2012) synthesized PS with different particle sizes (311–477 nm). Under the weaker MF (650 Gs), PC structural color changes from blue to purple with the increase of particle size (Figure 11E). The peak intensity of non-magnetic PS first increased and then decreased with the increase of the concentration of the magnetic fluid solution, reaching the highest value at 3% (Figure 11F), and the wavelength decreased continuously with the increase of the concentration of the magnetic fluid solution (Figure 11G). The non-magnetic PCs of this system are relatively stable as they still exhibit good reproducibility after multiple repetitions, and they could also maintain consistent peak intensity under a continuous 60-min MF.
The assembly of non-magnetic materials in magnetic fluids is fast and efficient. However, due to the natural adsorption of MNPs by MFs, it could only remain stable in liquids for a certain period. Recently, Ge’s group (Zhang et al., 2017) prepared micro-scale SiO2 inverse opal by emulsion polymerization and UV-curing on the previous basis and then mixed it with the magnetic fluid (Figure 12A). Under the MF, the response time decreased with the increase of the MF strength, showing an exponential correlation (Figure 12B); whereas the reflectivity increases with increasing MF intensity which is linearly correlated (Figure 12C). This system could simultaneously detect the relevant MF strength of both the indicator response time and reflectivity. The minimum detectable MF is 5.4 mT. The principle of this magnetometer is only magnetic tuning instead of magnetic assembly; therefore, it has subtly avoided the shortcomings of traditional magnetic fluids in measuring magnetic intensity by using magnetic assembly optical signals in the past, that is, avoiding the PCs structural colors and reflection peaks being too sensitive to EMF and also avoiding the reflectivity varies with the applied MF time (He et al., 2012).
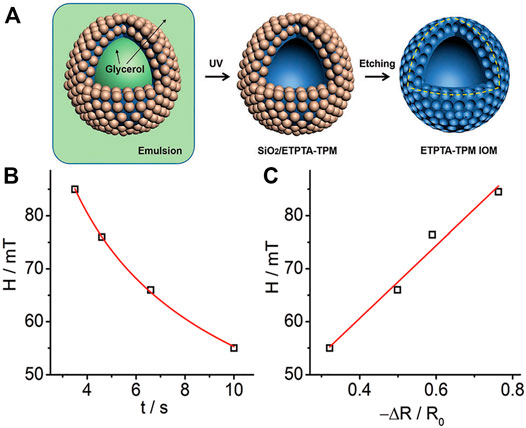
FIGURE 12. (A) Synthesis of hollow ETPTA-TPM IOMs by UV curing the double emulsion droplet in glycerol and etching the silica content from the shell of microspheres; (B) Negative correlation between field strength (H) and quenching time (t); and (C) Positive correlation between field strength (H) and quenching ratio (−∆R/R0). Reprinted with permission from Zhang et al. (2017) Copyright© 2017 Royal Society of Chemistry.
The combination of MRPCs with microfluidic technology and the artificial aggregation of nanometer PCs into micron scales have achieved the free control of micron-size PCs. Lee (Lee et al., 2017) synthesize the “Janus” microspheres microfluidic devices to include the PS and magnetic balls in equal proportion by the microfluidic devices. Since half of them were made of magnetic materials, when the incident light and MF direction was changed from 0° to 60°, the diffraction wavelength of the other half-formed PCs would shift accordingly. When the rotation angle is 180°, the orderly arrangement of PCs disappears instantaneously. This material not only has a stable PC structure but also has MF responsiveness; therefore, it could store photon signals and served as a carrier for biological materials (Shin et al., 2017). The magnetically induced non-magnetic material has a stable response signal and responds in a weak MF. Therefore, it has a good prospect in PCs magnetometer and photon color display.
Research Progress in Response Application of MRPC
The responsiveness of PCs refers to the influence of external environmental changes on its photonic bandgap structure. This responsiveness endows PCs with unique sensing characteristics, enabling the conversion of various external environmental responses into optical signals. MRPCs have the advantage of rapid assembly, which attracted much attention in the study of the responsiveness of PCs. The responsiveness of MRPCs could be easily classified as physical (temperature, humidity, pressure, etc.) and chemical (salt ion concentration and small molecule concentration) responsiveness. Mixing the magnetic material with a gel (such as acrylamide electrophoresis gel) could immobilize the MNPs; therein, MPs are induced to self-assemble into PCs. With the other external conditions such as strength and direction of the MF are not changed, according to Bragg’s diffraction law, the periodical changes of PCs caused by external conditions would lead to the change of λ. If changing one of the external conditions, it also would be altered of the diffraction structural color which was visible to the naked eye in the macroscopic. Due to its special and rapid assembly method, this kind of MRPCs is widely used in the response of PCs.
Magnetic Induction of Physical Responsive PCs
Physical parameters such as humidity, temperature, pressure, etc., greatly impact the periodic structure of PCs. Yin’s group (Wang et al., 2014) reported a light-printable rewritable paper based on color switching of commercial redox dyes which were introduced as the imaging layer and used titanium oxide-assisted photocatalytic reactions (Figure 13).
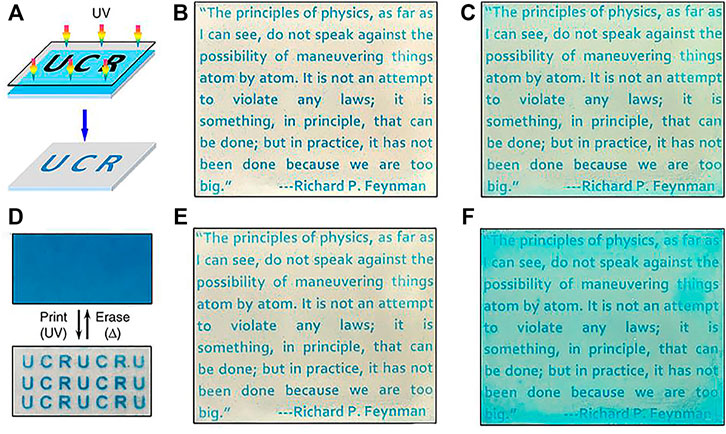
FIGURE 13. Printing, erasing, and legibility of letters on the rewritable paper. (A) Schematic representation of writing letters on the rewritable paper using photomask on UV light irradiation; (B) digital images of writing and erasing letters on the rewritable paper; Digital images of rewritable paper maintaining in ambient air after writing of (C) 10 min, (D) 1 day, (E) 3 days and (F) 5 days. Scale bars, 5 mm. The photomask was produced by ink-jet printing on plastic transparency. The slight variation in the background was due to the uneven thickness of the film resulting from the manual drop-casting. Reprinted with permission from Wang et al. (2014). Copyright© 2014 Springer Nature.
Fe3O4@SiO2 colloidal NPs were immobilized in a PEGDA array and rapidly magnetically induced to self-assemble into PCs. After contacting the “ink” of different humidity represented by the saturated salt solution, the interval diameter of the particles in the polymer matrix in the contact area increases, contributing to a redshift of the diffraction peak, and it’s very stable at RT and normal humidity. In addition, the residual salts on the surface of the ink-free light-printable rewritable paper could be washed away by using the distilled water, and the “ink” can also be wiped off. After it dried, the light-printable rewritable paper would be returned to its original state and could be recycled at least 20 times (Figures 13A–F). As the poly-N-isopropyl acrylamide (NIPAM) gels have hydrophilic amide and hydrophobic isopropyl on the macromolecular chain at the same time, the cross-linked PNIPAM is characterized as temperature-sensitive.
Guan’s group (Luo et al., 2017) have developed the first 1-D magnetic PC balls with tunable lattice constants by fixing collectively oriented periodical 1-D magnetic nanochain-like structures in responsive polymer PNIPAM hydrogel balls under magnetic field (H) and UV irradiation. They employed PNIPAM as the crystal structure to build the matrix, i.e., the MNPs and the gel mixture were then injected into the microinjection solution, and the microsphere-sized polymer spheres were subsequently prepared by dropwise addition to induce into PCs in a fixed MF. When the temperature rises from 10 to 40°C, the structural color changes from red to yellow, and then to green, and the temperature is inversely correlated with the wavelength of the reflected peak. Similarly, Wang (Wang et al., 2016) immobilized the Fe3O4@C in PNIPAM gel. In this case, the temperature changed in the range of 15–26°C which initiated a blueshift of 100 nm. The change from green to dark blue was visible by the naked eye, and the temperature-sensitive test paper was made with 5-time repeatability.
You (You et al., 2015) mixed the product of polymerization of Fe3O4@OA with monomer and crosslinker with polyacrylamide gel, and polymerization is initiated by using EMF, and the diffraction peak intensity of the obtained product film could take a blueshift with the stretch of external force and generate a redshift with extrusion because stretching reduced the interval between adjacent particles in a self-assembled single chain. Accordingly, Wang (Wang et al., 2017) offered simultaneous multicolor displayed on the photopolymerization of hydrogel under MF. Under a tensile force of 0–12 kPa, with the tensile force increased the structural color changed from brown to blue, whereby, a blueshift could reach 250 nm, and a gradient color card was also made by the change of color with tension. The color fixed by PCs would not fade over time, with long-term stability which not only provides a good prospect for real-time pressure sensing but also broadens the application of PCs hydrogels in fast-response sensors. Research on MRPCs’ temperature, humidity, and pressure response properties is an interesting exploration of the physical response of MRPCs. These rapid, reversible, and visualized features will broaden the application of physicochemical sensors, and renewable photonic papers and inks.
Magnetic Induction of Chemical Responsive PCs
The uniform and orderly arrangement of MNPs colloids in an MF is a dynamic equilibrium system. Changes in conditions in the system, such as salt ion concentration and compound concentration, would destroy this dynamic equilibrium and cause it to be rearranged into a new equilibrium, which would result in changes in the PCs cycle. The nano-colloids such as Fe3O4@PAA could build the MRPCs which rely on the interaction between the surface electrostatic repulsion and the magnetic dipole force. The electrons in the surface layer become thinner as the ion concentration increases and the electrostatic repulsion would also decrease or even disappear. As a result, when the concentration of salt ions increases to a certain concentration, MRPCs’ suspension would lose its color response. While MNPs colloids rely on the long-range repulsive provided by MNPs surface macromolecule flexible polymer PVP (Luo et al., 2014), the salt ions have little effect on their surface spatial conformation, and therefore the long-range repulsion of the surface remains unchanged. And the suspension of the MF could be tuned to a wavelength of 700–460 nm with a salt concentration of 0–100 mM. Chen’s group (Wang et al., 2016) put the PNIPAM gel immobilized with Fe3O4@C into the concentration gradient of 200–1,200 mM NaCl solution. They suggested that with increasing salt ion concentration, the gel color was gradually changed from green to dark blue and could be observed by the naked eyes along with the blueshift of the responsive diffracted wavelength, and the color response to salt concentration could be completed in a matter of seconds with good repeatability. The salt ion concentration test strip manufactured on this principle has the advantages of fast response and portability and is suitable for rapid on-site detection.
You (You et al., 2015; You et al., 2016) combined MRPCs with molecularly imprinted technology with specific recognition to achieve an optical response to melamine and L-phenylalanine. The surface of Fe3O4@OA was coated with a molecularly imprinted polymer, and the holes left after removing the template molecule had a specific recognition effect on the template molecule. Under the action of a certain MF, the specific recognition of template molecules with different concentrations could be expressed directly through the optical signal and could be visible to the naked eye. The study was able to realize the visual detection of 10−2–10–5 mg/ml melamine and 6.0 × 10−7–6.0 × 10–4 ml-phenylalanine, which only took 5 min with a diffraction shift of 200 nm. Although the response system is fast and reversible, its stability, specificity, and responsiveness to actual samples are a long way to go.
At present, although the application of MRPCs’ responsiveness still focuses on some simple physical and chemical properties, the advantages of MRPCs have been fully reflected and laid the foundation for their application development. In the future research of MRPCs, the on-site rapid detection of environmental pollutants, clinical targets, and biological macromolecules will be the focus of researchers’ attention and development.
Conclusion
In recent years, MRPCs have received more and more attention. Compared with other materials, it has many performance advantages: the speedy assembling process which only takes only a few seconds; the photonic bandgap is easily tuned which could control the reflection peaks by controlling particle size, solvent, and MF size, and achieve arbitrary regulation in the whole spectrum; PCs can be formed in solvents including water under the MF, thereby broadening their application in the biological field. Photonic band gaps and colors of MRPCs are responsive to external environments such as temperature, humidity, pressure, salt ion concentration, and small molecule compounds. Macroscopically, these changes could produce visible color changes in the naked eye. Therefore, MRPCs are prominent in application in the fields of anti-counterfeiting, physical and biological sensors, chemical contaminant detection, and monitoring. Current researches on MRPCs are still in the exploratory stage and there are still faces many technical troubles and challenges, including some optical mechanisms are required to be further explored; further research is needed to govern the interaction between MRPCs; currently, most of the researches on MRPCs have stayed in the field of materials and optics; therefore, the cross-complementation of multi-disciplinary and multi-disciplinary fields and the expansion of applications of MRPCs in the fields of biology and rapid detection are necessary, and they would become the focuses of future researches.
Author Contributions
NL: validation, investigation, and writing-original draft. FZ and LD: conceptualization, writing and editing the review, and funding acquisition. PW and RA: methodology and conceptualization. ST, PL, and CW: conceptualization, methodology, and funding acquisition. YL and LD: resources. All authors contributed to the article and approved the submitted version.
Funding
We are grateful for the financial support by the National Natural Science Foundation of China (No. 81872584), the National 863 Young Scientist Program (No. 2015AA020940), Natural Science Foundation of Guangdong Province (No. 2016A030313138), Key Projects of Guangzhou Science and Technology Program (No. 201704020056), Natural Science Foundation of Shenzhen (No. JCYJ20210324093211030), the Medical Scientific Research Foundation of Guangdong Province (No. A2020490), Military Logistics Research Project (No. CKJ20J031), and Interdisciplinary Research for First-class Discipline Construction Project of Henan University (No. 2019YLXKJC04).
Conflict of Interest
The authors declare that the research was conducted in the absence of any commercial or financial relationships that could be construed as a potential conflict of interest.
Publisher’s Note
All claims expressed in this article are solely those of the authors and do not necessarily represent those of their affiliated organizations, or those of the publisher, the editors and the reviewers. Any product that may be evaluated in this article, or claim that may be made by its manufacturer, is not guaranteed or endorsed by the publisher.
References
Chen, Z., Xue, L., Yu, B., Liu, X., and Cong, H. (2014). Fabrication and Application Progress of Colloidal Crystals. Chin. Sci. Bull. 59 (9), 752–762. doi:10.1360/972013-897
Deng Hai-Dong, H., and Li Hai, H. (2013). Interaction and Assembly of Non-magnetic Spheres and Magnetic Nanoparticles Dispersed in Magnetic Fluid. Acta Phys. Sin. 62 (12), 127501. doi:10.7498/aps.62.127501
Ding, T., Song, K., Clays, K., and Tung, C.-H. (2009). Fabrication of 3D Photonic Crystals of Ellipsoids: Convective Self-Assembly in Magnetic Field. Adv. Mater. 21 (19), 1936–1940. doi:10.1002/adma.200803564
Dong, Y., Wen, B., Chen, Y., Cao, P., and Zhang, C. (2016). Autoclave-Free Facile Approach to the Synthesis of Highly Tunable Nanocrystal Clusters for Magnetic Responsive Photonic Crystals. RSC Adv. 6 (69), 64434–64440. doi:10.1039/c6ra10355c
Ge, J., Hu, Y., Biasini, M., Beyermann, W. P., and Yin, Y. (2007). Superparamagnetic Magnetite Colloidal Nanocrystal Clusters. Angew. Chem. Int. Ed. 46 (23), 4342–4345. doi:10.1002/anie.200700197
Ge, J., Lee, H., He, L., Kim, J., Lu, Z., Kim, H., et al. (2009). Magnetochromatic Microspheres: Rotating Photonic Crystals. J. Am. Chem. Soc. 131, 15687–15694. doi:10.1021/ja903626h
Gu, J., Chen, J., Zhang, D., and Yang, L. (2014). The Optical Response of Co-plated Butterfly Wing Scales Controlled by a Magnetic Field. Chin. Sci. Bull. 59 (25), 2499–2504. doi:10.1360/n972014-00048
He, L., Hu, Y., Kim, H., Ge, J., Kwon, S., and Yin, Y. (2010). Magnetic Assembly of Nonmagnetic Particles into Photonic Crystal Structures. Nano Lett. 10 (11), 4708–4714. doi:10.1021/nl103008v
He, L., Janner, M., Lu, Q., Wang, M., Ma, H., and Yin, Y. (2015). Magnetochromatic Thin-Film Microplates. Adv. Mater. 27 (1), 86–92. doi:10.1002/adma.201403836
He, L., Wang, M., Ge, J., and Yin, Y. (2012). Magnetic Assembly Route to Colloidal Responsive Photonic Nanostructures. Acc. Chem. Res. 45 (9), 1431–1440. doi:10.1021/ar200276t
Hong, X., Peng, Y., Bai, J., Ning, B., Liu, Y., Zhou, Z., et al. (2014). A Novel Opal Closest-Packing Photonic Crystal for Naked-Eye Glucose Detection. Small 10 (7), 1308–1313. doi:10.1002/smll.201302788
Hu, F., Gao, S., Zhu, L., Liao, F., Yang, L., and Shao, M. (2016). Tunable Fluorescence Enhancement Based on Bandgap-Adjustable 3D Fe3O4nanoparticles. Nanotechnology 27 (24), 245709. doi:10.1088/0957-4484/27/24/245709
Hu, H., Chen, Q.-W., Cheng, K., and Tang, J. (2012). Visually Readable and Highly Stable Self-Display Photonic Humidity Sensor. J. Mater. Chem. 22 (3), 1021–1027. doi:10.1039/c1jm14463d
Hu, Y., Wang, J., Li, C., Wang, Q., Wang, H., Zhu, J., et al. (2013). Janus Photonic Crystal Microspheres: Centrifugation-Assisted Generation and Reversible Optical Property. Langmuir 29 (50), 15529–15534. doi:10.1021/la404082y
Jia, X., Wang, J., Wang, K., and Zhu, J. (2015). Highly Sensitive Mechanochromic Photonic Hydrogels with Fast Reversibility and Mechanical Stability. Langmuir 31 (31), 8732–8737. doi:10.1021/acs.langmuir.5b02134
John, S. (1987). Strong Localization of Photons in Certain Disordered Dielectric Superlattices. Phys. Rev. Lett. 58 (23), 2486–2489. doi:10.1103/PhysRevLett.58.2486
Joo, A., Kim, Y.-S., Cho, H. M., Lee, C. J., and Kim, Y. (2014). Tunable Assembly of Hydrophobic and Cationic Charged Fe3O4/SiO2 Colloids. J. Nanosci. Nanotechnol. 14 (11), 8549–8553. doi:10.1166/jnn.2014.9983
Katagiri, K., Tanaka, Y., Uemura, K., Inumaru, K., Seki, T., and Takeoka, Y. (2017). Structural Color Coating Films Composed of an Amorphous Array of Colloidal Particles via Electrophoretic Deposition. NPG Asia Mater. 9 (3), e355. doi:10.1038/am.2017.13
Kim, L. N., Kim, E.-G., Kim, J., Choi, S.-E., Park, W., and Kwon, S. (2012). Fabrication and Manipulation of Gold 1D Chain Assemblies Using Magnetically Controllable Gold Nanoparticles. Bull. Korean Chem. Soc. 33 (11), 3735–3739. doi:10.5012/bkcs.2012.33.11.3735
Klöckner, B., Daniel, P., Brehmer, M., Tremel, W., and Zentel, R. (2017). Liquid Crystalline Phases from Polymer Functionalized Ferri-Magnetic Fe3O4nanorods. J. Mater. Chem. C 5 (27), 6688–6696. doi:10.1039/c7tc01106g
Lai, X., Ren, Q., Vogelbacher, F., Sha, W. E. I., Hou, X., Yao, X., et al. (2022). Bioinspired Quasi‐3D Multiplexed Anti‐Counterfeit Imaging via Self‐Assembled and Nanoimprinted Photonic Architectures. Adv. Mater. 34 (3), 2107243. doi:10.1002/adma.202107243
Lee, J., Seo, J., Kim, D., Shin, S., Lee, S., Mahata, C., et al. (2014). Capillary Force-Induced Glue-free Printing of Ag Nanoparticle Arrays for Highly Sensitive SERS Substrates. ACS Appl. Mater. Inter. 6 (12), 9053–9060. doi:10.1021/am5000382
Lee, S. Y., Choi, J., Jeong, J.-R., Shin, J. H., and Kim, S.-H. (2017). Magnetoresponsive Photonic Microspheres with Structural Color Gradient. Adv. Mater. 29 (13), 1605450. doi:10.1002/adma.201605450
Li, Z., Qian, C., Xu, W., Zhu, C., and Yin, Y. (2021). Coupling Morphological and Magnetic Anisotropy for Assembling Tetragonal Colloidal Crystals. Sci. Adv. 7, eabh1289. doi:10.1126/sciadv.abh1289
Liao, J., Zhu, C., Gao, B., Zhao, Z., Liu, X., Tian, L., et al. (2019). Multiresponsive Elastic Colloidal Crystals for Reversible Structural Color Patterns. Adv. Funct. Mater. 29 (39), 1902954. doi:10.1002/adfm.201902954
Liu, B., Ni, H., Zhang, D., Wang, D., Fu, D., Chen, H., et al. (2017). Ultrasensitive Detection of Protein with Wide Linear Dynamic Range Based on Core-Shell SERS Nanotags and Photonic Crystal Beads. ACS Sens. 2 (7), 1035–1043. doi:10.1021/acssensors.7b00310
Liu, H., Wang, C., Wang, P., Liu, N., and Du, Q. (2019). A Two-step Strategy for Fabrication of Biocompatible 3D Magnetically Responsive Photonic Crystals. Front. Chem. 7, 26. doi:10.3389/fchem.2019.00026
Liu, J., Mao, Y., and Ge, J. (2012). The Magnetic Assembly of Polymer Colloids in a Ferrofluid and its Display Applications. Nanoscale 4 (5), 1598–1605. doi:10.1039/c2nr12024k
Liu, J., Xiao, M., Li, C., Li, H., Wu, Z., Zhu, Q., et al. (2019). Rugby-Ball-Like Photonic Crystal Supraparticles with Non-close-packed Structures and Multiple Magneto-Optical Responses. J. Mater. Chem. C 7 (47), 15042–15048. doi:10.1039/C9TC05438C
Liu, Y., Li, C., Zhang, H., Fan, X., Liu, Y., and Zhang, Q. (2015). One-Pot Hydrothermal Synthesis of Highly Monodisperse Water-Dispersible Hollow Magnetic Microspheres and Construction of Photonic Crystals. Chem. Eng. J. 259, 779–786. doi:10.1016/j.cej.2014.08.051
Luo, W., Ma, H., Mou, F., Zhu, M., Yan, J., and Guan, J. (2014). Steric-Repulsion-based Magnetically Responsive Photonic Crystals. Adv. Mater. 26 (7), 1058–1064. doi:10.1002/adma.201304134
Luo, W., Yan, J., Tan, Y., Ma, H., and Guan, J. (2017). Rotating 1-D Magnetic Photonic Crystal Balls with a Tunable Lattice Constant. Nanoscale 9 (27), 9548–9555. doi:10.1039/c7nr03335d
Ma, H., Tang, K., Luo, W., Ma, L., Cui, Q., Li, W., et al. (2017). Photonic Nanorods with Magnetic Responsiveness Regulated by Lattice Defects. Nanoscale 9 (9), 3105–3113. doi:10.1039/c6nr10022h
Shin, B. Y., Cha, B. G., Jeong, J. H., and Kim, J. (2017). Injectable Macroporous Ferrogel Microbeads with a High Structural Stability for Magnetically Actuated Drug Delivery. ACS Appl. Mater. Inter. 9 (37), 31372–31380. doi:10.1021/acsami.7b06444
Skjeltorp, A. T. (1983). One- and Two-Dimensional Crystallization of Magnetic Holes. Phys. Rev. Lett. 51 (25), 2306–2309. doi:10.1103/PhysRevLett.51.2306
Tang, S., Wang, C., Liu, N., Li, Y., Han, P., and Lu, Q. (2018). Instantaneous Magnetically Assembled and Hydrophilic Photonic Crystals with Controlled Diffraction Colors. J. Phys. Chem. C 122 (31), 18021–18028. doi:10.1021/acs.jpcc.8b05967
Wang, H., Sun, Y.-B., Chen, Q.-W., Yu, Y.-F., and Cheng, K. (2010). Synthesis of Carbon-Encapsulated Superparamagnetic Colloidal Nanoparticles with Magnetic-Responsive Photonic Crystal Property. Dalton Trans. 39 (40), 9565–9569. doi:10.1039/c0dt00621a
Wang, J., Le‐The, H., Shui, L., Bomer, J. G., Jin, M., Zhou, G., et al. (2020). Multilevel Spherical Photonic Crystals with Controllable Structures and Structure‐Enhanced Functionalities. Adv. Opt. Mater. 8 (10), 1902164. doi:10.1002/adom.201902164
Wang, J., Pinkse, P. W. H., Segerink, L. I., and Eijkel, J. C. T. (2021). Bottom-up Assembled Photonic Crystals for Structure-Enabled Label-free Sensing. ACS Nano 15 (6), 9299–9327. doi:10.1021/acsnano.1c02495
Wang, M., He, L., Xu, W., Wang, X., and Yin, Y. (2015). Magnetic Assembly and Field-Tuning of Ellipsoidal-Nanoparticle-Based Colloidal Photonic Crystals. Angew. Chem. Int. Ed. 54 (24), 7077–7081. doi:10.1002/anie.201501782
Wang, M., He, L., and Yin, Y. (2013). Magnetic Field Guided Colloidal Assembly. Mater. Today 16 (4), 110–116. doi:10.1016/j.mattod.2013.04.008
Wang, M., and Yin, Y. (2016). Magnetically Responsive Nanostructures with Tunable Optical Properties. J. Am. Chem. Soc. 138, 6315–6323. doi:10.1021/jacs.6b02346
Wang, W., Tang, B., Ju, B., and Zhang, S. (2015). Size-controlled Synthesis of Water-Dispersible Superparamagnetic Fe3O4 Nanoclusters and Their Magnetic Responsiveness. RSC Adv. 5 (92), 75292–75299. doi:10.1039/c5ra14354c
Wang, W., Xie, N., He, L., and Yin, Y. (2014). Photocatalytic Colour Switching of Redox Dyes for Ink-free Light-Printable Rewritable Paper. Nat. Commun. 5, 5459. doi:10.1038/ncomms6459
Wang, X.-Q., Hong, R., Wang, C.-F., Tan, P.-F., Ji, W.-Q., and Chen, S. (2017). Ultrafast Mechano-Responsive Photonic Hydrogel towards Multicolor Displays via the Pressure Sensation. Mater. Lett. 189, 321–324. doi:10.1016/j.matlet.2016.11.007
Wang, X. Q., Yang, S., Wang, C. F., Chen, L., and Chen, S. (2016). Multifunctional Hydrogels with Temperature, Ion, and Magnetocaloric Stimuli‐Responsive Performances. Macromol. Rapid Commun. 37 (9), 759–768. doi:10.1002/marc.201500748
Xiao, M., Liu, J., Chen, Z., Liu, W., Zhang, C., Yu, Y., et al. (2021). Magnetic Assembly and Manipulation of Janus Photonic Crystal Supraparticles from a Colloidal Mixture of Spheres and Ellipsoids. J. Mater. Chem. C 9, 11788–11793. doi:10.1039/D1TC01543E
Xu, X., Friedman, G., Humfeld, K. D., Majetich, S. A., and Asher, S. A. (2001). Superparamagnetic Photonic Crystals. Adv. Mater. 13 (22), 1681–1684. doi:10.1002/1521-4095(200111)13:22<1681::aid-adma1681>3.0.co;2-g
Xu, X., Majetich, S. A., and Asher, S. A. (2002). Mesoscopic Monodisperse Ferromagnetic Colloids Enable Magnetically Controlled Photonic Crystals. J. Am. Chem. Soc. 124, 13864–13868. doi:10.1021/ja026901k
Yablonovitch, E. (1987). Inhibited Spontaneous Emission in Solid-State Physics and Electronics. Phys. Rev. Lett. 58 (20), 2059–2062. doi:10.1103/PhysRevLett.58.2059
Yang, P., Li, H., Zhang, S., Chen, L., Zhou, H., Tang, R., et al. (2016). Gram-scale Synthesis of Superparamagnetic Fe3O4nanocrystal Clusters with Long-Term Charge Stability for Highly Stable Magnetically Responsive Photonic Crystals. Nanoscale 8 (45), 19036–19042. doi:10.1039/c6nr07155d
Yin, S.-N., Yang, S., Wang, C.-F., and Chen, S. (2016). Magnetic-Directed Assembly from Janus Building Blocks to Multiplex Molecular-Analogue Photonic Crystal Structures. J. Am. Chem. Soc. 138 (2), 566–573. doi:10.1021/jacs.5b10039
You, A., Cao, Y., and Cao, G. (2016). Colorimetric Sensing of Melamine Using Colloidal Magnetically Assembled Molecularly Imprinted Photonic Crystals. RSC Adv. 6 (87), 83663–83667. doi:10.1039/c6ra18617c
You, A., Cao, Y., and Cao, G. (2017). Facile and Convenient Fabrication of Color-Controlled Colloidal Magnetically Assembled Photonic Crystals. Chem. Res. Chin. Univ. 33 (4), 525–529. doi:10.1007/s40242-017-6310-z
You, A., Cao, Y., and Cao, G. (2015). Facile Fabrication of a Magnetically Assembled Colloidal Photonic Crystal Film via Radical Polymerization. RSC Adv. 5 (114), 93945–93950. doi:10.1039/c5ra13900g
Keywords: magnetically responsive photonic crystals, self-assembly, magnetic particles, magnetic nanoparticles, external magnetic field
Citation: Liu N, Deng L, Wang P, Tang S, Li P, Wang C, Li Y, Ayyanu R and Zheng F (2022) Progress on Rapidly and Self-Assembly Magnetically Responsive Photonic Crystals With High Tunability and Stability. Front. Mater. 9:843097. doi: 10.3389/fmats.2022.843097
Received: 24 December 2021; Accepted: 28 January 2022;
Published: 24 February 2022.
Edited by:
Xiao-Dong Chen, Sun Yat-sen University, ChinaReviewed by:
Zihui Meng, Beijing Institute of Technology, ChinaMun Ho Kim, Pukyong National University, South Korea
Copyright © 2022 Liu, Deng, Wang, Tang, Li, Wang, Li, Ayyanu and Zheng. This is an open-access article distributed under the terms of the Creative Commons Attribution License (CC BY). The use, distribution or reproduction in other forums is permitted, provided the original author(s) and the copyright owner(s) are credited and that the original publication in this journal is cited, in accordance with accepted academic practice. No use, distribution or reproduction is permitted which does not comply with these terms.
*Correspondence: Nan Liu, 13688869875@163.com; Fengjiao Zheng, fjzheng458@126.com