- 1Department of Bioinformatics, School of Medical Informatics and Engineering, Xuzhou Medical University, Xuzhou, China
- 2Hefei National Laboratory for Physical Sciences at Microscale and Department of Physics, University of Science and Technology of China, Hefei, Anhui, China
- 3Hefei Innovation Research Institute, School of Microelectronics, Beihang University, Hefei, China
- 4Key Laboratory for Micro-Nano Physics and Technology of Hunan Province, College of Materials Science and Engineering, Hunan University, Changsha, Hunan, China
- 5Department of Physics and Astronomy and Graphene Research Institute, Sejong University, Seoul, South Korea
- 6Laboratory Medicine, Guangdong Provincial People’s Hospital, Guangdong Academy of Medical Sciences, Guangzhou, China
The controllable assembly of plasmonic nanoparticles has developed as one of the most significant approaches for surface enhanced Raman spectroscopy (SERS) applications. This study developed a simple approach to improve a large-scale ordered assembly of gold nanorods (GNRs) by controlling the droplet evaporation mode on hydrophobic substrates. The hydrophobic substrate was efficiently produced by spin coating the silicone oil onto the glass slides and annealing them. The analyte molecule rhodamine (R6G) was employed as a surface-enhanced Raman scattering probe to demonstrate the potential effects of the synthesized arrays. This hydrophobic platform enables the concentration and delivery of analyte molecules into the surface enhanced Raman spectroscopy sensitive site while suppressing the coffee ring effect generated by the smooth contraction motion of the base contact radius of the droplet without any pinning. Thus, the limit of detection (LOD) of the R6G analyte was lowered to 10−10 M and the homogenous dispersion of surface enhanced Raman spectroscopy hotspots within the self-assembly reproducible surface enhanced Raman spectroscopy signal. This new method enables a broad range of packing patterns and mechanisms by changing the host nanoparticles in the dispersion.
1 Introduction
The self-assembly of nanoparticles is becoming a common and effective approach for fabricating controlled colloidal patterns (Jana, 2004; Ko et al., 2008; Prasad et al., 2008; Grzelczak et al., 2010; Dai et al., 2011). Several methods for fabricating GNRs arrays have been developed to compact complicated particle structures with diverse symmetry and assembly configurations at the nanoscale (Zhu et al., 2011; Quan et al., 2014; Li et al., 2015; Wang et al., 2015; Lu and Tang, 2016; Luo et al., 2017a; Luo et al., 2017b). The assembly of anisotropic colloidal nanoparticles into complicated well-arranged structures has long been a hot topic in nanoscience due to their distinct collective optical and electronic properties and incorporation into nanophotonics (Zhu et al., 2011; Lu and Tang, 2016; Luo et al., 2017a; Quan et al., 2014; Li et al., 2015; Wang et al., 2015; Xia et al., 2003; Cho et al., 2010; Wang et al., 2012; Sun, 2013; Wang et al., 2015; Xu et al., 2015; Neretina et al., 2016; Abeyweera et al., 2017; Kim et al., 2017). The successful incorporation of such nanoparticles into efficient optoelectronic devices needs reproducible, highly organized, and macroscopic uniformity of performance from the structure (Zhu et al., 2011; Li et al., 2016; Lu and Tang, 2016).
The droplet evaporation has been intensively examined among the diverse approaches used to produce complicated structures due along with its functionality, cost-effectiveness, and broad applicability to numerous nanomaterials (Zhang et al., 2014; Vogel et al., 2015). Moreover, the drying droplet commonly leaves a non-uniform solid-ring on the substrate known as a coffee ring (Deegan et al., 1997). The configuration of a solid ring structure is strongly related to droplet-controlled dispersion, which is primarily focused on heat and mass loss during evaporation (Deegan, 2000). The heat loss causes evaporative cooling to sufficiently produce a surface tension slope capable of driving Marangoni flow (Larson, 2014). The evaporation process causes capillary flow outward from the center of the drop, bringing the suspension particles to the edges. The particles are significantly concentrated along the initial drop edge after evaporation, resulting in a non-uniform solid ring (Deegan et al., 2000). Recent research has focused on addressing the coffee-ring effect by controlling important configurations including particle size, particle shape, charges and surfactant concentration, to attain large-area arrays of self-assembled particles (Fan et al., 2004; Bigioni et al., 2006; Byun et al., 2010; Alvarez-Puebla et al., 2011; Singh et al., 2012; Chen et al., 2013; Peng et al., 2013; Ye et al., 2013; Peng et al., 2014; Zhang and Lin, 2014; Apte et al., 2015; Hamon et al., 2015; Li et al., 2016; Lu and Tang, 2016; Luo et al., 2017a; Luo et al., 2017b; Kim et al., 2017). The most effective method for overcoming the “diffusion limit” of analytes in highly diluted aqueous solutions is to use super hydrophobic surfaces, which have the dynamic ability to deliver analytes to SERS-active sites (De Angelis et al., 2011; Gentile et al., 2014). Moreover, the disadvantage of super hydrophobic surfaces for these applications is that air pockets among the surface structures can be spoiled by outward wetting pressure and could lose wetting property by destructive the surface structure (Reyssat et al., 2007). Recently, slippery surfaces (hydrophobic surfaces) have evolved into a superior substitute to super hydrophobic as a fluid repellent surface with a very small contact angle hysteresis (Bocquet and Lauga, 2011). To our understanding, only few studies has been accompanied on the controlled evaporation method-based SERS technique for analyte detection on cost-effective hydrophilic substrates.
This study proposed a novel method for fabricating self-assembled GNRs. The hydrophobic surface was fabricated in the first step and then controlled the evaporation rate of droplets in the second step. This method was used to reverse the coffee ring effect during droplet evaporation of an aqueous diffusion of GNRs assembly. The droplet evaporation process revealed GNRs formed at the interface between solvent and air, developing domains that improved in size and density as the solvent evaporated under microscope light. The optical properties of the attained superstructures were experimentally determined. Finally, the promising application of GNRs self-assembly arrays for analytical detection using R6G as a model analyte based on SERS was established.
2 Material and method
2.1 Preparation of GNRs
The study synthesized the GNRs and employed a seed-mediated growth method as described in our previous procedure (Usman et al., 2019). After the seed solution was formed, HAuCl4 (0.1 ml; 0.025 M) and CTAB (5 ml; 0.2 M) were combined at room temperature. The solution of ice-cold NaBH4 (0.01 M, 0.6 ml) was promptly introduced. The color of the solution gradually changed from yellow to brownish-yellow. The solution continues to stirring for 20 min. The solution was cooled down to room temperature for 30 min. The growth solution was prepared by adding hexadecyltrimethyl ammonium bromide (CTAB), 5 ml, 0.2 M, and different amounts of 0.05, 0.10, 0.15, 0.20, and 0.25 ml of 0.16 mM AgNO3 solutions at 30°C, respectively. Then, add 0.2 ml of 0.025 M HAuCl4 to the solution. Following constant stirring, the ascorbic acid (0.08 M, 70 ml) was added and changed the color from dark-yellow to colorless. Lastly, the seed solution of 12 ml was mixed with the growth solution at 30°C. The color of the mixture slowly changes in 15–30 min. The GNRs were well designed at room temperature after rapid growth.
2.2 Silicone oil-based hydrophobic surface preparation
The glass slides were cleaned for 10 min using sonication and plasma cleaning. The silicone oil, with a kinematic viscosity of 370 cSt, was applied to a clean glass slide and spin-coated at 1,000 rpm for 1 min. This method was effective for removing additional lubricating oil. The silicone oil-coated glass slide was then annealed at 150°C for 120 min. As a result, the surface chemical property was modified to be hydrophobic and a uniform silicone oil coating was formed on the glass slide.
2.3 Evaporation-induced assembly of aligned GNRs arrays
The GNRs were centrifuged at 7,000 rpm for 20 min to reduce extra CTAB. A 30-μl drop of GNRs was deposited on the hydrophobic substrate. Then the hydrophobic substrate was transferred onto a polystyrene Petri dish plate with dimensions of 94 mm in width and 15 mm in height. After that, add 1 ml of water to the Petri dish to regulate the humidity. The Petri dish was wrapped and put inside an incubator. After the evaporation, the GNRs solution on the hydrophobic substrate contracted into tiny droplets without forming coffee-rings. As a result, the well-arranged assemblies of GNRs were observed on the hydrophobic substrate.
2.4 Raman spectroscopy measurements
For the SERS measurements, R6G dye was working as a Raman probe molecule and was diluted to various concentrations. The concentrated molecules and GNRs solution of 30 μl was placed on the SERS substrate and evaporated at room temperature. All SERS measurements were carried out using a 633 nm laser excitation and a laser power of about 1.5 mW. A confocal Raman microscope (Renishaw PLC., England, United Kingdom) with a 50-microscope objective and a numerical aperture (NA) of 0.6 was used to detect in-elastically scattered radiation.
2.5 FEM simulation
We used the commercial program COMSOL Multiphysics 5.5 for numerical simulation to do electromagnetic analysis. Comsol uses the finite element method (FEM) to solve the following differential form of the Maxwell equation:
where
3 Results and discussion
The hydrophobic surface and high humidity environment are critical for GNRs self-assembly and enrichment. A lubricated substrate containing a silicon oil-coated glass slide is a simple depiction followed by spin coating and annealing. Hence, this would make the substrate hydrophobic because the high surface energy of silicon dioxide changes the surface energy of the glass through covalent bonding of silicone oil molecules with the glass surface. The hydrophobic surface played an important part in the development and self-assembly of GNRs. It is extremely important to keep droplet evaporation in a steady-state assembly process, which is primarily a thermodynamic procedure, in imperative to produce homogeneous self-assembled particle arrays. As a result, the environmental parameters must be carefully monitored, including the temperature and humidity of the substrates during the evaporation process. When the temperature falls below 25°C, the CTAB surfactant in the droplets crystallizes and precipitates from the solution. This clearly devastates the self-assembly process, resulting in poor quality GNRs arrays. When the temperature rises to 50°C, however, the convection in the droplet intensifies, causing serious damage in large non-uniform accumulations (Ming et al., 2008). The evaporation was carried out in a controlled environment with a high humidity level in maintenance. The high humidity environment slowed the evaporation of the GNRs droplets, allowing the solvent to evaporate at the same rate from the contact line to the droplet centers (Zhang and Lin, 2014). The slow evaporation could reduce the convection and inhibit GNRs from following from the center to the contact line, thus preventing the formation of a coffee-ring structure (Hu and Larson, 2006). The solution contracted into smaller droplets owing to the homogeneous evaporation speed and the absence of a pinned edge across the surface of the droplets. Figure 1A depicts a schematic demonstration of the fabrication method of the hydrophobic platform.
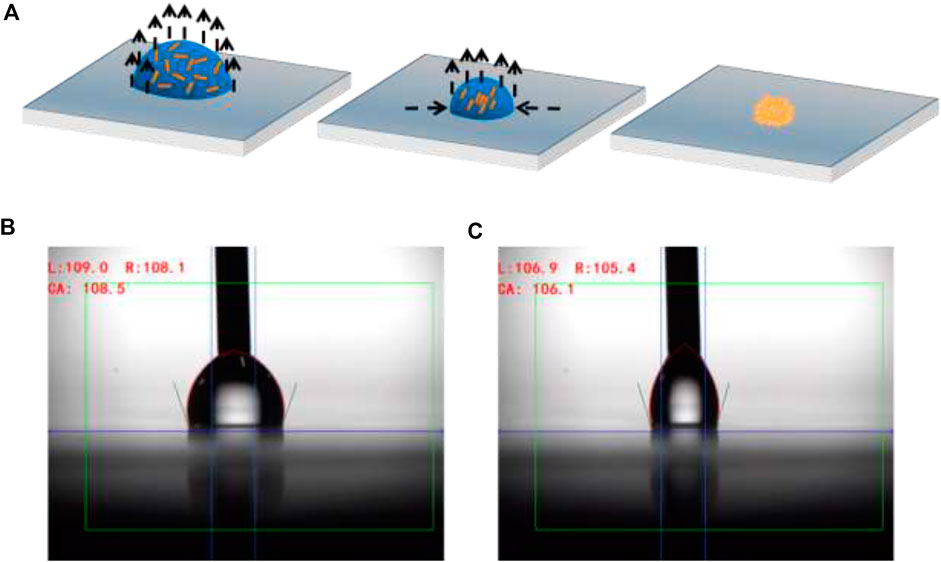
FIGURE 1. (A) A diagram showing the enrichment and self-assembly of GNRs on a hydrophobic surface. (B) Contact angles advancing and (C) receding on a hydrophobic surface.
After optimizing the conditions, we investigated the effects of substrate hydrophobicity and repellency by contact angle (
This study used an evaporation-induced self-assembly approach to obtain GNRs from a liquid drop containing GNRs. The UV-vis absorption spectrum of GNRs dispersed in water is shown in Figure 2A. The transverse and longitudinal modes were assigned to the surface plasmon resonance peaks that were obtained at wavelengths of 510 and 670 nm, respectively. The previous research has shown that the maximum SERS intensity was achieved when the laser excitation wavelength was slightly shorter than LSPR, so the laser excitation wavelength was in resonance with the substrate of the surface plasmon (Hossain et al., 2009; Kumar et al., 2020). Figure 2B depicts a transmission electron microscopy (TEM) representation of GNRs. The distributions of GNRs particle sizes measured in TEM images were plotted in (Figures 2C, D). The length of 41.5 + 3.9 nm and the width of 14.1 + 1.5 nm of the GNRs were obtained from the histogram diagram, and an aspect ratio of about 3. According to this, GNRs had uniform morphology and a narrow size distribution.
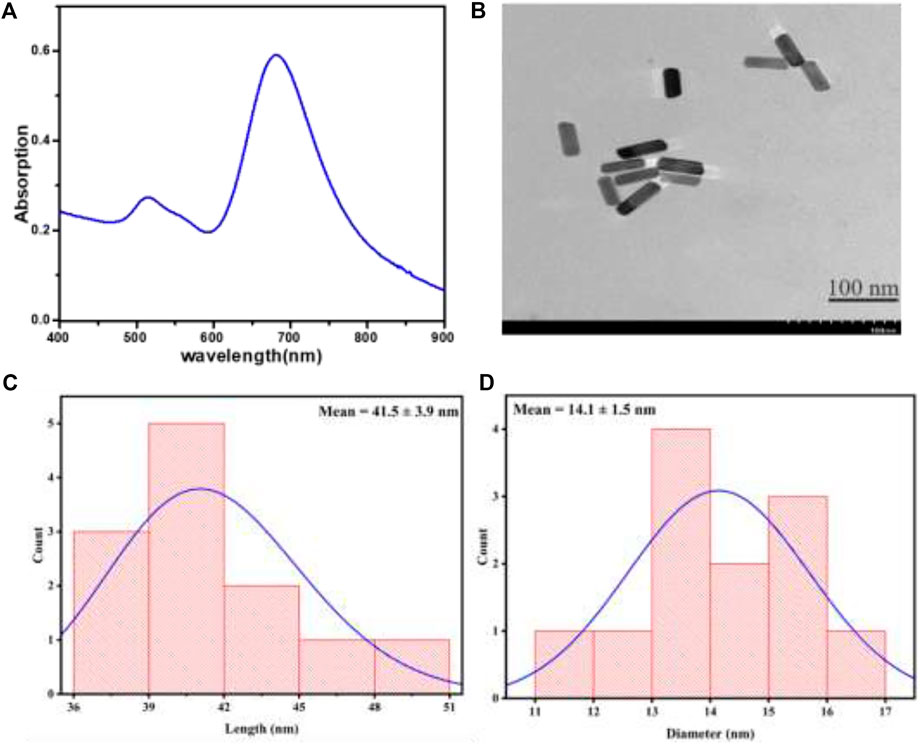
FIGURE 2. (A) The UV-vis spectrum of GNRs in aqueous medium (B) TEM image of GNRs demonstrating good size uniformity. The histogram figures show the (C) length and (D) diameter of GNRs from TEM images.
We investigated the effects of hydrophobicity on the development of GNRs arrays after optimizing the evaporation conditions, such as temperature at 30°C and humidity at 96%. The GNRs evaporated on the hydrophobic surface in a controlled environment, producing a highly concentrated pattern and repellency. The 20 μl GNRs were measured using an optical microscope at various times on hydrophilic and hydrophobic substrates (see Figure 3).
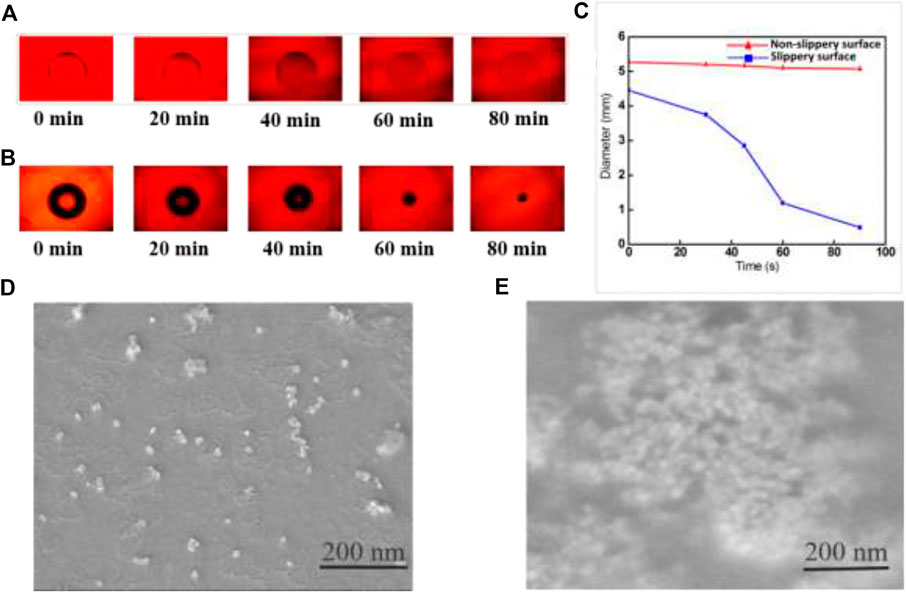
FIGURE 3. (A) Optical microscopy images of droplets evaporating at different times on a hydrophilic (B) hydrophobic surface. (C) The contact diameter (CD) of a droplet on non-slippery surfaces and slippery surfaces as a function of evaporation time. SEM images of GNRs solution after evaporation (D) on the hydrophilic surface and (E) lubricated liquid-infused hydrophobic surface.
The droplet of the initial contact diameter (d) on the non-slippery surface (hydrophilic surface) was large (
The scanning electron microscopy (SEM) images suggest an exciting effect of the enrichment and the concentration of GNRs particles on hydrophilic and hydrophobic surfaces after evaporation. In Figure 3D, the GNRs particles showed a scattered pattern after complete evaporation on a hydrophilic surface, with the maximum value at the droplet edge, as described by the “coffee ring effect.” This effect is commonly observed at pinning sites on surfaces. As a result, GNRs particles show an extensive configuration over a large area. However, the GNRs solution produced interesting results on the hydrophobic surface. The easy contraction of the droplet without pinning suppressed the coffee ring effect to enrich all the GNRs particles into a very small spot on the hydrophobic surface. The effective compact self-assembly of GNRs via droplet evaporation on the hydrophobic surface is shown in Figure 3E.
Furthermore, the condition for a high contact angle and low CAH is critical for improving SERS performance. In former studies has demonstrated that densely compacted metallic nanoparticles have significant SERS enhancement factors (Diebold et al., 2009; Huang et al., 2015). The formation of the hot spots between neighboring particles significantly increases SERS enhancement.
In this study, the fabricated substrate was covered by a closely packed GNRs array, which produced a significant field enhancement effect. The schematically Figure 4A illustrates the encapsulated GNRs on a hydrophobic surface. Here a rounded ends cylindrical GNRs (with orange color) coated with dye (blue color), shined by TE polarized light. The electric field is parallel to the plane of the hydrophobic surface with normal K-propagation vector. The densely-packed GNRs arrays and their uniform structure were able to produce a SERS signal for molecular detection at concentrations as low as 10−10 M. SERS measurements on the GNRs arrays were carried out using a Raman spectrometer with a 633 nm excitation. The target molecule was R6G solution, a common dye used in biological research. Figure 4B shows the SERS spectra of R6G at various concentrations ranging from 10−6 to 10−10 M. The Raman spectra of R6G were attributed to C-C-C ring in plane, out-of-plane being, and C-H in-plane bending vibrations in the sharp peaks at 611 and 776 cm−1, respectively. It also had Raman shift peaks at 1,180, 1,360, 1,509, and 1,650 cm−1, which were ascribed to symmetric modes of C-C stretching vibrations in plane. These R6G Raman peaks are consistent with those previously reported (Lu et al., 2005; Jensen and Schatz, 2006). The Raman spectra clearly showed the characteristic Raman peaks of R6G even when the concentration was as low as 10−10 M. The SERS substrates for the GNRs arrays had excellent reusability, enabling multiple detections after plasma etching to clean the previous substrate. The Raman signal decreased gradually with the decreasing the concentration of R6G. The previous results were also confirmed in the study of Wei et al. (2018) using R6G detection at a concentration was as low as 10−10 M. We obtained the calibration curves with this result analyzed as shown in Figure 4C. Notably, it had a relatively good linear correlation relationship (correlation coefficient, R2 = 0.9928) between the SERS signal ratio and R6G concentration. The suggested approach might, therefore, prove helpful in the quantitative analysis.
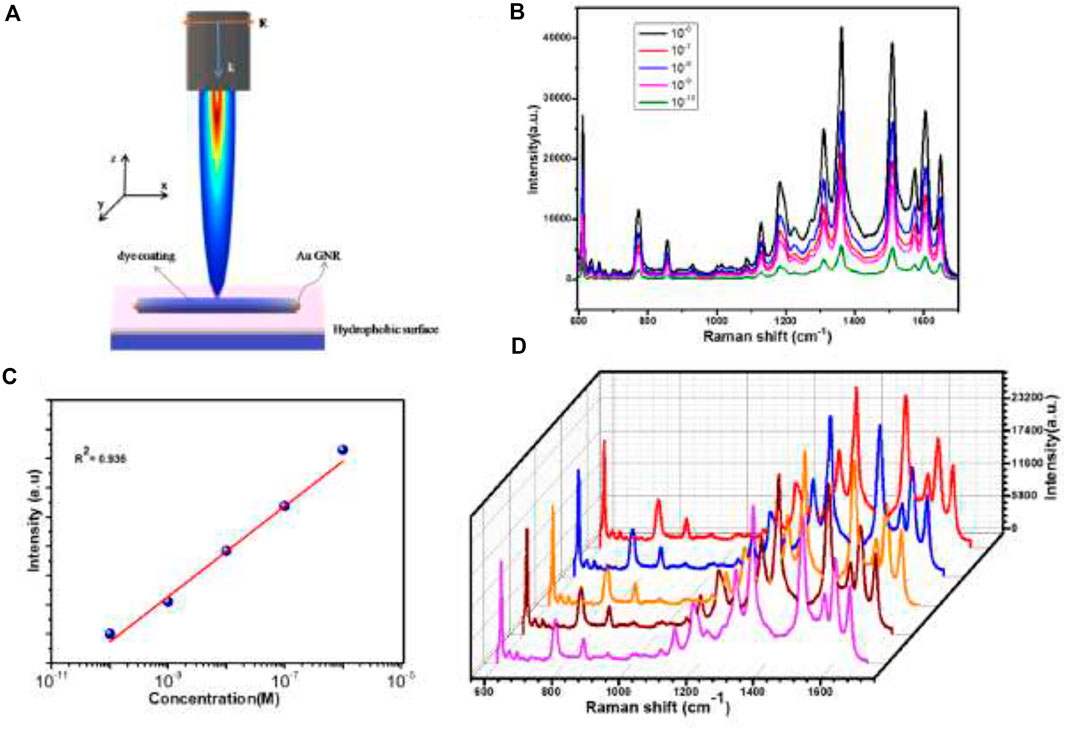
FIGURE 4. (A) Schematic diagram of GNRs coupling with dye analyte on a hydrophobic surface as a strategy to increase the electric field enhancement effect (B) Raman scattering spectra of various concentrations of solutions. (C) The peak intensities of various concentrations at 1,340 cm−1 (D) SERS spectra from R6G aqueous solution (10−6 M) dried on randomly chosen five spots of the GNRs arrays demonstrate the good reproducibility of the substrate.
In order to study the uniformity and ensure a homogeneous distribution, 10−6 M of the analyte R6G aqueous solution was dispersed on the surface of the hydrophobic surface. After that, we observed five random spots on the substrate with the same exciting line and integration time (Figure 4D). The relative signal deviation of R6G was about 10% on the GNRs arrays, revealing the overall reliability and uniformity of such a substrate for SERS detection. The excellent performance of the GNRs arrays could be attributed to a number of factors. For example, the GNRs were arranged in a regular pattern. In periodic structures, photon density redistribution was common, causing an increase in the density of optical modes and an improvement in the Raman scattering of the detected molecules (Gaponenko, 2002).
To effectively understand the physical mechanism underlying the detected SERS response, the electric field intensity enhancement of the GNRs array was investigated employing Comsol multiphysics simulation (see Figure 5), on hydrophobic and hydrophilic substrates, under 540 and 670 nm laser light. The GNRs arrays are assembled with a gap of 7 nm. The GNRs revealed the enhancement of electromagnetic fields in the case of the hydrophobic surface. Figure 5A indicates that at 540 nm of laser excitation, the field is a maximally localized electromagnetic field within the GNRs array in the hydrophobic substrate compared to the hydrophilic substrate in Figure 5B. Similarly, Figure 5C exhibits a similar excellent local field enhancement effect on a hydrophobic substrate as compared to the hydrophilic substrate using 670 nm laser excitation. Hence, when compared with the disordered substrate, the resonance of the local electromagnetic field around the GNRs arrays is relatively strong, and the dense “hot spots” can improve the SERS activity of the substrate. These results further confirm and demonstrate that the hydrophobic substrate can be used to enhance and detect the SERS signals, which is almost consistent with our experimental results.
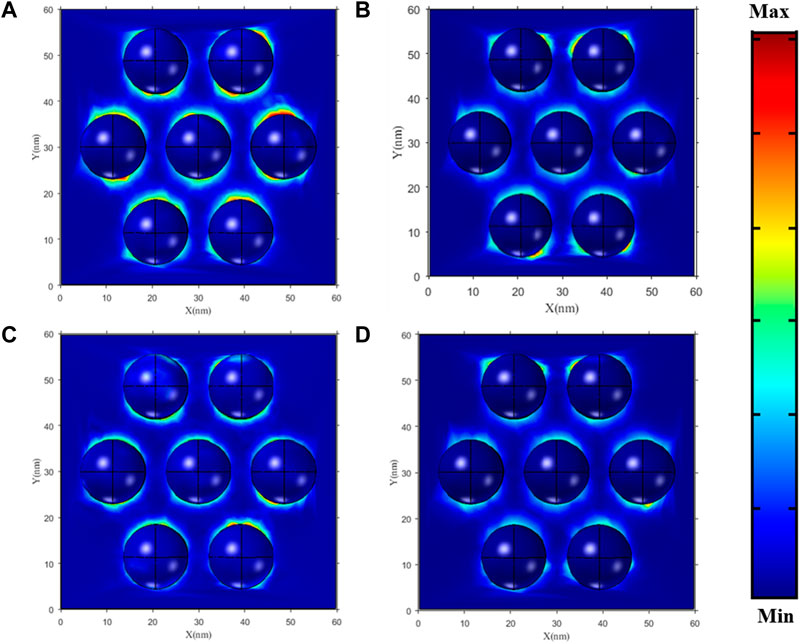
FIGURE 5. The enhancement of the electromagnetic field in GNRs with hydrophobic and hydrophilic substrates with 7-nm gaps (A,C) and (B,D), respectively.
4 Conclusion
In summary, we used the controlled evaporation process to fabricate large-area ordered self-assembly arrays of GNRs on the hydrophobic surface. The droplet contracts effectively because of the lack of pinning sites and hydrophobicity of the substrate, which prevent the coffee-ring effect and offer a smooth surface. Therefore, this process effectively delivers the molecules inside the droplet to a concentrated hot spot. The resulting arrays had constant structural and efficient homogeneity on a macroscopic scale. We also established that the fabricated GNRs arrays produced a SERS signal for detecting R6G at a concentration of 10−10 M. The exceptional results in SERS detection reproducibility are due to the uniformity in large areas. The structure of the GNRs relied solely on the hydrophobicity of substrates in an evaporating environment. The limitation of hydrophobic surfaces is also observed when there are many liquid drops sliding on them, which slowly removes the lubricating oil. In this case, the hydrophobic performance is degraded, but it can be rapidly recovered by coating the silicone oil on the surface again. As a result, this technology could be easily applied to different nanoparticle systems to produce ordered nanoscale arrays for the integration of microscopic devices.
Data availability statement
The original contributions presented in the study are included in the article/Supplementary Material, further inquiries can be directed to the corresponding authors.
Author contributions
MU conceived the core ideas and designed the outlines of the manuscript. MI and ZM contributed to the project administration. WA carried out the theoretical simulation analysis of literature. All authors made significant contributions to write and revise the manuscript. XZ and LW set-up the platforms, provided resources, and secured funding for the study. All authors read and approved the submitted version of the manuscript.
Funding
This work was supported in part by National Natural Science Foundation of China (31900022, 32171281), Young Science and Technology Innovation Team of Xuzhou Medical University (TD202001), and Jiangsu Qinglan Project (2020).
Acknowledgments
We acknowledge the assistance from the scanning electron microscopy analysis.
Conflict of interest
The authors declare that the research was conducted in the absence of any commercial or financial relationships that could be construed as a potential conflict of interest.
Publisher’s note
All claims expressed in this article are solely those of the authors and do not necessarily represent those of their affiliated organizations, or those of the publisher, the editors and the reviewers. Any product that may be evaluated in this article, or claim that may be made by its manufacturer, is not guaranteed or endorsed by the publisher.
References
Abeyweera, S. C., Rasamani, K. D., and Sun, Y. (2017). Ternary silver halide nanocrystals. Acc. Chem. Res. 50, 1754–1761. doi:10.1021/acs.accounts.7b00194
Alvarez-Puebla, R. A., Agarwal, A., Manna, P., Khanal, B. P., Aldeanueva-Potel, P., Carbó-Argibay, E., et al. (2011). Gold nanorods 3D-supercrystals as surface enhanced Raman scattering spectroscopy substrates for the rapid detection of scrambled prions. Proc. Natl. Acad. Sci. 108, 8157–8161. doi:10.1073/pnas.1016530108
Apte, A., Bhaskar, P., Das, R., Chaturvedi, S., Poddar, P., and Kulkarni, S. (2015). Self-assembled vertically aligned gold nanorod superlattices for ultra-high sensitive detection of molecules. Nano Res. 8, 907–919. doi:10.1007/s12274-014-0572-2
Bigioni, T. P., Lin, X. M., Nguyen, T. T., Corwin, E. I., Witten, T. A., and Jaeger, H. M. (2006). Kinetically driven self-assembly of highly ordered nanoparticle monolayers. Nat. Mat. 5, 265–270. doi:10.1038/nmat1611
Byun, M., Bowden, N. B., and Lin, Z. (2010). Hierarchically organized structures engineered from controlled evaporative self-assembly. Nano Lett. 10, 3111–3117. doi:10.1021/nl1018035
Chen, H., Shao, L., Li, Q., and Wang, J. (2013). Gold nanorods and their plasmonic properties. Chem. Soc. Rev. 42, 2679–2724. doi:10.1039/c2cs35367a
Cho, E. C., Camargo, P. H., and Xia, Y. (2010). Synthesis and characterization of noble-metal nanostructures containing gold nanorods in the center. Adv. Mat. 22, 744–748. doi:10.1002/adma.200903097
Dai, Q., Rettner, C. T., Davis, B., Cheng, J., and Nelson, A. (2011). Topographically directed self-assembly of gold nanoparticles. J. Mat. Chem. 42, 16863–16865. doi:10.1039/c1jm11683e
De Angelis, F., Gentile, F., Mecarini, F., Das, G., Moretti, M., Candeloro, P., et al. (2011). Breaking the diffusion limit with super-hydrophobic delivery of molecules to plasmonic nanofocusing SERS structures. Nat. Photonics 5, 682–687. doi:10.1038/nphoton.2011.222
Deegan, R. D., Bakajin, O., Dupont, T. F., Huber, G., Nagel, S. R., and Witten, T. A. (1997). Capillary flow as the cause of ring stains from dried liquid drops. Nature 389, 827–829. doi:10.1038/39827
Deegan, R. D., Bakajin, O., Dupont, T. F., Huber, G., Nagel, S. R., and Witten, T. A. (2000). Contact line deposits in an evaporating drop. Phys. Rev. E 62, 756–765. doi:10.1103/physreve.62.756
Deegan, R. D. (2000). Pattern formation in drying drops. Phys. Rev. E 61, 475–485. doi:10.1103/physreve.61.475
Diebold, E. D., Mack, N. H., Doorn, S. K., and Mazur, E. (2009). Femtosecond laser-nanostructured substrates for surface-enhanced Raman scattering. Langmuir 25, 1790–1794. doi:10.1021/la803357q
Fan, H., Yang, K., Boye, D. M., Sigmon, T., Malloy, K. J., Xu, H., et al. (2004). Self-assembly of ordered, robust, three-dimensional gold nanocrystal/silica arrays. Science 304, 567–571. doi:10.1126/science.1095140
Gaponenko, S. V. (2002). Effects of photon density of states on Raman scattering in mesoscopic structures. Phys. Rev. B 65, 140303. doi:10.1103/physrevb.65.140303
Gentile, F., Coluccio, M. L., Zaccaria, R. P., Francardi, M., Cojoc, G., Perozziello, G., et al. (2014). Selective on site separation and detection of molecules in diluted solutions with super-hydrophobic clusters of plasmonic nanoparticles. Nanoscale 6, 8208–8225. doi:10.1039/c4nr00796d
Grzelczak, M., Vermant, J., Furst, E. M., and Liz-Marzán, L. M. (2010). Directed self--assembly of nanoparticles. ACS Nano 4, 3591–3605. doi:10.1021/nn100869j
Hamon, C., Novikov, S. M., Scarabelli, L., Solís, D. M., Altantzis, T., Bals, S., et al. (2015). Collective plasmonic properties in few-layer gold nanorod supercrystals. ACS Photonics 2, 1482–1488. doi:10.1021/acsphotonics.5b00369
Hossain, M. K., Kitahama, Y., Huang, G. G., Han, X., and Ozaki, Y. (2009). Surface-enhanced Raman scattering: Realization of localized surface plasmon resonance using unique substrates and methods. Anal. Bioanal. Chem. 394, 1747–1760. doi:10.1007/s00216-009-2762-4
Hu, H., and Larson, R. G. (2006). Marangoni effect reverses coffee-ring depositions. J. Phys. Chem. B 110, 7090–7094. doi:10.1021/jp0609232
Huang, L., Mühlenbernd, H., Li, X., Song, X., Bai, B., Wang, Y., et al. (2015). Broadband hybrid holographic multiplexing with geometric metasurfaces. Adv. Mat. 27, 6444–6449. doi:10.1002/adma.201502541
Jana, N. R. (2004). Shape effect in nanoparticle self-assembly. Angew. Chem. Int. Ed. 43, 1536–1540. doi:10.1002/anie.200352260
Jensen, L., and Schatz, G. C. (2006). Resonance Raman scattering of rhodamine 6G as calculated using time-dependent density functional theory. J. Phys. Chem. A 110, 5973–5977. doi:10.1021/jp0610867
Kim, J., Song, X., Ji, F., Luo, B., Ice, N. F., Liu, Q., et al. (2017). Polymorphic assembly from beveled gold triangular nanoprisms. Nano Lett. 17, 3270–3275. doi:10.1021/acs.nanolett.7b00958
Ko, H., Singamaneni, S., and Tsukruk, V. V. (2008). Nanostructured surfaces and assemblies as SERS media. Small 4, 1576–1599. doi:10.1002/smll.200800337
Kumar, S., Tokunaga, K., Namura, K., Fukuoka, T., and Suzuki, M. (2020). Experimental evidence of a twofold electromagnetic enhancement mechanism of surface-enhanced Raman scattering. J. Phys. Chem. C 124, 21215–21222. doi:10.1021/acs.jpcc.0c07930
Larson, R. G. (2014). Transport and deposition patterns in drying sessile droplets. AIChE J. 60, 1538–1571. doi:10.1002/aic.14338
Li, P., Li, Y., Zhou, Z. K., Tang, S., Yu, X. F., Xiao, S., et al. (2016). Evaporative self-assembly of gold nanorods into macroscopic 3D plasmonic superlattice arrays. Adv. Mat. 28, 2511–2517. doi:10.1002/adma.201505617
Li, R., Bian, K., Wang, Y., Xu, H., Hollingsworth, J. A., Hanrath, T., et al. (2015). An obtuse rhombohedral superlattice assembled by Pt nanocubes. Nano Lett. 15, 6254–6260. doi:10.1021/acs.nanolett.5b02879
Lu, C., and Tang, Z. (2016). Advanced inorganic nanoarchitectures from oriented self-assembly. Adv. Mat. 28, 1096–1108. doi:10.1002/adma.201502869
Lu, Y., Liu, G. L., and Lee, L. P. (2005). High-density silver nanoparticle film with temperature-controllable interparticle spacing for a tunable surface enhanced Raman scattering substrate. Nano Lett. 5, 5–9. doi:10.1021/nl048965u
Luo, B., Smith, J. W., Ou, Z., and Chen, Q. (2017). Quantifying the self-assembly behavior of anisotropic nanoparticles using liquid-phase transmission electron microscopy. Acc. Chem. Res. 50, 1125–1133. doi:10.1021/acs.accounts.7b00048
Luo, B., Smith, J. W., Wu, Z., Kim, J., Ou, Z., and Chen, Q. (2017). Polymerization-like co-assembly of silver nanoplates and patchy spheres. ACS Nano 11, 7626–7633. doi:10.1021/acsnano.7b02059
Ming, T., Kou, X., Chen, H., Wang, T., Tam, H. L., Cheah, K. W., et al. (2008). Ordered gold nanostructure assemblies formed by droplet evaporation. Angew. Chem. Int. Ed. Engl. 47, 9831–9836. doi:10.1002/ange.200803642
Neretina, S., Hughes, R. A., Gilroy, K. D., and Hajfathalian, M. (2016). Noble metal nanostructure synthesis at the liquid–substrate interface: New structures, new insights, and new possibilities. Acc. Chem. Res. 49, 2243–2250. doi:10.1021/acs.accounts.6b00393
Peng, B., Li, G., Li, D., Dodson, S., Zhang, Q., Zhang, J., et al. (2013). Vertically aligned gold nanorod monolayer on arbitrary substrates: Self-assembly and femtomolar detection of food contaminants. ACS Nano 7, 5993–6000. doi:10.1021/nn401685p
Peng, B., Li, Z., Mutlugun, E., Martínez, P. L. H., Li, D., Zhang, Q., et al. (2014). Quantum dots on vertically aligned gold nanorod monolayer: Plasmon enhanced fluorescence. Nanoscale 6, 5592–5598. doi:10.1039/c3nr06341k
Prasad, B. L. V., Sorensen, C. M., and Klabunde, K. J. (2008). Gold nanoparticle superlattices. Chem. Soc. Rev. 37, 1871–1883. doi:10.1039/b712175j
Quan, Z., Xu, H., Wang, C., Wen, X., Wang, Y., Zhu, J., et al. (2014). Solvent-mediated self-assembly of nanocube superlattices. J. Am. Chem. Soc. 136, 1352–1359. doi:10.1021/ja408250q
Reyssat, M., Yeomans, J. M., and Quéré, D. (2007). Impalement of fakir drops. Europhys. Lett. 81, 26006. doi:10.1209/0295-5075/81/26006
Singh, A., Gunning, R. D., Ahmed, S., Barrett, C. A., English, N. J., Garate, J. A., et al. (2012). Controlled semiconductor nanorod assembly from solution: Influence of concentration, charge and solvent nature. J. Mat. Chem. 22, 1562–1569. doi:10.1039/c1jm14382d
Sun, Y. (2013). Controlled synthesis of colloidal silver nanoparticles in organic solutions: Empirical rules for nucleation engineering. Chem. Soc. Rev. 42, 2497–2511. doi:10.1039/c2cs35289c
Usman, M., Guo, X., Wu, Q., Barman, J., Su, S., Huang, B., et al. (2019). Facile silicone oil-coated hydrophobic surface for surface enhanced Raman spectroscopy of antibiotics. RSC Adv. 9, 14109–14115. doi:10.1039/c9ra00817a
Vogel, N., Retsch, M., Fustin, C. A., Del Campo, A., and Jonas, U. (2015). Advances in colloidal assembly: The design of structure and hierarchy in two and three dimensions. Chem. Rev. 115, 6265–6311. doi:10.1021/cr400081d
Wang, C., Siu, C., Zhang, J., and Fang, J. (2015). Understanding the forces acting in self-assembly and the implications for constructing three-dimensional (3D) supercrystals. Nano Res. 8, 2445–2466. doi:10.1007/s12274-015-0767-1
Wang, T., Zhuang, J., Lynch, J., Chen, O., Wang, Z., Wang, X., et al. (2012). Self-assembled colloidal superparticles from nanorods. Science 338, 358–363. doi:10.1126/science.1224221
Wei, W., Wang, Y., Ji, J., Zuo, S., Li, W., Bai, F., et al. (2018). Fabrication of large-area arrays of vertically aligned gold nanorods. Nano Lett. 18, 4467–4472. doi:10.1021/acs.nanolett.8b01584
Xia, Y., Yang, P., Sun, Y., Wu, Y., Mayers, B., Gates, B., et al. (2003). One-dimensional nanostructures: Synthesis, characterization, and applications. Adv. Mat. 15, 353–389. doi:10.1002/adma.200390087
Xu, H., Xu, Y., Pang, X., He, Y., Jung, J., Xia, H., et al. (2015). A general route to nanocrystal kebabs periodically assembled on stretched flexible polymer shish. Sci. Adv. 1, 1500025. doi:10.1126/sciadv.1500025
Ye, X., Millan, J. A., Engel, M., Chen, J., Diroll, B. T., Glotzer, S. C., et al. (2013). Shape alloys of nanorods and nanospheres from self-assembly. Nano Lett. 13, 4980–4988. doi:10.1021/nl403149u
Zhang, S. Y., Regulacio, M. D., and Han, M. Y. (2014). Self-assembly of colloidal one-dimensional nanocrystals. Chem. Soc. Rev. 43, 2301–2323. doi:10.1039/c3cs60397k
Zhang, Z., and Lin, M. (2014). High-yield preparation of vertically aligned gold nanorod arrays via a controlled evaporation-induced self-assembly method. J. Mat. Chem. C 2, 4545–4551. doi:10.1039/c4tc00325j
Keywords: plasmonic nanoparticles, self-assemblies, hydrophobic surface, coffee ring effect, Raman spectroscopy
Citation: Usman M, Ishafaq MUU, Muhammad Z, Ali W, Dastgeer G, Zhang X and Wang L (2023) Evaporation-induced self-assembly of gold nanorods on a hydrophobic substrate for surface enhanced Raman spectroscopy applications. Front. Mater. 9:1048011. doi: 10.3389/fmats.2022.1048011
Received: 19 September 2022; Accepted: 30 November 2022;
Published: 25 January 2023.
Edited by:
Yue Li, Hefei Institutes of Physical Science (CAS), ChinaReviewed by:
Samir Kumar, Korea University, South KoreaGuangqiang Liu, Qufu Normal University, China
Copyright © 2023 Usman, Ishafaq, Muhammad, Ali, Dastgeer, Zhang and Wang. This is an open-access article distributed under the terms of the Creative Commons Attribution License (CC BY). The use, distribution or reproduction in other forums is permitted, provided the original author(s) and the copyright owner(s) are credited and that the original publication in this journal is cited, in accordance with accepted academic practice. No use, distribution or reproduction is permitted which does not comply with these terms.
*Correspondence: Xiao Zhang, Y2hhbmdzaHVpQGhvdG1haWwuY29t; Liang Wang, aGVhbHRoc2NpZW5jZUBmb3htYWlsLmNvbQ==