- 1State Key Laboratory of Oral Diseases and National Clinical Research Center for Oral Diseases, Sichuan University, Chengdu, China
- 2Department of Oral and Maxillofacial Surgery, West China Hospital of Stomatology, Sichuan University, Chengdu, China
- 3College of Foreign Languages and Cultures, Sichuan University, Chengdu, China
Due to the lack of blood vessels, nerves and lymphatic vessels, the capacity of articular cartilage to heal is extremely limited. Once damaged, it is urgent for articular cartilage to repair the injury. In recent years, there has been an increase in cartilage tissue engineering studies. Self-assembling peptide hydrogel as a kind of hydrogels composed of peptides and water is widely used in cartilage tissue engineering. Under noncovalent interactions such as electrostatic interaction, hydrophobic interaction, hydrogen bonding and pi-pi stacking force, peptides self-assemble into three-dimensional (3D) structures that mimic the natural extracellular matrix and allow cells to grow, proliferate and differentiate. Because SAPHs have excellent biocompatibility and biodegradability, variable mechanical properties, low immunogenicity, injectability, and the ability to load cells and bioactive substances, many researchers utilized them to promote the repair and regeneration of articular cartilage after damage. Therefore, the purpose of this review is to sum up the composition, injury characteristics, and treatments of articular cartilage, as well as the action of SAPHs in repairing articular cartilage damage.
1 Introduction
Articular cartilage is located throughout the body and performs various functions. However, it is often damaged as a result of trauma, aging, and disease (Guo et al., 2022). Articular cartilage injury frequently leads to progressive tissue degradation, joint pain, dysfunction, and degenerative arthritis (Borrelli et al., 2019; Stefani et al., 2020). According to the World Health Organization, symptomatic osteoarthritis affects an estimated 240 million people worldwide (Allen et al., 2022). Nevertheless, because cartilage lacks blood vessels, nerves, and lymph tissue, it is difficult for cartilage to repair itself after injury (Azami and Beheshtizadeh, 2021; Gorronogoitia et al., 2022; Huang et al., 2022). At present, clinical treatment methods mainly include microfracture surgery, osteochondral autograft transfer (OAT) or osteochondral allograft transplantation (OCA), autologous chondrocyte implantation (ACI), joint replacement, etc. Nevertheless, these therapies have limitations, such as the inability to reverse cartilage degeneration, fibrocartilage regeneration, donor site complications, immune rejection, prosthesis loosening, and joint infections, making articular cartilage repair ineffective (Redondo et al., 2018; Yan et al., 2021; Gan et al., 2022). Cartilage tissue engineering has thus emerged, combining physical and chemical stimuli with scaffolds that mimic the extracellular matrix (ECM) to reconstruct cartilage (Payam et al., 2021; Yang et al., 2021).
In recent years, injectable, highly adjustable cartilage tissue engineering and drug delivery compound hydrogels have been considered ideal for invasive treatment (Ziadlou et al., 2021). Hydrogels are three-dimensional (3D) hydrophilic networks of natural or synthetic polymers that are physically or chemically crosslinked and can be designed with different mechanical, physical and chemical properties for various applications (Yu et al., 2020; Sreekumaran et al., 2021). With a similar structure to natural ECM, hydrogels support cell migration, adhesion, proliferation, and differentiation (Liu et al., 2017; Tomic et al., 2021). Most hydrogels are formed by covalent crosslinking, such as polyethylene glycol diacrylate (Zhang et al., 2021b), polyvinyl alcohol (Nazouri et al., 2020), and polyethylene glycol (Zeng et al., 2021). Although these chemically cross-linked hydrogels are inexpensive, the safety of their long-term use is unclear because of trace amounts of harmful reagents, including initiators or catalysts, which limit their application in the field of tissue engineering (Dou and Feng, 2017). Other natural polymers such as hyaluronic acid (HA) and collagen are also studied extensively (Ha et al., 2015; Cai et al., 2020). However, uncontrollable gelation process, rapid degradation, potential immunogenicity, and some unidentified enzymes in natural polymers hinder their clinical applications (Yang et al., 2018).
Self-assembling peptide hydrogel (SAPH) consists of amino acids and requires few crosslinking reagents or organic solvents for manufacture (Fu et al., 2021; Matsugami et al., 2021). Noncovalent interactions, such as electrostatic interaction, hydrophobic interaction, hydrogen bonding, and pi-pi stacking force, are what primarily drive the self-assembly process. These interactions have a number of advantages over covalent bonding, including reversibility, high synthetic convergence, and precise design (Whitesides and Grzybowski, 2002; Sun et al., 2017). The reactivity of SAPHs to external stimuli including pH, temperature, ionic strength, or enzymes is another characteristic that they share (Chassenieux and Tsitsilianis, 2016; Sarkar et al., 2019; Wang et al., 2022b). As a result, SAPHs have a wide range of applications in tissue engineering due to their superior biocompatibility, biodegradability, changeable mechanical properties, minimal immunogenicity, and injectability (Thomas et al., 2021; Zanotto et al., 2021; Wang et al., 2022a; Zhu et al., 2022). Additionally, there have been a large number of studies on the use of SAPHs in the treatment of articular cartilage injury in recent years, but there are few reviews in this field. Therefore, thorough research must be done on SAPHs’ ability to repair articular cartilage damage.
2 The basic information of articular cartilage
According to the composition of ECM, cartilage can be divided into three categories: hyaline cartilage, elastic cartilage, and fibrocartilage (Wachsmuth et al., 2006; Zhang et al., 2018; Wu et al., 2020). The hyaline cartilage matrix is rich in glycoaminoglycans (GAGs) and highly adherent collagen fibers (mainly type II collagen), which are mainly found in joints called articular cartilage, and in the ribs, nose, etc. In elastic cartilage, type II collagen and elastic fibers are densely distributed in multiple directions and exist in meniscus, external ear, and eustachian tube. The matrix in fibrocartilage consists of tightly woven collagen fibers (mainly type I collagen), which makes fibrocartilage highly resistant to compression. And fibrocartilage can be found in the pubic symphysis, ear, and temporomandibular joint. Interestingly, hyaline cartilage has lower collagen content and higher proteoglycan (PG) content than fibrocartilage, while elastic cartilage and fibrocartilage have more mature collagen crosslinking structure than hyaline cartilage (Bielajew et al., 2021). Hyaline cartilage is widely distributed in the body, so the disability caused by hyaline cartilage injury accounts for a high proportion of the world, and in the United States alone, more than 250,000 patients require knee replacement surgery each year to treat articular cartilage injury (Milena et al., 2013; Parto et al., 2017). Therefore, this review mainly focuses on articular cartilage. Meanwhile, the treatment methods of articular cartilage can also be used as a reference for other hyaline cartilage treatments.
2.1 Composition and structure of articular cartilage
Articular cartilage is connective tissue covering the surface of the synovial joint epiphysis and is composed of chondrocytes and ECM, which is highly hydrated, nerveless, and bloodless (Figure 1A) (Mow et al., 1992; Goldring, 2012; Yao et al., 2022). As the only cell resource in cartilage tissue, chondrocytes occupy 5% cartilage tissues, having the function of synthesizing and secreting ECM (Servin-Vences et al., 2018; Zhang et al., 2021b). ECM is mainly composed of water, collagen, and PGs. Water accounts for about 65–80% of ECM wet weight, compared with 22–25% to collagen and 10–15% to PGs (Sophia et al., 2009). It should be pointed out that type II collagen accounts for 90–95% of collagen. Collagen is mainly related to the structural framework and tensile strength of cartilage, while PGs provide compressive recovery of articular cartilage (Gottardi et al., 2016; Tanska et al., 2018).
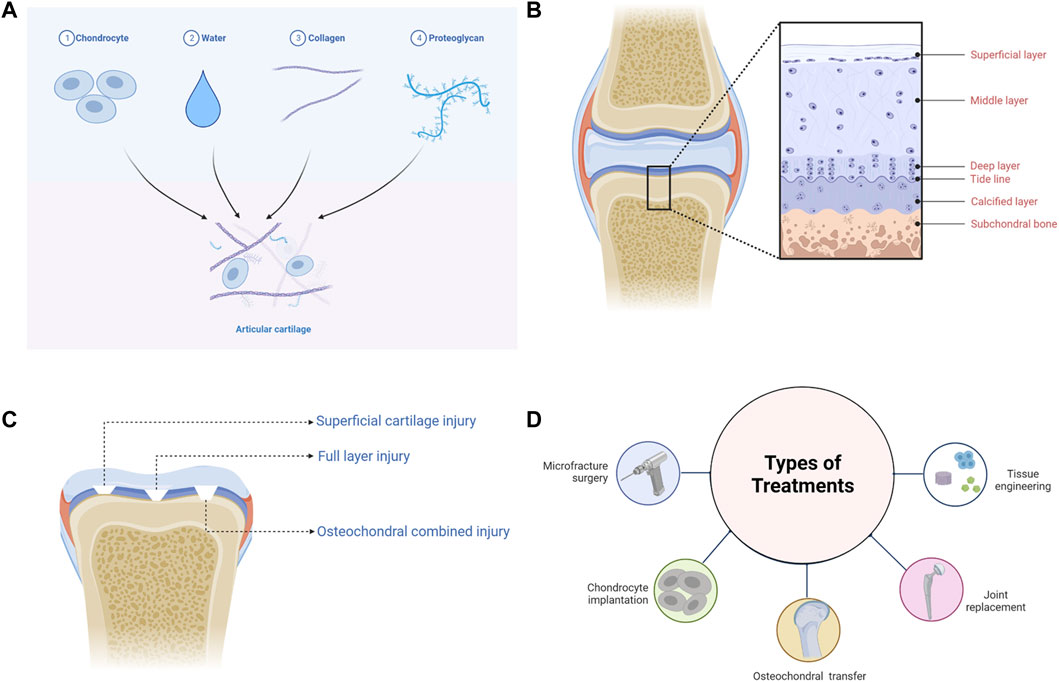
FIGURE 1. The basic information of articular cartilage. (A) The composition of articular cartilage. (B) The structure of articular cartilage. (C) The classification of articular cartilage injuries. (D) The treatments of articular cartilage injuries.
According to the arrangement and density of collagen fibers and PGs, articular cartilage can be divided into four distinct regions: the superficial layer, middle layer, deep layer, and calcified layer (Figure 1B) (Becerra et al., 2010; Danalache et al., 2021). The superficial layer is covered with synovial fluid, and type II collagen is densely distributed, while GAGs are least distributed in this layer (Muir et al., 1970). Chondrocytes located in the superficial layer are small and flat, and the cells and collagen fibers are arranged parallel to the surface of cartilage (Takagi et al., 1998; Elhamian et al., 2014; Elhamian et al., 2015). In the middle layer, chondrocytes are flattened and round, and collagen fibers arranged irregularly (Elhamian et al., 2014; Elhamian et al., 2015; Yin et al., 2018). The density of chondrocytes is lower than that of the superficial layer, while the distribution of GAGs is higher (Stockwell, 1967). Chondrocytes in the deep layer are oval and columnar in arrangement (Takagi et al., 1998; Yin et al., 2018). Collagen fibers are arranged perpendicular to the subchondral bone surface, among which type II collagen is the least distributed in the deep layer, while GAGs are the most distributed (Elhamian et al., 2014; Elhamian et al., 2015). There is a tide line transition from the deep layer to the calcified layer (Jiang et al., 2008). Hypertrophic chondrocytes are distributed in the calcified layer, and type X collagen is only visible in this layer, which contributes to the mineralization of articular cartilage (Shen, 2005; Antons et al., 2018). The calcified layer is connected to the subchondral bone downwards (Jiang et al., 2008).
2.2 Characteristics and challenges of articular cartilage injury
According to the International Cartilage Research Society (ICRS) Cartilage Defect Grading System, articular cartilage injuries can be divided into four grades: Grade 1 “superficial, blunt notch and superficial cracking”; Grade 2 “lesions extending down to <50% of cartilage thickness”; Grade 3 “cartilage defects extending down >50% of cartilage thickness but not up to subchondral bone”; Grade 4 “full thickness tear with subchondral bone exposure extending to the subchondral boneplate or deeper defect to the trabecular bone” (Merkely et al., 2018). Furthermore, articular cartilage injury can also be divided into simple cartilage injury (superficial cartilage injury and full layer injury) and osteochondral combined injury, according to the depth and level of involvement (Figure 1C) (Deng et al., 2019).
Superficial cartilage damage only affects three layers of articular cartilage surface and has not yet crossed the tide line. Due to the absence of blood vessels and lymphatic vessels, once superficial cartilage injured, other cells such as blood cells, bone progenitor cells, and mesenchymal stem cells (MSCs) cannot enter defect sites (Buckwalter, 1998). Repair can only be carried out by proliferation and migration of surrounding chondrocytes. Nevertheless, this kind of repair fails to restore the morphology and function of the defect, thus leading to permanent coloboma and degenerative osteoarthritis (Grenier et al., 2015; Hoelzl et al., 2022). The full-layer cartilage injury involves the entire cartilage that crosses the tide line and calcified layer to the subchondral bone. In addition to chondrocytes, other cells can also be recruited to repair this injury on account of the neurovascular network in the subchondral bone. Whereas, the cartilage produced is mainly fibrocartilage, and the functional recovery of the defect is not satisfactory (Hanie et al., 1992). The osteochondral injury penetrates the subchondral bone, resulting in damage to the cartilage and bone simultaneously (Berninger et al., 2013; Nie et al., 2020). The interaction between articular cartilage and subchondral bone ensures the homeostasis of the internal environment. But when injury occurs, achieving favourable repair of articular cartilage and subchondral bone is the biggest challenge facing the treatments of cartilage defects at present.
2.3 Treatment of articular cartilage injury
Microfracture is an arthroscopic procedure used to treat small and medium-sized cartilage defects (Figure 1D) (Weber et al., 2018). Debridement is performed first, and then holes are produced by puncturing the subchondral bone layer, enabling the inflow of blood (Liu et al., 2021; Medina et al., 2021). However, the newly generated cartilage is fibrocartilage, which has poor mechanical properties compared with the primary structure (Ebihara et al., 2012). Furthermore, abnormal cartilage tissue growth may occur in defect areas, resulting in an unsatisfactory long-term prognosis (Ebihara et al., 2012; Solheim et al., 2020).
Healthy cartilages derived from patients themselves or from other individuals can be grafted into regions of cartilage degeneration (Figure 1D). OAT is appropriate for tiny defects, although it has the probability to damage normal cartilage, and a secondary surgery may carry additional risks (Alonso-Polo et al., 2020; Deng et al., 2020; Shi et al., 2022). OCA is commonly used to treat severe osteochondral defects. But it is limited by the source of normal cartilage tissues and bears significant risks of immune rejection and disease transmission (Yang et al., 2020; Wang et al., 2022d).
ACI requires chondrocytes, which are extracted in vivo, to culture and amplify in vitro, and then chondrocytes are implanted to the damaged areas of articular cartilages to restore the defects (Figure 1D). The lesions > 2cm2 are appropriate for ACI (Matthews et al., 2022). ACI may, however, generate the same complications as OAT, and complete recovery of structure and function for the injured section is unrealistic (Hirschmueller et al., 2011; Su et al., 2021).
When the above treatments are ineffective, artificial joint replacements, which are made of titanium metal, ceramics, and other materials, can be implanted to relieve pain and restore the motor function of the joint (Figure 1D) (Meding et al., 2000; Kuroki et al., 2017; Aurich et al., 2018). Nevertheless, joint replacement is frequently limited by abrasion, the service life of prosthesis and infection (Batailler and Neyret, 2018).
In recent years, emerging tissue engineering techniques for cartilage have largely made up for the shortcomings of the above treatments (Figure 1D). And by combining tissue engineering cartilage with existing treatment methods, better morphological and functional recovery can be achieved. A 3D ECM-like scaffold structure can be constructed by using cells, scaffold materials, growth factors, etc. It is propitious to recruitment, proliferation and chondrogenic differentiation of chondrocytes and MSCs, thus promoting the repair of damaged cartilage (Bauza-Mayol et al., 2022; Mao et al., 2022; Yang et al., 2022). Among them, SAPHs offer significant advantages and application possibilities.
3 Self-assembling peptide hydrogels
In 1951, Pauling et al. first discovered self-assembling peptides (SAPs) and their fundamental molecular motifs of protein, namely α-helix and β-sheet (Pauling and Corey, 1951; Pauling et al., 1951). Under different conditions, α-helix and β-sheet could be converted into each other (Davidson and Fasman, 1967; Brack and Orgel, 1975; Yanagawa et al., 1984). The concept of peptides as well-defined nanostructures was not acknowledged, however, until the inadvertent discovery in 1990 that a SAP was a repeating segment in the yeast protein Zuotin, at which point the concept was adopted (Zhang et al., 1993). Since then, peptide nanofiber scaffolds have been developed as 3D scaffolds for a variety of applications in tissue engineering, cell culture, and repair and regenerative medicine (Li et al., 2019; Xu et al., 2019; Redondo-Gomez et al., 2020).
Self-assembly can be defined as disordered components forming hierarchically organized structures or patterns spontaneously and reversibly via specific, local interactions with the components themselves. At the molecular level, self-assembly is the organization of molecules without external guidance or management (Koutsopoulos, 2016). SAPs can spontaneously form ordered nanostructures such as nanotubes, nanoparticles, nanofibers, and nanoribbons, which eventually form SAPHs through noncovalent interactions including hydrogen bonding, electrostatic interaction, hydrophobic interaction, and pi-pi stacking force (Fan et al., 2017; Karavasili et al., 2017; Mijiddorj et al., 2020; Rani et al., 2020; Wang T. et al., 2022).
3.1 Noncovalent interactions of self-assembling peptides
SAPs self-assemble into 3D structures mainly through noncovalent interactions, including electrostatic interaction, hydrophobic interaction, hydrogen bonding, and pi-pi stacking force. According to Diaferia et al., hydrogen bonding, electrostatic interaction, and pi-pi stacking in peptide sequences played significant roles in the overall stability of peptides (Diaferia et al., 2021). In addition, pi-pi stacking is essentially a special hydrophobic interaction (Hu et al., 2009). Therefore, all four of the aforementioned intermolecular forces are essential for SAPs to maintain their own structures. Due to hydrophobic interaction, peptides composed of hydrophobic amino acids self-assemble to form SAPHs (Figure 2A). Polar amino acids with charged functional groups can also form SAPHs by means of hydrogen bonding and electrostatic interaction. Aromatic amino acids are capable of self-assembly by superimposing the pi-pi stacking force.
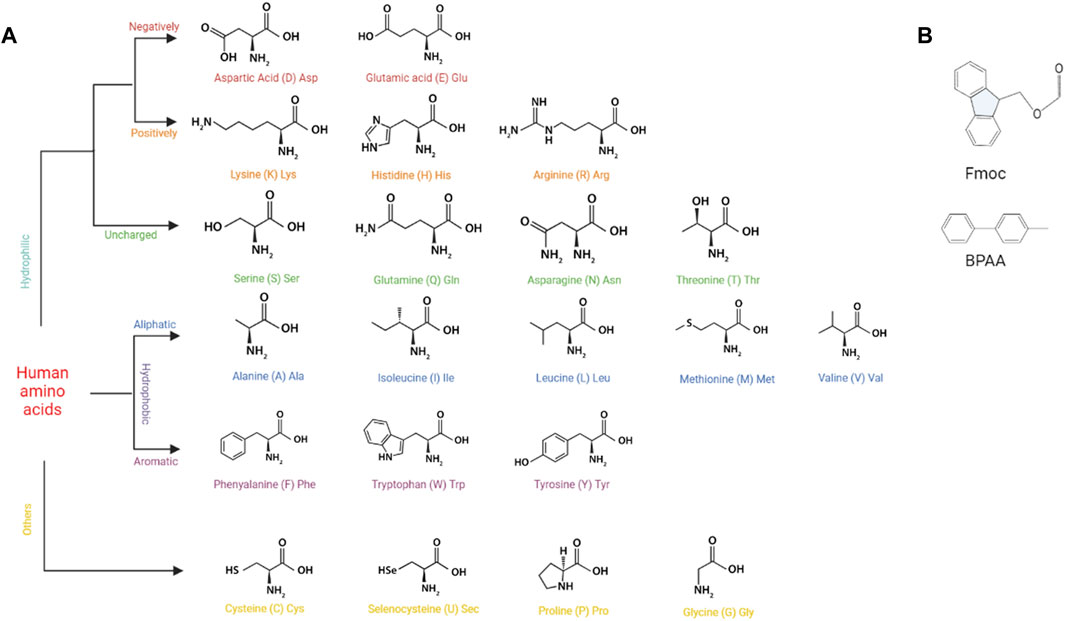
FIGURE 2. The basic structures of building components for SAPs. (A) The basic structures of human amino acids. (B) The basic structures of Fmoc and BPAA.
3.1.1 Electrostatic interaction
Electrostatic interaction, primarily between positive and negative ions, plays a crucial part in the self-assembly process of SAPs. Peptides with charged functionalities generate ion bonds by virtue of electrostatic interaction, and then self-assemble to form a secondary structure, maintaining the nanostructures’ stability. For instance, RADA16 nanofibers, which possess a hydrophilic surface composed of alternating arginine (R, positive charge) and aspartic acid (D, negative charge) residues, can intertwine to form a SAPH under the influence of electrostatic interaction (Nagai et al., 2006). External conditions, like pH, can affect electrostatic interaction. Consequently, modulating pH can achieve the influence of SAPH structures (Nagai et al., 2006; Zhang M. et al., 2021). Along with playing a role in the self-assembly of homogeneous SAPHs, electrostatic interaction also drives the self-assembly of heterotypic SAPHs. Shy et al. discovered that heterotypic SAPHs could be produced after mingling two solutions of peptides with opposite charges (Shy et al., 2021).
3.1.2 Hydrophobic interaction
Depending on the characteristics of their side chains, amino acids can be classified as hydrophilicity or hydrophobicity. In aqueous solution, their hydrophobic portions move inward, while their hydrophilic portions move outward. In the process of movement, peptides assemble in an irregular manner. Following that, hydrophobic and other interactions drive peptides to permute orderly and eventually shape a hydrogel (Criado-Gonzalez et al., 2020; Ruter et al., 2020; Yu et al., 2022). For example, MAX1 peptide, which in aqueous solution offers a hydrophobic interface of valine (V) residues and a hydrophilic interface of lysine (K) residues, conducted lateral assembly through hydrophobic interaction among side chains, finally generating a noncovalent crosslinking network (Lin et al., 2011). Meanwhile, Saini et al. also demonstrated that self-assembly of aliphatic peptides was mostly driven by hydrophobic interaction, and their designs based on hydrophobic Leucine-Isoleucine-Isoleucine (LII) motifs and a single residue polar terminus facilitated hydrogelation (Saini and Chauhan, 2014). Ren et al. found the enhancement of hydrophobic interaction between SAP molecules also contributed to the increase in the stiffness of hydrogels (Ren et al., 2020).
3.1.3 Hydrogen bonding
In the process of peptide self-assembly, the carbonyl and amide groups in the peptide backbone contribute to the formation of hydrogen bonding, which causes the peptides to align in a particular orientation and produce functional nanostructures. Since molecules that rely solely on hydrogen bonding for self-assembly are prone to undergo solution (sol) rather than gelation (gel), most hydrogels assembled by hydrogen bonding are amphiphilic (Omar et al., 2022). In proteins and peptides, hydrogen bonding is essential for the formation of secondary structures and super secondary structures. Wang et al. confirmed that the insertion of uncharged polar residues into the hydrophobic or hydrophilic interface of peptides could facilitate the emergence of super secondary structures via side-chain hydrogen bonding among β-sheet, eventually forming unique nanoribbon structures (Wang M. et al., 2018). Clarke et al. reported that the antiparallel β-sheet structure formed by lateral connection could enhance the strength of hydrogen bonding (Clarke et al., 2018). Additionally, adjusting hydrogen bonding of peptides could be realized through substitution of amino acid residues and strong intermolecular hydrogen bonding is conducive to instantaneous self-healing of hydrogels (Ren et al., 2020). And the incremental density of hydrogen bonding in peptides can strengthen the stiffness of nanofibers (Godbe et al., 2021). Among noncovalent interactions of biomolecules, hydrogen bonding is probably the most critical link in peptide self-assembly (Xing et al., 2022).
3.1.4 Pi-pi s tacking force
Pi-pi stacking mainly exists in aromatic amino acids, such as phenylalanine (F) and tryptophan (W), and is the driving force behind self-assembly of aromatic amino acids and 9-fluorenylmethyloxycarbonyl (Fmoc) modified aromatic amino acids (Figure 2B) (Bian et al., 2017; Koshti et al., 2022). For instance, the diphenylalanine (FF) sequence could provide sufficient pi-pi stacking force to allow self-assembly of 4-diphenylacetate-glycine-diphenylalanine (BPAA-GFF) and 4-diphenylacetate-alanine-diphenylalanine (BPAA-AFF), while the other two phenylalanines (BPAA-FGF and BPAA-FAF) could not develop a hydrogel owing to the absence of FF structure (Sun et al., 2022). In addition, pi-pi stacking force can affect the release rate of molecules carried by aromatic amino acids, so that controlled drug release can be actualized by modifying its magnitude (Qian et al., 2019).
3.2 Formation of self-assembling peptide hydrogels
The formation of SAPHs includes two stages: 1) Peptides self-assemble to form secondary structures and nanofibers; 2) Nanofibers elongate, combine and tangle to form a 3D network. Secondary structures mainly involving α-helix, β-sheet, β-turn and β-hairpin assemble into nanotubes, nanoparticles, nanofibers, and nanoribbons, which crosslink to produce ECM-like hydrogels (Figure 3) (Fu et al., 2021; Hao et al., 2022).
The α-helix, which is self-assembled by hydrophobic interaction, is one of the main secondary structures. The α-helical coiled coil is also known as hydrogenated self-assembled fibers (Ryadnov and Woolfson, 2003; Papapostolou et al., 2007). A typical α-helical peptide is composed of repeated amino acid sequences, with a sequence of seven residues (abcdefg) as its basic unit (Banwell et al., 2009; Fletcher et al., 2011). The a and d positions (hydrophobic residues) and the e and g positions (charged residues) are responsible for directing the dimer interface between adjacent α-helical peptides (Fu et al., 2021). Meanwhile, amino acids with a high helical tendency, especially L and A, are often placed at sites a and d as hydrophobic amino acids (Chen and Zou, 2019; Fu et al., 2021). Residues at positions b, c, and f, which are often occupied by hydrophobic A or glutamine (Q) with a tendency to form hydrogen bonding, are exposed to the surface of the component and vary from design to design (Chen and Zou, 2019). The composition of the α-helical coiled coil can be controlled by changing environmental conditions such as concentration, pH, and ionic strength (Fletcher et al., 2011; O'Leary et al., 2011; Kornmueller et al., 2015; Huang et al., 2014).
The β-sheet is composed of almost completely extended peptide chains. Peptides with β-sheet structures are composed of 12–16 alternating hydrophilic (R or K as positive groups, Glutamic acid (E) or Aspartic acid (D) as negative groups) and hydrophobic residues (A or L group), and in the aqueous medium, their hydrophilic portions are distributed on one side, hydrophobic portions on the other side under the effect of hydrophobic interaction (Chen and Zou, 2019). According to the arrangement of the charge on the hydrophilic side, β-sheet structures can commonly be divided into four moduli: modulus I “- + - + - + -+“; modulus II “--++--++”; modulus III “---+++”; and modulus IV “----++++” (Zhang, 2003). The formation of β-sheet structure is the result of hydrophobic interaction and electrostatic interaction (Barco et al., 2018; Zhang et al., 2020). Meanwhile, intermolecular hydrogen bonding is the main driving force for β-sheet stability (Godbe et al., 2021). It has been found that the structure of SAP layered supramolecules depends on the number of β-sheet formed (Barco et al., 2018). Moreover, Clarke et al. found that by altering the density and concentration of β-sheet peptide grafted into the polymer network, the mechanical properties of the composite hydrogel could be regulated in the range of 10–200 kPa (Clarke et al., 2017).
The β-turn is also a simple secondary structure of peptides. The β-turn usually consists of four amino acid residues, each of which is represented as i, i + 1, i + 2 and i + 3, and the distance between i and i + 3 residues is less than 7 Å(Panasik et al., 2005; Fu et al., 2009; Yu et al., 2018). The specific conformation of β-turn depends on its constituents to some extent, and amino acids such as G and Proline (P) are often present in the β-turn (Fu et al., 2009; Zhang R. et al., 2022). G lacks side chains, merely one hydrogen atom, so it can splendidly modulate the spatial obstruction of other residues in the β-turn. P has a ring structure and fixed angle, which can constrain the formation of β-turn. Although tetrapeptides are the most common structure, dipeptides and tripeptides can form β-turn as well (Boussard and Marraud, 1985). Yu et al. described how the secondary structure of peptides realized the transformation from β-sheet to α-helix and β-turn in the process of SAPH formation, and β-turn was pivotal to stabilizing the SAPH (Yu et al., 2022).
The β-hairpin structure consists of two inversely parallel β-sheet components and a β-turn region. The formation of the β-hairpin structure is derived from hydrophobic interaction and electrostatic interaction (Zhang et al., 2020). Altering the hydrophobic properties of β-hairpins structures can be employed to control the temperature at which peptide folding and self-assembly are triggered (Rughani and Schneider, 2008). Furthermore, Haines et al. incorporated a charged residue into the hydrophobic surface, which caused a shifty electrostatic interaction and disrupted the hydrophobic interaction, thus inhibiting the folding and self-assembly of β-hairpin peptides (Haines et al., 2005). Therefore, it is possible to modify the self-assembly characteristics and properties of β-hairpin peptides by changing the sequence of hydrophilic and hydrophobic amino acids and the number of charged residues (Branco et al., 2009).
3.3 Classification of self-assembling peptide hydrogels
Different researchers have classified SAPHs differently. For example, Hao et al. thought that SAPs included iterative peptides, amphiphilic peptides, and multi-repeating helical peptides, among others (Hao et al., 2022). Based on the selection and distribution of hydrophilic amino acids, the first subtype can be classified into ionic-complementary peptides, self-repulsive peptides, multidomain peptides, β-hairpin peptides and β-tape peptides. The second subtype is distinguished by its long hydrophobic tail and its hydrophilic head. According to the composition of hydrophobic tails, amphiphilic peptides can be divided into four categories: peptide amphiphiles, aromatic peptide amphiphiles, ultrashort peptides, and aromatic short peptides (Hao et al., 2022). The last multi-repeating helical peptides made from repeating units include α-helical coiled-coil peptides, β-spiral elastin-like polypeptides, and triple-helical collagen-mimetic peptides (Hao et al., 2022).
3.4 Influence factors of self-assembling peptide hydrogels
Peptide-based hydrogels undergoing sol-gel transformations in response to external stimuli such as temperature, pH, and ionic strength are known as stimulus-responsive or “smart” hydrogels (Figure 4) (Katyal et al., 2020; Ligorio et al., 2022). In addition, enzymes can influence the assembly of SAPHs (Shy et al., 2019; Wu et al., 2019). Changes in temperature regulate the mechanism and process of peptide self-assembly by primarily disrupting hydrogen bonding and altering hydrophobic interaction in SAPs (Jalali et al., 2022). Among the amino acid residues in SAPs, pH mostly affects the carbonyl and amide groups. As a consequence, the self-assembly of SAPs can be administrated by modulating the competitive solvation between the donor and acceptor sites of the hydrogen bonds. Besides, the pH of the solution can influence the charges to modify electrostatic interaction in SAPs (Nagai et al., 2006; Zhang M. et al., 2021). Similarly, ionic strength functions in electrostatic interaction (Barco et al., 2018). Enzymes can make precursor molecules convert into SAPs, and then peptides self-assemble into SAPHs under the influence of various intermolecular forces.
3.4.1 Temperature
Temperature is the most prevalent approach for achieving reversible self-assembly of peptides. Different temperatures will lead to sol-gel transition of SAPs, and this transformation is reversible within a specific temperature range (Panda et al., 2008; Zhu et al., 2021). At a critical gel concentration of 5 mM, the solution of BPAA-FF developed a homogeneous and transparent hydrogel by a heating and cooling process, and after damage by external pressures, the hydrogel could be restored in the same manner (Bian et al., 2017). The current research focus is to achieve controlled release of therapeutic drugs by adjusting the gelation temperature of SAPHs. For instance, Li et al. utilized SAPHs to encapsulate doxorubicin, which could be released to kill tumors via shifting temperature (Li B. et al., 2022). Meanwhile, temperature is an essential stimulant for the formation of β-sheet peptide nanofibers. RADA16 could retain 46% β-sheet structures during thermal denaturation from 25 to 70°C, but at higher temperatures, it would assemble into small spherical aggregates (Ye et al., 2008). As the density of β-sheet affects the stiffness of SAPHs, temperature regulation is critical in preparing peptide-based biomaterial scaffolds.
3.4.2 pH
Altering pH is a common, convenient, and direct method to prepare supramolecular hydrogels. The sol-gel transition of SAPHs could be achieved by adjusting pH of the solution (Sun et al., 2022). pH mainly affects charged amino acids and hydrogen bonding, resulting in self-assembly of SAPs (Lopez-Silva et al., 2019). For example, Dehsorkhi et al. found the structure of SAP transformed from spherical micelles to nanofibers, when the pH of the solution increased (Dehsorkhi et al., 2013). Furthermore, Zhang et al. demonstrated in acidic microenvironments (pH 6.5) that negative charged pharmaceutical peptides could be activated to be positive charged and self-assemble into SAPH with a prominent capability of cell uptake and endocytosis (Zhang J. M. et al., 2022). The change of repulsion force between charges caused by adjusting pH can affect the sol-gel transition of SAPH and the quantity of electrostatic charge can also affect the pH of the environment in the process of self-assembly (Fletcher et al., 2011; Kaur et al., 2020).
3.4.3 Ionic strength
Ions can affect the formation of β-sheet through electrostatic interaction, and SAPHs with diverse structures and properties can be produced under different ion concentrations (Barco et al., 2018). Although hydrophobic interaction and hydrogen bonding are considered to be the dominant forces for self-assembly, it is now known that the introduction of additional ionic groups into gel designs can create highly stable supramolecular structures (Kaur et al., 2020). For example, the introduction of biologically relevant metal ions (Ca2+/Mg2+) into the SAP enables it to self-assemble at physiological pH, and achieves tunable mechanical strength and improved cellular response (Pal and Roy, 2022). Furthermore, the SAPs synthesized by the Stupp group could achieve the sol-gel transition by adding Ca2+, mainly by eliminating electrostatic repulsion between nanofibers (Matson et al., 2012).
3.4.4 Enzyme
Enzymes can specifically degrade the target groups in peptide chains to form SAPs, which self-assemble into nanofibers, thus converting them into SAPHs. Enzymatic reactions usually involve enzyme-catalyzed chemical bond breaking, the most common of which is the dephosphorylation of phosphate by phosphatase (Hu et al., 2022; Runser et al., 2022). For poorly soluble SAPs, it is possible to synthesize precursors with high solubility and then form SAPHs with the help of enzymes (Li X. et al., 2022). Enzymes can also catalyze the binding of compounds to peptides, hence improving the weak mechanical properties of SAPHs (Okesola et al., 2020). In addition, Guilbaud et al. found that FEFK tetrapeptide was hydrolyzed by a protease enzyme to develop two kinds of octapeptides, FEFEFKFK and FEFKFEFK, which were prone to self-assembly, and the presence of the enzyme led to the improvement of mechanical properties of SAPHs as well (Guilbaud et al., 2013). Yu et al. found that after 120 or 150 min of hydrolysis by alkaline protease, buckwheat proteins could self-assemble into a SAPH without any chemical induction or treatment (Yu et al., 2022).
3.5 Advantages of self-assembling peptide hydrogels
Firstly, SAPs consist of natural amino acids, which do not interfere with the body’s normal metabolism and undergo a sol-gel transition without the need for any toxic cross-linkers that are usually needed for natural and synthetic hydrogels (Pan et al., 2016; Fu et al., 2021; Matsugami et al., 2021). Moreover, based on their compositions, SAPs can be well biodegraded by various proteases in vitro and in vivo and exhibit superior biocompatibility with tissues (Kim et al., 2015; Lu et al., 2018; Ishikawa et al., 2021). In addition, various studies showed that SAPs did not elicit an immunogenic or inflammatory response in vitro or in vivo (Miller et al., 2010; Lv et al., 2020; Dufour et al., 2021). Along with their biocompatibility and biodegradability, SAPHs are highly hydrated (>95%) and composed of a nanofibrous microenvironment that imitates the native ECM and creates an appropriate platform for cell encapsulation and 3D culture (Miller et al., 2010; Recha-Sancho et al., 2016; Mohammed et al., 2021). Through noncovalent interactions, especially electrostatic interaction, some bioactive molecules including growth factors, Decorin molecules and Chondroitin Sulfate (CS) can be encapsulated in SAPHs and released for several days (Kopesky et al., 2014; Recha-Sancho and Semino, 2016a). SAPHs are shear thinning and can transform from solid to the low-viscosity or liquid state under the high shear rate, mainly due to the long peptide nanofibers and their noncovalent interaction (Jonker et al., 2012; Yamada et al., 2019). Therefore, SAPHs can be easily injected via syringes or catheters, with the ability to recover their bulk properties after injection/transplant with a few seconds due to their fast self-assembled speed, which makes them an ideal candidate for non-uniform cartilage defects (Sun et al., 2016; Dufour et al., 2021). Natural materials including collagen, fibrin, alginate or HA among others present variability from batch to batch, while SAPHs are reproducible, controllable and customizable (Recha-Sancho and Semino, 2016a; Recha-Sancho et al., 2016). Moreover, mechanical properties of SAPHs can be modulated by changing the peptide sequence, concentration and external conditions (Fernandez-Muinos et al., 2015; Li et al., 2019). Nowadays, some SAPHs are readily available in the market and have found their way into clinical products (Gelain et al., 2021).
4 Self-assembling peptide hydrogels in repairing articular cartilage defects
Some studies have shown that SAPs could promote the regeneration of cartilage by promoting cell proliferation and differentiation (Kisiday, 2002; Kisiday et al., 2019). However, other studies have found SAPs exhibit insufficient contribution to articular cartilage regeneration due to the lack of specific motifs in their native sequences (Li et al., 2017; Lu et al., 2018). Therefore, binding some short functional peptides to SAPs to form new functionalized SAPHs becomes an alternative treatment. Along with SAPHs themselves, cells and bioactive substances such as growth factors and miRNA also play a significant role in cartilage repair. By embedding them into SAPHs, cells or bioactive substances can play the effect of one plus one more than two. Since some researchers reported SAPHs were in poor mechanical properties, adding other materials can meet the clinical need of cartilage repair (Figure 5; Table 1) (Ishikawa et al., 2020; Ishikawa et al., 2021).
4.1 Self-assembling peptide hydrogels alone
In SAPHs, KLD-12 peptide is often used as a scaffold material for cartilage defect repair. Kisiday et al. reported that KLD-12 hydrogel could maintain the phenotype and facilitate ECM secretion of chondrocytes (Kisiday, 2002). Furthermore, the stiffness of SAPH increased with the accumulation of ECM (Kisiday, 2002). Kisiday et al. also confirmed that culturing BMSCs in KLD-12 SAPH supported their differentiation into chondrogenic mesenchymal stem cells (CMSCs) (Kisiday et al., 2019). In vivo, Miller et al. used KLD-12 hydrogel to treat critical size lesions involving full-layer of rabbit cartilage, and it filled defects in situ, facilitating cartilage repair (Miller et al., 2010). When applied to equine cartilage defect, it could also prevent morphological changes in the defect regions compared with microfracture treatment alone (Miller et al., 2014).
Some short functional peptides can bind to the C-terminus of KLD-12 to form new functionalized SAPHs, without eliminating the biological function of the short peptides themselves. As one of the link protein degradation products, link protein N-terminal peptide (LPP) played an important role in regulating the proliferation and ECM synthesis of chondrocytes as well as in inducing directional migration of cartilage-derived stem cells (He et al., 2018). LLP was bound to KLD-12 to obtain KLD-12-LPP SAP, which was mixed with KLD-12 SAP in 1:1 to self-assemble into KLPP hydrogel. The KLPP hydrogel ameliorated the interface integration of cartilage defects and boosted cartilage regeneration by promoting the recruitment of endogenous chondrocytes and BMSCs(Lv et al., 2020). N-cadherin, a major component of adhesion junctions, was a transmembrane protein that mediated intercellular interactions and intracellular signal transduction, which was essential for the development and formation of articular cartilage (Gao et al., 2010). Li et al. synthesized n-cadherin functionalized peptide hydrogels (KLD-Cad), which could self-assemble in phosphate buffered saline at 37°C, by combining a simulated peptide (HAVDI) with KLD-12 peptide (Li et al., 2017). KLD-Cad hydrogel could enhance the chondrogenesis of encapsulated human mesenchymal stem cells (hMSCs) via inhibiting the typical Wnt signaling pathway in hMSCs (Li et al., 2017). Substance P (SP) was an endogenous neuropeptide consisting of 11 amino acids that increased the proliferation rate of chondrocytes in a dose-dependent manner, and promoted tissue regeneration through recruiting endogenous stem cells and angiogenesis (Sook et al., 2009; Opolka et al., 2012; Muhammad et al., 2015). KLD-12-SP SAP was synthesized by combining SP neuropeptide with KLD-12 (Kim et al., 2016). KLD-12-SP SAPH could improve cartilage regeneration by influencing the anti-inflammatory effect of cytokines, inducing endogenous MSC recruitment, and inhibiting chondrocyte apoptosis in rat knee osteoarthritis for 28 days (Kim et al., 2016).
Aromatic short peptides have nanofiber structures like natural collagen fibers and can be used as cell carriers to construct bionic ECM scaffolds for cartilage tissue engineering. BPAA-β alanine-FF tripeptides (BPAA-βAFF) could self-assemble into a hydrogel with β-sheet by temperature transformation, ion induction, and pH change, and could be preserved in rabbit articular cartilage defects by injection (Li et al., 2021). In vitro BPAA-βAFF facilitated chondrocyte proliferation, GAGs and collagen secretion (especially type II collagen) by simulating natural collagen environment (Li et al., 2021). Another n-cadherin functionalized peptide containing His-Ala-Val (HAV) motifs could not develop a hydrogel at pH 7.4, but via the usage of Fmoc-FF which could generate tremendous pi-pi stacking force, HAV peptides self-assembled into SAPH (Mohammed et al., 2021). The SAPH enhanced the differentiation of hMSCs into chondrocytes through stiffness regulation of the matrix, thus promoting the formation of cartilage (Mohammed et al., 2021).
Other SAPHs also assist in the repair of cartilage defects. Bovine chondrocytes were cultured in FEFEFKFK SAPH under two-dimensional (2D) and 3D conditions for 21 days (Mujeeb et al., 2013). It was found that 2D culture resulted in type I collagen deposition, while 3D culture kept good morphology of chondrocytes and a large amount of type II collagen deposition (Mujeeb et al., 2013).
4.2 Growth factors
Growth factors are soluble bioactive molecules capable of inducing specific cellular responses in the biological environment, so as to stimulate the growth of natural tissues. Cellular responses primarily include differentiation, proliferation, and survival (Ren et al., 2019; Nima et al., 2021). The administration of growth factors, however, has been a technical challenge due to their fragile structures and short half-life. Nowadays, their sustained effects can be achieved by employing scaffold materials loaded with growth factors.
Transforming growth factor β (TGF-β) belonged to the TGF superfamily and were cytokines that could induce the differentiation of MSCs and promote the secretion of type II collagen of MSCs (Ghandforoushan et al., 2022; Ye et al., 2022). Kisiday et al. found that compared with the agarose hydrogel group equipped with TGF-β, the gene expression of type X collagen of CMSCs in SAPH alone group was 65 times higher, and 7% of CMSCs expressed histocompatibility complex II (MHCII), resulting in immunogenicity (Kisiday et al., 2019). Therefore, Kisiday et al. concluded that TGF-β should be incorporated into SAPHs to diminish the generation of type X collagen and MHCII (Kisiday et al., 2019). When TGF-β1 was encapsulated into KLD-12 SAPH, the retention rate of TGF-β1 was 5 times higher than that of the agarose hydrogel, and the initial release rate was slower (Kopesky et al., 2011; Kopesky et al., 2014). Furthermore, TGF-β1-loaded KLD-12 hydrogel could stimulate chondrogenesis of horse BMSCs (Kopesky et al., 2011; Kopesky et al., 2014). KLD-12 SAPH equipped with TGF-β1 and platelet-derived growth factor-BB (PDGF-BB), which possessed chemotactic effect, could migrate bone marrow progenitor cells in the SAPH to defect areas and recruit more endogenous cells to aid microfracture surgery for cartilage repair (Ponte et al., 2007; Liebesny et al., 2016).
Bone morphogenetic protein 2 (BMP-2), a member of TGF-β superfamily, plays an important role in cartilage development. Dufour et al. cultured nasal chondrocytes pretreated with BMP-2, insulin, and triiodothyronine in IEIK13 SAPH, which could effectively promote proliferation and cartilage formation of nasal chondrocytes (Dufour et al., 2019). In addition, it was found to accelerate cartilage production when applied to monkey articular chondrocytes, and in vivo, IEIK13 hydrogel could promote cartilage repair and regeneration by recruiting surrounding BMSCs to the defect (Dufour et al., 2021).
Insulin-like growth factor 1 (IGF-1) facilitates the production of aggregation proteins and reduces their catabolism, but unmodified IGF-1 has a short half-life and can easily diffuse outside of the joint, leading to unfavorable side effects (Tyler, 1989; Shi et al., 2020; Prabhath et al., 2022). Sustained and local delivery of IGF-1 to cartilage can come true by combining the heparin-binding epidermal growth factor-like growth factor (HB-EGF) with IGF-1 to generate heparin-binding insulin-like growth factor 1 (HB-IGF-1) (Mujeeb et al., 2013; Sarkar et al., 2019; Zhang X. et al., 2021). Via electrostatic action, HB-IGF-1 was adsorbed into RADA16 SAPHs contributing to cartilage repair by enhancing ECM production and boundary integration with natural tissues (Florine et al., 2015). Zanotto et al. utilized KLD-12 SAPH equipped with PDGF-BB and HB-IGF-1 to treat cartilage injury in the equine femoral patella joint with pancreatic enzyme pretreatment and microfracture technology (Zanotto et al., 2021). Although complete regeneration of hyaline cartilage could not be achieved, compared with microfracture technique alone, the PDGF-BB/HB-IGF-1/KLD-12 hydrogel group acquired more restorative healing (i.e., more type II collagen and PG content in the repaired tissue), more favorable cartilage histological results, and stronger biomechanical properties (Zanotto et al., 2021). Similar results were obtained by using PDGF-BB/HB-IGF-1/KLD-12 hydrogel accompanied with pancreatic enzyme pretreatment in rabbit cartilage defect (Zanotto et al., 2019).
4.3 Composite scaffold
Sometimes, the mechanical properties of SAPHs cannot meet the needs of clinical treatment. The use of multi-component composite scaffolds can not only enhance the mechanical strength of SAPHs, but also further simulate the ECM environment through multiple functional segments of several components.
An interpenetrating polymer network (IPN) hydrogel was synthesized by chitosan cross-linked with poly (ethylene glycol)-block-poly (dl-lactide)-block-poly (ethylene glycol) as the covalent cross-linked networks with hydrolysis properties and RADA16 peptides as the self-assembling networks (Ishikawa et al., 2021). Based on the hydrolysis of the poly (dl-lactide) domain, the IPN hydrogel showed overall degradability, and the increase in spatial structure caused by degradation could promote the production and accumulation of ECM of chondrocytes in the hydrogel (Ishikawa et al., 2021). And the scaffold induced chondrocytes to express hyaline cartilage-related genes, preventing the expression of fibrocartilage-related genes, which benefited the repair of articular cartilage (Ishikawa et al., 2021). Another IPN hydrogel formed by the covalent network of chitosan cross-linked N-hydroxysuccinimide ester-terminated poly (ethylene glycol) and RADA16 peptide self-assembly network could also promote cartilage repair, but the repair was more oriented to fibrocartilage (Ishikawa et al., 2020; Ishikawa et al., 2021).
Polycaprolactone (PCL) scaffolds also have high mechanical strength, but due to their structural and functional characteristics, it is difficult for PCL scaffolds to imitate the 3D microenvironment of cells in vivo, thus restraining from the purpose of cell culture. A composite scaffold composed of FEFEFKFK SAPH coating and 3D-printed PCL scaffold could promote chondrocyte proliferation and cartilage regeneration in vitro, and in addition, significantly induced cartilage and subchondral bone regeneration after 8 and 12 weeks of implantation in rabbit femoral condyle (Li et al., 2019). PCL/RADA16 complex, which combined RADA16 SAP with PCL scaffold, was used to culture dedifferentiated human articular chondrocytes and provided important mechanical requirements for cartilage replacement and a biomimetic microenvironment to re-establish the chondrogenic phenotype of human expanded articular chondrocytes (Recha-Sancho et al., 2016).
Other polymer synthetic materials can also be integrated with SAPHs as scaffold materials for cartilage tissue engineering. Polyacrylamide and EFK were covalently combined to develop a polymer double network gel (PS-DN gel), which was suitable for the culture of the chondrogenic cell line ATDC5 due to its adjustable mechanical properties (Sun et al., 2016). The composite scaffold, which could promote the expression of genes and proteins related to cartilage formation in rat precartilaginous stem cells, was synthesized by RADA16, poly l-lysine-coated poly (lactic-l-glutamic acid) (PLGA) and plasmid DNA containing hTGFβ3 and may be a scaffold suitable for cartilage repair (Pan et al., 2016). The KLD12-CMP7 SAP was synthesized by combining KLD-12 with collagen mimetic peptides (CMPs), which could bind to intracellular collagen and promote rapid accumulation of ECM (Lee et al., 2008). To compensate for the weak mechanical properties of KLD12-CMP7 SAPH, poly (l-lactate-co-caprolactone) (PLCL) scaffold was added to produce PLCL-SAP hydrogel complex, and rabbit BMSCs were also encapsulated in the system as a whole (Kim et al., 2015). Researchers confirmed that this PLCL-SAP hydrogel complex could effectively promote chondroblast differentiation of rabbit BMSCs in vivo and in vitro, resulting in repairing cartilage defects (Kim et al., 2015).
In addition to synthetic polymers, some natural bioactive substances can also be combined with SAPs to form composite scaffolds that can restore cartilage damage. A composite hydrogel scaffold by uniting acellular cartilage matrix (ACM) with a bone marrow homing peptide (PFS)-functionalized SAP could facilitate the proliferation, adhesion, and chondrogenic differentiation of rabbit MSCs in vitro (Lu et al., 2018). In vivo, the ACM/SAP scaffold promoted endogenous MSCs recruitment and cartilage-like tissue formation at defects as well, and the quality of cartilage repair was higher than that in the microfracture group (Lu et al., 2018). Recha-Sancho et al. mixed RADA16 with CS or Decorin to develop two bioactive materials to imitate the native ECM respectively, in order to induce redifferentiation of human articular chondrocytes and differentiation of human adipose-derived stem cells (ADSC) into chondroblasts (Recha-Sancho and Semino, 2016a). Results showed that both bioactive materials could induce chondrogenic differentiation and mature cartilage ECM synthesize (Recha-Sancho and Semino, 2016a). Barco et al. found that SAP P11-X family (P11-4, P11-8) with CS might supplement the depleted GAGs on the surface of damaged cartilage by mimicking the surface environment of cartilage under physiological conditions, and be used as one of the potential therapies for minimally invasive treatments of early osteoarthritis (Barco et al., 2018). But Barco et al. did not conduct experiments to prove.
4.4 Cell
Chondrocytes are the only resident cells in mature articular cartilage and are therefore responsible for the synthesis and remodeling of ECM. In the therapy of chondrocyte-based cartilage injuries, the therapeutic effect is limited by the number of chondrocytes, and therefore, cell expansion in vitro is required. However, chondrocytes tend to lose their phenotype and develop hypertrophy in the process of expansion, causing them to be characterized by decreased synthesis of PGs and type II collagen, and increased secretion of type I and X collagen (Chung and Burdick, 2008; Bian et al., 2013; Demoor et al., 2014; Recha-Sancho and Semino, 2016b). Consequently, the current strategy is to use tissue-engineered scaffolds to maintain the differentiated state of chondrocytes and impel cells to chondrogenesis (Liu et al., 2019). In a prospective multicenter phase III clinical study, hydrogels with autologous chondrocytes were implanted to treat knee articular cartilage defects, and over a 2-year follow-up, repair tissues matured, recombined, and integrated with surrounding tissues, demonstrating that hydrogel-based ACI was a valuable measure for the treatment of knee cartilage defects in patients (Niemeyer et al., 2022). Other exogenous MSCs could also play a similar role in the treatment of cartilage defects as autologous chondrocytes.
Bussmann et al. used RADA16 SAPH with human normal dermal fibroblasts to build the 3D structure of cartilage (Bussmann et al., 2016). In the process of culture, the change of cell morphology first occurred, followed by an increase in the expression of cartilage-related proteins, confirming that the system could form cartilage-like structures, and there was a prospect as a treatment for cartilage defects (Bussmann et al., 2016). BMSCs were cultured in KDL-12 SAPH, and after 21 days of chondrogenic induction, the biomechanical properties of the aggregation proteins produced by BMSCs were superior to those of horse articular cartilage at the corresponding age, supporting the use of adult BMSCs for cartilage defect repair (Lee et al., 2010). Kim et al. utilized PLCL-SAP hydrogel complex loaded with rabbit BMSCs as a composite material, and the differentiation of rabbit BMSCs into chondroblasts was observed both in vivo and in vitro (Kim et al., 2015). Zheng et al. devised a lentiviral vector of LV-mTGF-β3 to direct the long-term stable release of TGF-β3 with matrix metalloproteinase (MMP) so as to facilitate the differentiation of encapsulated ADSCs within RPG SAPH scaffold into chondrocytes, and similar conclusions could obtain in vivo (Zheng et al., 2015).
Some researchers have also compared the therapeutic effects of different cell types on cartilage repair. Kisiday et al. found that compared with ADSCs, BMSCs encapsulated in SAPH had a stronger potential for chondrogenic differentiation (Kisiday et al., 2008). Erickson et al. demonstrated that ECM produced by chondrocytes in Puramatrix SAPH had better mechanical properties than that from MSCs in SAPH (Erickson et al., 2009). Other researchers, however, have confirmed that when KDL-12 hydrogel coated equine chondrocytes and MSCs of different ages for chondroblast induction, MSCs in SAPH contributed to favorable mechanical stiffness of the new generated cartilage tissue compared with the chondrocyte group (Kopesky et al., 2010). In addition, the aggregation proteins produced by MSCs had more phenotypic characteristics of young tissue, indicating better chondrogenic performance of MSCs (Kopesky et al., 2010).
4.5 Other biologically active molecules
In addition to carrying cytokines and cells to treat cartilage defects, SAPHs can load some other bioactive molecules as well, such as miRNA and heparin, for therapeutic purposes.
An aging-related miRNA, miR-29b-5p, upregulation of which could inhibit the expression of MMPs and aging-related genes (P16INK4a/P21), was significantly down-regulated in osteoarthritis cartilage (Zhu et al., 2022). By hydrogen bonding and electrostatic interaction, miR-29b-5p could attach to a stem cell homing peptide SKPPGTSS for recruiting endogenous synovial-derived mesenchymal stem cells (SMSCs), and then SKPPGTSS was bound to RADA16 sequences, forming a SKP@miR SAP (Sun et al., 2018; Zhu et al., 2022). On the one hand, the sustained release of miR-29b-5p could inhibit chondrocyte senescence to protect the joint, thereby alleviating the imbalance of matrix catabolism and anabolism to avoid excessive or accidental clearance of damaged chondrocyte population caused by senolytic therapy (Zhu et al., 2022). On the other hand, SKPPGTSS peptide in SKP@miR augmented the number of new chondrocytes by recruiting endogenous SMSCs and inducing differentiation into chondrocytes, which further contributed to the rejuvenation of aging joints (Zhu et al., 2022).
After using RADA16 SAP with heparin sodium salt, the material enhanced the specific binding and release capacity of growth factors due to the presence of heparin portion, and researchers found that this novel scaffold could improve ADSCs survival and chondrogenesis capacity (Fernandez-Muinos et al., 2015). Other researchers applied RADA16 SAPH loaded with bilayer heparin as a platform for 3D culture of dedifferentiated human articular chondrocytes, and after a period of culture, the scaffold material promoted phenotypic remodeling and ECM secretion of articular chondrocytes (Recha-Sancho and Semino, 2016b).
Icariin, as the main active component of Herb Epimedium, could boost the proliferation of chondrocytes and the synthesis of ECM (Kankala et al., 2018; Wang et al., 2020). The usage of RADA16 SAPH equipped with icariin promoted chondrocyte aggregation and secretion of ECM including type II collagen, and further increased mRNA expression of chondrogenic specific genes involving col2α1 and early chondrogenic marker SOX9 (Wang et al., 2018b).
4.6 The possible mechanism
4.6.1 The cell-cell and cell-ECM interactions
Cell-cell and cell-ECM interactions play a significant role in signal transduction pathways that further regulate the proliferation of chondrocytes and chondrogenic differentiation of stem cells. Since SAPHs are formed by weak noncovalent interactions, which allow cells to freely migrate, interact and extend different cellular processes, the nanofiber network of SAPHs promotes cell-cell and cell-matrix interactions (Recha-Sancho et al., 2016). Although some studies showed that SAPs such as RADA16 and KLD-12 had insufficient contribution to articular cartilage regeneration due to the lack of specific motifs in their native sequences and can be defined as “non-instructive” from the point of view of cell receptor recognition/activation, new functionalized SAPHs can be generated by binding some short functional peptides, which had been widely used as targeted ligands to guide drug delivery based on ligand-receptor-specific binding, to SAPs (Fernandez-Muinos et al., 2015; Li et al., 2017; Behrendt et al., 2018; Lu et al., 2018).
The bone marrow homing peptide PFS can home to bone marrow and bind to stem cells (Lu et al., 2018). By introducing the functional motif PFS to the RADA16, a functionalized SAP was prepared, which could stimulate rabbit MSC proliferation, attachment and chondrogenic differentiation during in vitro culture (Lu et al., 2018). Other short peptides including HAV, HAVDI, Arg-Gly-Asp (RGD), SP and LPP also show a similar function (Zheng et al., 2015; Kim et al., 2016; Li et al., 2017; Lv et al., 2020; Mohammed et al., 2021). Therefore, they can be utilized to modify SAPs or design new functionalized SAPs to promote chondrogenesis. At the same time, SP can have an anti-inflammatory role in osteoarthritis by increasing anti-inflammatory factor IL-4 and reducing the levels of pro-inflammatory cytokines such as IL-2 and TNF-α (Kim et al., 2016).
4.6.2 Mechanical properties
Since articular cartilage need serve as the “shock absorber” to protect the joints from mechanical hurt, the resulting mechanical stress is essential in maintaining chondrocyte cell variability and synthesizing cartilaginous matrix (Sun et al., 2016). Previously researchers have described materials with storage modulus (G′) values in the range 10–100 kPa that are known to promote chondrocyte attachment and G′ of 1–25 kPa is ideal for chondrocyte differentiation (Jayawarna et al., 2009; Mohammed et al., 2021). The G’ value of FEFEFKFK SAPH ranging from 10Pa to 35 kPa can be tunable by adjusting SAP concentration and pH and increase with the deposition of ECM inside (Mujeeb et al., 2013; Li et al., 2019). Fmoc-GGHAVDI exhibited the storage modulus of 21 kPa, which is also propitious to the attachment and differentiation of chondrocytes (Mohammed et al., 2021). Therefore, SAPHs may maintain the chondrocyte phenotype and stimulate chondrogenesis of MSCs partly via mechanical capacity.
4.6.3 ECM-like structure
SAPHs is composed of abundant amounts of water, even accounting for 99.6% and have well-defined nanostructures such as nanofibers ranging from 5 to 200 nm that partially resemble physical characteristics of the native ECM, which guides the cells during tissue remodeling and provides a highly defined microenvironment (Miller et al., 2010; Mohammed et al., 2021). In addition, SAPHs closely mimic the porosity with sizes in the range of 100–500 nm and gross structure of the native ECM from cells which enable them to reside and migrate in a microenvironment (Miller et al., 2010; Kim et al., 2016; Zhu et al., 2022). And then SAPHs such as KLD-12 and RADA16 can promote ECM secretion including aggrecan and collagen type II content and stimulate chondrogenesis, resulting in improved cartilage healing as compared with empty controls (Miller et al., 2010; Florine et al., 2015; Zanotto et al., 2021).
5 Conclusion
Articular cartilage often has difficulty in repairing itself after injury due to the lack of blood vessels, nerves, and lymphatic vessels. Although small lesions can be treated with microfracture surgery, medium-sized lesions are treated with ACI, OAT, or OCA; a wide variety of serious defect are addressed with ACI or OCA; and those who are difficult to treat are given joint replacements. However, the above therapies have various defects, so articular cartilage repair cannot achieve a satisfactory therapeutic effect. Therefore, treatments that achieve superior repair are urgently needed. At present, cartilage tissue engineering is developing rapidly. Hydrogels are widely used in tissue engineering because of their similarity to ECM. Among hydrogels, SAPHs are composed of amino acids and possess excellent biological characteristics, adjustable mechanical properties, and injectable ability, and are appropriate for cartilage tissue engineering.
They, SAPHs, play a therapeutic role primarily by maintaining cell morphology and viability, promoting the secretion of a cartilage-like ECM and filling defects in situ. However, because of their limited therapeutic effects, other molecules or compounds are added to function together. For instance, through binding some short functional peptides, which can facilitate simultaneous recruitment of endogenous chondrocytes and BMSCs, to SAPHs to form new functionalized SAPHs, more powerful therapeutic effects can be achieved. Furthermore, cells and cytokines participate in the process of repair when cartilages damage. The composite SAPHs with encapsulated cells or cytokines can enhance therapeutic actions of hydrogels themselves and promote the proliferation and chondrogenic differentiation of surrounding cells. Nevertheless, the weak mechanical properties of hydrogels limit their application in cartilage injury. Therefore, many researchers alter SAPHs via the incorporation of other scaffolds in order to exploit their specific properties to either modify the performance of the hydrogel or add functionality. Indeed, many peptide systems are now readily available in the market, including PuraMatrix (from 3-D Matrix, Japan), PuraStat (from 3-D Matrix, Japan), Sciobio (from Chengdu Sciobio Biotech, PR China) and PeptiGels (from Manchester BIOGEL, United Kingdom), which are approved for use in accelerated wound healing, hemostasis, uterine repair and myocardial infarction repair. Therefore, SAPHs have great potential to be exploited in the treatment of articular cartilage defects by themselves or in combination with other molecules and can be applied to the clinic in the near future after more research.
Author contributions
All authors listed have made a substantial, direct and intellectual contribution to the work, add approved it for publication.
Funding
The present study was funded by the project of Research and Develop Program, West China Hospital of Stomatology Sichuan University (LCYJ 2019-1) and the Science and Technology Project of the Health Planning Committee of Sichuan (21PJ062).
Acknowledgments
We appreciated the help from Department of Oral and Maxillofacial Surgery, West China Hospital of Stomatology, Sichuan University. The present study was funded by the project of Research and Develop Program, West China Hospital of Stomatology Sichuan University (LCYJ 2019-1) and the Science and Technology Project of the Health Planning Committee of Sichuan (21PJ062).
Conflict of interest
The authors declare that the research was conducted in the absence of any commercial or financial relationships that could be construed as a potential conflict of interest.
Publisher’s note
All claims expressed in this article are solely those of the authors and do not necessarily represent those of their affiliated organizations, or those of the publisher, the editors and the reviewers. Any product that may be evaluated in this article, or claim that may be made by its manufacturer, is not guaranteed or endorsed by the publisher.
References
Allen, K. D., Thoma, L. M., and Golightly, Y. M. (2022). Epidemiology of osteoarthritis. Osteoarthr. Cartil. 30, 184–195. doi:10.1016/j.joca.2021.04.020
Alonso-Polo, B., Sobron, F. B., and Rodriguez-Guerrero, J. (2020). Medium-term results of autologous osteochondral transplantation in patellar cartilage injuries. Acta Ortop. Mex. 34, 27–30.
Antons, J., Marascio, M. G. M., Nohava, J., Martin, R., Applegate, L. A., Bourban, P. E., et al. (2018). Zone-dependent mechanical properties of human articular cartilage obtained by indentation measurements. J. Mat. Sci. Mat. Med. 29, 57. doi:10.1007/s10856-018-6066-0
Aurich, M., Koenig, V., and Hofmann, G. (2018). Comminuted intraarticular fractures of the tibial plateau lead to posttraumatic osteoarthritis of the knee: Current treatment review. Asian J. Surg. 41, 99–105. doi:10.1016/j.asjsur.2016.11.011
Azami, M., and Beheshtizadeh, N. (2021). Identification of regeneration-involved growth factors in cartilage engineering procedure promotes its reconstruction. Regen. Med. 16, 719–731. doi:10.2217/rme-2021-0028
Banwell, E. F., Abelardo, E. S., Adams, D. J., Birchall, M. A., Corrigan, A., Donald, A. M., et al. (2009). Rational design and application of responsive alpha-helical peptide hydrogels. Nat. Mat. 8, 596–600. doi:10.1038/NMAT2479
Barco, A., Ingham, E., Fisher, J., Fermor, H., and Davies, R. P. W. (2018). On the design and efficacy assessment of self-assembling peptide-based hydrogel-glycosaminoglycan mixtures for potential repair of early stage cartilage degeneration. J. Pept. Sci. 24, e3114. doi:10.1002/psc.3114
Batailler, C., and Neyret, P. (2018). Trochlear dysplasia: Imaging and treatment options. EFORT Open Rev. 3, 240–247. doi:10.1302/2058-5241.3.170058
Bauza-Mayol, G., Quintela, M., Brozovich, A., Hopson, M., Shaikh, S., Cabrera, F., et al. (2022). Biomimetic scaffolds modulate the posttraumatic inflammatory response in articular cartilage contributing to enhanced neoformation of cartilaginous tissue in vivo. Adv. Healthc. Mat. 11, 2101127. doi:10.1002/adhm.202101127
Becerra, J., Andrades, J. A., Guerado, E., Zamora-Navas, P., Lopez-Puertas, J. M., and Reddi, A. H. (2010). Articular cartilage: Structure and regeneration. Tissue Eng. Part B Rev. 16, 617–627. doi:10.1089/ten.teb.2010.0191
Behrendt, P., Feldheim, M., Preusse-Prange, A., Weitkamp, J. T., Haake, M., Eglin, D., et al. (2018). Chondrogenic potential of IL-10 in mechanically injured cartilage and cellularized collagen ACI grafts. Osteoarthr. Cartil. 26, 264–275. doi:10.1016/j.joca.2017.11.007
Berninger, M. T., Wexel, G., Rummeny, E. J., Imhoff, A. B., Anton, M., Henning, T. D., et al. (2013). Treatment of osteochondral defects in the rabbit's knee joint by implantation of allogeneic mesenchymal stem cells in fibrin clots. J. Vis. Exp. 75, e4423. doi:10.3791/4423
Bian, L., Hou, C., Tous, E., Rai, R., Mauck, R. L., and Burdick, J. A. (2013). The influence of hyaluronic acid hydrogel crosslinking density and macromolecular diffusivity on human MSC chondrogenesis and hypertrophy. Biomaterials 34, 413–421. doi:10.1016/j.biomaterials.2012.09.052
Bian, S. Q., Cai, H. X., Cui, Y. N., He, M. M., Cao, W. X., Chen, X. N., et al. (2017). Temperature and ion dual responsive biphenyl-dipeptide supramolecular hydrogels as extracellular matrix mimic-scaffolds for cell culture applications. J. Mat. Chem. B 5, 3667–3674. doi:10.1039/c7tb00576h
Bielajew, B. J., Hu, J. C., and Athanasiou, K. A. (2021). Methodology to quantify collagen subtypes and crosslinks: Application in minipig cartilages. Cartilage 13, 1742S–1754S. doi:10.1177/19476035211060508
Borrelli, J., Olson, S. A., Godbout, C., Schemitsch, E. H., Stannard, J. P., and Giannoudis, P. V. (2019). Understanding articular cartilage injury and potential treatments. J. Orthop. Trauma 33, S6–S12. doi:10.1097/BOT.0000000000001472
Boussard, G., and Marraud, M. (1985). Beta-Turns in model dipeptides. An infrared quantitative analysis with NMR correlation. J. Am. Chem. Soc. 107, 1825–1828. doi:10.1021/ja00293a004
Brack, A., and Orgel, L. E. (1975). β structures of alternating polypeptides and their possible prebiotic significance. Nature 256, 383–387. doi:10.1038/256383a0
Branco, M. C., Nettesheim, F., Pochan, D. J., Schneider, J. P., and Wagner, N. J. (2009). Fast dynamics of semiflexible chain networks of self-assembled peptides. Biomacromolecules 10, 1374–1380. doi:10.1021/bm801396e
Buckwalter, J. A. (1998). Articular cartilage: Injuries and potential for healing. J. Orthop. Sports Phys. Ther. 28, 192–202. doi:10.2519/jospt.1998.28.4.192
Bussmann, B. M., Reiche, S., Mari-Buye, N., Castells-Sala, C., Meisel, H. J., and Semino, C. E. (2016). Chondrogenic potential of human dermal fibroblasts in a contractile, soft, self-assembling, peptide hydrogel. J. Tissue Eng. Regen. Med. 10, E54–E62. doi:10.1002/term.1766
Cai, H. X., Wang, P. L., Xu, Y., Yao, Y., Liu, J., Li, T., et al. (2020). BMSCs-assisted injectable Col I hydrogel-regenerated cartilage defect by reconstructing superficial and calcified cartilage. Regen. Biomater. 7, 35–45. doi:10.1093/rb/rbz028
Chassenieux, C., and Tsitsilianis, C. (2016). Recent trends in pH/thermo-responsive self-assembling hydrogels: From polyions to peptide-based polymeric gelators. Soft Matter 12, 1344–1359. doi:10.1039/c5sm02710a
Chen, J., and Zou, X. N. (2019). Self-assemble peptide biomaterials and their biomedical applications. Bioact. Mat. 4, 120–131. doi:10.1016/j.bioactmat.2019.01.002
Chung, C., and Burdick, J. A. (2008). Engineering cartilage tissue. Adv. Drug Deliv. Rev. 60, 243–262. doi:10.1016/j.addr.2007.08.027
Clarke, D. E., Parmenter, C. D. J., and Scherman, O. A. (2018). Tunable pentapeptide self-assembled beta-sheet hydrogels. Angew. Chem. Int. Ed. 57, 7709–7713. doi:10.1002/anie.201801001
Clarke, D. E., Pashuck, E. T., Bertazzo, S., Weaver, J. V. M., and Stevens, M. M. (2017). Self-healing, self-assembled β-sheet peptide–poly(γ-glutamic acid) hybrid hydrogels. J. Am. Chem. Soc. 139, 7250–7255. doi:10.1021/jacs.7b00528
Criado-Gonzalez, M., Iqbal, M. H., Carvalho, A., Schmutz, M., Jierry, L., Schaaf, P., et al. (2020). Surface triggered self-assembly of Fmoc-tripeptide as an antibacterial coating. Front. Bioeng. Biotechnol. 8, 938. doi:10.3389/fbioe.2020.00938
Danalache, M., Beutler, K. R., Rolauffs, B., Wolfgart, J. M., Bonnaire, F. C., Fischer, S., et al. (2021). Exploration of changes in spatial chondrocyte organisation in human osteoarthritic cartilage by means of 3D imaging. Sci. Rep. 11, 9783. doi:10.1038/s41598-021-89582-w
Davidson, B., and Fasman, G. D. (1967). The conformational transitions of uncharged poly-L-lysine. Alpha helix-random coil-beta structure. Biochemistry 6, 1616–1629. doi:10.1021/bi00858a008
Dehsorkhi, A., Castelletto, V., Hamley, I. W., Adamcik, J., and Mezzenga, R. (2013). The effect of pH on the self-assembly of a collagen derived peptide amphiphile. Soft Matter 9, 6033–6036. doi:10.1039/c3sm51029h
Demoor, M., Ollitrault, D., Gomez-Leduc, T., Bouyoucef, M., Hervieu, M., Fabre, H., et al. (2014). Cartilage tissue engineering: Molecular control of chondrocyte differentiation for proper cartilage matrix reconstruction. Biochimica Biophysica Acta - General Subj. 1840, 2414–2440. doi:10.1016/j.bbagen.2014.02.030
Deng, C. J., Chang, J., and Wu, C. T. (2019). Bioactive scaffolds for osteochondral regeneration. J. Orthop. Transl. 17, 15–25. doi:10.1016/j.jot.2018.11.006
Deng, E., Shi, W. L., Jiang, Y. F., and Guo, Q. W. (2020). Comparison of autologous osteoperiosteal cylinder and osteochondral graft transplantation in the treatment of large cystic osteochondral lesions of the talus (OLTs): A protocol for a non-inferiority randomised controlled trial. BMJ Open 10, e033850. doi:10.1136/bmjopen-2019-033850
Diaferia, C., Rosa, E., Gallo, E., Smaldone, G., Stornaiuolo, M., Morelli, G., et al. (2021). Self-supporting hydrogels based on Fmoc-derivatized cationic hexapeptides for potential biomedical applications. Biomedicines 9, 678. doi:10.3390/biomedicines9060678
Dou, X. Q., and Feng, C. L. (2017). Amino acids and peptide-based supramolecular hydrogels for three-dimensional cell culture. Adv. Mat. 29, 1604062. doi:10.1002/adma.201604062
Dufour, A., Buffier, M., Vertu-Ciolino, D., Disant, F., Mallein-Gerin, F., and Perrier-Groult, E. (2019). Combination of bioactive factors and IEIK13 self-assembling peptide hydrogel promotes cartilage matrix production by human nasal chondrocytes. J. Biomed. Mat. Res. A 107, 893–903. doi:10.1002/jbm.a.36612
Dufour, A., Lafont, J. E., Buffier, M., Verset, M., Cohendet, A., Contamin, H., et al. (2021). Repair of full-thickness articular cartilage defects using IEIK13 self-assembling peptide hydrogel in a non-human primate model. Sci. Rep. 11, 4560. doi:10.1038/s41598-021-83208-x
Ebihara, G., Sato, M., Yamato, M., Mitani, G., Kutsuna, T., Nagai, T., et al. (2012). Cartilage repair in transplanted scaffold-free chondrocyte sheets using a minipig model. Biomaterials 33, 3846–3851. doi:10.1016/j.biomaterials.2012.01.056
Elhamian, S. M. M., Alizadeh, M., Shokrieh, M. M., and Karimi, A. (2015). A depth dependent transversely isotropic micromechanic model of articular cartilage. J. Mat. Sci. Mat. Med. 26, 111. doi:10.1007/s10856-015-5449-8
Elhamian, S. M. M., Alizadeh, M., Shokrieh, M. M., Karimi, A., and Madani, S. P. (2014). Transversely isotropic micromechanics model to determine effect of collagen fibre angle in mechanical properties of articular cartilage. Mat. Technol. (N. Y. N. Y). 29, 377–383. doi:10.1179/1753555714Y.0000000178
Erickson, I. E., Huang, A. H., Chung, C., Li, R. T., Burdick, J. A., and Mauck, R. L. (2009). Differential maturation and structure-function relationships in mesenchymal stem cell- and chondrocyte-seeded hydrogels. Tissue Eng. Part A 15, 1041–1052. doi:10.1089/ten.tea.2008.0099
Fan, T. T., Yu, X. Y., Shen, B., and Sun, L. M. (2017). Peptide self-assembled nanostructures for drug delivery applications. J. Nanomater. 2017, 1–16. doi:10.1155/2017/4562474
Fernandez-Muinos, T., Recha-Sancho, L., Lopez-Chicon, P., Castells-Sala, C., Mata, A., and Semino, C. E. (2015). Bimolecular based heparin and self-assembling hydrogel for tissue engineering applications. Acta Biomater. 16, 35–48. doi:10.1016/j.actbio.2015.01.008
Fletcher, N. L., Lockett, C. V., and Dexter, A. F. (2011). A pH-responsive coiled-coil peptide hydrogel. Soft Matter 7, 10210–10218. doi:10.1039/c1sm06261a
Florine, E. M., Miller, R. E., Liebesny, P. H., Mroszczyk, K. A., Lee, R. T., Patwari, P., et al. (2015). Delivering heparin-binding insulin-like growth factor 1 with self-assembling peptide hydrogels. Tissue Eng. Part A 21, 637–646. doi:10.1089/ten.tea.2013.0679
Fu, H., Grimsley, G. R., Razvi, A., Scholtz, J. M., and Pace, C. N. (2009). Increasing protein stability by improving beta-turns. Proteins. 77, 491–498. doi:10.1002/prot.22509
Fu, K., Wu, H. G., and Su, Z. Q. (2021). Self-assembling peptide-based hydrogels: Fabrication, properties, and applications. Biotechnol. Adv. 49, 107752. doi:10.1016/j.biotechadv.2021.107752
Gan, D. L., Jiang, Y. A., Hu, Y. L., Wang, X., Wang, Q. G., Wang, K. F., et al. (2022). Mussel-inspired extracellular matrix-mimicking hydrogel scaffold with high cell affinity and immunomodulation ability for growth factor-free cartilage regeneration. J. Orthop. Transl. 33, 120–131. doi:10.1016/j.jot.2022.02.006
Gao, L., McBeath, R., and Chen, C. S. (2010). Stem cell shape regulates a chondrogenic versus myogenic fate through Rac1 and N-cadherin. Stem Cells 28, 564–572. doi:10.1002/stem.308
Gelain, F., Luo, Z. L., Rioult, M., and Zhang, S. G. (2021). Self-assembling peptide scaffolds in the clinic. npj Regen. Med. 6, 9. doi:10.1038/s41536-020-00116-w
Ghandforoushan, P., Hanaee, J., Aghazadeh, Z., Samiei, M., Navali, A. M., Khatibi, A., et al. (2022). Novel nanocomposite scaffold based on gelatin/PLGA-PEG-PLGA hydrogels embedded with TGF-beta 1 for chondrogenic differentiation of human dental pulp stem cells in vitro. Int. J. Biol. Macromol. 201, 270–287. doi:10.1016/j.ijbiomac.2021.12.097
Godbe, J. M., Freeman, R., Lewis, J. A., Sasselli, I. R., Sangji, M. H., and Stupp, S. I. (2021). Hydrogen bonding stiffens peptide amphiphile supramolecular filaments by aza-glycine residues. Acta Biomater. 135, 87–99. doi:10.1016/j.actbio.2021.08.044
Goldring, M. B. (2012). Chondrogenesis, chondrocyte differentiation, and articular cartilage metabolism in health and osteoarthritis. Ther. Adv. Musculoskelet. Dis. 4, 269–285. doi:10.1177/1759720X12448454
Gorronogoitia, I., Urtaza, U., Zubiarrain-Laserna, A., Alonso-Varona, A., and Zaldua, A. M. (2022). A study of the printability of alginate-based bioinks by 3D bioprinting for articular cartilage tissue engineering. Polymers 14, 354. doi:10.3390/polym14020354
Gottardi, R., Hansen, U., Raiteri, R., Loparic, M., Duggelin, M., Mathys, D., et al. (2016). Supramolecular organization of collagen fibrils in healthy and osteoarthritic human knee and hip joint cartilage. PLoS ONE 11, e0163552. doi:10.1371/journal.pone.0163552
Grenier, S., Donnelly, P. E., Gittens, J., and Torzilli, P. A. (2015). Resurfacing damaged articular cartilage to restore compressive properties. J. Biomech. 48, 122–129. doi:10.1016/j.jbiomech.2014.10.023
Guilbaud, J. B., Rochas, C., Miller, A. F., and Saiani, A. (2013). Effect of enzyme concentration of the morphology and properties of enzymatically triggered peptide hydrogels. Biomacromolecules 14, 1403–1411. doi:10.1021/bm4000663
Guo, L., Duan, Q. Q., Wu, G. G., Zhang, B. Y., Huang, L. A., Xue, J. J., et al. (2022). Novel multifunctional delivery system for chondrocytes and articular cartilage based on carbon quantum dots. Sensors Actuators B Chem. 356, 131348. doi:10.1016/j.snb.2021.131348
Ha, C. W., Park, Y. B., Chung, J. Y., and Park, Y. G. (2015). Cartilage repair using composites of human umbilical cord blood-derived mesenchymal stem cells and hyaluronic acid hydrogel in a minipig model. Stem Cells Transl. Med. 4, 1044–1051. doi:10.5966/sctm.2014-0264
Haines, L. A., Rajagopal, K., Ozbas, B., Salick, D. A., Pochan, D. J., and Schneider, J. P. (2005). Light-activated hydrogel formation via the triggered folding and self-assembly of a designed peptide. J. Am. Chem. Soc. 127, 17025–17029. doi:10.1021/ja054719o
Hanie, E. A., Sullins, K. E., Powers, B. E., and Nelson, P. R. (1992). Healing of full-thickness cartilage compared with full-thickness cartilage and subchondral bone defects in the equine 3rd carpal bone. Equine Vet. J. 24, 382–386. doi:10.1111/j.2042-3306.1992.tb02860.x
Hao, Z. W., Li, H. K., Wang, Y., Hu, Y. K., Chen, T. H., Zhang, S. W., et al. (2022). Supramolecular peptide nanofiber hydrogels for bone tissue engineering: From multihierarchical fabrications to comprehensive applications. Adv. Sci. (Weinh). 9, 2103820. doi:10.1002/advs.202103820
He, R. J., Wang, B. C., Cui, M., Xiong, Z. K., Lin, H., Zhao, L., et al. (2018). Link protein n-terminal peptide as a potential stimulating factor for stem cell-based cartilage regeneration. Stem Cells Int. 2018, 1–11. doi:10.1155/2018/3217895
Hirschmueller, A., Baur, H., Braun, S., Kreuz, P. C., Suedkamp, N. P., and Niemeyer, P. (2011). Rehabilitation after autologous chondrocyte implantation for isolated cartilage defects of the knee. Am. J. Sports Med. 39, 2686–2696. doi:10.1177/0363546511404204
Hoelzl, K., Fuersatz, M., Goecerler, H., Schaedl, B., Zigon-Branc, S., Markovic, M., et al. (2022). Gelatin methacryloyl as environment for chondrocytes and cell delivery to superficial cartilage defects. J. Tissue Eng. Regen. Med. 16, 207–222. doi:10.1002/term.3273
Hu, X. G., Zhu, Y. Q., Yu, S., Zhang, H. J., Liu, F., and Yu, L. (2009). Aromatic pi-pi self-stacking of some aromatic amino acids in aqueous solutions. Acta Phys. Chim. Sin. 25, 729–734. doi:10.3866/PKU.WHXB20090413
Hu, Y. H., Wang, Y., Deng, J., Ding, X. Y., Lin, D. Q., Shi, H., et al. (2022). Enzyme-instructed self-assembly of peptide-drug conjugates in tear fluids for ocular drug delivery. J. Control. Release 344, 261–271. doi:10.1016/j.jconrel.2022.03.011
Huang, B., Li, P. X., Chen, M. X., Peng, L. Q., Luo, X. J., Tian, G. Z., et al. (2022). Hydrogel composite scaffolds achieve recruitment and chondrogenesis in cartilage tissue engineering applications. J. Nanobiotechnol. 20, 25. doi:10.1186/s12951-021-01230-7
Huang, C. C., Ravindran, S., Yin, Z., and George, A. (2014). 3-D self-assembling leucine zipper hydrogel with tunable properties for tissue engineering. Biomaterials 35, 5316–5326. doi:10.1016/j.biomaterials.2014.03.035
Ishikawa, S., Iijima, K., Matsukuma, D., Asawa, Y., Hoshi, K., Osawa, S., et al. (2020). Interpenetrating polymer network hydrogels via a one-pot and in situ gelation system based on peptide self-assembly and orthogonal cross-linking for tissue regeneration. Chem. Mat. 32, 2353–2364. doi:10.1021/acs.chemmater.9b04725
Ishikawa, S., Iijima, K., Matsukuma, D., Iijima, M., Osawa, S., and Otsuka, H. (2021). An interpenetrating polymer network hydrogel with biodegradability through controlling self-assembling peptide behavior with hydrolyzable cross-linking networks. Mat. Today Adv. 9, 100131. doi:10.1016/j.mtadv.2021.100131
Jalali, S., Yang, Y., Mahmoudinobar, F., Singh, S. M., Nilsson, B. L., and Dias, C. (2022). Using all-atom simulations in explicit solvent to study aggregation of amphipathic peptides into amyloid-like fibrils. J. Mol. Liq. 347, 118283. doi:10.1016/j.molliq.2021.118283
Jayawarna, V., Richardson, S. M., Hirst, A. R., Hodson, N. W., Saiani, A., Gough, J. E., et al. (2009). Introducing chemical functionality in Fmoc-peptide gels for cell culture. Acta Biomater. 5, 934–943. doi:10.1016/j.actbio.2009.01.006
Jiang, J., Leong, N. L., Mung, J. C., Hidaka, C., and Lu, H. H. (2008). Interaction between zonal populations of articular chondrocytes suppresses chondrocyte mineralization and this process is mediated by PTHrP. Osteoarthr. Cartil. 16, 70–82. doi:10.1016/j.joca.2007.05.014
Jonker, A. M., Löwik, D. W. P. M., and van Hest, J. C. M. (2012). Peptide- and protein-based hydrogels. Chem. Mat. 24, 759–773. doi:10.1021/cm202640w
Kankala, R. K., Lu, F. J., Liu, C. G., Zhang, S. S., Chen, A. Z., and Wang, S. B. (2018). Effect of icariin on engineered 3D-printed porous scaffolds for cartilage repair. Materials 11, 1390. doi:10.3390/ma11081390
Karavasili, C., Komnenou, A., Katsamenis, O. L., Charalampidou, G., Kofidou, E., Andreadis, D., et al. (2017). Self-assembling peptide nanofiber hydrogels for controlled ocular delivery of timolol maleate. Acs Biomater. Sci. Eng. 3, 3386–3394. doi:10.1021/acsbiomaterials.7b00706
Katyal, P., Mahmoudinobar, F., and Montclare, J. K. (2020). Recent trends in peptide and protein-based hydrogels. Curr. Opin. Struct. Biol. 63, 97–105. doi:10.1016/j.sbi.2020.04.007
Kaur, H., Sharma, P., Patel, N., Pal, V. K., and Roy, S. (2020). Accessing highly tunable nanostructured hydrogels in a short ionic complementary peptide sequence via pH trigger. Langmuir 36, 12107–12120. doi:10.1021/acs.langmuir.0c01472
Kim, J. E., Kim, S. H., and Jung, Y. (2015). In situ chondrogenic differentiation of bone marrow stromal cells in bioactive self-assembled peptide gels. J. Biosci. Bioeng. 120, 91–98. doi:10.1016/j.jbiosc.2014.11.012
Kim, S. J., Kim, J. E., Kim, S. H., Kim, S. J., Jeon, S. J., Kim, S. H., et al. (2016). Therapeutic effects of neuropeptide substance P coupled with self-assembled peptide nanofibers on the progression of osteoarthritis in a rat model. Biomaterials 74, 119–130. doi:10.1016/j.biomaterials.2015.09.040
Kisiday, J. D., Colbath, A. C., and Tangtrongsup, S. (2019). Effect of culture duration on chondrogenic preconditioning of equine bone marrow mesenchymal stem cells in self-assembling peptide hydrogel. J. Orthop. Res. 37, 1368–1375. doi:10.1002/jor.24123
Kisiday, J. D., Kopesky, P. W., Evans, C. H., Grodzinsky, A. J., McIlwraith, C. W., and Frisbie, D. D. (2008). Evaluation of adult equine bone marrow- and adipose-derived progenitor cell chondrogenesis in hydrogel cultures. J. Orthop. Res. 26, 322–331. doi:10.1002/jor.20508
Kisiday, J., Jin, M., Kurz, B., Hung, H., Semino, C., Zhang, , S., et al. (2002). Self-assembling peptide hydrogel fosters chondrocyte extracellular matrix production and cell division: Implications for cartilage tissue repair. Proc. Natl. Acad. Sci. U. S. A. 99, 9996–10001. doi:10.1073/pnas.142309999
Kopesky, P. W., Byun, S., Vanderploeg, E. J., Kisiday, J. D., Frisbie, D. D., and Grodzinsky, A. J. (2014). Sustained delivery of bioactive TGF-beta 1 from self-assembling peptide hydrogels induces chondrogenesis of encapsulated bone marrow stromal cells. J. Biomed. Mat. Res. A 102, 1275–1285. doi:10.1002/jbm.a.34789
Kopesky, P. W., Lee, H. Y., Vanderploeg, E. J., Kisiday, J. D., Frisbie, D. D., Plaas, A. H. K., et al. (2010). Adult equine bone marrow stromal cells produce a cartilage-like ECM mechanically superior to animal-matched adult chondrocytes. Matrix Biol. 29, 427–438. doi:10.1016/j.matbio.2010.02.003
Kopesky, P. W., Vanderploeg, E. J., Kisiday, J. D., Frisbie, D. D., Sandy, J. D., and Grodzinsky, A. J. (2011). Controlled delivery of transforming growth factor beta 1 by self-assembling peptide hydrogels induces chondrogenesis of bone marrow stromal cells and modulates smad2/3 signaling. Tissue Eng. Part A 17, 83–92. doi:10.1089/ten.tea.2010.0198
Kornmueller, K., Letofsky-Papst, I., Gradauer, K., Mikl, C., Cacho-Nerin, F., Leypold, M., et al. (2015). Tracking morphologies at the nanoscale: Self-assembly of an amphiphilic designer peptide into a double helix superstructure. Nano Res. 8, 1822–1833. doi:10.1007/s12274-014-0683-9
Koshti, B., Kshtriya, V., Naskar, S., Narode, H., and Gour, N. (2022). Controlled aggregation properties of single amino acids modified with protecting groups. New J. Chem. 46, 4746–4755. doi:10.1039/d1nj05172e
Koutsopoulos, S. (2016). Self-assembling peptide nanofiber hydrogels in tissue engineering and regenerative medicine: Progress, design guidelines, and applications. J. Biomed. Mat. Res. A 104, 1002–1016. doi:10.1002/jbm.a.35638
Kuroki, K., Stoker, A. M., Stannard, J. P., Bozynski, C. C., Cook, C. R., Pfeiffer, F. M., et al. (2017). Biologic joint repair strategies: The mizzou bioJoint story. Toxicol. Pathol. 45, 931–938. doi:10.1177/0192623317735786
Lee, H. J., Yu, C., Chansakul, T., Hwang, N. S., Varghese, S., Yu, S. M., et al. (2008). Enhanced chondrogenesis of mesenchymal stem cells in collagen mimetic peptide-mediated microenvironment. Tissue Eng. Part A 14, 1843–1851. doi:10.1089/ten.tea.2007.0204
Lee, H. Y., Kopesky, P. W., Plaas, A., Sandy, J., Kisiday, J., Frisbie, D., et al. (2010). Adult bone marrow stromal cell-based tissue-engineered aggrecan exhibits ultrastructure and nanomechanical properties superior to native cartilage. Osteoarthr. Cartil. 18, 1477–1486. doi:10.1016/j.joca.2010.07.015
Li, B., Criado-Gonzalez, M., Adam, A., Bizeau, J., Melart, C., Carvalho, A., et al. (2022a). Peptide hydrogels assembled from enzyme-adsorbed mesoporous silica nanostructures for thermoresponsive doxorubicin release. ACS Appl. Nano Mat. 5, 120–125. doi:10.1021/acsanm.1c03959
Li, L., Li, J. Y., Guo, J. M., Zhang, H. K., Zhang, X., Yin, C. Y., et al. (2019). 3D molecularly functionalized cell-free biomimetic scaffolds for osteochondral regeneration. Adv. Funct. Mat. 29, 1807356. doi:10.1002/adfm.201807356
Li, R., Xu, J., Wong, D. S. H., Li, J., Zhao, P., and Bian, L. (2017). Self-assembled N-cadherin mimetic peptide hydrogels promote the chondrogenesis of mesenchymal stem cells through inhibition of canonical Wnt/β-catenin signaling. Biomaterials 145, 33–43. doi:10.1016/j.biomaterials.2017.08.031
Li, X., Bian, S., Zhao, M., Han, X., Liang, J., Wang, K., et al. (2021). Stimuli-responsive biphenyl-tripeptide supramolecular hydrogels as biomimetic extracellular matrix scaffolds for cartilage tissue engineering. Acta Biomater. 131, 128–137. doi:10.1016/j.actbio.2021.07.007
Li, X., Wang, Y., Zhang, Y., Yang, Z., Gao, J., and Shi, Y. (2022b). Enzyme-instructed self-assembly (EISA) assists the self-assembly and hydrogelation of hydrophobic peptides. J. Mat. Chem. B 10, 3242–3247. doi:10.1039/d2tb00182a
Liebesny, P. H., Byun, S., Hung, H. H., Pancoast, J. R., Mroszczyk, K. A., Young, W. T., et al. (2016). Growth Factor-mediated migration of bone marrow progenitor cells for accelerated scaffold recruitment. Tissue Eng. Part A 22, 917–927. doi:10.1089/ten.tea.2015.0524
Ligorio, C., Vijayaraghavan, A., Hoyland, J. A., and Saiani, A. (2022). Acidic and basic self-assembling peptide and peptide-graphene oxide hydrogels: Characterisation and effect on encapsulated nucleus pulposus cells. Acta Biomater. 143, 145–158. doi:10.1016/j.actbio.2022.02.022
Lin, Y. Y., Qiao, Y., Tang, P. F., Li, Z. B., and Huang, J. B. (2011). Controllable self-assembled laminated nanoribbons from dipeptide-amphiphile bearing azobenzene moiety. Soft Matter 7, 2762–2769. doi:10.1039/c0sm01050b
Liu, J., Lu, Y., Xing, F., Liang, J., Wang, Q. G., Fan, Y. J., et al. (2021). Cell-free scaffolds functionalized with bionic cartilage acellular matrix microspheres to enhance the microfracture treatment of articular cartilage defects. J. Mat. Chem. B 9, 1686–1697. doi:10.1039/d0tb02616f
Liu, M., Zeng, X., Ma, C., Yi, H., Ali, Z., Mou, X. B., et al. (2017). Injectable hydrogels for cartilage and bone tissue engineering. Bone Res. 5, 17014. doi:10.1038/boneres.2017.14
Liu, Y. B., Yang, J. R., Luo, Z. C., Li, D. X., Lu, J., Wang, Q. G., et al. (2019). Development of an injectable thiolated icariin functionalized collagen/hyaluronic hydrogel to promote cartilage formation in vitro and in vivo. J. Mat. Chem. B 7, 2845–2854. doi:10.1039/c9tb00211a
Lopez-Silva, T. L., Leach, D. G., Li, I. C., Wang, X., and Hartgerink, J. D. (2019). Self-assembling multidomain peptides: Design and characterization of neutral peptide-based materials with pH and ionic strength independent self-assembly. ACS Biomater. Sci. Eng. 5, 977–985. doi:10.1021/acsbiomaterials.8b01348
Lu, J. J., Shen, X. Z., Sun, X., Yin, H. Y., Yang, S. H., Lu, C. F., et al. (2018). Increased recruitment of endogenous stem cells and chondrogenic differentiation by a composite scaffold containing bone marrow homing peptide for cartilage regeneration. Theranostics 8, 5039–5058. doi:10.7150/thno.26981
Lv, X., Sun, C. X., Hu, B. W., Chen, S. F., Wang, Z., Wu, Q., et al. (2020). Simultaneous recruitment of stem cells and chondrocytes induced by a functionalized self-assembling peptide hydrogel improves endogenous cartilage regeneration. Front. Cell Dev. Biol. 8, 864. doi:10.3389/fcell.2020.00864
Mao, B., Zhang, Z., Lai, S., Zhang, K., Li, J., and Fu, W. (2022). Demineralized cortical bone matrix augmented with peripheral blood-derived mesenchymal stem cells for rabbit medial meniscal reconstruction. Front. Bioeng. Biotechnol. 10, 855103. doi:10.3389/fbioe.2022.855103
Matson, J. B., Newcomb, C. J., Bitton, R., and Stupp, S. I. (2012). Nanostructure-templated control of drug release from peptide amphiphile nanofiber gels. Soft Matter 8, 3586–3595. doi:10.1039/c2sm07420f
Matsugami, D., Murakami, T., Yoshida, W., Imamura, K., Bizenjima, T., Seshima, F., et al. (2021). Treatment with functionalized designer self-assembling peptide hydrogels promotes healing of experimental periodontal defects. J. Periodontal Res. 56, 162–172. doi:10.1111/jre.12807
Matthews, J. R., Brutico, J. M., Abraham, D. T., Heard, J. C., Tucker, B. S., Tjoumakaris, F. P., et al. (2022). Differences in clinical and functional outcomes between osteochondral allograft transplantation and autologous chondrocyte implantation for the treatment of focal articular cartilage defects. Orthop. J. Sports Med. 10, 232596712110584. doi:10.1177/23259671211058425
Medina, J., Garcia-Mansilla, I., Fabricant, P. D., Kremen, T. J., Sherman, S. L., and Jones, K. (2021). Microfracture for the treatment of symptomatic cartilage lesions of the knee: A survey of international cartilage regeneration & joint preservation society. Cartilage 13, 1148S–1155S. doi:10.1177/1947603520954503
Meding, J. B., Anderson, A. R., Faris, P. M., Keating, E. M., and Ritter, M. A. (2000). Is the preoperative radiograph useful in predicting the outcome of a total hip replacement? Clin. Orthop. Relat. Res. 376, 156–160. doi:10.1097/00003086-200007000-00022
Merkely, G., Ackermann, J., and Lattermann, C. (2018). Articular cartilage defects: Incidence, diagnosis, and natural history. Oper. Tech. Sports Med. 26, 156–161. doi:10.1053/j.otsm.2018.06.008
Mijiddorj, B., Shirakata, H., Nakagawa, T., Ueda, K., Yokoyama, Y., and Kawamura, I. (2020). Stereochemical effects on the self-assembly of pyrenylalanine-phenylalanine dipeptide. Bull. Chem. Soc. Jpn. 93, 969–977. doi:10.1246/bcsj.20190376
Milena, F., Stefania, P., Gianluca, G., Monica, D. M., Alessia, O., Katia, V., et al. (2013). Functional tissue engineering in articular cartilage repair: Is there a role for electromagnetic biophysical stimulation? Tissue Eng. Part B Rev. 19, 353–367. doi:10.1089/ten.teb.2012.0501
Miller, R. E., Grodzinsky, A. J., Barrett, M. F., Hung, H. H., Frank, E. H., Werpy, N. M., et al. (2014). Effects of the combination of microfracture and self-assembling peptide filling on the repair of a clinically relevant trochlear defect in an equine model. J. Bone Jt. Surg. 96A, 1601–1609. doi:10.2106/JBJS.M.01408
Miller, R. E., Grodzinsky, A. J., Vanderploeg, E. J., Lee, C., Ferris, D. J., Barrett, M. F., et al. (2010). Effect of self-assembling peptide, chondrogenic factors, and bone marrow-derived stromal cells on osteochondral repair. Osteoarthr. Cartil. 18, 1608–1619. doi:10.1016/j.joca.2010.09.004
Mohammed, M., Lai, T. S., and Lin, H. C. (2021). Substrate stiffness and sequence dependent bioactive peptide hydrogels influence the chondrogenic differentiation of human mesenchymal stem cells. J. Mat. Chem. B 9, 1676–1685. doi:10.1039/d0tb02008g
Mow, V. C., Ratcliffe, A., and Poole, A. R. (1992). Cartilage and diarthrodial joints as paradigms for hierarchical materials and structures. Biomaterials 13, 67–97. doi:10.1016/0142-9612(92)90001-5
Muhammad, S., Youngmee, J., and Hyun, K. S. (2015). Stem cell recruitment, angiogenesis, and tissue regeneration in substance P-conjugated poly(l-lactide-co-ɛ-caprolactone) nonwoven meshes. J. Biomed. Mat. Res. A 103, 2673–2688. doi:10.1002/jbm.a.35400
Muir, H., Bullough, P., and Maroudas, A. (1970). The distribution of collagen in human articular cartilage with some of its physiological implications. J. Bone Jt. Surg. Br. volume 52, 554–563. doi:10.1302/0301-620x.52b3.554
Mujeeb, A., Miller, A. F., Saiani, A., and Gough, J. E. (2013). Self-assembled octapeptide scaffolds for in vitro chondrocyte culture. Acta Biomater. 9, 4609–4617. doi:10.1016/j.actbio.2012.08.044
Nagai, Y., Unsworth, L. D., Koutsopoulos, S., and Zhang, S. (2006). Slow release of molecules in self-assembling peptide nanofiber scaffold. J. Control. Release 115, 18–25. doi:10.1016/j.jconrel.2006.06.031
Nazouri, M., Seifzadeh, A., and Masaeli, E. (2020). Characterization of polyvinyl alcohol hydrogels as tissue-engineered cartilage scaffolds using a coupled finite element-optimization algorithm. J. Biomech. 99, 109525. doi:10.1016/j.jbiomech.2019.109525
Nie, X., Yang, J., Chuah, Y. J., Zhu, W., Peck, Y., He, P., et al. (2020). Full-scale osteochondral regeneration by sole graft of tissue-engineered hyaline cartilage without co-engraftment of subchondral bone substitute. Adv. Healthc. Mat. 9, 1901304. doi:10.1002/adhm.201901304
Niemeyer, P., Hanus, M., Belickas, J., Laszlo, T., Gudas, R., Fiodorovas, M., et al. (2022). Treatment of large cartilage defects in the knee by hydrogel-based autologous chondrocyte implantation: Two-year results of a prospective, multicenter, single-arm phase III trial. Cartilage 13, 194760352210851. doi:10.1177/19476035221085146
Nima, B., Alireza, B. R., Sharifi, S. M., and Mahmoud, A. (2021). An in-silico study on the most effective growth factors in retinal regeneration utilizing tissue engineering concepts. J. Ophthalmic Vis. Res. 16, 56–67. doi:10.18502/jovr.v16i1.8251
O'Leary, L. E. R., Fallas, J. A., Bakota, E. L., Kang, M. K., and Hartgerink, J. D. (2011). Multi-hierarchical self-assembly of a collagen mimetic peptide from triple helix to nanofibre and hydrogel. Nat. Chem. 3, 821–828. doi:10.1038/NCHEM.1123
Okesola, B. O., Ni, S., Derkus, B., Galeano, C. C., Hasan, A., Wu, Y., et al. (2020). Growth-factor free multicomponent nanocomposite hydrogels that stimulate bone formation. Adv. Funct. Mat. 30, 1906205. doi:10.1002/adfm.201906205
Omar, J., Ponsford, D., Dreiss, C. A., Lee, T. C., and Loh, X. J. (2022). Supramolecular hydrogels: Design strategies and contemporary biomedical applications. Chem. Asian J. 17, e202200081. doi:10.1002/asia.202200081
Opolka, A., Straub, R. H., Pasoldt, A., Grifka, J., and Grassel, S. (2012). Substance P and norepinephrine modulate murine chondrocyte proliferation and apoptosis. Arthritis Rheum. 64, 729–739. doi:10.1002/art.33449
Pal, V. K., and Roy, S. (2022). Cooperative metal ion coordination to the short self-assembling peptide promotes hydrogelation and cellular proliferation. Macromol. Biosci. 22, 2100462. doi:10.1002/mabi.202100462
Pan, Q. Y., Li, W. K., Yuan, X. F., Rakhmanov, Y., Wang, P. C., Lu, R., et al. (2016). Chondrogenic effect of cell-based scaffold of self-assembling peptides/PLGA-PLL loading the hTGF beta 3 plasmid DNA. J. Mat. Sci. Mat. Med. 27, 19. doi:10.1007/s10856-015-5631-z
Panasik, N., Fleming, P. J., and Rose, G. D. (2005). Hydrogen-bonded turns in proteins: The case for a recount. Protein Sci. 14, 2910–2914. doi:10.1110/ps.051625305
Panda, J. J., Mishra, A., Basu, A., and Chauhan, V. S. (2008). Stimuli responsive self-assembled hydrogel of a low molecular weight free dipeptide with potential for tunable drug delivery. Biomacromolecules 9, 2244–2250. doi:10.1021/bm800404z
Papapostolou, D., Smith, A. M., Atkins, E. D. T., Oliver, S. J., Ryadnov, M. G., Serpell, L. C., et al. (2007). Engineering nanoscale order into a designed protein fiber. Proc. Natl. Acad. Sci. U. S. A. 104, 10853–10858. doi:10.1073/pnas.0700801104
Parto, B., Mehdi, E. H., and Anousheh, Z. K. (2017). Designing an optimized novel femoral stem. J. Med. Signals Sens. 7, 170–177. doi:10.4103/jmss.jmss_1_17
Pauling, L., Corey, R. B., and Branson, H. R. (1951). The structure of proteins; two hydrogen-bonded helical configurations of the polypeptide chain. Proc. Natl. Acad. Sci. U. S. A. 37, 205–211. doi:10.1073/pnas.37.4.205
Pauling, L., and Corey, R. B. (1951). Configurations of polypeptide chains with favored orientations around single bonds: Two new pleated sheets. Proc. Natl. Acad. Sci. U. S. A. 37, 729–740. doi:10.1073/pnas.37.11.729
Payam, B., Hamed, D., Farhad, M., Forough, A. S., Hossein, B., and Mohamadreza, B. E. (2021). A tough polysaccharide-based cell-laden double-network hydrogel promotes articular cartilage tissue regeneration in rabbits. Chem. Eng. J. 418, 129277. doi:10.1016/j.cej.2021.129277
Ponte, A. L., Marais, E., Gallay, N., Langonne, A., Delorme, B., Herault, O., et al. (2007). The in vitro migration capacity of human bone marrow mesenchymal stem cells: Comparison of chemokine and growth factor chemotactic activities. Stem. Cells 25, 1737–1745. doi:10.1634/stemcells.2007-0054
Prabhath, A., Vernekar, V. N., Esdaille, C. J., Eisenberg, E., Lebaschi, A., Badon, M., et al. (2022). Pegylated insulin-like growth factor-1 biotherapeutic delivery promotes rotator cuff regeneration in a rat model. J. Biomed. Mat. Res. A 110, 1356–1371. doi:10.1002/jbm.a.37378
Qian, Y., Kaur, K., Foster, J. C., and Matson, J. B. (2019). Supramolecular tuning of H2S release from aromatic peptide amphiphile gels: Effect of core unit substituents. Biomacromolecules 20, 1077–1086. doi:10.1021/acs.biomac.8b01732
Rani, A., Kavianini, I., De Leon-Rodriguez, L. M., McGillivray, D. J., Williams, D. E., and Brimble, M. A. (2020). Nanoribbon self-assembly and hydrogel formation from an N-Octanoyl octapeptide derived from the antiparallel beta-Interface of a protein homotetramer. Acta Biomater. 114, 233–243. doi:10.1016/j.actbio.2020.07.023
Recha-Sancho, L., Moutos, F. T., Abella, J., Guilak, F., and Semino, C. E. (2016). Dedifferentiated human articular chondrocytes redifferentiate to a cartilage-like tissue phenotype in a poly(ε-caprolactone)/self-assembling peptide composite scaffold. Materials 9, 472. doi:10.3390/ma9060472
Recha-Sancho, L., and Semino, C. E. (2016a). Chondroitin Sulfate- and Decorin-based self-assembling scaffolds for cartilage tissue engineering. PLoS ONE 11, e0157603. doi:10.1371/journal.pone.0157603
Recha-Sancho, L., and Semino, C. E. (2016b). Heparin-based self-assembling peptide scaffold reestablish chondrogenic phenotype of expanded de-differentiated human chondrocytes. J. Biomed. Mat. Res. A 104, 1694–1706. doi:10.1002/jbm.a.35699
Redondo, M. L., Naveen, N. B., Liu, J. N., Tauro, T. M., Southworth, T. M., and Cole, B. J. (2018). Preservation of knee articular cartilage. Sports. Med. Arthrosc. Rev. 26, e23–e30. doi:10.1097/JSA.0000000000000226
Redondo-Gomez, C., Padilla-Lopategui, S., Azevedo, H. S., and Mata, A. (2020). Host-guest-mediated epitope presentation on self-assembled peptide amphiphile hydrogels. ACS Biomater. Sci. Eng. 6, 4870–4880. doi:10.1021/acsbiomaterials.0c00549
Ren, P., Li, J., Zhao, L., Wang, A., Wang, M., Li, J., et al. (2020). Dipeptide self-assembled hydrogels with shear-thinning and instantaneous self-healing properties determined by peptide sequences. ACS Appl. Mat. Interfaces 12, 21433–21440. doi:10.1021/acsami.0c03038
Ren, X., Zhao, M., Lash, B., Martino, M. M., and Julier, Z. (2019). Growth factor engineering strategies for regenerative medicine applications. Front. Bioeng. Biotechnol. 7, 469. doi:10.3389/fbioe.2019.00469
Rughani, R. V., and Schneider, J. P. (2008). Molecular design of beta-hairpin peptides for material construction. MRS Bull. 33, 530–535. doi:10.1557/mrs2008.106
Runser, J. Y., Criado-Gonzalez, M., Fneich, F., Rabineau, M., Senger, B., Weiss, P., et al. (2022). Non-monotonous enzyme-assisted self-assembly profiles resulting from reaction-diffusion processes in host gels. J. Colloid Interface Sci. 620, 234–241. doi:10.1016/j.jcis.2022.03.150
Ruter, A., Kuczera, S., Gentile, L., and Olsson, U. (2020). Arrested dynamics in a model peptide hydrogel system. Soft Matter 16, 2642–2651. doi:10.1039/c9sm02244a
Ryadnov, M. G., and Woolfson, D. N. (2003). Engineering the morphology of a self-assembling protein fibre. Nat. Mat. 2, 329–332. doi:10.1038/nmat885
Saini, A., and Chauhan, V. S. (2014). Self-assembling properties of peptides derived from TDP-43 C-terminal fragment. Langmuir 30, 3845–3856. doi:10.1021/la404710w
Sarkar, B., Siddiqui, Z., Nguyen, P. K., Dube, N., Fu, W., Park, S., et al. (2019). Membrane-disrupting nanofibrous peptide hydrogels. ACS Biomater. Sci. Eng. 5, 4657–4670. doi:10.1021/acsbiomaterials.9b00967
Servin-Vences, M. R., Richardson, J., Lewin, G. R., and Poole, K. (2018). Mechanoelectrical transduction in chondrocytes. Clin. Exp. Pharmacol. Physiol. 45, 481–488. doi:10.1111/1440-1681.12917
Shen, G. (2005). The role of type X collagen in facilitating and regulating endochondral ossification of articular cartilage. Orthod. Craniofac. Res. 8, 11–17. doi:10.1111/j.1601-6343.2004.00308.x
Shi, S., Wang, C., and Trippel, S. B. (2020). Hyaluronic acid-binding insulin-like growth factor-1: Creation of a gene encoding a bifunctional fusion protein. Mol. Biol. Rep. 47, 9749–9756. doi:10.1007/s11033-020-06034-w
Shi, W., Yang, S., Xiong, S., Xu, M., Pi, Y., Chen, L., et al. (2022). Comparison of autologous osteoperiosteal and osteochondral transplantation for the treatment of large, medial cystic osteochondral lesions of the talus. Am. J. Sports Med. 50, 769–777. doi:10.1177/03635465211068529
Shy, A. N., Kim, B. J., and Xu, B. (2019). Enzymatic noncovalent synthesis of supramolecular soft matter for biomedical applications. Matter 1, 1127–1147. doi:10.1016/j.matt.2019.09.015
Shy, A. N., Wang, H., Feng, Z., and Xu, B. (2021). Heterotypic supramolecular hydrogels formed by noncovalent interactions in inflammasomes. Molecules 26, 77. doi:10.3390/molecules26010077
Solheim, E., Hegna, J., and Inderhaug, E. (2020). Long-term survival after microfracture and mosaicplasty for knee articular cartilage repair: A comparative study between two treatments cohorts. Cartilage 11, 71–76. doi:10.1177/1947603518783482
Sook, H. H., Jungsun, L., EunAh, L., Sam, K. Y., Eunkyung, L., Woosung, A., et al. (2009). A new role of substance P as an injury-inducible messenger for mobilization of CD29(+) stromal-like cells. Nat. Med. 15, 425–435. doi:10.1038/nm.1909
Sophia, F. A. J., Bedi, A., and Rodeo, S. A. (2009). The basic science of articular cartilage: Structure, composition, and function. Sports Health. 1, 461–468. doi:10.1177/1941738109350438
Sreekumaran, S., Radhakrishnan, A., Rauf, A. A., and Kurup, G. M. (2021). Nanohydroxyapatite incorporated photocrosslinked gelatin methacryloyl/poly(ethylene glycol)diacrylate hydrogel for bone tissue engineering. Prog. Biomater. 10, 43–51. doi:10.1007/s40204-021-00150-x
Stefani, R. M., Lee, A. J., Tan, A. R., Halder, S. S., Hu, Y., Guo, X. E., et al. (2020). Sustained low-dose dexamethasone delivery via a PLGA microsphere-embedded agarose implant for enhanced osteochondral repair. Acta Biomater. 102, 326–340. doi:10.1016/j.actbio.2019.11.052
Stockwell, R. A. (1967). The cell density of human articular and costal cartilage. J. Anat. 101, 753–763.
Su, C. A., Trivedi, N. N., Le, H. T., Sivasundaram, L., Maak, T. G., Salata, M. J., et al. (2021). Clinical and radiographic outcomes after treatment of patellar chondral defects: A systematic review. Sports Health. 13, 490–501. doi:10.1177/19417381211003515
Sun, L., Zheng, C., and Webster, T. J. (2017). Self-assembled peptide nanomaterials for biomedical applications: Promises and pitfalls. Int. J. Nanomedicine 12, 73–86. doi:10.2147/IJN.S117501
Sun, W., Xue, B., Li, Y., Qin, M., Wu, J., Lu, K., et al. (2016). Polymer-supramolecular polymer double-network hydrogel. Adv. Funct. Mat. 26, 9044–9052. doi:10.1002/adfm.201603512
Sun, X., Yin, H., Wang, Y., Lu, J., Shen, X., Lu, C., et al. (2018). In situ articular cartilage regeneration through endogenous reparative cell homing using a functional bone marrow-specific scaffolding system. ACS Appl. Mat. Interfaces 10, 38715–38728. doi:10.1021/acsami.8b11687
Sun, Y., Li, X., Zhao, M., Chen, Y., Xu, Y., Wang, K., et al. (2022). Bioinspired supramolecular nanofiber hydrogel through self-assembly of biphenyl-tripeptide for tissue engineering. Bioact. Mat. 8, 396–408. doi:10.1016/j.bioactmat.2021.05.054
Takagi, T., Takahashi, S., Koshino, T., Okamoto, R., and Jasin, H. E. (1998). Isolation and differential secretion of metalloproteinase by superficial chondrocytes in articular cartilage. Int. Orthop. 22, 55–58. doi:10.1007/s002640050208
Tanska, P., Julkunen, P., and Korhonen, R. K. (2018). A computational algorithm to simulate disorganization of collagen network in injured articular cartilage. Biomech. Model. Mechanobiol. 17, 689–699. doi:10.1007/s10237-017-0986-3
Thomas, J., Gupta, N., Joseph, J. P., Chopra, V., Pal, A., and Ghosh, D. (2021). Mechanical integrity in a dynamic interpenetrating hydrogel network of supramolecular peptide-polysaccharide supports enhanced chondrogenesis. ACS Biomater. Sci. Eng. 7, 5798–5809. doi:10.1021/acsbiomaterials.1c01120
Tomic, S. L., Nikodinovic-Runic, J., Vukomanovic, M., Babic, M. M., and Vukovic, J. S. (2021). Novel hydrogel scaffolds based on alginate, gelatin, 2-hydroxyethyl methacrylate, and hydroxyapatite. Polymers 13, 932. doi:10.3390/polym13060932
Tyler, J. A. (1989). Insulin-like growth factor 1 can decrease degradation and promote synthesis of proteoglycan in cartilage exposed to cytokines. Biochem. J. 260, 543–548. doi:10.1042/bj2600543
Wachsmuth, L., Soder, S., Fan, Z., Finger, F., and Aigner, T. (2006). Immunolocalization of matrix proteins in different human cartilage subtypes. Histol. Histopathol. 21, 477–485. doi:10.14670/HH-21.477
Wang, C. F., Li, Z., Zhang, K., and Zhang, C. M. (2022a). Self-assembling peptides with hBMP7 biological activity promote the differentiation of ADSCs into nucleus pulposus-like cells. J. Orthop. Surg. Res. 17, 197. doi:10.1186/s13018-022-03102-8
Wang, C. L., Zheng, Y. Y., Zheng, Y. X., Wu, C., Wang, X. J., Huang, M. H., et al. (2022b). Enzymatic synthesis of peptide nanofibers for self-delivery of indomethacin and tyroservatide in cancer therapy. ACS Biomater. Sci. Eng. 8, 3010–3021. doi:10.1021/acsbiomaterials.2c00574
Wang, M., Wang, J. Q., Zhou, P., Deng, J., Zhao, Y. R., Sun, Y. W., et al. (2018a). Nanoribbons self-assembled from short peptides demonstrate the formation of polar zippers between beta-sheets. Nat. Commun. 9, 5118. doi:10.1038/s41467-018-07583-2
Wang, P. Z., Meng, Q. Q., Wang, W., Zhang, S. H., Xiong, X. F., Qin, S. N., et al. (2020). Icariin inhibits the inflammation through down-regulating NF-kappa B/HIF-2 alpha signal pathways in chondrocytes. Biosci. Rep. 40, BSR20203107. doi:10.1042/BSR20203107
Wang, T., Meng, Q., Lin, L., Yang, L., Zhao, W. J., and Sun, D. Q. (2022c). Self-assembled dehydropeptide nanocarrier as a delivery system for antitumor drug temozolomide. Bioorg. Chem. 124, 105842. doi:10.1016/j.bioorg.2022.105842
Wang, X., Zhang, D., Zhang, F. Q., Jin, L., Shi, D. L., and Hou, Z. Y. (2022d). Effect analysis of iliac bone autografting for Hepple V osteochondral lesions of the talus. J. Orthop. Surg. Res. 17, 33. doi:10.1186/s13018-022-02924-w
Wang, Z. C., Li, K. H., Sun, H. J., Wang, J., Fu, Z. D., and Liu, M. Z. (2018b). Icariin promotes stable chondrogenic differentiation of bone marrow mesenchymal stem cells in self-assembling peptide nanofiber hydrogel scaffolds. Mol. Med. Rep. 17, 8237–8243. doi:10.3892/mmr.2018.8913
Weber, A. E., Locker, P. H., Mayer, E. N., Cvetanovich, G. L., Tilton, A. K., Erickson, B. J., et al. (2018). Clinical outcomes after microfracture of the knee midterm follow-up. Orthop. J. Sports Med. 6, 232596711775357. doi:10.1177/2325967117753572
Whitesides, G. M., and Grzybowski, B. (2002). Self-assembly at all scales. Science 295, 2418–2421. doi:10.1126/science.1070821
Wu, C., Liu, J., Tang, X., Zhai, Z., Xu, K., and Zhong, W. (2019). An enzyme-assisted self-delivery system of lonidamine-peptide conjugates for selectively killing cancer cells. Chem. Commun. 55, 14852–14855. doi:10.1039/c9cc06204a
Wu, J. W., Chen, Q., Deng, C., Xu, B. P., Zhang, Z. Y., Yang, Y., et al. (2020). Exquisite design of injectable hydrogels in cartilage repair. Theranostics 10, 9843–9864. doi:10.7150/thno.46450
Xing, H., Rodger, A., Comer, J., Picco, A. S., Huck-Iriart, C., Ezell, E. L., et al. (2022). Urea-modified self-assembling peptide amphiphiles that form well-defined nanostructures and hydrogels for biomedical applications. ACS Appl. Bio Mat. doi:10.1021/acsabm.2c00158
Xu, Y. J., Wu, X., Wang, S. Y., Yang, C. Z., Li, Y., and Cao, Y. (2019). Hydroxyapatite nanoparticle-crosslinked peptide hydrogels for three-dimensional culture and differentiation of MC3T3-E1 osteoblasts. J. Biomed. Nanotechnol. 15, 2351–2362. doi:10.1166/jbn.2019.2856
Yamada, Y., Patel, N. L., Kalen, J. D., and Schneider, J. P. (2019). Design of a peptide-based electronegative hydrogel for the direct encapsulation, 3D culturing, in vivo syringe-based delivery, and long-term tissue engraftment of cells. ACS Appl. Mat. Interfaces 11, 34688–34697. doi:10.1021/acsami.9b12152
Yan, J. Y., Liu, C. X., Tu, C., Zhang, R. Z., Tang, X. Y., Li, H., et al. (2021). Hydrogel-hydroxyapatite-monomeric collagen type-I scaffold with low-frequency electromagnetic field treatment enhances osteochondral repair in rabbits. Stem Cell Res. Ther. 12, 572. doi:10.1186/s13287-021-02638-6
Yanagawa, H., Nishizawa, M., and Kojima, K. (1984). A possible prebiotic peptide formation from glycinamide and related compounds. Orig. Life 14, 267–272. doi:10.1007/BF00933667
Yang, C. Y., Yu, Y. R., Wang, X. C., Wang, Q., and Shang, L. R. (2021). Cellular fluidic-based vascular networks for tissue engineering. Eng. Regen. 2, 171–174. doi:10.1016/J.ENGREG.2021.09.006
Yang, F., Zhang, Y., Liu, B. Y., Cao, M., Yang, J. H., Tian, F. D., et al. (2020). Basic fibroblast growth factor and agarose gel promote the ability of immune privilege of allogeneic cartilage transplantation in rats. J. Orthop. Transl. 22, 73–80. doi:10.1016/j.jot.2019.07.001
Yang, J. R., Liu, Y. B., He, L., Wang, Q. G., Wang, L., Yuan, T., et al. (2018). Icariin conjugated hyaluronic acid/collagen hydrogel for osteochondral interface restoration. Acta Biomater. 74, 156–167. doi:10.1016/j.actbio.2018.05.005
Yang, K., Li, C. M., Wang, Y. P., and Hao, J. P. (2022). Micro-vibration environment promotes bone marrow mesenchymal stem cells (BMSCs) healing of fracture ends and matrix metalloproteinase-9 (MMP-9) expression. J. Biomater. tissue Eng. 12, 1169–1174. doi:10.1166/jbt.2022.3006
Yao, B. J., Zhou, Z. W., Zhang, M., Leng, X. Y., and Zhao, D. Q. (2022). Comparison of gene expression patterns in articular cartilage and xiphoid cartilage. Biochem. Genet. 60, 676–706. doi:10.1007/s10528-021-10127-x
Ye, W. L., Yang, Z., Cao, F. Y., Li, H., Zhao, T. Y., Zhang, H., et al. (2022). Articular cartilage reconstruction with TGF-β1-simulating self-assembling peptide hydrogel-based composite scaffold. Acta Biomater. 146, 94–106. doi:10.1016/j.actbio.2022.05.012
Ye, Z. Y., Zhang, H. Y., Luo, H. L., Wang, S. K., Zhou, Q. H., Du, X. P., et al. (2008). Temperature and pH effects on biophysical and morphological properties of self-assembling peptide RADA16-I. J. Pept. Sci. 14, 152–162. doi:10.1002/psc.988
Yin, L., Wu, Y. N., Yang, Z., Denslin, V., Ren, X. F., Tee, C. A., et al. (2018). Characterization and application of size-sorted zonal chondrocytes for articular cartilage regeneration. Biomaterials 165, 66–78. doi:10.1016/j.biomaterials.2018.02.050
Yu, C. P., Gerlei, K. Z., Rágyanszki, A., Jensen, S. J. K., Viskolcz, B., and Csizmadia, I. G. (2018). Reactivity of Ala-Gly dipeptide with beta-turn secondary structure. Chem. Phys. Lett. 692, 402–406. doi:10.1016/j.cplett.2017.12.057
Yu, M. R., Lin, S. Z., Ge, R., Xiong, C. Y., Xu, L. L., Zhao, M. T., et al. (2022). Buckwheat self-assembling peptide-based hydrogel: Preparation, characteristics and forming mechanism. Food Hydrocoll. 125, 107378. doi:10.1016/j.foodhyd.2021.107378
Yu, T. T., Wang, H. F., Zhang, Y. F., Wang, X., and Han, B. (2020). The delivery of RNA-interference therapies based on engineered hydrogels for bone tissue regeneration. Front. Bioeng. Biotechnol. 8, 445. doi:10.3389/fbioe.2020.00445
Zanotto, G., Liebesny, P., Barrett, M., Zlotnick, H., Grodzinsky, A., and Frisbie, D. (2019). Trypsin pre-treatment combined with growth factor functionalized self-assembling peptide hydrogel improves cartilage repair in rabbit model. J. Orthop. Res. 37, 2307–2315. doi:10.1002/jor.24414
Zanotto, G. M., Liesbeny, P., Barret, M., Zlotnick, H., Frank, E., Grodzinsky, A. J., et al. (2021). Microfracture augmentation with trypsin pretreatment and growth factor-functionalized self-assembling peptide hydrogel scaffold in an equine model. Am. J. Sports Med. 49, 2498–2508. doi:10.1177/03635465211021798
Zeng, W. N., Zhang, Y., Wang, D., Zeng, Y. P., Yang, H., Li, J., et al. (2021). Intra-articular injection of kartogenin-enhanced bone marrow-derived mesenchymal stem cells in the treatment of knee osteoarthritis in a rat model. Am. J. Sports Med. 49, 2795–2809. doi:10.1177/03635465211023183
Zhang, J. M., Lin, W. J., Yang, L. J., Zhang, A. J., Zhang, Y. M., Liu, J. J., et al. (2022a). Injectable and pH-responsive self-assembled peptide hydrogel for promoted tumor cell uptake and enhanced cancer chemotherapy. Biomater. Sci. 10, 854–862. doi:10.1039/d1bm01788h
Zhang, M., Li, L., An, H., Zhang, P. X., and Liu, P. L. (2021a). Repair of peripheral nerve injury using hydrogels based on self-assembled peptides. Gels 7, 152. doi:10.3390/gels7040152
Zhang, R., Stahr, M. C., and Kennedy, M. A. (2022b). Introduction of a new scheme for classifying beta-turns in protein structures. Proteins. 90, 110–122. doi:10.1002/prot.26190
Zhang, S. (2003). Fabrication of novel biomaterials through molecular self-assembly. Nat. Biotechnol. 21, 1171–1178. doi:10.1038/nbt874
Zhang, S., Holmes, T., Lockshin, C., and Rich, A. (1993). Spontaneous assembly of a self-complementary oligopeptide to form a stable macroscopic membrane. Proc. Natl. Acad. Sci. U. S. A. 90, 3334–3338. doi:10.1073/pnas.90.8.3334
Zhang, W. S., Xi, J. D., Zhang, Y. C., Su, Z. Q., and Wei, G. (2020). Green synthesis and fabrication of an electrochemical and colorimetric sensor based on self-assembled peptide-Au nanofibril architecture. Arab. J. Chem. 13, 1406–1414. doi:10.1016/j.arabjc.2017.11.012
Zhang, X. M., Zhang, W., and Yang, M. B. (2018). Application of hydrogels in cartilage tissue engineering. Curr. Stem Cell Res. Ther. 13, 497–516. doi:10.2174/1574888X12666171017160323
Zhang, X., Yan, Z. H., Guan, G. T., Lu, Z. J., Yan, S. J., Du, A. Z., et al. (2021b). Polyethylene glycol diacrylate scaffold filled with cell-laden methacrylamide gelatin/alginate hydrogels used for cartilage repair. J. Biomater. Appl. 36, 1019–1032. doi:10.1177/08853282211044853
Zheng, D., Dan, Y., Yang, S. H., Liu, G. H., Shao, Z. W., Yang, C., et al. (2015). Controlled chondrogenesis from adipose-derived stem cells by recombinant transforming growth factor-beta 3 fusion protein in peptide scaffolds. Acta Biomater. 11, 191–203. doi:10.1016/j.actbio.2014.09.030
Zhu, H. Y., Liu, Y., Gu, D. X., Rao, Z. K., Li, Y., and Hao, J. Y. (2021). Dual thermoresponsive mPEG-b-poly(O-benzyl-L-threonine acid) hydrogel based on beta-sheet nano-structural disassembly and PEG dehydration. Polymer 226, 123841. doi:10.1016/j.polymer.2021.123841
Zhu, J. J., Yang, S. H., Qi, Y. D., Gong, Z., Zhang, H. T., Liang, K. Y., et al. (2022). Stem cell-homing hydrogel-based miR-29b-5p delivery promotes cartilage regeneration by suppressing senescence in an osteoarthritis rat model. Sci. Adv. 8, eabk0011. doi:10.1126/sciadv.abk0011
Ziadlou, R., Rotman, S., Teuschl, A., Salzer, E., Barbero, A., Martin, I., et al. (2021). Optimization of hyaluronic acid-tyramine/silk-fibroin composite hydrogels for cartilage tissue engineering and delivery of anti-inflammatory and anabolic drugs. Mater. Sci. Eng. C 120, 111701. doi:10.1016/j.msec.2020.111701
Keywords: self-assembling, peptide, hydrogel, treatment, articular cartilage defects
Citation: Wang R, Wang Y, Yang H, Zhao C and Pan J (2022) Research progress of self-assembling peptide hydrogels in repairing cartilage defects. Front. Mater. 9:1022386. doi: 10.3389/fmats.2022.1022386
Received: 18 August 2022; Accepted: 16 September 2022;
Published: 10 October 2022.
Edited by:
Tonghe Zhu, Shanghai University of Engineering Sciences, ChinaReviewed by:
Yun Qian, Shanghai Jiao Tong University, ChinaJingfeng Li, Wuhan University, China
Miryam Criado-Gonzalez, University of the Basque Country, Spain
Copyright © 2022 Wang, Wang, Yang, Zhao and Pan. This is an open-access article distributed under the terms of the Creative Commons Attribution License (CC BY). The use, distribution or reproduction in other forums is permitted, provided the original author(s) and the copyright owner(s) are credited and that the original publication in this journal is cited, in accordance with accepted academic practice. No use, distribution or reproduction is permitted which does not comply with these terms.
*Correspondence: Jian Pan, amlhbnBhbmNuQHNjdS5lZHUuY24=