- 1Shanghai Institute of Space Propulsion, Shanghai, China
- 2Shanghai Engineering Research Center of Space Engine, Shanghai, China
Hydrogen is regarded as a promising solution to fulfill the energy demand of Mars human base in the future. Through in-situ resource utilization (ISRU) on Mars, the composite of metal-organic frameworks (MOFs) and magnesium hydride which demonstrates synergistic effect of physi- and chemisorption has been proposed to be an attractive approach for long-term hydrogen storage. MOFs may act either as scaffolds to confine Mg nanoparticles or as catalysts/precursors to lower the energy barrier for hydrogen dissociation on Mg surfaces. The corresponding mechanisms for faster hydrogenation/dehydrogenation kinetics and lower operation temperature were further discussed and analyzed.
Introduction
In 2020, United Arab Emirates (UAE), China, the United States launched Mars probes successively. Mars has become a major concern in space exploration in the 21st century. Several countries have already seriously put crewed missions to Mars on the agenda. Considering the high cost of launching resources from Earth, in-situ resource utilization (ISRU) has become increasingly important to make these missions economically feasible and sustainable.
Evidence showed that there was water resource on Mars, in the form of water ice in polar regions, subglacial liquid water, or mineral hydrate in lithosphere. In 2015, National Aeronautics and Space Administration (NASA) confirmed evidence that liquid salt water flowed on Mars (NASA, 2015). In 2018, European Space Agency (ESA) announced the detection of a 20 km-wide lake of liquid water underneath solid ice in the Planum Australe region, according to the radar profiles from the Mars Express spacecraft (Orosei et al., 2018). If the extraterrestrial water is efficiently acquired and utilized, sufficient hydrogen will be provided for permanent human base, which is regarded as an ideal fuel with high energy density (142 MJ/kg) and no harmful emission (water as the only combustion product).
However, hydrogen is too small and too active to be steadily stored, so that various hydrogen storage approaches have been developed. Conventional phase conversion processes, such as liquefied storage and cryo-compressed storage, have low volumetric capacity and demand high infrastructure cost (Thornton et al., 2017). The temperature on Mars varies greatly from −133 to 27°C, which is demanding for the thermal control of cryogenic storage system. The ultralow atmospheric pressure on Mars (less than 1% on Earth) may greatly increase the cost of the pressure vessel as well. Therefore, it is essential to find an efficient hydrogen storage solution to meet the requirements of long-term in-space application. Adsorption in solid materials is regarded as the most safe hydrogen storage method, and there are two strategies: associated adsorption and dissociated adsorption of hydrogen (Seayad and Antonelli, 2004).
Light metals can form chemical bonds with dissociated hydrogen atoms, generating stable metal hydrides, e.g. LiH, MgH2, AlH3 (Duan et al., 2015; Wan et al., 2015; Wang et al., 2016; Sadhasivam et al., 2017; Duan et al., 2018). This is a typical dissociated adsorption strategy. Among metal hydrides, MgH2 has been regarded as one of the most promising hydrogen storage carriers owing to its non-toxicity, low cost and excellent reversibility (Grochala and Edwards, 2004). The theoretical hydrogen uptake capacity of Mg/MgH2 matrix is as high as 7.6 wt%. Moreover, abundant Mg content within Martian soil makes it an attractive option for ISRU on Mars (Robinson and Morrison, 2018). According to the chemical compositions of Martian soil detected with the Alpha Particle X-ray Spectrometer on the Opportunity rover (Rieder et al., 2004), MgO has an abundance of 7.2%, mainly in the form of olivine.
However, the slow sorption kinetics and high dehydrogenation thermal stability (ΔH = 75 kJ/mol H2) of MgH2 attributed to the strong Mg-H chemical bond impedes its large-scale application. In order to further enhancing the hydrogen uptake capacity and accelerating hydrogen de/adsorption kinetics of MgH2, various strategies have been applied. For example, multiple catalytic metals, metal oxides, and metal halides have been added into Mg/MgH2 matrix to improve the sorption kinetic performances.
Carbon nanoarchitectures, e.g. activated carbons, graphene, carbon nanotubes (CNTs), and metal-organic frameworks (MOFs), have been regarded as a promising class of associated adsorption hydrogen storage materials due to their good chemical stability, high specific surface area, and low density (Xia et al., 2013). It is favorable that more than 95% of Martian atmosphere is composed of carbon dioxide, which guarantees the easily and sustainably accessible carbon source. In this case, promoting hydrogen storage properties of MgH2 with carbon nanoarchitectures seems to be an attractive solution for the in-space application of hydrogen energy.
The Promotion Effect of Carbon Nanoarchitectures for Hydrogen Storage
In earlier researches (before 2010), porous carbon materials were regarded as a kind of good additives to improve hydrogen storage properties by enhancing the hydrogen diffusion in MgH2-C systems. Graphite was firstly used as anti-sticking agent to enhance the ball milling efficiency (Imamura et al., 2003; Imamura et al., 2005). Interestingly, comparing with noncarbon additives, all the carbon additives showed positive effects on the hydrogen storage properties of Mg, especially on the increment of hydrogenation capacity (Wu et al., 2006a). Whereafter, carbon materials with three-dimensional structures have attracted more attention. For instance, a C60 fullerene molecule is capable of storing up to 58 H atoms remaining a metastable structure, which is equivalent to a storage capacity of 7.5 wt% (Pupysheva et al., 2008). Owing to the strong adsorption ability for hydrogen and the excellent heat transfer performance, CNTs may enhance the hydrogen storage capacity and lower the hydrogen desorption platform temperature of Mg (Lyu et al., 2019).
The corresponding hydrogenation mechanism was reported to compose of three consecutive steps: 1) the dissociation of hydrogen molecules on Mg or alloy surfaces; 2) the diffusion of atomic hydrogen along grain boundaries; 3) the interaction between metal and atomic hydrogen. CNTs played a dominated role in the second step by forming thousands of 10–50 nm grains in the Mg particle (500–1,000 nm) (Yao et al., 2007), which built hydrogen diffusion channels to drive hydrogenation/dehydrogenation reactions towards complete (Wu et al., 2006b). Besides, absorbed carbon component on freshly exposed Mg surfaces inhibits the reform of oxide layers. In the carbon fragments produced from ball milling during preparation, unhybridized π electrons are delocalized and then interact with hydrogen appropriately (Wu and Cheng, 2010).
However, fullerenes or CNTs have an inherent spatial structure, so that their properties can only be adjusted using physical approaches like ball milling. In order to lower the energy barrier for hydrogen dissociation, the addition of metallic catalyst is supposed to be achieved by alloying with Mg, which demands stringent preparation conditions for melting (Xu et al., 2019). Therefore, a class of more flexible and reproducible carbon-based materials with exposed metal sites is in demand. MOFs, which are assembled by various inorganic nodes and organic linkers through coordination bonds, have become a more competitive option for hydrogen storage in the past decade.
Advantages and Limitations of Using Metal-Organic Frameworks for Hydrogen Storage
MOFs and MOF-derived carbon nanoarchitectures have been applied in various fields, e.g. separation (Cui et al., 2019), desalination (Wang et al., 2019b; Xu et al., 2020), electrocatalysis (Zhang et al., 2020), chemical sensing (Khan et al., 2019), pollutant adsorption (Wen et al., 2019), supercapacitor (Xu et al., 2017), CO2 reduction (Xuan et al., 2020), etc. Since hydrogen adsorption in MOFs was innovatively proposed by Yaghi’s group in 2003 (Rosi et al., 2003), extensive researches on MOFs as hydrogen storage materials have been done by functionalization of ligands and modification of textural properties (Langmi et al., 2014). The nanoconfined environments of MOFs provide ideal conditions for hydrogen capture, storage, and release with considerable safety, convenience, and efficiency. Besides, MOFs may also act as precursors to design desired hierarchically porous structures (Guo et al., 2021; Liu et al., 2021). The advantages of using MOFs for hydrogen storage mainly lie in: 1) low density; 2) high specific surface area; 3) tunable porous structure; 4) various exposed metal nodes; 5) reproducible and facile synthesis; 6) controllable chemical functionality; 7) amenability to scale-up.
The interaction between hydrogen and adsorbent surfaces are mainly van der Waals force, the energy of which is as low as 4–8 kJ/mol. Adsorbent pore sizes close to the kinetic diameter of hydrogen molecule (2.89 Å) can promote the interaction with hydrogen, when the potential fields from opposite walls overlap (Perles et al., 2005). Although MOFs are favorable owing to their tunable porosities, high hydrogen storage capacities (up to 4.5–7.5 wt%) are normally achieved at cryogenic temperature (77 K) and high pressure, which is not desirable for practical use (Thomas, 2007; Shet et al., 2021). In order to improve the hydrogen storage capacities of MOFs, several approaches to introduce dissociated adsorption of hydrogen by forming hybrid composites have been come up with.
The hydrogen spillover mechanism has aroused wide interest. Hydrogen molecules are firstly dissociated on the catalyst, and then newly generated hydrogen atoms will more easily migrate to the substrate. The introduction of chemisorption hence enables to store hydrogen at room temperature. The addition of metal dopants, e.g. Pd, Pt, Ni, working as hydrogen spillover receptor can effectively enhance hydrogen storage capacity (Zhou et al., 2015; Guo et al., 2020). This mechanism gives a new perspective on the interaction between MOFs and magnesium for hydrogen storage.
Rowsell and Yaghi (2005) proposed that combining light metals with MOFs was one of the most effective strategies to promote hydrogen adsorption in MOFs. Even substituting metal nodes within MOFs with Mg can enhance hydrogen uptake. According to the theoretical calculation result given by Han et al. (2009), Mg4O(CO2)6H6 cluster showed a stronger H2 binding energy than original Zn4O (CO2)6H6 and Be-substituted one, so that Mg-MOF had the highest hydrogen uptake at low pressure (1 bar).
Metal-Organic Frameworks as Scaffolds to Enhance Hydrogen Storage Capacity of Magnesium
The diffusion path of hydrogen can be prominently shortened when Mg nanoparticles are confined inside a scaffold with fine porosity, which promotes the thermodynamics, kinetics, and reversibility of hydrogen storage (Nielsen et al., 2009; Zhang et al., 2017). The imposed nanostructural geometry of Mg/MgH2 matrix creates a great number of defects, forming specific interactions between Mg-H bonds (Wu, 2008). For hydrogen storage, chemisorption positively correlates to the temperature while physisorption inversely correlates. A balance of high storage capacity and low operation temperature is supposed to be achieved by appropriate combination of associated adsorption and dissociated adsorption. In this way, magnesium showed considerable synergistic effect in the presence of MOFs for hydrogen de/adsorption properties.
Compared with bare Mg, the Mg/ZIF-67 composite lowered dehydrogenation activation energy by 43.2 kJ/mol. When hydrogen adsorbed by Mg/ZIF-67 was completely released at 338°C, only 44% hydrogen within Mg was released. Microstructural characterization revealed the formation of core-shell structure within the Mg/ZIF-67 nanocomposite (Figure 1). The MOF scaffold impeded the growth and agglomeration of Mg/MgH2 nanocrystals as an “aggregation blocker” in the hydrogen de/adsorption processes and ensured outstanding cyclic stability. Therefore, the Mg/ZIF-67 composite showed no degradation in hydrogenation/dehydrogenation capacity even after 100 cycles (Wang et al., 2019a).
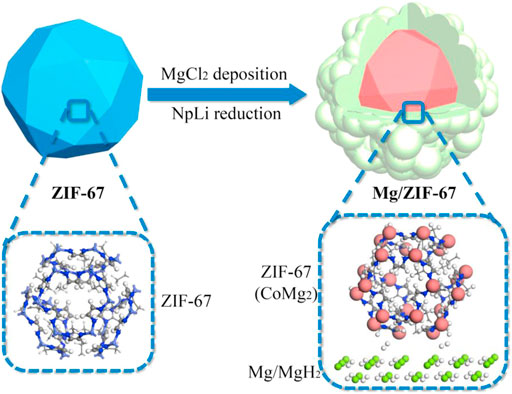
FIGURE 1. Schematic diagram of the core-shell structure within the Mg/ZIF-67 nanocomposite (Wang et al., 2019a).
The research of Lim et al. (2012) showed that Mg embedded MOF (Mg@SNU-90′) had lower desorption temperature and higher hydrogen adsorption isosteric heat, owing to significantly reduced chemisorption temperature of Mg nanocrystals within SNU-90′ comparing with pure Mg powder. The estimated hydrogen uptake capacity of Mg alone within the composite reached 7.5 wt% at 473 K and 30 bar, indicating 99% of the Mg nanocrystals were fully utilized, which was very close to the theoretical limit of 7.6 wt%. Desorption experiments showed that the organic ligands of SNU-90′ were not hydrogenated, indicating that Mg@SNU-90’ demonstrated synergistic behavior of physisorption by MOF and chemisorption by Mg. Jia et al. (2013) found that a fraction of hydrogen was desorbed below 80 °C from MgH2 clusters which were confined to mesoporous carbons, owing to partially transferring electrons from the Mg/MgH2 matrix. Electron transfer capability between Mg and appropriate hosts hence lowers energy barriers for hydrogenation/dehydrogenation processes.
Metal-Organic Frameworks as Catalysts or Precursors to Modify Hydrogen Storage Kinetics of Magnesium
It is reported that the size effect of catalytic active sites plays an important role in preparing Mg-based nanocomposites (De Jongh and Adelhelm, 2010). MOFs not only adsorb hydrogen through their porosities, but also act as excellent catalyst carriers to enhance hydrogenation thermodynamic properties. Owing to the self-assembled inerratic spatial structures, the metal active sites within MOFs may achieve quite high dispersion. In this way, a fraction of transition metals is introduced into the nanocomposites to modify the high energy barrier for hydrogen dissociation on Mg surfaces with no obvious density increasement.
Ma et al. (2019) used trimasic acid (TMA) as organic linker to prepare TMA-TM MOF (TM = Co., Fe). After ball milled with MgH2, the activation energy (Ea) for hydrogenation of the dehydrogenated MgH2-Fe MOF dropped to 66.8 kJ/mol H2, which was much lower than that of pure Mg (100.7 kJ/mol H2). The dehydrogenation activation energy (Ed) of MgH2-Fe MOF composites (142.3 kJ/mol H2) was also much lower than that of pure MgH2 (181.4 kJ/mol H2). MOFs showed considerable catalytic effects in hydrogen storage.
Jia et al. (2015) fabricated ultrafine (2–3 nm) metallic Ni nanoparticles embedded in the crushed MgH2 matrix by in-situ bottom-up reduction of the Ni-MOF-74 precursor. The composite adsorbed 6.2 wt% hydrogen within only 30 s at a temperature as low as 150 C and fully desorbed within just 20 min at 300 C, which exhibited faster kinetics than conventional MgH2. Theoretical calculation showed that dissociated hydrogen was capable of shifting quickly from Ni active sites to pure Mg with an energy increment of only 0.07 eV. Hydrogen diffusion on Ni cluster hence became the rate limiting step, so that limited Ni nanoparticle size significantly promoted hydrogenation/dehydrogenation kinetics.
In the Mg embedded Ni-MOF composite, in-situ formation of Mg2NiH4 around the MgH2 nanocrystals (∼3 nm) was observed (Ma et al., 2020). The “hydrogen pump” effect owing to mutual phase transformation between Mg2Ni and Mg2NiH4 made it easier to release and uptake hydrogen for MgH2 matrix. Huang et al. (2021) further studied the catalytic mechanism of MOF-derived Ni nanoparticles on monolayer Ti3C2 MXene carrier. DFT calculations showed that Mg2Ni (Ti) H4 had lower formation energy than the neat one, so that Mg2Ni/Mg2NiH4 transformation was facilitated by in-situ formed Ti0 from MXene during ball milling, which kept a steady state with no phase or valence change. Comparing with the pristine MgH2, over 120 C decrease in desorption peak temperature and over 60% reduction in dehydrogenation activation energy were achieved owing to the “facilitated hydrogen pump” effect. MOF-promoted hydrogen storage properties of MgH2 have been summarized in Table 1.
Conclusions and Perspectives
The storage and utilization of hydrogen have great potential to meet the energy demand not only on Earth but also on Mars. Promoting hydrogen storage properties of MgH2 with MOFs is regarded as an attractive solution for long-term in-space application of hydrogen energy. The rationality and feasibility to imply this concept on Mars have been demonstrated in this article. Mechanisms and effects of enhanced hydrogen storage when combining associated adsorption with dissociated adsorption have been discussed and analyzed. In particular, the introduction of MOFs as catalysts or precursors during preparation seems to be more promising. On the one hand, it has great potential to simplify the synthesis procedure into one step, which reduces resource consuming resulting from by-products. On the other hand, the in-situ formed matrix of Mg and metal nodes within MOFs is easily designed and modified to achieve lower activation energy. Moreover, the hydrogenation/dehydrogenation kinetics under the condition of weak gravity on Mars are supposed to be further studied to bring this concept into practice.
Author Contributions
YW and QL contributed the conception of the study. ZZ produced and wrote the article. HW and XX edited the article. All authors contributed to the article and approved the submitted version.
Funding
This study was sponsored by Shanghai Sailing Program (21YF1430200).
Conflict of Interest
The authors declare that the research was conducted in the absence of any commercial or financial relationships that could be construed as a potential conflict of interest.
Publisher’s Note
All claims expressed in this article are solely those of the authors and do not necessarily represent those of their affiliated organizations, or those of the publisher, the editors and the reviewers. Any product that may be evaluated in this article, or claim that may be made by its manufacturer, is not guaranteed or endorsed by the publisher.
References
Cui, W. G., Hu, T. L., and Bu, X. H. (2019). Metal-Organic Framework Materials for the Separation and Purification of Light Hydrocarbons. Adv. Mater. 32, 1806445. doi:10.1002/adma.201806445
De Jongh, P. E., and Adelhelm, P. (2010). Nanosizing and Nanoconfinement: New Strategies towards Meeting Hydrogen Storage Goals. ChemSusChem. 3, 1332–1348. doi:10.1002/cssc.201000248
Duan, C. W., Hu, L. X., and Ma, J. L. (2018). Ionic Liquids as an Efficient Medium for the Mechanochemical Synthesis of α-AlH3 Nano-Composites. J. Mater. Chem. A. 6, 6309–6318. doi:10.1039/c8ta00533h
Duan, C. W., Hu, L. X., and Xue, D. (2015). Solid State Synthesis of Nano-Sized AlH3 and its Dehydriding Behaviour. Green. Chem. 17, 3466–3474. doi:10.1039/c5gc00426h
Grochala, W., and Edwards, P. P. (2004). Thermal Decomposition of the Non-interstitial Hydrides for the Storage and Production of Hydrogen. Chem. Rev. 104, 1283–1316. doi:10.1021/cr030691s
Guo, J.-H., Li, S.-J., Su, Y., and Chen, G. (2020). Theoretical Study of Hydrogen Storage by Spillover on Porous Carbon Materials. Int. J. Hydrogen Energ. 45, 25900–25911. doi:10.1016/j.ijhydene.2019.12.146
Guo, J., Xu, X., Hill, J. P., Wang, L., Dang, J., Kang, Y., et al. (2021). Graphene-carbon 2D Heterostructures with Hierarchically-Porous P,N-doped Layered Architecture for Capacitive Deionization. Chem. Sci. 12, 10334–10340. doi:10.1039/d1sc00915j
Han, S. S., Mendoza-Cortés, J. L., and Goddard III, W. A. (2009). Recent Advances on Simulation and Theory of Hydrogen Storage in Metal-Organic Frameworks and Covalent Organic Frameworks. Chem. Soc. Rev. 38, 1460–1476. doi:10.1039/b802430h
Huang, T., Huang, X., Hu, C., Wang, J., Liu, H., Xu, H., et al. (2021). MOF-derived Ni Nanoparticles Dispersed on Monolayer MXene as Catalyst for Improved Hydrogen Storage Kinetics of MgH. Chem. Eng. J. 421, 127851. doi:10.1016/j.cej.2020.127851
Imamura, H., Kusuhara, M., Minami, S., Matsumoto, M., Masanari, K., Sakata, Y., et al. (2003). Carbon Nanocomposites Synthesized by High-Energy Mechanical Milling of Graphite and Magnesium for Hydrogen Storage. Acta Materialia 51, 6407–6414. doi:10.1016/j.actamat.2003.08.010
Imamura, H., Masanari, K., Kusuhara, M., Katsumoto, H., Sumi, T., and Sakata, Y. (2005). High Hydrogen Storage Capacity of Nanosized Magnesium Synthesized by High Energy ball-milling. J. Alloys Compd. 386, 211–216. doi:10.1016/j.jallcom.2004.04.145
Jia, Y., Sun, C., Cheng, L., Abdul Wahab, M., Cui, J., Zou, J., et al. (2013). Destabilization of Mg-H Bonding through Nano-Interfacial Confinement by Unsaturated Carbon for Hydrogen Desorption from MgH2. Phys. Chem. Chem. Phys. 15, 5814–5820. doi:10.1039/c3cp50515d
Jia, Y., Sun, C., Peng, Y., Fang, W., Yan, X., Yang, D., et al. (2015). Metallic Ni Nanocatalyst In Situ Formed from a Metal-Organic-Framework by Mechanochemical Reaction for Hydrogen Storage in Magnesium. J. Mater. Chem. A. 3, 8294–8299. doi:10.1039/c5ta00278h
Khan, M. A., Rao, M. V., and Li, Q. (2019). Recent Advances in Electrochemical Sensors for Detecting Toxic Gases: NO2, SO2 and H2S. Sensors 19 (4), 905. doi:10.3390/s19040905
Langmi, H. W., Ren, J., North, B., Mathe, M., and Bessarabov, D. (2014). Hydrogen Storage in Metal-Organic Frameworks: A Review. Electrochimica Acta 128, 368–392. doi:10.1016/j.electacta.2013.10.190
Lim, D.-W., Yoon, J. W., Ryu, K. Y., and Suh, M. P. (2012). Magnesium Nanocrystals Embedded in a Metal-Organic Framework: Hybrid Hydrogen Storage with Synergistic Effect on Physi- and Chemisorption. Angew. Chem. Int. Ed. 51, 9814–9817. doi:10.1002/anie.201206055
Liu, X., Zhang, S., Feng, G., Wu, Z.-G., Wang, D., Albaqami, M. D., et al. (2021). Core-Shell MOF@COF Motif Hybridization: Selectively Functionalized Precursors for Titanium Dioxide Nanoparticle-Embedded Nitrogen-Rich Carbon Architectures with Superior Capacitive Deionization Performance. Chem. Mater. 33, 1657–1666. doi:10.1021/acs.chemmater.0c04129
Lyu, J., Lider, A., and Kudiiarov, V. (2019). Using Ball Milling for Modification of the Hydrogenation/Dehydrogenation Process in Magnesium-Based Hydrogen Storage Materials: An Overview. Metals 9 (7), 768. doi:10.3390/met9070768
Ma, Z., Zhang, Q., Panda, S., Zhu, W., Sun, F., Khan, D., et al. (2020). In Situ catalyzed and Nanoconfined Magnesium Hydride Nanocrystals in a Ni-MOF Scaffold for Hydrogen Storage. Sustain. Energ. Fuels 4, 4694–4703. doi:10.1039/d0se00818d
Ma, Z., Zou, J., Khan, D., Zhu, W., Hu, C., Zeng, X., et al. (2019). Preparation and Hydrogen Storage Properties of MgH2-Trimesic Acid-TM MOF (TM=Co, Fe) Composites. J. Mater. Sci. Tech. 35, 2132–2143. doi:10.1016/j.jmst.2019.05.049
NASA (2015). NASA Confirms Evidence that Liquid Water Flows on Today’s Mars. Available: https://www.nasa.gov/press-release/nasa-confirms-evidence-that-liquid-water-flows-on-today-s-mars (Accessed September 28, 2015).
Nielsen, T. K., Manickam, K., Hirscher, M., Besenbacher, F., and Jensen, T. R. (2009). Confinement of MgH2 Nanoclusters within Nanoporous Aerogel Scaffold Materials. ACS Nano 3, 3521–3528. doi:10.1021/nn901072w
Orosei, R., Lauro, S. E., Pettinelli, E., Cicchetti, A., Coradini, M., Cosciotti, B., et al. (2018). Radar Evidence of Subglacial Liquid Water on Mars. Science 361, 490–493. doi:10.1126/science.aar7268
Perles, J., Iglesias, M., Martín-Luengo, M.-Á., Monge, M. Á., Ruiz-Valero, C., and Snejko, N. (2005). Metal−Organic Scandium Framework: Useful Material for Hydrogen Storage and Catalysis. Chem. Mater. 17, 5837–5842. doi:10.1021/cm051362e
Pupysheva, O. V., Farajian, A. A., and Yakobson, B. I. (2008). Fullerene Nanocage Capacity for Hydrogen Storage. Nano Lett. 8, 767–774. doi:10.1021/nl071436g
Rieder, R., Gellert, R., Anderson, R. C., Brückner, J., Clark, B. C., Dreibus, G., et al. (2004). Chemistry of Rocks and Soils at Meridiani Planum from the Alpha Particle X-ray Spectrometer. Science 306, 1746–1749. doi:10.1126/science.1104358
Robinson, J. W., and Morrison, C. (2018). “ISRU Propulsion Architectures for Space Travel beyond Earth Orbit,” in 2018 Joint Propulsion Conference, Cincinnati, OH, July 9–11, 2018 (American Institute of Aeronautics and Astronautics). doi:10.2514/6.2018-4705
Rosi, N. L., Eckert, J., Eddaoudi, M., Vodak, D. T., Kim, J., Keeffe, M., et al. (2003). Hydrogen Storage in Microporous Metal-Organic Frameworks. Science 300, 1127–1129. doi:10.1126/science.1083440
Rowsell, J. L. C., and Yaghi, O. M. (2005). Strategies for Hydrogen Storage in Metal-Organic Frameworks. Angew. Chem. Int. Ed. 44, 4670–4679. doi:10.1002/anie.200462786
Sadhasivam, T., Kim, H.-T., Jung, S., Roh, S.-H., Park, J.-H., and Jung, H.-Y. (2017). Dimensional Effects of Nanostructured Mg/MgH 2 for Hydrogen Storage Applications: A Review. Renew. Sustain. Energ. Rev. 72, 523–534. doi:10.1016/j.rser.2017.01.107
Seayad, A. M., and Antonelli, D. M. (2004). Recent Advances in Hydrogen Storage in Metal-Containing Inorganic Nanostructures and Related Materials. Adv. Mater. 16, 765–777. doi:10.1002/adma.200306557
Shet, S. P., Shanmuga Priya, S., Sudhakar, K., and Tahir, M. (2021). A Review on Current Trends in Potential Use of Metal-Organic Framework for Hydrogen Storage. Int. J. Hydrogen Energ. 46, 11782–11803. doi:10.1016/j.ijhydene.2021.01.020
Thomas, K. M. (2007). Hydrogen Adsorption and Storage on Porous Materials☆. Catal. Today 120, 389–398. doi:10.1016/j.cattod.2006.09.015
Thornton, A. W., Simon, C. M., Kim, J., Kwon, O., Deeg, K. S., Konstas, K., et al. (2017). Materials Genome in Action: Identifying the Performance Limits of Physical Hydrogen Storage. Chem. Mater. 29, 2844–2854. doi:10.1021/acs.chemmater.6b04933
Wan, L., Chen, J., Tan, Y., Gu, Q., and Yu, X. (2015). Ammonia Borane Destabilized by Aluminium Hydride: A Mutual Enhancement for Hydrogen Release. Int. J. Hydrogen Energ. 40, 1047–1053. doi:10.1016/j.ijhydene.2014.11.065
Wang, L., Quadir, M. Z., and Aguey-Zinsou, K.-F. (2016). Direct and Reversible Hydrogen Storage of Lithium Hydride (LiH) Nanoconfined in High Surface Area Graphite. Int. J. Hydrogen Energ. 41, 18088–18094. doi:10.1016/j.ijhydene.2016.07.073
Wang, Y., Lan, Z., Huang, X., Liu, H., and Guo, J. (2019a). Study on Catalytic Effect and Mechanism of MOF (MOF = ZIF-8, ZIF-67, MOF-74) on Hydrogen Storage Properties of Magnesium. Int. J. Hydrogen Energ. 44, 28863–28873. doi:10.1016/j.ijhydene.2019.09.110
Wang, Z., Xu, X., Kim, J., Malgras, V., Mo, R., Li, C., et al. (2019b). Nanoarchitectured Metal-Organic Framework/polypyrrole Hybrids for Brackish Water Desalination Using Capacitive Deionization. Mater. Horiz. 6, 1433–1437. doi:10.1039/c9mh00306a
Wen, M., Li, G., Liu, H., Chen, J., An, T., and Yamashita, H. (2019). Metal-organic Framework-Based Nanomaterials for Adsorption and Photocatalytic Degradation of Gaseous Pollutants: Recent Progress and Challenges. Environ. Sci. Nano 6, 1006–1025. doi:10.1039/c8en01167b
Wu, C., and Cheng, H.-M. (2010). Effects of Carbon on Hydrogen Storage Performances of Hydrides. J. Mater. Chem. 20, 5390–5400. doi:10.1039/b926880d
Wu, C. Z., Wang, P., Yao, X., Liu, C., Chen, D. M., Lu, G. Q., et al. (2006a). Effect of Carbon/noncarbon Addition on Hydrogen Storage Behaviors of Magnesium Hydride. J. Alloys Compd. 414, 259–264. doi:10.1016/j.jallcom.2005.07.021
Wu, C. Z., Wang, P., Yao, X., Liu, C., Chen, D. M., Lu, G. Q., et al. (2006b). Hydrogen Storage Properties of MgH2/SWNT Composite Prepared by ball Milling. J. Alloys Compd. 420, 278–282. doi:10.1016/j.jallcom.2005.10.028
Wu, H. (2008). Strategies for the Improvement of the Hydrogen Storage Properties of Metal Hydride Materials. Chemphyschem. 9, 2157–2162. doi:10.1002/cphc.200800498
Xia, Y., Yang, Z., and Zhu, Y. (2013). Porous Carbon-Based Materials for Hydrogen Storage: Advancement and Challenges. J. Mater. Chem. A. 1, 9365–9381. doi:10.1039/c3ta10583k
Xu, C., Lin, H.-J., Wang, Y., Zhang, P., Meng, Y., Zhang, Y., et al. (2019). Catalytic Effect of In Situ Formed Nano-Mg2Ni and Mg2Cu on the Hydrogen Storage Properties of Mg-Y Hydride Composites. J. Alloys Compd. 782, 242–250. doi:10.1016/j.jallcom.2018.12.223
Xu, X., Tang, J., Qian, H., Hou, S., Bando, Y., Hossain, M. S. A., et al. (2017). Three-Dimensional Networked Metal-Organic Frameworks with Conductive Polypyrrole Tubes for Flexible Supercapacitors. ACS Appl. Mater. Inter. 9, 38737–38744. doi:10.1021/acsami.7b09944
Xu, X., Yang, T., Zhang, Q., Xia, W., Ding, Z., Eid, K., et al. (2020). Ultrahigh Capacitive Deionization Performance by 3D Interconnected MOF-Derived Nitrogen-Doped Carbon Tubes. Chem. Eng. J. 390, 124493. doi:10.1016/j.cej.2020.124493
Xuan, X., Chen, S., Zhao, S., Yoon, J. Y., Boczkaj, G., and Sun, X. (2020). Carbon Nanomaterials from Metal-Organic Frameworks: A New Material Horizon for CO2 Reduction. Front. Chem. 8, 573797. doi:10.3389/fchem.2020.573797
Yao, X., Wu, C., Du, A., Zou, J., Zhu, Z., Wang, P., et al. (2007). Metallic and Carbon Nanotube-Catalyzed Coupling of Hydrogenation in Magnesium. J. Am. Chem. Soc. 129, 15650–15654. doi:10.1021/ja0751431
Zhang, J., Zhu, Y., Lin, H., Liu, Y., Zhang, Y., Li, S., et al. (2017). Metal Hydride Nanoparticles with Ultrahigh Structural Stability and Hydrogen Storage Activity Derived from Microencapsulated Nanoconfinement. Adv. Mater. 29, 1700760. doi:10.1002/adma.201700760
Zhang, S., Xia, W., Yang, Q., Valentino Kaneti, Y., Xu, X., Alshehri, S. M., et al. (2020). Core-shell Motif Construction: Highly Graphitic Nitrogen-Doped Porous Carbon Electrocatalysts Using MOF-Derived Carbon@COF Heterostructures as Sacrificial Templates. Chem. Eng. J. 396, 125154. doi:10.1016/j.cej.2020.125154
Keywords: hydrogen storage, metal-organic framework (MOF), magnesium hydride, in-situ resource utilization (ISRU), Mars
Citation: Zhang Z, Wang Y, Wang H, Xue X and Lin Q (2021) Metal-Organic Frameworks Promoted Hydrogen Storage Properties of Magnesium Hydride for In-Situ Resource Utilization (ISRU) on Mars. Front. Mater. 8:766288. doi: 10.3389/fmats.2021.766288
Received: 28 August 2021; Accepted: 28 September 2021;
Published: 21 October 2021.
Edited by:
Xingtao Xu, National Institute for Materials Science, JapanCopyright © 2021 Zhang, Wang, Wang, Xue and Lin. This is an open-access article distributed under the terms of the Creative Commons Attribution License (CC BY). The use, distribution or reproduction in other forums is permitted, provided the original author(s) and the copyright owner(s) are credited and that the original publication in this journal is cited, in accordance with accepted academic practice. No use, distribution or reproduction is permitted which does not comply with these terms.
*Correspondence: Yuanding Wang, d3lkMTk4NzAzMjhAMTYzLmNvbQ==