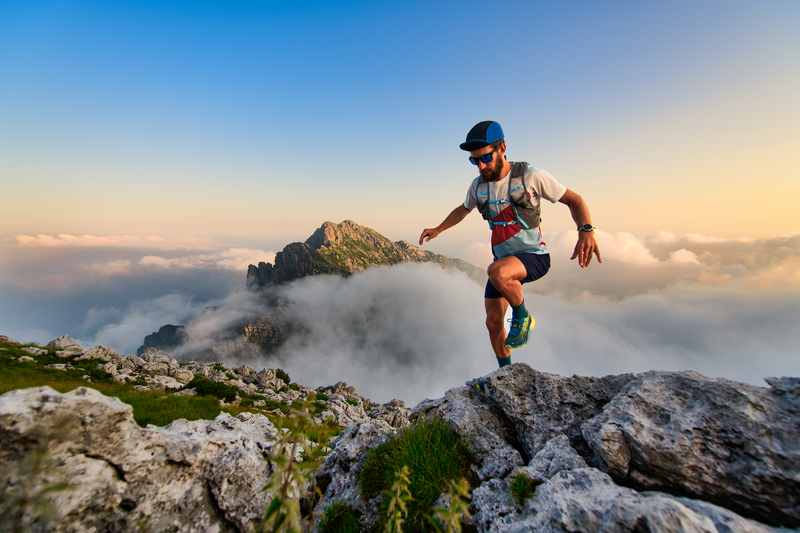
95% of researchers rate our articles as excellent or good
Learn more about the work of our research integrity team to safeguard the quality of each article we publish.
Find out more
ORIGINAL RESEARCH article
Front. Mater. , 12 October 2021
Sec. Biomaterials
Volume 8 - 2021 | https://doi.org/10.3389/fmats.2021.754973
This article is part of the Research Topic Hydrogels Formed from Natural Polysaccharides: Preparation and Biomedical Applications View all 5 articles
Rotator cuff injury causes pain in the shoulder and is a challenge to be repaired even after surgical reconstruction. Here, we developed a dual-factor releasing hydrogel based on sulfhydrylated chitosan to deliver KGN and FGF-2 to the injured area to enable fast healing of the tendon–bone interface, which is essential for the repair of rotator cuff injury. We found that the two factors could be easily loaded into the hydrogel, which could in turn continuously release the factors in physiological conditions. The hydrogel was found to be a porous structure through a scanning electron microscope (SEM). The micropores in the hydrogel structure enable the loading and releasing of these molecules. This study showed that KGN and FGF-2 could play a synergistic effect by recruiting and promoting stem cell proliferation and chondrogenesis, thus accelerating the healing of the tendon–bone interface. An in vivo study based on a rabbit rotator cuff injury model demonstrated that the dual-factor releasing hydrogel possesses superior repair capacity than a single-factor releasing hydrogel and the untreated groups. In conclusion, the KGN and FGF-2 dual-factor releasing hydrogel could be a promising biomaterial for the regeneration of the tendon–bone interface and rotator cuff injury repair.
Rotator cuff injury is the most common pathogeny of pain in the shoulder, with an incidence of about 30% in elderly patients (Atesok et al., 2014). As the shoulder joint is essential for the movement of the upper limb, rotator cuff injury seriously affects the daily work and life of patients. Currently, the most important method for the treatment of rotator cuff injury is surgical reconstruction, however, which is limited by the slow healing process of the tendon–bone interface (Gulotta et al., 2008; Honda et al., 2017; Karjalainen et al., 2019; Schmucker et al., 2020). Generally, after the reconstruction, the mechanical strength of the tendon–bone interface is maintained by the new fiber and cartilage tissue, but these tissues exhibit a disordered structure within 12 weeks after the operation (Rothrauff and Tuan, 2014; Patel et al., 2018; Ozdemir et al., 2021; Zhang et al., 2021). The disordered structure of the neofiber tissue could not supply sufficient mechanical strength so that even common excessive activities may lead to rotator cuff re-tearing (Hao et al., 2016; Patel et al., 2018; Cai et al., 2019). This failure of reconstruction requires prolonged shoulder joint fixation and limited movement, which is not conducive to the recovery of motor function of the shoulder joint (Mazuquin et al., 2018; Longo et al., 2021). Previous studies have indicated that the insufficient mechanical strength in the early stage after a reconstruction could be caused by a lack of cells in the tendon–bone interface (Lu et al., 2021; Zhang et al., 2021). Therefore, increasing the number of stem cells in the tendon–bone interface could be a useful method of promoting fiber regeneration and improving mechanical strength.
Tissue engineering is a promising technique that uses cells, scaffolds, and growth factors to regenerate tissues (Ozdemir et al., 2021; Zhang et al., 2021). The scaffolds could be constructed by the following kinds of sources: synthetic polymers, proteins, and polysaccharides. Among these sources, chitosan is widely investigated for its antibacterial properties and good biocompatibility. Sulfhydrylated chitosan can be obtained by amino modification of chitosan (Kafedjiiski et al., 2005). Compared with ordinary chitosan, sulfhydrylated chitosan has better tissue adhesion, stability, and protein release properties (Matsuda et al., 2005). A recent study showed a chitosan-4-thiobutylamidine (CS-TBA) hydrogel prepared by sulfhydryl chitosan (Liu et al., 2016; Chen et al., 2017) has the following advantages: 1) self-sterilization: reduce the risk of surgical contamination; 2) low cost: can be synthesized in a large scale; 3) injectable: it is a liquid at room temperature, and it can quickly gel under the conditions in vivo (higher temperature); 4) high compatibility: can carry a variety of biological factors and composite materials, and has a wide range of applications (Liu et al., 2016; Chen et al., 2017). Therefore, the CS-TBA hydrogel could be a suitable candidate as the factor carrier for rotator cuff repair.
Kartogenin (KGN) is a small molecular compound extracted by Johnson in 2012 (Johnson et al., 2012). KGN can promote the chondrogenic differentiation of mesenchymal stem cells (MSCs) by binding to its ligand FLNA (Kang et al., 2014; Cai et al., 2019). It can also bind to the core binding factor subunit β (CBF-β), promote the entry of CBF-β into the nucleus, and combine with the DNA transcription factor RUNX1 to form a dimer, thus activating some genes that promote the chondrogenesis of stem cells (Jing et al., 2019). In vitro and in vivo studies have proved that KGN can effectively promote the differentiation of MSCs from various sources into chondrocytes, thus promoting the regeneration of cartilage at the tendon–bone interface (Wang et al., 2018; Chen et al., 2021; Shao et al., 2021; Zhu et al., 2021). Fibroblast growth factor (FGF) is a family of 23 structure-related peptides that play an important role in angiogenesis and mesenchymal cell mitosis. The most abundant fibroblast growth factors in normal adult tissues are FGF-1 and FGF-2. FGF-2 has a stronger mitotic promoting effect (Marquez et al., 2017; Gromolak et al., 2020; Sedlar et al., 2021), and can effectively promote the proliferation of stem cells in the process of tendon repair (Ide et al., 2009; Tokunaga et al., 2015; Guo et al., 2020). Previous reports have proved the effect of FGF-2 on the regeneration of tendons and other soft tissues (Tokunaga et al., 2015; Zhao et al., 2019). Moreover, recent studies have also found that FGF-2 can promote the migration of tendon-derived stem cells and solve the problem of the lack of cells in the tendon–bone interface at the early stage of rotator cuff repair (Zhang et al., 2016; Zhao et al., 2019). Therefore, KGN and FGF-2 could play a synergistic effect by recruiting and promoting stem cell proliferation and chondrogenesis, thus accelerating the tendon–bone healing.
Here, in this study, the CS-TBA hydrogel was fabricated as a carrier to load and continuously release KGN and FGF-2 to the tendon–bone interface. The sustained release of these molecules from the CS-TBA hydrogel could promote healing in the early stage of rotator cuff repair. The morphology of CS-TBA hydrogels was observed by scanning electron microscopy (SEM). The cytotoxicity of the hydrogel was tested in vitro to confirm its safety. Finally, an in vivo study based on a rabbit model was performed to demonstrate the repair function of the KGN/FGF-2–loaded hydrogels. This study shows that the CS-TBA hydrogel with KGN and FGF-2 can effectively promote rotator cuff repair and provide new therapeutic ideas for accelerating tendon–bone healing.
Chitosan was purchased from Macleans (Shanghai), and 2-iminosulfane hydrochloride and β-glycerophosphate sodium were purchased from Sigma-Aldrich (United States). Kartogenin and FGF-2 were purchased from MedChemExpress (United States).
Sulfhydrylated chitosan was prepared according to previously reported methods (Chen et al., 2017). Briefly, 800 mg of chitosan was first dissolved in 400 ml of 1% acetic acid. Then 80 mg of 2-iminosulfide hydrochloride was added to the above solution, and the mixture was stirred for 24 h. The pH value of the solution was adjusted to six using 5 M sodium hydroxide. Then the mixture was dialyzed once with 5 mM HCl, twice with 5 mM HCl containing 1% sodium chloride, and once with 5 mM HCl for 24 h each time. After freeze-drying at −80°C and vacuum for 48 h, sulfhydrylated chitosan was obtained.
To prepare sulfhydryl modified chitosan hydrogels, 200 mg of sulfhydrylated chitosan was added into deionized water (9 ml) and stirred for 1 h to get completely dissolved. Then 200 mg of hydroxyapatite was added into the solution and ultrasonically dispersed. After static placement, KGN and FGF-2 were added to make the final concentration of the two factors at 10 μM and 1.25 μg/ml, respectively. The mixture was stirred for 1 h until it was completely dissolved. Then 560 mg of β-glycerophosphate solution was added dropwise along with stirring. According to the addition of different factors, the hydrogels were recorded as CS-KGN-TBA, CS-FGF-TBA, CS-KGN/FGF-TBA, and CS-TBA. Finally, different types of CS-TBA solutions were placed at 37°C for 10 min to form CS-TBA hydrogels. The schematic of CS-TBA loading and releasing KGN and FGF-2 is shown in Figure 1A.
FIGURE 1. (A) Schematic of dual-factor containing and releasing CS-TBA hydrogel. (B) SEM images of the CS-TBA hydrogel (scale bar: 200 μm). (C) Cytotoxicity of CS-TBA hydrogel at 1, 3, 5, and 7 days. (D) Releasing curve of KGN from the CS-TBA hydrogel. (E) Releasing curve of FGF-2 from the CS-TBA hydrogel.
The CS-KGN/FGF-TBA hydrogel was freeze-dried in a vacuum at −80°C, and then the thin slices of 2 mm in length and 10 mm in width were prepared with a blade. The CS-KGN/FGF-TBA gel slices were dipped on the sample table, and the microstructure was observed by SEM.
CS-KGN/FGF-TBA hydrogels (0.5 ml) were first placed in 15 ml tubes. Then 2 ml of phosphate-buffered saline (PBS) was added, and the tubes were placed in a shaker with a constant temperature at 37°C for uniform oscillation. At predetermined time points, 0.5 ml of the solution was taken out for detection, while 0.5 ml of fresh PBS was added to keep the total amount of the releasing medium unchanged. The content of KGN and FGF-2 was detected through UV-Vis and ELISA, respectively.
All animals were treated according to the guidelines approved by the Zhejiang University Ethics Committee (ZJU20210177). The subcutaneous adipose tissue is placed in a clean bench and washed with PBS (containing 1% penicillin and streptomycin) thrice until there is no obvious muscle and fascia on the fat surface. The fat was cut into a paste with sterile scissors, digested with 0.01% type II collagenase for 30 min, and centrifuged at 1200 g for 5 min. After removing the supernatant, it was resuspended in high glucose DMEM medium (containing 10% FBS and 1% penicillin), inoculated in a culture flask at 5 × 104 cells/cm2, and incubated in a 37°C incubator. P2 and P3 generation cells were taken for subsequent experiments.
First of all, 10 mg of KGN was dissolved in 0.3 ml of dimethyl sulfoxide (DMSO) to prepare a 100-mM KGN stock solution. Before the test, the stock solution was diluted to 250, 500, 750, 1,000, and 1,500 μM with high glucose DMEM medium. Similarly, FGF-2 was prepared as a 100 μg/ml solution with double-distilled water and diluted to 100, 250, 500, and 750 ng/ml with high glucose DMEM medium.
To investigate the cytotoxicity of the material, ADSCs were inoculated into the CS-TBA hydrogel at a rate of 1 × 104/mL. At 48 h of culture, a 10% CCK-8 solution was added and incubated for 4 h, after which the absorbance was measured at 450 nm wavelength. For the cytotoxicity of KGN and FGF-2, ADSCs were inoculated in 96-well plates with 250, 500, 750, 1,000, and 1,500 μM of KGN or 100, 250, 500, and 750 ng/ml of FGF-2, respectively. A normal cell culture medium without KGN and FGF-2 was used as a control group. At 48 h of culture, the CCK-8 assay was used, and the absorbance was measured at a wavelength of 450 nm.
ADSCs were inoculated into CS-TBA hydrogels containing different amounts of FGF-2 at 1 × 104/ml. After culturing for 1, 3, 5, and 7 days, the 10% CCK-8 solution was added and incubated for 4 h, and then the absorbance was detected at 450 nm wavelength.
The chondrogenesis of ADSCs induced by CS-TBA hydrogels containing different concentrations of KGN was characterized by the staining of the extracellular matrix and the detection of gene expression. Firstly, ADSCs were inoculated into CS-TBA hydrogels containing different amounts of KGN at 1 × 104/mL. Alcian blue (AB) staining was applied to detect the chondrogenesis of ADSCs after 21 days of culture. The hydrogel was fixed with 4% paraformaldehyde for 10 min, then the AB acidification solution was added for 3 min, and the AB staining solution was added for 30 min. After washing with PBS thrice, AB-positive cells were observed under an optical microscope. Besides, after 21 days of culture, real-time PCR (RT-PCR) was used to detect the gene expression of ADSCs. Cell total RNA was lysed with TRIzol (Invitrogen Inc., Carlsad, CA, United State). PCR was performed using a transcript cDNA Synth Kit and FastStart Universal SYBR Green Master (Roche). PCR amplification included 40 cycles of template DNA with primer annealing at 60°C. Then the 2−ΔΔCt method was used to calculate the relative expression of each target gene. The amplification efficiency of the primer pair is verified, and the quantitative comparison of gene expression becomes possible. All primer sequences were designed using Primer 5.0 software (Invitrogen Inc., Carlsad, CA, United State). Each real-time polymerase chain reaction is performed on at least three different experimental samples, and the representative results are shown as a target gene expression normalized to the reference gene β-actin. The error bar reflects one standard deviation of the technical repeat average.
Adult male New Zealand rabbits were anesthetized by inhalation with sevoflurane and were fixed on the operating table in a lateral position. The right forelimb was sterilized and skin prepared, a 2-cm median incision was made at the greater tuberosity of the humerus, and the subcutaneous tissue was bluntly separated until the biceps brachii muscle was exposed. The biceps brachii muscle was retracted to reveal the infraspinatus tendon stop point. A blade was used to transversely break off the tendon stop point, and the remaining tendon tissue on the greater tubercle was cleaned. A bone drill was used to grind out a bone groove (8 mm in length, 2 mm in width, and 2 mm in depth). CS-KGN-TBA, CS-FGF-TBA, CS-KGN/FGF-TBA, and CS-TBA hydrogels were prepared as described above. The final concentration of KGN and FGF-2 was made into 10 μM and 1.25 μg/ml, respectively. Then each kind of hydrogel (0.5 ml) was implanted into the bone groove. Holes were drilled with a diameter of 0.8 mm from the outside of the humerus to the bone groove, and the suture was passed through the drilled holes to fix the broken subspinatus muscle above the bone groove. The incision is sutured layer by layer.
Gentamicin (800,000 units) was intramuscularly injected every day for 1 week after surgery to prevent infection of the surgical site, and drug analgesia was given after surgery if necessary. Strenuous activities were limited within 2 weeks after surgery, and normal activities were resumed after 2 weeks. At 4 weeks and 8 weeks after operation, six animals in each group were sacrificed, and the intact supraspinatus muscle and the humerus were removed.
The tendon at the insertion site of the infraspinatus muscle and the attached part of the humerus was collected and fixed with paraformaldehyde. After being decalcified, the samples were made into paraffin sections. Hematoxylin and eosin (H&E), AB, and periodic acid–Schiff (PAS) were used to stain the sections. Immunohistochemical (IHC) staining for type I collagen was also performed according to a standard protocol. The stained sections were observed under a microscope, and the level of cartilage formation and the shape of collagen fibers of the samples were assessed.
Numerical data are presented as the mean ± standard deviation and were tested for normality. All statistical analyses were performed with SPSS software (version 22.0). Statistical significance was calculated by one-way ANOVA with Bonferroni’s post hoc test. p value of <0.05 was considered significant. *p < 0.05, **p < 0.01, and ***p < 0.001.
The chemical feature of CS-TBA hydrogel was characterized by FTIR (Supplementary Figure S1A). The broad adsorption at 3,410 cm−1 corresponded to the O–H stretching. The adsorption at 2,935 cm−1 corresponded to the C–H stretching vibration. The weak adsorption at ∼2,536 cm−1 attributed to thiol groups. The characteristic absorption appeared at 1,650 cm−1 attributed to the C–O stretching of the amide bond. The adsorption at 1,537 cm−1 is attributed to the N–H blending vibration of amidines. The adsorption at 1,116 cm−1 can be assigned to C–O–C stretching vibration in the glucopyranose ring, while the adsorption at 907 cm−1 can be assigned to the β (1–4) glycoside bridge structure. The TGA curve of the CS-TBA hydrogel showed a fast moisture loss and a slow backbone decomposition (Supplementary Figure S1B). The flipped upside down test of CS-TBA hydrogel demonstrated the sol of CS-TBA was able to gelate after 10 min at 37°C (Supplementary Figure S1C).
In order to clarify the delivery capacity of CS-TBA hydrogel for KGN and FGF-2, we investigated the morphology of the CS-TBA hydrogel. From SEM images, a regular distribution of pores can be observed on the surface of CS-TBA hydrogel (Figure 1B). The existence of these micropores is reasonable to the delivery (storage and release) of KGN and FGF-2, and, on the other hand, is also conducive to the migration and colonization of stem cells in the hydrogel. Besides, cytotoxicity tests showed the CS-TBA hydrogel was not cytotoxic to ADSCs and had no negative effect on the proliferation of ADSCs (Figure 1C).
The release of KGN and FGF-2 from the CS-TBA hydrogel was measured in PBS, and the results are shown in Figures 1D, E. The release rate of KGN consists of a burst release (about 27%) on day 1 and a sustained release in the following days. On the other side, the daily release of FGF-2 also shows a smooth release curve that shows a slow release at early days and fast release in the following. The sustained release of these two factors could be resulted from the diffusion of drug molecules and the surface erosion of the hydrogel, and might continuously affect the process of tendon–bone healing.
We intend to use KGN to promote chondrogenic differentiation of stem cells at the tendon–bone interface, so the cytotoxicity and the effect of chondrogenic induction of KGN were assessed in vitro. From the results of the CCK-8 assay, it was found that KGN had no significant cytotoxicity to ADSCs when the concentration was in the range of 250–1,000 μM, while 1,500 μM of KGN significantly inhibited the activity of ADSCs (Figure 2A).
FIGURE 2. (A) CCK-8 assay of ADSCs cultured with cell culture medium containing different amounts of KGN. (B) AB staining of ADSCs after chondrogenic differentiation for 21 days induced by CS-TBA and different concentrations of KGN (scale bar: 20 μm). (C–E) The expression levels of chondrogenesis-related genes such as ACAN (C), COL2A (D), and SOX9 (E) after chondrogenic differentiation induced by different concentrations of KGN. The statistic comparison was made between a control group (0 μM) and each KGN group (250 μM, 500 μM, 750 μM, and 1,000 μM). p value of <0.05 was considered significant. *p < 0.05 and ***p < 0.001.
To understand the effect of KGN on the chondrogenic differentiation of ADSCs, AB staining and RT-PCR for chondrogenic genes were performed on ADSCs after 21 days of KGN induction. AB staining (Figure 2B) showed that a larger range of AB nodules could be observed microscopically at a KGN concentration of 1,000 μM, indicating a higher degree of chondrogenesis. At a concentration of 250 μM, only a small number of nodules were observed, but with the increase of KGN concentration, the area and number of nodules increased gradually. This suggests that KGN promotes chondrogenic differentiation of ADSCs in a concentration-dependent manner. On the other side, the expression levels of chondrogenic genes such as ACAN, SOX9, and COL2A were detected by RT-PCR, and the results are exhibited in Figures 2C–E. As can be seen from the figures, the expression levels of ACAN, SOX9, and COL2A gradually increased with the increase of KGN concentration, consistent with the results of AB staining. The expression level of ACAN, SOX9, and COL2A reached the highest when the concentration of KGN was 1,000 μM.
In this study, FGF-2 was used to promote the cell proliferation that would further improve the repair of the tendon–bone interface. ADSCs were used to investigate the cytotoxicity and functions of FGF-2. By the CCK-8 assay, we found that FGF-2 had no significant toxicity to ADSCs when the concentration was up to 750 ng/ml (Figure 3A). Besides, it was found that the proliferation of ADSCs could be enhanced by the addition of FGF-2 (Figure 3B). However, the cell proliferation of ADSCs was independent from the concentration of FGF-2 such that a higher amount of FGF-2 would not lead to a higher rate of cell proliferation. Besides, FGF-2 was found to have less effect on the chondrogenesis of ADSCs (Supplementary Figure S2).
FIGURE 3. (A) Cytotoxicity of FGF-2 to ADSCs. (B) Cell proliferation of ADSCs in culture medium with different concentrations of FGF-2 (1, 3, 5, and 7 days). The statistic comparison was made between a control group (0 ng/ml) and each FGF-2 group (100 ng/ml, 250 ng/ml, 500 ng/ml, and 750 ng/ml). p value of <0.05 was considered significant.
In order to verify the effect of CS-KGN/FGF-TBA hydrogel on the tendon–bone interface healing in vivo, we made rotator cuff injury models of New Zealand rabbits and implanted the hydrogels in the rotator cuff injury (Figure 4). After 4 and 8 weeks, the animals were sacrificed, and the rotator cuffs were collected for H&E, AB, PAS staining, and immunohistochemical staining for type I collagen (COL I). The staining results are shown in Figure 5. After 4 weeks, more fibrocartilage and mucopolysaccharide deposits were observed at the tendon–bone interface in the CS-KGN/FGF-TBA gel group. Less fibrocartilage and mucopolysaccharide were found in the CS-FGF-TBA gel group and the CS-KGN-TBA gel group. This suggests that the combination of KGN and FGF-2 accelerates the formation of fibrocartilage in the early stage of tendon–bone healing. Eight weeks post-surgery, H&E staining results showed that the structure of the tendon–bone interface in the CS-KGN/FGF-TBA hydrogel-treated group was close to that of a normal rotator cuff. Moreover, AB and PAS staining also showed more fibrocartilage in this group. Although fibrocartilage could be seen in the CS-FGF-TBA gel group and the CS-KGN-TBA gel group, the structure of the tendon–bone interface was relatively disordered. Furthermore, the immunohistochemical staining for type I collagen showed that more type I collagen was produced in the CS-KGN/FGF-TBA hydrogel-treated group, indicating more collagenous fiber that enhanced the healing of the tendon–bone interface could be formed. In addition, quantitative PCR results also support the histological staining outcomes of these sections (Supplementary Figure S3). These results suggest that the combined application of KGN and FGF-2 can promote the remodeling of rotator cuff tissue in the later stage of tendon–bone healing.
FIGURE 4. (A) Bone groove (8 mm in length, 2 mm in width, and 2 mm in depth) was made at the tendon stop point. (B) The hydrogels were implanted into the bone groove. (C) Holes for a suture pass were drilled with a diameter of 0.8 mm from the outside of the humerus to the bone groove. (D) Absorbable suture was used to fix the broken subspinatus muscle above the bone groove. (E) The bursal surface of rotator cuff after the repair of CS-KGN/FGF-TBA for 8 weeks. (F) The articular surface of rotator cuff after the repair of CS-KGN/FGF-TBA for 8 weeks.
FIGURE 5. H&E, AB, PAS staining, and type I collagen (COL I) immunohistochemical staining of the healing point of the tendon–bone (red arrows point to the tendon–bone interfaces; (scale bar: black-500 μm (H&E, AB, and AB + PAS), blue-200 μm (COL I)).
Accelerating the healing of the tendon–bone interface is a challenge after rotator cuff injury. Here, in this study, we used the CS-TBA hydrogel dual-delivering KGN and FGF-2 to effectively heal the tendon–bone interface. This strategy could be recognized as a modified tissue engineering technique that is promising for clinical translation (Zhang et al., 2021).
The normal tendon–bone interface consists of four layers of tissue, which are the tendon, fibrous tissue, fibrocartilage, and bone tissue in turn (Atesok et al., 2014; Patel et al., 2018; Lei et al., 2021). The arrangement of the four layers of tissue can disperse the stress of tendon and bone tissue, thus enhancing the strength of the tendon–bone interface. Fibrocartilage is an important structure to maintain mechanical stability of the tendon–bone interface at the early stage of tendon–bone healing (Atesok et al., 2014; Patel et al., 2018; Lei et al., 2021). Accelerating the regeneration of fibrocartilage is important to promote rotator cuff healing (Zhu et al., 2021). As a synthetic compound, KGN has been shown to promote cartilage tissue regeneration in vitro and in vivo (Hong et al., 2020; Chen et al., 2021; Hou et al., 2021; Zhu et al., 2021). Johnson et al. used KGN to repair articular cartilage defects in mice and found that KGN could induce cartilage formation through the CBFβ-RUNX1 pathway (Johnson et al., 2012). Xu et al. found that the injection of KGN into the joint cavity of animals could effectively repair the full thickness damage of articular cartilage (Xu et al., 2015). At present, some studies have applied it to repair the damaged tendon and ligament tissue and achieved satisfactory results. Therefore, in this study, we aimed to use the chondrogenic effect of KGN to promote the differentiation of stem cells in the tendon–bone interface to form the fibrocartilage that accelerates the healing process. In vitro studies have shown that KGN at concentrations below 1,000 μM can induce chondrogenic differentiation without affecting cell activity (Figure 2). Coculturing with KGN significantly increased the expression levels of chondrogenesis-related genes such as ACAN, SOX9, and COL-2A (Figures 2C–E). However, it was also observed that when the concentration was greater than 1,000 nM, KGN could cause extensive apoptosis of ADSCs, suggesting that an excessive dose of KGN may have a negative effect on the cells (Figure 2A). Although previous studies believed that the systemic toxicity of KGN below the concentration of 100 μM was negligible and the proportion of KGN absorbed into the blood in the articular cavity was very small, there is a lack of studies on the systemic safety of the long-term application of KGN (Johnson et al., 2012; Zhang et al., 2017). Moreover, a high concentration of KGN would inhibit the activity of tendon–bone interface cells, which in turn would affect the regeneration fibrocartilage (Zhang and Wang, 2014; Zhou et al., 2017). In order to reduce the potential harm, this study used 1,000 μM of KGN, which achieved the best induction effect while avoiding the activity of stem cells, as the optimal concentration for the repair of rotator cuff injury. This concentration was the same as that used in other studies (Huang et al., 2017).
In the early stage of tendon–bone healing, the number of stem cells at the tendon–bone interface was maintained at a low level under the action of tissue necrosis and ischemia, which leads to slow healing of the rotator cuff. Moreover, the reduction of stem cells inhibits the regeneration of fibrocartilage and reduces the mechanical strength of the tendon after rotator cuff surgery (Patel et al., 2018; Zhang et al., 2021). By replenishing the number of stem cells at the tendon–bone interface, the healing process could be accelerated (Mifune et al., 2013; Hao et al., 2016; Lu et al., 2021). In this study, FGF-2 was used to promote stem cell proliferation in order to increase the number of stem cells at the tendon–bone interface in the early postoperative period. In vitro results showed that FGF-2 could increase the proliferation rate of ADSCs at an early stage (Figure 3B), but this effect was not enhanced with the increase of FGF-2 concentration. Therefore, the two factors (KGN and FGF-2) have complementary effects, which can promote the proliferation of stem cells at the tendon–bone interface and enhance the chondrogenic differentiation to accelerate the regeneration of fibrocartilage.
In the absence of vectors, direct injection of KGN and FGF-2 in the articular cavity would lead to rapid metabolism and reduce the duration of onset. For this reason, the CS-TBA hydrogel was used as the carrier of KGN and FGF-2. The CS-TBA hydrogel has the advantages of high biocompatibility, degradability, and stable factor release properties. In vitro release experiments showed that the CS-TBA hydrogel could maintain a stable release rate of KGN and FGF-2 (Figure 1). The sustained release property of the CS-TBA hydrogel can avoid excessive fluctuation of drug concentration at the tendon–bone interface and prolong the actual action of the drug. Compared with factor vectors such as PRP, which could release only for 1 week, the CS-TBA hydrogel could release factors over the whole early healing period (Chen et al., 2017). Therefore, after implantation of CS-KGN/FGF-TBA hydrogel, stable factor concentration can be maintained at the tendon–bone interface without regular administration. The degradability of CS-TBA hydrogel can reduce the effect on tissue reconstruction. No gel material was observed at the tendon–bone interface in pathological specimens, indicating that the CS-TBA hydrogel had been catabolized after tendon–bone regeneration and would not affect subsequent structural reconstruction.
The chondrogenic effect of KGN not only promotes the regeneration of lesion cartilage but also has the risk of inducing chondrogenic differentiation from normal tissue. Zhang et al. found that the injection of KGN for the treatment of anterior cruciate ligament injury would induce abnormal cartilage of the patellar ligament and affect the normal joint structure (Zhang and Wang, 2014). Therefore, in in vivo experiments, bone grooves (8 mm long, 2 mm wide, and 2 mm deep) were made inside the rotator cuff insertion point for filling CS-KGN/FGF-TBA hydrogel and covering the rotator cuff above the bone grooves. This mode of administration allows the CS-KGN/FGF-TBA hydrogel to act directly on the tendon–bone interface while reducing the impact on other structures in the joint cavity. In the pathological results, no abnormal differentiated cartilage tissue was observed in the tissues around the ligament or in the articular cavity, which indicated that the factors in the CS-TBA hydrogel were accurately released at the location of the lesion, avoiding the influence on normal tissues.
An in vivo study was performed to demonstrate the rotator cuff repair capacity of the CS-KGN/FGF-TBA hydrogel. At 4 weeks postoperatively, more fibrocartilage regeneration was observed in the CS-KGN/FGF-TBA hydrogel group, significantly more than that in the blank control group (Figure 5). However, in the single-factor group, CS-KGN-TBA gel, and CS-FGF-TBA gel, only a small amount of fibrocartilage could be seen at the tendon–bone interface. This suggests that the combination of KGN and FGF-2 accelerates tendon–bone healing. In the absence of FGF-2, fewer stem cells at the tendon–bone interface were induced to chondrogenic differentiation by KGN, leading to a slower regeneration of fibrocartilage. In the absence of KGN, FGF-2 can increase the number of stem cells, but the degree of chondrogenic differentiation of cells is low, which also slows down the regeneration of fibrocartilage. Therefore, the combination of these two factors can accelerate the early regeneration of fibrocartilage after rotator cuff surgery. At 8 weeks postoperatively, the CS-KGN/FGF-TBA hydrogel group had a normal rotator cuff structure with a large amount of fibrocartilage tissue. Fibrocartilage regeneration was observed in the unifactor group, but the tendon structure was disorganized. These results further demonstrate the promoting effect of CS-KGN/FGF-TBA hydrogel on tendon–bone healing.
The CS-KGN/FGF-TBA hydrogel showed a significantly higher repair effect of the rotator cuff, but there were still several shortcomings in this study. For example, there was no in-depth study on the interaction between KGN and FGF-2. KGN and FGF-2 showed a good synergistic effect in experiments, but the interaction mechanism between the two factors was still unclear. Previous studies also found that Col-I and Col-II levels in the tendon tissues were increased under the action of FGF-2. This indicates that FGF-2 also has an influence on chondrogenic differentiation. Therefore, we speculate that the two factors may have an intersection in promoting the chondrogenic differentiation of stem cells in addition to their complementary effects, and the mechanism of action between them is worthy of further study.
In conclusion, our results suggest that the cell proliferation promotion effect of FGF-2 and the chondrogenic differentiation effect of KGN can jointly promote fibrocartilage regeneration at the tendon–bone interface. The CS-KGN/FGF-TBA hydrogel prepared by CS-TBA as a carrier can accelerate the regeneration of fibrocartilage after rotator cuff reconstruction and improve the tendon healing at the tendon–bone interface. CS-KGN/FGF-TBA has a wide application prospect in rotator cuff repair, and provides an important reference for the research and development of new clinical treatment methods for rotator cuff injury.
The raw data supporting the conclusions of this article will be made available by the authors, without undue reservation.
The animal study was reviewed and approved by Zhejiang University.
ZY and CT conceived the idea and designed the experiments. CT led and performed most of the experiments. YF conducted histological analysis and participated in polymer synthesis, material characterization, and in vivo experiments. HZ conducted the in vitro experiments. LH and YJ performed the animal experiments. CT wrote the manuscript.
This work was supported by the Key Disciplines of Jinhua and the Science and Technology Plan of Yiwu.
The authors declare that the research was conducted in the absence of any commercial or financial relationships that could be construed as a potential conflict of interest.
All claims expressed in this article are solely those of the authors and do not necessarily represent those of their affiliated organizations, or those of the publisher, the editors and the reviewers. Any product that may be evaluated in this article, or claim that may be made by its manufacturer, is not guaranteed or endorsed by the publisher.
The Supplementary Material for this article can be found online at: https://www.frontiersin.org/articles/10.3389/fmats.2021.754973/full#supplementary-material
Atesok, K., Fu, F. H., Wolf, M. R., Ochi, M., Jazrawi, L. M., Doral, M. N., et al. (2014). Augmentation of Tendon-To-Bone Healing. J. Bone Jt. Surg Am. 96, 513–521. doi:10.2106/jbjs.m.00009
Cai, J.-y., Zhang, L., Chen, J., and Chen, S.-y. (2019). Kartogenin and its Application in Regenerative Medicine. Curr. Med. Sci. 39, 16–20. doi:10.1007/s11596-019-1994-6
Chen, Y., Liu, X., Liu, R., Gong, Y., Wang, M., Huang, Q., et al. (2017). Zero-order Controlled Release of BMP2-Derived Peptide P24 from the Chitosan Scaffold by Chemical Grafting Modification Technique for Promotion of Osteogenesis In Vitro and Enhancement of Bone Repair In Vivo. Theranostics 7, 1072–1087. doi:10.7150/thno.18193
Chen, C. Y., Li, C., Ke, C. J., Sun, J. S., and Lin, F. H. (2021). Kartogenin Enhances Chondrogenic Differentiation of MSCs in 3D Tri-copolymer Scaffolds and the Self-Designed Bioreactor System. Biomolecules 11, 115. doi:10.3390/biom11010115
Gromolak, S., Krawczenko, A., Antończyk, A., Buczak, K., Kiełbowicz, Z., and Klimczak, A. (2020). Biological Characteristics and Osteogenic Differentiation of Ovine Bone Marrow Derived Mesenchymal Stem Cells Stimulated with FGF-2 and BMP-2. Int. J. Mol. Sci. 21, 9726. doi:10.3390/ijms21249726
Gulotta, L. V., Kovacevic, D., Ying, L., Ehteshami, J. R., Montgomery, S., and Rodeo, S. A. (2008). Augmentation of Tendon-To-Bone Healing with a Magnesium-Based Bone Adhesive. Am. J. Sports Med. 36, 1290–1297. doi:10.1177/0363546508314396
Guo, D., Li, H., Liu, Y., Yu, X., Zhang, X., Chu, W., et al. (2020). Fibroblast Growth Factor-2 Promotes the Function of Tendon-Derived Stem Cells in Achilles Tendon Restoration in an Achilles Tendon Injury Rat Model. Biochem. Biophys. Res. Commun. 521, 91–97. doi:10.1016/j.bbrc.2019.10.082
Hao, Z.-C., Wang, S.-Z., Zhang, X.-J., and Lu, J. (2016). Stem Cell Therapy: a Promising Biological Strategy for Tendon-Bone Healing after Anterior Cruciate Ligament Reconstruction. Cell Prolif. 49, 154–162. doi:10.1111/cpr.12242
Honda, H., Gotoh, M., Kanazawa, T., Ohzono, H., Nakamura, H., Ohta, K., et al. (2017). Hyaluronic Acid Accelerates Tendon-To-Bone Healing after Rotator Cuff Repair. Am. J. Sports Med. 45, 3322–3330. doi:10.1177/0363546517720199
Hong, Y., Liu, N., Zhou, R., Zhao, X., Han, Y., Xia, F., et al. (2020). Combination Therapy Using Kartogenin-Based Chondrogenesis and Complex Polymer Scaffold for Cartilage Defect Regeneration. ACS Biomater. Sci. Eng. 6, 6276–6284. doi:10.1021/acsbiomaterials.0c00724
Hou, M., Zhang, Y., Zhou, X., Liu, T., Yang, H., Chen, X., et al. (2021). Kartogenin Prevents Cartilage Degradation and Alleviates Osteoarthritis Progression in Mice via the miR-146a/NRF2 axis. Cell Death Dis 12, 483. doi:10.1038/s41419-021-03765-x
Huang, H., Xu, H., and Zhao, J. (2017). A Novel Approach for Meniscal Regeneration Using Kartogenin-Treated Autologous Tendon Graft. Am. J. Sports Med. 45, 3289–3297. doi:10.1177/0363546517721192
Ide, J., Kikukawa, K., Hirose, J., Iyama, K.-i., Sakamoto, H., Fujimoto, T., et al. (2009). The Effect of a Local Application of Fibroblast Growth Factor-2 on Tendon-To-Bone Remodeling in Rats with Acute Injury and Repair of the Supraspinatus Tendon. J. Shoulder Elbow Surg. 18, 391–398. doi:10.1016/j.jse.2009.01.013
Jing, H., Zhang, X., Gao, M., Luo, K., Fu, W., Yin, M., et al. (2019). Kartogenin Preconditioning Commits Mesenchymal Stem Cells to a Precartilaginous Stage with Enhanced Chondrogenic Potential by Modulating JNK and β‐catenin-related Pathways. FASEB j. 33, 5641–5653. doi:10.1096/fj.201802137rrr
Johnson, K., Zhu, S., Tremblay, M. S., Payette, J. N., Wang, J., Bouchez, L. C., et al. (2012). A Stem Cell-Based Approach to Cartilage Repair. Science 336, 717–721. doi:10.1126/science.1215157
Kafedjiiski, K., Föger, F., Werle, M., and Bernkop-Schnürch, A. (2005). Synthesis and In Vitro Evaluation of a Novel Chitosan-Glutathione Conjugate. Pharm. Res. 22, 1480–1488. doi:10.1007/s11095-005-6248-6
Kang, M. L., Ko, J.-Y., Kim, J. E., and Im, G.-I. (2014). Intra-articular Delivery of Kartogenin-Conjugated Chitosan Nano/microparticles for Cartilage Regeneration. Biomaterials 35, 9984–9994. doi:10.1016/j.biomaterials.2014.08.042
Karjalainen, T. V., Jain, N. B., Heikkinen, J., Johnston, R. V., Page, C. M., and Buchbinder, R. (2019). Surgery for Rotator Cuff Tears. Cochrane Database Syst. Rev. 12, CD013502. doi:10.1002/14651858.CD013502
Lei, T., Zhang, T., Ju, W., Chen, X., Heng, B. C., Shen, W., et al. (2021). Biomimetic Strategies for Tendon/ligament-To-Bone Interface Regeneration. Bioact. Mater. 6, 2491–2510. doi:10.1016/j.bioactmat.2021.01.022
Liu, X., Yu, B., Huang, Q., Liu, R., Feng, Q., Cai, Q., et al. (2016). In Vitro BMP-2 Peptide Release from Thiolated Chitosan Based Hydrogel. Int. J. Biol. Macromol. 93, 314–321. doi:10.1016/j.ijbiomac.2016.08.048
Longo, U. G., Risi Ambrogioni, L., Berton, A., Candela, V., Migliorini, F., Carnevale, A., et al. (2021). Conservative versus Accelerated Rehabilitation after Rotator Cuff Repair: a Systematic Review and Meta-Analysis. BMC Musculoskelet. Disord. 22, 637. doi:10.1186/s12891-021-04397-0
Lu, C. C., Ho, C. J., Huang, H. T., Lin, S. Y., Chou, S. H., Chou, P. H., et al. (2021). Effect of Freshly Isolated Bone Marrow Mononuclear Cells and Cultured Bone Marrow Stromal Cells in Graft Cell Repopulation and Tendon-Bone Healing after Allograft Anterior Cruciate Ligament Reconstruction. Int. J. Mol. Sci. 22, 2791. doi:10.3390/ijms22062791
Marquez, M. P., Alencastro, F., Madrigal, A., Jimenez, J. L., Blanco, G., Gureghian, A., et al. (2017). The Role of Cellular Proliferation in Adipogenic Differentiation of Human Adipose Tissue-Derived Mesenchymal Stem Cells. Stem Cell Dev. 26, 1578–1595. doi:10.1089/scd.2017.0071
Matsuda, A., Kobayashi, H., Itoh, S., Kataoka, K., and Tanaka, J. (2005). Immobilization of Laminin Peptide in Molecularly Aligned Chitosan by Covalent Bonding. Biomaterials 26, 2273–2279. doi:10.1016/j.biomaterials.2004.07.032
Mazuquin, B. F., Wright, A. C., Russell, S., Monga, P., Selfe, J., and Richards, J. (2018). Effectiveness of Early Compared with Conservative Rehabilitation for Patients Having Rotator Cuff Repair Surgery: an Overview of Systematic Reviews. Br. J. Sports Med. 52, 111–121. doi:10.1136/bjsports-2016-095963
Mifune, Y., Matsumoto, T., Takayama, K., Terada, S., Sekiya, N., Kuroda, R., et al. (2013). Tendon Graft Revitalization Using Adult Anterior Cruciate Ligament (ACL)-derived CD34+ Cell Sheets for ACL Reconstruction. Biomaterials 34, 5476–5487. doi:10.1016/j.biomaterials.2013.04.013
Özdemir, E., Karaguven, D., Turhan, E., and Huri, G. (2021). Biological Augmentation Strategies in Rotator Cuff Repair. Med. Glas (Zenica) 18, 186–191. doi:10.17392/1305-21
Patel, S., Caldwell, J. M., Doty, S. B., Levine, W. N., Rodeo, S., Soslowsky, L. J., et al. (2018). Integrating Soft and Hard Tissues via Interface Tissue Engineering. J. Orthop. Res. 36, 1069–1077. doi:10.1002/jor.23810
Rothrauff, B. B., and Tuan, R. S. (2014). Cellular Therapy in Bone-Tendon Interface Regeneration. Organogenesis 10, 13–28. doi:10.4161/org.27404
Schmucker, C., Titscher, V., Braun, C., Nussbaumer-Streit, B., Gartlehner, G., and Meerpohl, J. (2020). Surgical and Non-surgical Interventions in Complete Rotator Cuff Tears. Dtsch Arztebl Int. 117, 633–640. doi:10.3238/arztebl.2020.0633
Sedlar, A., Travnickova, M., Matejka, R., Prazak, S., Meszaros, Z., Bojarova, P., et al. (2021). Growth Factors VEGF-A165 and FGF-2 as Multifunctional Biomolecules Governing Cell Adhesion and Proliferation. Int. J. Mol. Sci. 22, 1843. doi:10.3390/ijms22041843
Shao, J., Zhu, J., Chen, Y., Fu, Q., Li, L., Ding, Z., et al. (2021). Exosomes from Kartogenin-Pretreated Infrapatellar Fat Pad Mesenchymal Stem Cells Enhance Chondrocyte Anabolism and Articular Cartilage Regeneration. Stem Cell Int 2021, 6624874. doi:10.1155/2021/6624874
Tokunaga, T., Shukunami, C., Okamoto, N., Taniwaki, T., Oka, K., Sakamoto, H., et al. (2015). FGF-2 Stimulates the Growth of Tenogenic Progenitor Cells to Facilitate the Generation of Tenomodulin-Positive Tenocytes in a Rat Rotator Cuff Healing Model. Am. J. Sports Med. 43, 2411–2422. doi:10.1177/0363546515597488
Wang, D., Tan, H., Lebaschi, A. H., Nakagawa, Y., Wada, S., Donnelly, P. E., et al. (2018). Kartogenin Enhances Collagen Organization and Mechanical Strength of the Repaired Enthesis in a Murine Model of Rotator Cuff Repair. Arthrosc. J. Arthroscopic Relat. Surg. 34, 2579–2587. doi:10.1016/j.arthro.2018.04.022
Xu, X., Shi, D., Shen, Y., Xu, Z., Dai, J., Chen, D., et al. (2015). Full-thickness Cartilage Defects Are Repaired via a Microfracture Technique and Intraarticular Injection of the Small-Molecule Compound Kartogenin. Arthritis Res. Ther. 17, 20. doi:10.1186/s13075-015-0537-1
Zhang, J., and Wang, J. H. (2014). Kartogenin Induces Cartilage-like Tissue Formation in Tendon-Bone junction. Bone Res. 2, 14008. doi:10.1038/boneres.2014.8
Zhang, C., Li, Q., Deng, S., Fu, W., Tang, X., Chen, G., et al. (2016). bFGF- and CaPP-Loaded Fibrin Clots Enhance the Bioactivity of the Tendon-Bone Interface to Augment Healing. Am. J. Sports Med. 44, 1972–1982. doi:10.1177/0363546516637603
Zhang, J., Yuan, T., Zheng, N., Zhou, Y., Hogan, M. V., and Wang, J. H.-C. (2017). The Combined Use of Kartogenin and Platelet-Rich Plasma Promotes Fibrocartilage Formation in the Wounded Rat Achilles Tendon Entheses. Bone Jt. Res. 6, 231–244. doi:10.1302/2046-3758.64.bjr-2017-0268.r1
Zhang, C., Wu, J., Li, X., Wang, Z., Lu, W. W., and Wong, T.-M. (2021). Current Biological Strategies to Enhance Surgical Treatment for Rotator Cuff Repair. Front. Bioeng. Biotechnol. 9, 657584. doi:10.3389/fbioe.2021.657584
Zhao, T., Qi, Y., Xiao, S., Ran, J., Wang, J., Ghamor-Amegavi, E. P., et al. (2019). Integration of Mesenchymal Stem Cell Sheet and bFGF-Loaded Fibrin Gel in Knitted PLGA Scaffolds Favorable for Tendon Repair. J. Mater. Chem. B 7, 2201–2211. doi:10.1039/c8tb02759e
Zhou, Y., Zhang, J., Yang, J., Narava, M., Zhao, G., Yuan, T., et al. (2017). Kartogenin with PRP Promotes the Formation of Fibrocartilage Zone in the Tendon-Bone Interface. J. Tissue Eng. Regen. Med. 11, 3445–3456. doi:10.1002/term.2258
Keywords: rotator cuff injury, hydrogel, KGN, FGF-2, tendon–bone interface
Citation: Teng C, Fang Y, Zhu H, Huang L, Jin Y and Ye Z (2021) A Dual-Factor Releasing Hydrogel for Rotator Cuff Injury Repair. Front. Mater. 8:754973. doi: 10.3389/fmats.2021.754973
Received: 07 August 2021; Accepted: 08 September 2021;
Published: 12 October 2021.
Edited by:
Yangjun Chen, Wenzhou Medical University, ChinaCopyright © 2021 Teng, Fang, Zhu, Huang, Jin and Ye. This is an open-access article distributed under the terms of the Creative Commons Attribution License (CC BY). The use, distribution or reproduction in other forums is permitted, provided the original author(s) and the copyright owner(s) are credited and that the original publication in this journal is cited, in accordance with accepted academic practice. No use, distribution or reproduction is permitted which does not comply with these terms.
*Correspondence: Zhaoming Ye, eWV6aGFvbWluZ0B6anUuZWR1LmNu
Disclaimer: All claims expressed in this article are solely those of the authors and do not necessarily represent those of their affiliated organizations, or those of the publisher, the editors and the reviewers. Any product that may be evaluated in this article or claim that may be made by its manufacturer is not guaranteed or endorsed by the publisher.
Research integrity at Frontiers
Learn more about the work of our research integrity team to safeguard the quality of each article we publish.