- 1Department of Clinical Pharmacy, School of Pharmacy, Shiraz University of Medical Sciences, Shiraz, Iran
- 2Pharmaceutical Sciences Research Center, School of Pharmacy, Shiraz University of Medical Sciences, Shiraz, Iran
- 3Department of Pharmaceutics, School of Pharmacy, Shiraz University of Medical Sciences, Shiraz, Iran
Different polymeric materials have been used as drug delivery vehicles for decades. Natural, semisynthetic, and synthetic polymers each have their own specific characteristics and, due to the physicochemical limitations of each polymer, tuning the release rate and targeting the active ingredient to a specific organ or site of action is a complicated task for pharmaceutical scientists. In this regard, polymer blending has been considered as an attractive approach to fabricate novel and unique drug delivery systems with modified physical and/or chemical characteristics. There are three major polymer blending approaches that are used for drug delivery purposes: physical mixtures, core-shell model, and block copolymer model. Each of these types of polymer blends could significantly affect the loading capacities and the kinetics of drug release from the relevant formulations. Drug release from these blended polymers can be tuned through the changes in temperature and pH of the environment, and physiochemical properties of the target organs. Furthermore, the possible molecular interactions among polymers and drug molecules can significantly affect the drug release profile from these blended polymeric micro- and nanocarriers. In this review, first of all, different types of polymers and their various applications in biomedical sciences have been discussed and smart or stimuli responsive polymers are introduced and categorized based on their nature. Then, the purpose of polymer blending in drug delivery systems has been discussed. Different types of polymer blends including physical mixtures, core-shell polymeric carriers, and block copolymers have been summarized with focus on the effect of polymer blending on encapsulated drug release profiles. Finally, the consequence of each blending approach on drug release profile and kinetics of drug release have been mentioned in tabular format.
Introduction
Controlled drug delivery systems have been considered as novel strategies in pharmaceutical sciences in order to minimize unwanted adverse drug reactions and achieve optimum efficacy by minimizing the concentration fluctuations and increasing the interval of drug administration and drug delivery to the site of action. In this regard, different types of polymeric and non-polymeric carriers with different particle size ranges have been studied (Ghasemiyeh et al., 2017; Ghasemiyeh and Mohammadi-Samani, 2018; Ghasemiyeh et al., 2019; Ghasemiyeh and Mohammadi-Samani, 2019; Ghasemiyeh and Mohammadi-Samani, 2020). Polymeric nanoparticles can be fabricated using different natural and synthetic polymers (Chakravarthi et al., 2007). Polymeric nanoparticles have the advantages of biocompatibility and low toxicity in comparison to other types of nanocarriers (Ahmadi et al., 2015; Ghasemiyeh and Mohammadi-Samani, 2020). Natural polymeric nanoparticles are fabricated through the recruitment of polymers that come from natural sources such as alginate, albumin, gelatin, and chitosan. These polymers are produced through extraction followed by several purification processes. Natural polymers have the potential of hydrogel formation which makes them a suitable candidate for delivery of hydrophilic drugs, oligonucleotides, peptides, and proteins (Zhang et al., 2013). Due to the lack of purity and potential batch-to-batch variations of some of these available natural polymers, synthetic polymers were introduced. Synthetic polymers are suitable for delivery of both hydrophilic and lipophilic agents. Also, programmed drug release can be achieved through the recruitment of these polymeric nanoparticles as novel drug delivery systems. The most commonly used biodegradable synthetic polymers for drug delivery purposes are polylactide (PLA), poly (lactide-co-glycolide) (PLGA), and poly (ε-caprolactone) (PCL). While the most common non-degradable ones are polystyrene, poly (methyl methacrylate), and polyacrylate (Zhang et al., 2013). Different applications of polymers and polymeric nanoparticles are summarized in Table 1.
Controlled drug delivery and drug release from polymers can follow one or more of these three major mechanisms. The first is Fickian diffusion of the drug molecule through the pores or backbone of the polymer structure which is the rate limiting step of drug release from polymeric nanoparticles (Langer, 1993). The second mechanism is related to chemical reaction in term of hydrolytic or enzymatic degradation of the polymers that can result in the release of the entrapped therapeutic agents. Also, chemical reaction can appear as a bond cleavage between drug and polymer that release the encapsulated drug conjugated to the polymer. This chemical reaction can be triggered with various environmental conditions in smart or stimuli-responsive polymers (Langer, 1993). The third mechanism of drug release from polymers can be attributed to the osmotic pressure and solvent-activated drug release. In this regard, polymer will absorb large amount of solvent through a semi-permeable membrane and swell accordingly. The dissolved osmotic agent will induce osmotic pressure that can result in drug release from the polymer shell (Langer, 1993).
Polymer blending has been considered as an attractive method in which the physicochemical characteristics of these systems would be different from the characteristics of each polymer alone. Among the different types of polymeric materials as drug carrier, stimuli-responsive polymers are considered attractive polymeric carriers which undergo phase transitions in response to different environmental stimuli including temperature, pH, light, and enzymes. (Keogh et al., 2020). Thermo-responsive polymers are a common type of smart or stimuli-responsive polymeric carrier that show a change in solubility at a point called critical solution temperature (CST) (Ward and Georgiou, 2011). This trigger can be considered as a stimulus in drug release initiation. This attractive potential makes the thermo-responsive polymers suitable nanoparticles for different biomedical applications including gene delivery, drug delivery, tissue engineering, protein-ligand recognition, enzyme immobilization, and artificial organs production (Ward and Georgiou, 2011; Cirillo et al., 2015). Among these thermo-responsive polymeric nanoparticles, those that are fabricated using N-isopropylacrylamide (NIPAAm) are more popular in pharmaceutical sciences for drug delivery purposes (Cirillo et al., 2015). Most of these delivery systems are block copolymer with unique characteristics. Polymeric blends and different approaches of polymer blending could significantly affect this CST and tune the drug release profile for drug delivery purposes (Keogh et al., 2020). For biomedical applications, the trigger to form thermo-responsive hydrogels would be the change in temperature from environment to physiologic condition which is called in situ hydrogel formation (Klouda, 2015). Thermally-responsive polymers can be divided into two categories: natural and synthetic polymers. The detail of these thermo-responsive hydrogels are summarized in Table 2 (Klouda, 2015).
Polymer blending approaches can result in possible molecular interactions among blended polymers which can be used in the fabrication of novel polymeric nanoparticles with unique characteristics. In this regard, it has been reported that the molecular interaction between chitosan and collagen through physical mixture could result in a polymeric carrier with novel characteristics (Sionkowska et al., 2004). These molecular interactions between chitosan and collagen are polyelectrolytic interactions through the polyanion/polycation complex formation. FTIR results revealed that a new hydrogen bond (between–OH, –COOH, or–NH2 groups of collagen and–OH or–NH2 groups of chitosan) was formed in this blended polymers structure, which confirmed the collagen denaturation process during physical mixture with chitosan. The blended chitosan-collagen polymers were miscible, which proved the altered molecular characteristics of the blended polymers in comparison to each one alone. Further, the viscosity of the finalized blended product was higher than each component alone which also emphasized the creation of molecular interactions between chitosan and collagen (Sionkowska et al., 2004). Also, it has been reported that blending and copolymerization of chitosan with PEG can result in improved ductility properties of chitosan which can be attributed to the inter-molecular interactions between chitosan and PEG polymers (Kolhe and Kannan, 2003).
In this focused review, first of all, the purpose of polymer blending in drug delivery systems and their advantages have been discussed. After that, different methods of polymer blending including physical mixture, core-shell polymeric carriers, and block copolymer have been summarized (Figure 1). Finally, the consequence of each blending approach on drug release profile and kinetics of drug release have been discussed. In the conclusion, the recently used polymer blends, the loaded drug, and the suggested drug release profile and kinetics have been summarized in tabular format.
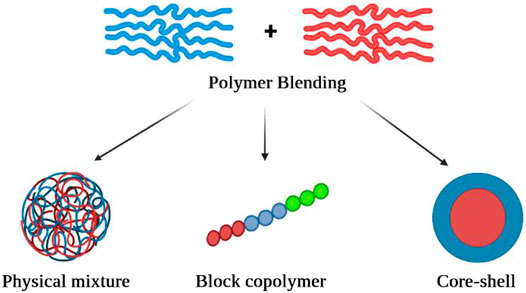
FIGURE 1. A schematic view of different polymer blending approaches including physical mixture, block copolymer, and core-shell model.
Polymers Blending
Drug delivery scaffolds are considered smart novel drug delivery systems that are used in controlled spatiotemporal drug release. These scaffolds are composed of various natural and/or synthetic polymer blends to fabricate novel and unique systems with improved characteristics (Calori et al., 2020). The process of polymer blending can result in changes in physicochemical characteristics of the final product in comparison to the homopolymers alone. The main advantages of polymers blending is the fabrication of miscible polymer mixtures. The physical characteristics of the blended polymers depend not only on the characteristics of each homopolymer alone but also depend on the possible intramolecular interactions among blended polymers. Furthermore, the polymer blending process can result in fabrication of a novel product with second functionality that would have potential for the formation of new interactions with other polymers and drugs (Jones et al., 2005). The most common natural polymers that are used in this field are hyaluronic acid (HA), chitosan, alginate, collagen, gelatin, elastin, keratin, and silk fibroin. Also, the most commonly available synthetic polymers used for this purpose are polylactide (PLA), polyglycolic acid (PGA), polyvinyl alcohol (PVA), and poly-ε-caprolactone (PCL) (Calori et al., 2020).
Physical Mixtures
Simply blending low molecular weight hyaluronic acid (HA) with carboxymethyl hexanoyl chitosan (CHC) under stirring without thermal treatment can result in the fabrication of in situ injectable hydrogels. The probable mechanisms of this hydrogel formation are hydrophilic-hydrophobic interactions, supramolecular assembly, and electrostatic micelle formation in a pH-dependent manner. The prepared hydrogel was biocompatible, biodegradable, bioadhesive, and shape-persistent. This polymer blend showed a pH-sensitive extended drug release profile at pH 6. The rate of these polymers blend degradation was accelerated at physiologic pH (pH = 7.4) (Lu et al., 2019). These injectable hydrogels are potentially applicable in tissue engineering and soft tissue repairing through a non-invasive procedure. Also, CHC can be physically mixed with thermal responsive agents including Pluronic F-127 and β-sodium glycerophosphate (β-GP) to form in situ temperature-sensitive injectable hydrogel (Lu et al., 2019). The major mechanism of drug release from the hydrogels would be Fickian diffusion through the pores of macromolecular chains in swollen hydrogels. However, the mechanism of drug release from these thermo-responsive hydrogels would be highly dependent on the process of drug entrapment within the hydrogel, drug-polymer affinity, and the polymeric network structure (Cirillo et al., 2015). In recent decades, chitosan has been used as a suitable carrier with mucoadhesive potential for buccal drug delivery purposes. However, chitosan polymers have some limitations including poor tensile and weak adhesion strength characteristics. These limitations can be overcome through the recruitment of polymer blending approaches (Freag et al., 2018). It has been reported that a physical mixture of chitosan and hydroxypropyl methylcellulose (HPMC) can result in a novel blended polymer with improved mucoadhesive properties for the buccal route of administration (Freag et al., 2018). Physical blending of HPMC with chitosan can result in enhanced hydration capacity which is required for controlled and uniform drug release through the buccal route (Freag et al., 2018). Furthermore, results of this study revealed that chitosan mixed with of Sodium carboxymethyl cellulose (NCMC), Sodium alginate (NALG), or hyaluronic acid (HA), respectively, fail to enhance the mucoadhesive potential while blending chitosan with either HPMC or Carbopol® (CRB) could result in significantly better mucoadhesive characteristics and residence time in the buccal cavity (Freag et al., 2018). Gelatin, agar, and κ-carrageenan are considered as suitable polymers to fabricate hydrogels with controlled drug release potential; however, the release rate of hydrophilic agents from each of these polymers would be fast. The effect of physically two-by-two blending of either gelatin, agar, or κ-carrageenan polymers (with 1:1 ratio) on the release pattern of theophylline from hydrogels has been studied (Liu et al., 2005). Results revealed that polymer blending was accompanied by sustained and prolonged drug release from hydrogels that can be attributed to the longer and more tortuous pathways that drug molecules should pass to exit from the structure of the hydrogels. Furthermore, the viscosity of the hydrogel can reduce the drug release by inducing a hindrance in molecular diffusion. Also, it has been reported that the addition of a polysaccharide including agar and κ-carrageenan to the gelatin could result in a slower release pattern in comparison to the blending of these two polysaccharides together (Liu et al., 2005). The effect of temperature on the release profile revealed that by enhancing the temperature, the release rate was increased in each studied polymer blend. Among all of these assessed polymer blends, hydrogels fabricated from agar and gelatin physical mixture (with 1:1 ratio) resulted in the slowest drug release profile (Liu et al., 2005). The miscibility studies on pairwise polymer blends revealed that physical mixture of hydroxypropyl methylcellulose acetate succinate (HPMCAS)/PVP, HPMC/carboxymethyl cellulose acetate butyrate (CMCAB), and PVP/HPMC were miscible while Eudragit 100 (E100)/PVP and E100/HPMC had a miscibility gap. A physical mixture of these immiscible polymers can result in polymer blends with balanced amorphous solid dispersions (ASDs) that can result in controlled drug release rate and formation of polymer-polymer and polymer-drug interactions to avoid further nucleation and crystal growth of hydrophobic drugs (Marks et al., 2014).
Physical mixture of some synthetic polymers including polyethylene terephthalate (PET) and polyacrylonitrile (PAN) can result in the production of an activated carbon product with different medical, pharmaceutical, and industrial applications including pesticide removal from the liquid phase. The activated carbon can act as an efficient adsorbent agent (Belo et al., 2017). Different natural and synthetic polymers can be blended physically in order to fabricate novel polymeric micro- and nanoparticles with unique characteristics. In this regard, collagen as a natural polymer can be blended with different synthetic polymers including PVP, PEO, PVA, and PEG (Sionkowska, 2011). These blended polymers can be used for different applications including drug delivery systems, wound healing process, tissue engineering, and medical devices production (Sionkowska, 2011). Furthermore, chitosan is another natural polymer that can be blended with different synthetic polymers including PEG, PVP, PCL, PEO, and PLA, and polyacrylamide (PAAm) (Sionkowska, 2011). Other natural polymers that can blend with synthetic polymers are keratin, elastin, silk fibroin, and starch (Vaidya and Bhattacharya, 1994; Sionkowska, 2011).
Core-shell Polymeric Carriers
Polymeric nanofibers and microfibers that are composed of either synthetic or natural polymers with a diameter range of a few nanometers to several micrometers have been considered for use as drug delivery systems. A novel class of nanofibers that have a core-shell or core-sheath structure have been developed using coaxial and emulsion electrospinning techniques (Monfared et al., 2019). The most important advantages of polymeric core-shell nanofibers are their high surface area, nanoscale particle size, porous structure, simply controlled structure, flexible platform, biomimetic and biocompatible properties, high encapsulation efficiency, and sustained controlled drug release at the site of action (Monfared et al., 2019). The main drawback of these blended polymeric nanofibers for drug delivery purposes is their limited ability to sustain drug release for hydrophilic agents. Small molecule hydrophilic drugs that are highly soluble in release medium while poorly compatible with insoluble polymers with poor partitioning coefficient can result in initial burst release and overall faster release rate (Chou et al., 2015). In contrast, burst drug release would be avoided and overall prolonged drug release would be achieved for hydrophobic agents due to their poor solubility in release medium, higher compatibility with insoluble polymers, and sufficient partitioning into the hydrophobic polymers (Monfared et al., 2019; Zupančič, 2019). In order to obtain controlled drug release, core-shell structures, also known as double wall microspheres, containing a bulk-eroding core (like PLGA) and a surface eroding sheath (like polyanhydride) has been suggested to significantly prevent initial burst release of encapsulated agents (Mohammadi-Samani and Taghipour, 2015). Drug release patterns from biodegradable core-shell nanofibers would take place based on three different mechanisms: diffusion, shell thickness, and degradation (Vashisth et al., 2016). Most of the added drug would be entrapped within the core phase of the polymeric nanofibers and molecular diffusion is the most common mechanism responsible for the extended drug release from these core-shell nanofibers. While the polymer matrix is degrading, the incorporated drug will be released from the nanofibers. Drug release from these core-shell nanofibers is almost completely a two-phasic release pattern containing an initial burst release followed by a sustained and controlled drug release. This biphasic release pattern in core-shell polymeric nanoparticles can be used as dual action drug delivery system (one dissolves in the core phase and the other in the shell) with different rates of release (Monfared et al., 2019). It has been reported that in a core-shell nanofiber fabricated from poly (methyl methacrylate) (PMMA) in the shell and PMMA/PVA blend in the core phase, the lower the ratio of PVA in the core, the lower the incidence of initial burst release (Zupančič et al., 2016). Recruitment of electrospun core-shell nanofibers can induce partition-controlled drug release especially for hydrophilic agents such as DNA, proteins, and peptides. In this regard, PVA/PCL core-shell, PVA/poly-L-lactide (PLLA) core-shell, and PVA/PLGA core-shell had been studied. In this study, hydrophilic drug release from each of these monolithic fibers, ie. PVA, PLLA, PCL, and PLGA, resulted in initial burst release which was highest for PVA and lowest for PLLA since the PVA showed immediate swelling in release medium and resulted in 90% initial burst release (Tiwari et al., 2010). However, the PLLA monoliths, with a high Tg [glass transition temperature] value, could entrap most of the insoluble drug into the fiber bulk and resulted in the least initial burst release (about 10%). Using PVA as the core phase in core-shell polymer blends could significantly reduce the initial burst release and sustain the overall drug release pattern in comparison to each monolith fiber. These observed data could be attributed to the fewer undissolved drug molecules through the recruitment of core polymer (Tiwari et al., 2010). Using PCL as the core phase of the core-shell nanofibers can result in facilitated drug release due to the nanoporous structure of PCL that can result in water sorption within the core of the nanofiber which in turn facilitates drug desorption from the surface of the core phase. Drug desorption, which is the rate-limiting step of drug release, could be bypassed through this core-shell polymeric carrier fabrication and faster drug release would be predictable (Srikar et al., 2008). Fabrication of blended core-shell PVA/PLGA nanofibers in comparison to the monolithic PLGA fibers could significantly sustain the drug release profile. The probable reason for these observed data regarding drug release from PLGA and PVA/PLGA fibers would be the ease of molecular diffusion through the monolithic PLGA fiber that results in faster drug release while drug partitioning through the core-shell PVA/PLGA nanofiber was more complicated and resulted in a slower release pattern (Tiwari et al., 2010). In general, in order to obtain optimized controlled drug release using core-shell nanofibers, the core and shell layers should have different hydrophilic/hydrophobic characteristics. Furthermore, the incorporated drug should be more soluble in the core phase. In addition, sufficient polymer concentration in the shell layer is essential to avoid pore formation and initial burst release. The molecular diffusion through the shell layer should not be too slow to hinder drug release from the nanocarrier (Tiwari et al., 2010). Polymer-based microspheres have the advantage of biocompatibility, ease of fabrication, tunable physicochemical characteristics, and controlled drug release patterns. Core-shell polymeric microspheres have many additional advantages that can be attributed to the tunable properties of the shell layer. The shell phase of these core-shell microspheres can induce more control on drug release profile and release kinetics of the encapsulated drug within the core phase. Initial burst release would be avoided by drug diffusion through both core and shell layers. Using suitable shell layers can result in significantly reduced initial burst release followed by further prolonged and extended drug release. Also, the shell layer’s thickness can significantly affect the drug release kinetics (Kong et al., 2013). Furthermore, other advantages of these core-shell polymeric microspheres are their potential in the protection of the fragile therapeutic agents encapsulated within the core layer from the harsh environment, targeted drug delivery through the attachment of reagents and functional groups at the surface of the shell layer, and simultaneous dual drug delivery of two distinct therapeutic agents encapsulated separately in core and shell layers (Kong et al., 2013). In this regard, alginate-PLGA core-shell microspheres had the potential of higher entrapment efficiency and could prevent the unwanted leakage of encapsulated hydrophilic agents, while PLGA-alginate core-shell microspheres could reduce initial burst release and provide overall extended drug release profile for encapsulated hydrophobic agents within the PLGA as core layer (Kong et al., 2013). In general, PLGA has been considered as a suitable polymer to control the release rate of encapsulated hydrophobic agents while alginate is a suitable candidate to provide high encapsulation efficiency of hydrophilic agents (Kong et al., 2013). A short list of several polymers used in the fabrication of core-shell polymeric carriers has been shown in Table 3.
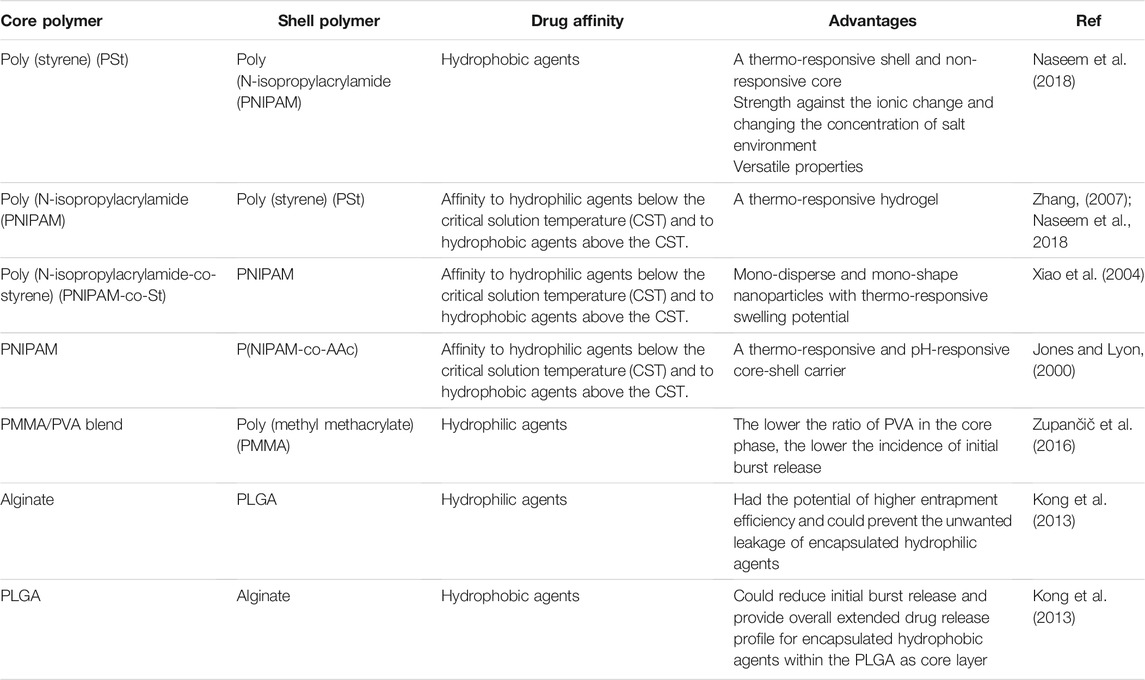
TABLE 3. Different polymers which were used in the fabrication of core-shell polymeric carriers as novel drug delivery systems.
Block Copolymers
Block copolymers are sophisticated compounds with two or more different chemical moieties that are linked together through chemical covalent bonding. The process of copolymerization can be tuned in order to fabricate polymer blends with different arrangements and characteristics especially for tri-block copolymers design and synthesis (Calori et al., 2020). The most common techniques used in the synthesis of di-block and/or tri-block copolymers are ionic (anionic and cationic) polymerization, free-radical polymerization, metal-catalyzed polymerization (ring-opening metathesis polymerization (ROMP), and α-olefin polymerization) (Hillmyer, 1999). The copolymers’ compositions and the length of polymers’ blocks can significantly affect the degree of phase separation and transition phase (Calori et al., 2020). Block copolymers are synthesized with different purposes including enhancing the hydrophilicity that can be achieved by the addition of polyethylene oxide (PEO) moieties to the hydrophobic polymers. Also, the PEG/PCL block copolymer is a smart thermo-responsive hydrogel that gained FDA approval for local drug delivery via the parenteral route (Calori et al., 2020). Poly (lactic-co-Glycolic Acid) (PLGA) is a kind of block copolymer in which PLA and PGA moieties have been covalently bonded (Calori et al., 2020). PLGA is used in the delivery of various peptides, proteins, genes, nucleotides, and many other therapeutic agents. So, it has been considered as a favorable scaffold for drug delivery and gene delivery purposes especially in the field of biomedicine (Roointan et al., 2018a). PLGA block copolymer has the advantage of biocompatibility, biodegradability, and mechanical strength. Also, it could produce non-toxic metabolites including glycolic acid and lactic acid. Using the PLGA polymeric blend, drug release profile and release kinetics would be tuned more appropriately (Mohammadi-Samani and Taghipour, 2015). The most addressed advantage of using PLGA for drug delivery purposes are the need for less frequent drug administration due to extended drug release capability, lower total dose requirements, avoidance of unwanted fluctuations in plasma concentration, and reduced adverse drug reactions (Mohammadi-Samani and Taghipour, 2015). The PLGA polyesters exist in two forms, namely crystalline and amorphous states, which are affected by the lactide/glycolide ratio used in PLGA synthesis. The higher the lactide/glycolide ratio, the longer the time spent for block copolymer degradation, and a more sustained release profile would be expected (Mohammadi-Samani and Taghipour, 2015; Roointan et al., 2018a). Also, the hydrophilicity, drug encapsulation, degradation rate, and drug release profile are highly affected by the PLGA terminal group that can vary from COOH to COOR with different length and moieties (Mohammadi-Samani and Taghipour, 2015). The possible mechanisms of PLGA erosion are bulk (homogenous) erosion and surface (heterogeneous) erosion; the former is the dominant mechanism. One of the main drawbacks of using these block copolymers for drug delivery purposes is the possibility of burst release and irregular release profile of the encapsulated proteins that can be attributed to surface adsorption of proteins. Also, there are many water-filled pores and cracks in block copolymer structures that can induce burst release (Mohammadi-Samani and Taghipour, 2015). One of the reported efforts to overcome this drawback would be the fabrication of microparticle composite, containing PLGA and another polymer, with a core-shell structure while a hydrophilic polymer is located in the core and PLGA in the shell and results in sustained release of encapsulated hydrophilic therapeutic agents (Mohammadi-Samani and Taghipour, 2015). Also, recruitment of alginate and a surfactant in the fabrication of PLGA copolymer would be helpful to enhance drug entrapment efficiency and reduce initial burst effects (Roointan et al., 2018a). Drug release kinetics of PLGA block copolymer follows a multiphasic profile. Also, the method of drug incorporation could significantly affect the release profile. In this regard, surface adsorption of the drug to the PLGA block copolymer could result in 60–70% initial burst release (Mohammadi-Samani and Taghipour, 2015). Copolymer blending is a type of polymer blending approach in which two or more block copolymers with different characteristics, functionalities, and stimuli-responsiveness are mixed in order to form a unique polymer blend with special characteristics (Keogh et al., 2020). It has been reported that recruitment of albumin in the synthesis of block copolymers could affect drug release profile from hydrogels, while the gel consistency of the fabricated block copolymer was reduced (Perinelli et al., 2014). The possible mechanism of dissolution and drug release from Poloxamer-containing hydrogels, fabricated through the block copolymer approach, was a combination of drug diffusion and hydrogel erosion. Using bovine serum albumin (BSA) in preparation of these thermo-responsive hydrogels resulted in slower drug release with a lower terminal of release. However, the results of this study revealed that the recruitment of proteins such as BSA in the fabrication of thermo-responsive block copolymers affects Poloxamer gelation more than its micellization process. In addition, it has been emphasized that although the gel consistency of the finalized block copolymer was reduced in the presence of BSA, the rate of drug release was also reduced in this condition. These results could be attributed to different mechanisms of drug release that BSA might be involved in other than the well-recognized drug dissolution and hydrogel erosion mechanisms (Perinelli et al., 2014). Using block copolymers that are composed of PEG and PLA polymers could result in the fabrication of novel drug delivery systems with desired drug release profiles. Carboxymethyloxysuccinic acid (CMOSA), a non-toxic, hydrophilic, and biodegradable polycarboxylic acid, has been blended with the PLA-PEG-PLA tri-block copolymer through copolymerization and group modification (esterification) (Zhang et al., 2008). The novel synthesized HO2C-PLA-PEG-PLA-CO2H copolymer had more hydrophilic characteristics and could enhance drug encapsulation potential and improve drug release profile. Results of this study reveal that the synthesized HO2C-PLA-PEG-PLA-CO2H copolymer has a porous spherical shape with enhanced encapsulation efficiency and loading capacity values in comparison to the PLA-PEG-PLA tri-block copolymer for both hydrophilic and lipophilic therapeutic agents. Also, this novel copolymer showed a reduced initial burst release and a more sustained and controlled release profile in comparison to the PLA-PEG-PLA block copolymer alone. This phenomenon could be attributed to the hydrogenic bond formation between carboxyl groups of HO2C-PLA-PEG-PLA-CO2H and the hydroxyl group of the hydrophilic encapsulated therapeutic agents that could prevent drug adsorption to the surface of nanoparticles or microparticles and avoid faster initial burst release. Also, the same results were reported for hydrophobic drugs encapsulated in the HO2C-PLA-PEG-PLA-CO2H block copolymer. In general, the drug release rate from these copolymers was faster for hydrophilic drugs in comparison to the hydrophobic ones that can be the consequence of free diffusion of hydrophilic agents through the aqueous medium of the hydrophilic block copolymers (Zhang et al., 2008). The recruitment of polyethylene glycol-b-polyaspartic acid (PEG-b-PAsp) as an interesting pH-sensitive block copolymer to sheath the lipid nanoparticles’ surface could significantly enhance the systemic circulation, plasma concentration, and physiologic activity of the loaded drug that can be attributed to the protective effect of sheathed PEG. Also, the pH-responsiveness of this block copolymer could result in targeted drug release in the tumor area to achieve passive tumor-targeting potential in order to provide drug delivery to the drug-resistant cancerous cells (Tran et al., 2015). Dispersion of gancyclovir-loaded PLGA microspheres in thermo-responsive PLGA-PEG-PLGA tri-block copolymer could result in a three-phasic release pattern (sigmoidal equation) including initial drug diffusion, matrix hydration, and subsequent matrix degradation. This polymer blending approach could significantly enhance the encapsulation efficiency and reduce the initial burst release due to closer packing potential in comparison to the PLGA microspheres alone (Duvvuri et al., 2005; Duvvuri et al., 2006). The effect of physically blending PLGA with PLGA-b-PEG block copolymer on entrapment efficiency and release pattern of paclitaxel as a hydrophobic drug has been studied. Based on the reported results it was revealed that by enhancing the PLGA/PLGA-b-PEG ratio, the percentage of entrapment efficiency was enhanced due to the increment in hydrophobicity characteristics of the polymer blend that could result in higher paclitaxel (hydrophobic agent) entrapment in its structure. The mixture of PLGA-b-PEG block copolymer with PLGA polymer could result in the fabrication of a novel blended polymer with an enhanced rough surface area. However, the release rate from the neat PLGA-b-PEG copolymer was slower than the blended PLGA-b-PEG and PLGA polymers. This would be attributed to the much lower surface area of neat PLGA-b-PEG in comparison to the blended polymers or differences in hydrophobicity of these carriers. Results of this study revealed that the paclitaxel release from this novel polymer blend was much more diffusion-controlled (Fick’s second law of diffusion) and polymer degradation would be less probable. Finally, these physically blended polymers showed suitable biocompatibility and cell viability that would be promising for drug and gene delivery purposes (Hussain et al., 2017).
Drug Release Profile and Release Kinetics
Polymer blending and the approach that has been used in this regard can significantly affect the release profile and kinetics of drug release. Each blending approach has its pros and cons that should be considered separately in order to achieve targeted drug delivery with optimal drug release kinetics. Sometimes the combination of these three principle blending methods, namely physical mixture, core-shell model, and block copolymerization, can be combined to resolve some important disadvantages of each method. A list of some recent research on polymer blending approaches used for drug delivery purposes with a focus on blending type and the consequent drug release profile and release kinetics have been summarized in Table 4.
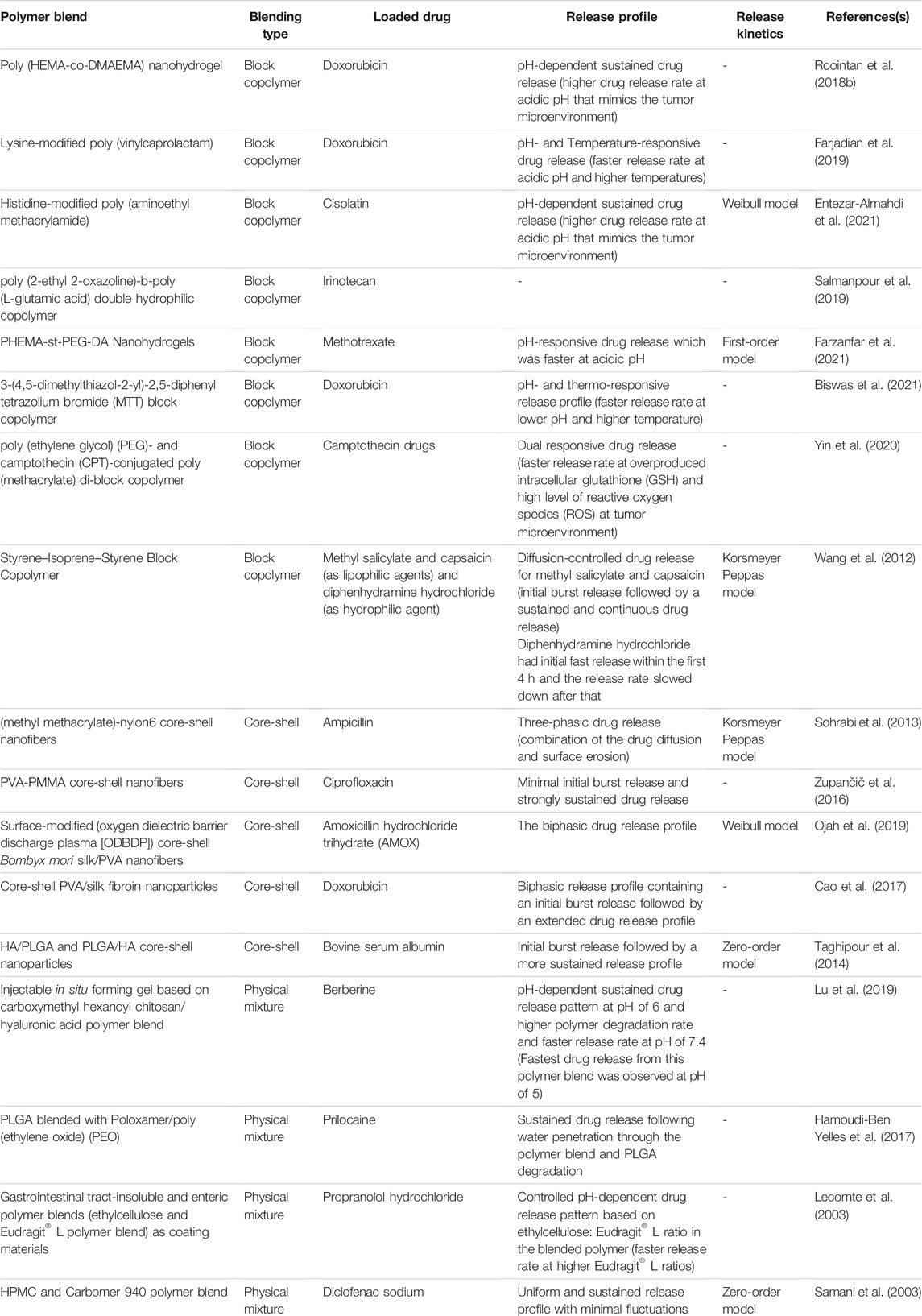
TABLE 4. Recently used polymeric blends for drug delivery purposes, the blending type, the loaded drug, and their release kinetics.
Discussion and Conclusion
Although polymers from different origins and with different chemical natures were introduced in biomedicine as carriers in drug delivery purposes, a survey in literature reveals that, in many cases, recruitment of a single polymer does not fulfill the appropriate characteristics of a sophisticated drug delivery system in terms of site-specific and time-controlled manner of release profiles. In this regard, using different type of polymer blends from simple physical mixture to more sophisticated core-shell strategy up to recruitment of polymeric block copolymer will open new aspects in drug delivery as site-specific and rate-controlling means in pharmaceutical sciences. Although there is not any simple recommendation for all instances, in order to solve formulation limitations, application of different strategies relevant to polymers blending would be useful.
Current Investigations Limitations
In this focused review only pharmaceutical and biomedical applications of polymer blends were considered, but different applications in chemistry and water treatment and other fields of science exist which have not been addressed in this manuscript.
Future Directions
Focus on new applications of polymer blends and attention to new polymers for modulating the rate, site, and kinetics of drug release would be necessary to maximize the drug therapy efficiency and reduce the common side effects of present drugs.
Author Contributions
PG contributed to study design, data gathering, and writing-original draft, writing-reviewing and revising. S-MS contributed to conceptualization, study design, supervision, project administration, and writing-reviewing and revising. All authors have read and approved the final manuscript.
Conflict of Interest
The authors declare that the research was conducted in the absence of any commercial or financial relationships that could be construed as a potential conflict of interest.
Publisher’s Note
All claims expressed in this article are solely those of the authors and do not necessarily represent those of their affiliated organizations, or those of the publisher, the editors and the reviewers. Any product that may be evaluated in this article, or claim that may be made by its manufacturer, is not guaranteed or endorsed by the publisher.
References
Abdelhameed, R. M., Abdel-Gawad, H., and Emam, H. E. (2021). Macroporous Cu-MOF@cellulose Acetate Membrane Serviceable in Selective Removal of Dimethoate Pesticide from Wastewater. J. Environ. Chem. Eng. 9 (2), 105121. doi:10.1016/j.jece.2021.105121
Abdelhameed, R. M., El-Shahat, M., and Emam, H. E. (2020). Employable Metal (Ag & Pd)@MIL-125-NH2@cellulose Acetate Film for Visible-Light Driven Photocatalysis for Reduction of nitro-aromatics. Carbohydr. Polym. 247, 116695. doi:10.1016/j.carbpol.2020.116695
Ahmadi, F., Oveisi, Z., Samani, S. M., and Amoozgar, Z. (2015). Chitosan Based Hydrogels: Characteristics and Pharmaceutical Applications. Res. Pharm. Sci. 10 (1), 1–16.
Ahmed, H. B., and Emam, H. E. (2019). Synergistic Catalysis of Monometallic (Ag, Au, Pd) and Bimetallic (Ag Au, Au Pd) versus Trimetallic (Ag-Au-Pd) Nanostructures Effloresced via Analogical Techniques. J. Mol. Liquids 287, 110975. doi:10.1016/j.molliq.2019.110975
Aranaz, I., Acosta, N., Civera, C., Elorza, B., Mingo, J., Castro, C., et al. (2018). Cosmetics and Cosmeceutical Applications of Chitin, Chitosan and Their Derivatives. Polymers 10 (2), 213. doi:10.3390/polym10020213
Asthana, N., Pal, K., Aljabali, A. A. A., Tambuwala, M. M., de Souza, F. G., and Pandey, K. (2021). Polyvinyl Alcohol (PVA) Mixed green-clay and Aloe Vera Based Polymeric Membrane Optimization: Peel-Off Mask Formulation for Skin Care Cosmeceuticals in green Nanotechnology. J. Mol. Struct. 1229, 129592. doi:10.1016/j.molstruc.2020.129592
Belo, C. R., Cansado, I. P. d. P., and Mourão, P. A. M. (2017). Synthetic Polymers Blend Used in the Production of High Activated Carbon for Pesticides Removals from Liquid Phase. Environ. Technol. 38 (3), 285–296. doi:10.1080/09593330.2016.1190409
Biswas, G., Jena, B. C., Samanta, P., Mandal, M., and Dhara, D. (2021). Synthesis, Self-Assembly and Drug Release Study of a New Dual-Responsive Biocompatible Block Copolymer Containing Phenylalanine Derivative. J. Macromolecular Sci. A, 1–12. doi:10.1080/10601325.2021.1947748
Calori, I. R., Braga, G., de Jesus, P. d. C. C., Bi, H., and Tedesco, A. C. (2020). Polymer Scaffolds as Drug Delivery Systems. Eur. Polym. J. 129, 109621. doi:10.1016/j.eurpolymj.2020.109621
Cao, Y., Liu, F., Chen, Y., Yu, T., Lou, D., Guo, Y., et al. (2017). Drug Release from Core-Shell PVA/silk Fibroin Nanoparticles Fabricated by One-step Electrospraying. Sci. Rep. 7 (1), 11913–11919. doi:10.1038/s41598-017-12351-1
Chakravarthi, S. S., Robinson, D. H., and De, S. (2007). Nanoparticles Prepared Using Natural and Synthetic Polymers. Nanoparticulate Drug Delivery Systems. CRC Press, 51–60. doi:10.1201/9781420008449-3
Chou, S.-F., Carson, D., and Woodrow, K. A. (2015). Current Strategies for Sustaining Drug Release from Electrospun Nanofibers. J. Controlled Release 220, 584–591. doi:10.1016/j.jconrel.2015.09.008
Cirillo, G., Spataro, T., Curcio, M., Spizzirri, U. G., Nicoletta, F. P., Picci, N., et al. (2015). Tunable Thermo-Responsive Hydrogels: Synthesis, Structural Analysis and Drug Release Studies. Mater. Sci. Eng. C 48, 499–510. doi:10.1016/j.msec.2014.12.045
Doberenz, F., Zeng, K., Willems, C., Zhang, K., and Groth, T. (2020). Thermoresponsive Polymers and Their Biomedical Application in Tissue Engineering - a Review. J. Mater. Chem. B 8 (4), 607–628. doi:10.1039/c9tb02052g
Duarah, S., Pujari, K., Durai, R. D., and Narayanan, V. H. B. (2016). Nanotechnology-based Cosmeceuticals: a Review. Int. J. Appl. Pharm. 8 (1), 8–12. doi:10.22159/ijap.2016v8i1.10533
Duvvuri, S., Janoria, K. G., and Mitra, A. K. (2005). Development of a Novel Formulation Containing Poly(d,l-Lactide-Co-Glycolide) Microspheres Dispersed in PLGA-PEG-PLGA Gel for Sustained Delivery of Ganciclovir. J. Control. Release 108 (2-3), 282–293. doi:10.1016/j.jconrel.2005.09.002
Duvvuri, S., Gaurav Janoria, K., and Mitra, A. K. (2006). Effect of Polymer Blending on the Release of Ganciclovir from PLGA Microspheres. Pharm. Res. 23 (1), 215–223. doi:10.1007/s11095-005-9042-6
Emam, H. E., and Ahmed, H. B. (2019). Comparative Study between Homo-Metallic & Hetero-Metallic Nanostructures Based agar in Catalytic Degradation of Dyes. Int. J. Biol. macromolecules 138, 450–461. doi:10.1016/j.ijbiomac.2019.07.098
Emam, H. E., El-Shahat, M., and Abdelhameed, R. M. (2021). Observable Removal of Pharmaceutical Residues by Highly Porous Photoactive Cellulose Acetate@MIL-MOF Film. J. Hazard. Mater. 414, 125509. doi:10.1016/j.jhazmat.2021.125509
Emam, H. E., Saad, N. M., Abdallah, A. E. M., and Ahmed, H. B. (2020). Acacia Gum versus Pectin in Fabrication of Catalytically Active Palladium Nanoparticles for Dye Discoloration. Int. J. Biol. macromolecules 156, 829–840. doi:10.1016/j.ijbiomac.2020.04.018
Entezar-Almahdi, E., Heidari, R., Ghasemi, S., Mohammadi-Samani, S., and Farjadian, F. (2021). Integrin Receptor Mediated pH-Responsive Nano-Hydrogel Based on Histidine-Modified Poly(aminoethyl Methacrylamide) as Targeted Cisplatin Delivery System. J. Drug Deliv. Sci. Technol. 62, 102402. doi:10.1016/j.jddst.2021.102402
Farjadian, F., Rezaeifard, S., Naeimi, M., Ghasemi, S., Mohammadi-Samani, S., Welland, M. E., et al. (2019). Temperature and pH-Responsive Nano-Hydrogel Drug Delivery System Based on Lysine-Modified Poly (Vinylcaprolactam). Int. J. Nanomedicine 14, 6901–6915. doi:10.2147/ijn.s214467
Farzanfar, J., Farjadian, F., Roointan, A., Mohammadi-Samani, S., and Tayebi, L. (2021). Assessment of pH Responsive Delivery of Methotrexate Based on PHEMA-St-PEG-DA Nanohydrogels. Macromol. Res. 29 (1), 54–61. doi:10.1007/s13233-021-9007-6
Freag, M. S., Saleh, W. M., and Abdallah, O. Y. (2018). Exploiting Polymer Blending Approach for Fabrication of Buccal Chitosan-Based Composite Sponges with Augmented Mucoadhesive Characteristics. Eur. J. Pharm. Sci. 120, 10–19. doi:10.1016/j.ejps.2018.04.041
Ghasemiyeh, P., Azadi, A., Daneshamouz, S., and Mohammadi Samani, S. (2017). Cyproterone Acetate-Loaded Solid Lipid Nanoparticles (SLNs) and Nanostructured Lipid Carriers (NLCs): Preparation and Optimization. Trends Pharm. Sci. 3 (4), 275–286.
Ghasemiyeh, P., and Mohammadi-Samani, S. (2019). Hydrogels as Drug Delivery Systems; Pros and Cons. Trends Pharm. Sci. 5 (1), 7–24. doi:10.30476/TIPS.2019.81604.1002
Ghasemiyeh, P., and Mohammadi-Samani, S. (2018). Solid Lipid Nanoparticles and Nanostructured Lipid Carriers as Novel Drug Delivery Systems: Applications, Advantages and Disadvantages. Res. Pharm. Sci. 13 (4), 288–303. doi:10.4103/1735-5362.235156
Ghasemiyeh, P., Azadi, A., Daneshamouz, S., Heidari, R., Azarpira, N., and Mohammadi-Samani, S. (2019). Cyproterone Acetate-Loaded Nanostructured Lipid Carriers: Effect of Particle Size on Skin Penetration and Follicular Targeting. Pharm. Dev. Technol. 24 (7), 812–823. doi:10.1080/10837450.2019.1596133
Ghasemiyeh, P., and Mohammadi-Samani, S. (2020). Potential of Nanoparticles as Permeation Enhancers and Targeted Delivery Options for Skin: Advantages and Disadvantages. Drug Des. Devel Ther. 14, 3271–3289. doi:10.2147/dddt.s264648
Guo, B., and Ma, P. X. (2018). Conducting Polymers for Tissue Engineering. Biomacromolecules 19 (6), 1764–1782. doi:10.1021/acs.biomac.8b00276
Hamoudi-Ben Yelles, M. C., Tran Tan, V., Danede, F., Willart, J. F., and Siepmann, J. (2017). PLGA Implants: How Poloxamer/PEO Addition Slows Down or Accelerates Polymer Degradation and Drug Release. J. Controlled Release 253, 19–29. doi:10.1016/j.jconrel.2017.03.009
Hillmyer, M. (1999). Block Copolymer Synthesis. Curr. Opin. Solid State. Mater. Sci. 4 (6), 559–564. doi:10.1016/s1359-0286(00)00006-1
Hussain, M., Xie, J., Hou, Z., Shezad, K., Xu, J., Wang, K., et al. (2017). Regulation of Drug Release by Tuning Surface Textures of Biodegradable Polymer Microparticles. ACS Appl. Mater. Inter. 9 (16), 14391–14400. doi:10.1021/acsami.7b02002
Jones, C. D., and Lyon, L. A. (2000). Synthesis and Characterization of Multiresponsive Core−Shell Microgels. Macromolecules 33 (22), 8301–8306. doi:10.1021/ma001398m
Jones, S. A., Martin, G. P., Royall, P. G., and Brown, M. B. (2005). Biocompatible Polymer Blends: Effects of Physical Processing on the Molecular Interaction of Poly(vinyl Alcohol) and Poly(vinyl Pyrrolidone). J. Appl. Polym. Sci. 98 (5), 2290–2299. doi:10.1002/app.22390
Keogh, R., Blackman, L. D., Foster, J. C., Varlas, S., and O'Reilly, R. K. (2020). The Importance of Cooperativity in Polymer Blending: Toward Controlling the Thermoresponsive Behavior of Blended Block Copolymer Micelles. Macromol. Rapid Commun. 41 (6), 1900599. doi:10.1002/marc.201900599
Klouda, L. (2015). Thermoresponsive Hydrogels in Biomedical Applications. Eur. J. Pharmaceutics Biopharmaceutics 97, 338–349. doi:10.1016/j.ejpb.2015.05.017
Kolhe, P., and Kannan, R. M. (2003). Improvement in Ductility of Chitosan through Blending and Copolymerization with PEG: FTIR Investigation of Molecular Interactions. Biomacromolecules 4 (1), 173–180. doi:10.1021/bm025689+
Kong, T., Wu, J., Yeung, K. W. K., To, M. K. T., Wang, L., and Wang, L. (2013). Microfluidic Fabrication of Polymeric Core-Shell Microspheres for Controlled Release Applications. Biomicrofluidics 7 (4), 044128. doi:10.1063/1.4819274
Langer, R. (1993). Polymer-controlled Drug Delivery Systems. Acc. Chem. Res. 26 (10), 537–542. doi:10.1021/ar00034a004
Lecomte, F., Siepmann, J., Walther, M., MacRae, R. J., and Bodmeier, R. (2003). Blends of Enteric and GIT-Insoluble Polymers Used for Film Coating: Physicochemical Characterization and Drug Release Patterns. J. controlled release 89 (3), 457–471. doi:10.1016/s0168-3659(03)00155-x
Liechty, W. B., Kryscio, D. R., Slaughter, B. V., and Peppas, N. A. (2010). Polymers for Drug Delivery Systems. Annu. Rev. Chem. Biomol. Eng. 1, 149–173. doi:10.1146/annurev-chembioeng-073009-100847
Liu, J., Lin, S., Li, L., and Liu, E. (2005). Release of Theophylline from Polymer Blend Hydrogels. Int. J. Pharmaceutics 298 (1), 117–125. doi:10.1016/j.ijpharm.2005.04.006
Lu, K.-Y., Lin, Y.-C., Lu, H.-T., Ho, Y.-C., Weng, S.-C., Tsai, M.-L., et al. (2019). A Novel Injectable In Situ Forming Gel Based on Carboxymethyl Hexanoyl Chitosan/hyaluronic Acid Polymer Blending for Sustained Release of Berberine. Carbohydr. Polym. 206, 664–673. doi:10.1016/j.carbpol.2018.11.050
Marks, J. A., Wegiel, L. A., Taylor, L. S., and Edgar, K. J. (2014). Pairwise Polymer Blends for Oral Drug Delivery. J. Pharm. Sci. 103 (9), 2871–2883. doi:10.1002/jps.23991
Mohammadi, F., Mohammadi Samani, S., Tanideh, N., and Ahmadi, F. (2018). Hybrid Scaffolds of Hyaluronic Acid and Collagen Loaded with Prednisolone: An Interesting System for Osteoarthritis. Adv. Pharm. Bull. 8 (1), 11–19. doi:10.15171/apb.2018.002
Mohammadi-Samani, S., and Taghipour, B. (2015). PLGA Micro and Nanoparticles in Delivery of Peptides and Proteins; Problems and Approaches. Pharm. Dev. Technol. 20 (4), 385–393. doi:10.3109/10837450.2014.882940
Monfared, M., Taghizadeh, S., Zare-Hoseinabadi, A., Mousavi, S. M., Hashemi, S. A., Ranjbar, S., et al. (2019). Emerging Frontiers in Drug Release Control by Core-Shell Nanofibers: a Review. Drug Metab. Rev. 51 (4), 589–611. doi:10.1080/03602532.2019.1642912
Naseem, K., Begum, R., Wu, W., Irfan, A., and Farooqi, Z. H. (2018). Advancement in Multi-Functional Poly(styrene)-Poly(N-Isopropylacrylamide) Based Core-Shell Microgels and Their Applications. Polym. Rev. 58 (2), 288–325. doi:10.1080/15583724.2017.1423326
Ojah, N., Saikia, D., Gogoi, D., Baishya, P., Ahmed, G. A., Ramteke, A., et al. (2019). Surface Modification of Core-Shell Silk/PVA Nanofibers by Oxygen Dielectric Barrier Discharge Plasma: Studies of Physico-Chemical Properties and Drug Release Behavior. Appl. Surf. Sci. 475, 219–229. doi:10.1016/j.apsusc.2018.12.270
Perinelli, D. R., Bonacucina, G., Pucciarelli, S., Cespi, M., Casettari, L., Polzonetti, V., et al. (2014). Could Albumin Affect the Self-Assembling Properties of a Block Co-polymer System and Drug Release? an In-Vitro Study. Pharm. Res. 32 (3), 1094–1104. doi:10.1007/s11095-014-1521-1
Pillai, O., and Panchagnula, R. (2001). Polymers in Drug Delivery. Curr. Opin. Chem. Biol. 5 (4), 447–451. doi:10.1016/s1367-5931(00)00227-1
Roointan, A., Farzanfar, J., Mohammadi-Samani, S., Behzad-Behbahani, A., and Farjadian, F. (2018). Smart pH Responsive Drug Delivery System Based on poly(HEMA-Co-DMAEMA) Nanohydrogel. Int. J. Pharm. 552 (1-2), 301–311. doi:10.1016/j.ijpharm.2018.10.001
Roointan, A., Kianpour, S., Memari, F., Gandomani, M., Gheibi Hayat, S. M., and Mohammadi-Samani, S. (2018). Poly(lactic-co-glycolic Acid): The Most Ardent and Flexible Candidate in Biomedicine!. Int. J. Polymeric Mater. Polymeric Biomater. 67 (17), 1028–1049. doi:10.1080/00914037.2017.1405350
Salmanpour, M., Yousefi, G., Samani, S. M., Mohammadi, S., Anbardar, M. H., and Tamaddon, A. (2019). Nanoparticulate Delivery of Irinotecan Active Metabolite (SN38) in Murine Colorectal Carcinoma through Conjugation to Poly (2-ethyl 2-Oxazoline)-B-Poly (L-Glutamic Acid) Double Hydrophilic Copolymer. Eur. J. Pharm. Sci. 136, 104941. doi:10.1016/j.ejps.2019.05.019
Samani, S. M., Montaseri, H., and Kazemi, A. (2003). The Effect of Polymer Blends on Release Profiles of Diclofenac Sodium from Matrices. Eur. J. pharmaceutics biopharmaceutics 55 (3), 351–355. doi:10.1016/s0939-6411(03)00030-4
Sionkowska, A. (2011). Current Research on the Blends of Natural and Synthetic Polymers as New Biomaterials: Review. Prog. Polym. Sci. 36 (9), 1254–1276. doi:10.1016/j.progpolymsci.2011.05.003
Sionkowska, A., Wisniewski, M., Skopinska, J., Kennedy, C. J., and Wess, T. J. (2004). Molecular Interactions in Collagen and Chitosan Blends. Biomaterials 25 (5), 795–801. doi:10.1016/s0142-9612(03)00595-7
Sohrabi, A., Shaibani, P. M., Etayash, H., Kaur, K., and Thundat, T. (2013). Sustained Drug Release and Antibacterial Activity of Ampicillin Incorporated Poly(methyl Methacrylate)-Nylon6 Core/shell Nanofibers. Polymer 54 (11), 2699–2705. doi:10.1016/j.polymer.2013.03.046
Srikar, R., Yarin, A. L., Megaridis, C. M., Bazilevsky, A. V., and Kelley, E. (2008). Desorption-limited Mechanism of Release from Polymer Nanofibers. Langmuir 24 (3), 965–974. doi:10.1021/la702449k
Srivastava, A., Yadav, T., Sharma, S., Nayak, A., Kumari, A. A., and Mishra, N. (2015). Polymers in Drug Delivery. J. Biosciences Medicines 4 (1), 69–84. doi:10.4236/jbm.2016.41009
Taghipour, B., Yakhchali, M., Haririan, I., Tamaddon, A. M., and Samani, S. M. (2014). The Effects of Technical and Compositional Variables on the Size and Release Profile of Bovine Serum Albumin from PLGA Based Particulate Systems. Res. Pharm. Sci. 9 (6), 407–420.
Tiwari, S. K., Tzezana, R., Zussman, E., and Venkatraman, S. S. (2010). Optimizing Partition-Controlled Drug Release from Electrospun Core-Shell Fibers. Int. J. Pharm. 392 (1-2), 209–217. doi:10.1016/j.ijpharm.2010.03.021
Tran, T. H., Ramasamy, T., Choi, J. Y., Nguyen, H. T., Pham, T. T., Jeong, J. H., et al. (2015). Tumor-targeting, pH-Sensitive Nanoparticles for Docetaxel Delivery to Drug-Resistant Cancer Cells. Int. J. Nanomedicine 10, 5249–5262. doi:10.2147/IJN.S89584
Vaidya, U. R., and Bhattacharya, M. (1994). Properties of Blends of Starch and Synthetic Polymers Containing Anhydride Groups. J. Appl. Polym. Sci. 52 (5), 617–628. doi:10.1002/app.1994.070520505
Vashisth, P., Singh, R. P., and Pruthi, V. (2016). A Controlled Release System for Quercetin from Biodegradable Poly(lactide-Co-Glycolide)-Polycaprolactone Nanofibers and its In Vitro Antitumor Activity. J. Bioactive Compatible Polym. 31 (3), 260–272. doi:10.1177/0883911515613098
Wang, C., Han, W., Tang, X., and Zhang, H. (2012). Evaluation of Drug Release Profile from Patches Based on Styrene-Isoprene-Styrene Block Copolymer: The Effect of Block Structure and Plasticizer. Aaps Pharmscitech 13 (2), 556–567. doi:10.1208/s12249-012-9778-3
Ward, M. A., and Georgiou, T. K. (2011). Thermoresponsive Polymers for Biomedical Applications. Polymers 3 (3), 1215–1242. doi:10.3390/polym3031215
Wu, T., Ding, M., Shi, C., Qiao, Y., Wang, P., Qiao, R., et al. (2020). Resorbable Polymer Electrospun Nanofibers: History, Shapes and Application for Tissue Engineering. Chin. Chem. Lett. 31 (3), 617–625. doi:10.1016/j.cclet.2019.07.033
Xiao, X.-C., Chu, L.-Y., Chen, W.-M., Wang, S., and Xie, R. (2004). Preparation of Submicrometer-Sized Monodispersed Thermoresponsive Core−Shell Hydrogel Microspheres. Langmuir 20 (13), 5247–5253. doi:10.1021/la036230j
Yin, W., Ke, W., Lu, N., Wang, Y., Japir, A. A.-W. M. M., Mohammed, F., et al. (2020). Glutathione and Reactive Oxygen Species Dual-Responsive Block Copolymer Prodrugs for Boosting Tumor Site-specific Drug Release and Enhanced Antitumor Efficacy. Biomacromolecules 21 (2), 921–929. doi:10.1021/acs.biomac.9b01578
Zhang, H., Chang, L., Zhang, B., Li, P., Wang, M., Yao, K. D., et al. (2008). Synthesis and Characterization of Microparticles Made of Carboxymethyloxysuccinic-Acid-Modified PLA-PEG Co-polymer. J. Biomater. Sci. Polym. Edition 19 (1), 99–111. doi:10.1163/156856208783227677
Zhang, L. (2007). Thermally-responsive Core-Shell Particles. Bethlehem, Pennsylvania: Lehigh University.
Zhang, Z., Tsai, P.-C., Ramezanli, T., and Michniak-Kohn, B. B. (2013). Polymeric Nanoparticles-Based Topical Delivery Systems for the Treatment of Dermatological Diseases. WIREs Nanomed Nanobiotechnol 5 (3), 205–218. doi:10.1002/wnan.1211
Zupančič, Š. (2019). Core-shell Nanofibers as Drug Delivery Systems. Acta Pharm. 69 (2), 131–153. doi:10.2478/acph-2019-0014
Keywords: polymers blending, core-shell polymeric carriers, block copolymers, physical mixtures, release profile, drug delivery
Citation: Ghasemiyeh P and Mohammadi-Samani S (2021) Polymers Blending as Release Modulating Tool in Drug Delivery. Front. Mater. 8:752813. doi: 10.3389/fmats.2021.752813
Received: 03 August 2021; Accepted: 22 October 2021;
Published: 09 November 2021.
Edited by:
Nafisa Gull, University of the Punjab, PakistanReviewed by:
Hossam Elsayed Emam, National Research Centre, EgyptShahzad Sharif, Government College University, Lahore, Pakistan
Copyright © 2021 Ghasemiyeh and Mohammadi-Samani. This is an open-access article distributed under the terms of the Creative Commons Attribution License (CC BY). The use, distribution or reproduction in other forums is permitted, provided the original author(s) and the copyright owner(s) are credited and that the original publication in this journal is cited, in accordance with accepted academic practice. No use, distribution or reproduction is permitted which does not comply with these terms.
*Correspondence: Soliman Mohammadi-Samani, c21zYW1hbmlAc3Vtcy5hYy5pcg==
†ORCID: Parisa Ghasemiyeh, orcid.org/0000-0002-8640-5724; Soliman Mohammadi-Samani, orcid.org/0000-0002-1007-1422