- 1Research Institute, Nozaki Tokushukai Hospital, Osaka, Japan
- 2Radioisotope Research Center, Division of Biochemical Engineering, Kyoto University, Kyoto, Japan
Mechanical stimulation by the extracellular matrix (ECM) controls physiological and pathological cellular responses, such as stem cell differentiation, organogenesis, and tumor progression. Polyacrylamide (PA) gels have been widely used to study cell-ECM mechanical interactions. Typically, sulfosuccinimidyl 6-(4′-azido-2′-nitrophenylamino)hexanoate (sulfo-SANPAH) is used as a protein crosslinker in these gels. However, its low solubility, unstable binding with proteins, and high cost are barriers to its application. The objective of this study was to improve and simplify the preparation of PA gels using an economical crosslinker, N-hydroxysuccinimide-acrylamide (NHS-AA) ester, to enable increased stability in protein coating. By exposing excess NHS to the gel surface, we found an optimal ratio of NHS-AA ester:AA to obtain NHS-AA ester-containing PA gels with a uniform ECM protein coating and stiffness similar to that of sulfo-SANPAH-containing PA gels. The biological behavior of MCF7 and MCF10A cells were similar on NHS-AA ester and sulfo-SANPAH gels. Acini formation in Matrigel overlay culture were also consistent on NHS-AA ester and sulfo-SANPAH gels. This novel PA gel preparation method using NHS-AA ester can effectively replace the sulfo-SANPAH method and will be immensely useful in the evaluation of cell-ECM mechanical interactions.
Introduction
Mechanical stimulation by the extracellular matrix (ECM) controls physiological and pathological cellular responses. In regenerative medicine, optimal stiffness of the culture substrate increases the efficiency of induction of differentiation and establishment of three-dimensional (3D) culture systems for organ regeneration (Engler et al., 2006; Kolahi et al., 2012). In cancer biology, a relationship has been demonstrated between mammary tissue stiffness and breast cancer progression (Paszek and Weaver, 2004; Paszek et al., 2005; Levental et al., 2007, 2009; Butcher et al., 2009).
Various cell culture materials are used to investigate the effects of ECM stiffness on cellular responses, including biomaterials such as collagen (Parenteau-Bareil et al., 2010), Matrigel (Kleinman et al., 1986), polysaccharide (Baldwin and Kiick, 2010), polyacrylamide (PA) (Kandow et al., 2007), polyethylene glycol (PEG) (Zhu, 2010), and self-assembling peptides (Koutsopoulos, 2016). Among them, PA gels are most widely used to study cell-ECM mechanical interactions, because of their convenient usage, biocompatibility, and reproducibility of stiffness (Kandow et al., 2007; Tilghman et al., 2010; Dupont et al., 2011; Wen et al., 2014; Tsou et al., 2016; Domura et al., 2017; Martín et al., 2017).
PA gels require protein crosslinkers to crosslink ECM proteins to the gel for cell adhesion. Sulfosuccinimidyl 6-(4′-azido-2′-nitrophenylamino)hexanoate (sulfo-SANPAH) is a commonly used PA gel crosslinker (Pelham and Wang, 1997). ECM proteins displace the sulfosuccinimidyl groups of sulfo-SANPAH molecules, forming amide bonds (Figure 1A). However, ECM protein binding by sulfo-SANPAH is unstable and can result in inconsistent effects of gel stiffness on cellular responses (Kandow et al., 2007; Yip et al., 2013). This is presumably due to the nonspecific binding of sulfo-SANPAH to the PA gel through ultraviolet (UV) irradiation (Figure 1B, top). In addition, the low solubility and high cost of sulfo-SANPAH can hinder experiments that require large amounts of PA gel (Pelham and Wang, 1997; Kandow et al., 2007). To improve the stability of protein bound to PA gels, several alternative methods have been developed, which attach ECM proteins to the gels by covalent bonds using 1-ethyl-3-(3-dimethylaminopropyl)carbodiimide (Beningo and Wang, 2002; Kandow et al., 2007), N-acryloyl-6-aminocaproic acid (ACA) (Yip et al., 2013), or the N-succinimidyl ester of acrylamidohexanoic acid (N6; Table 1) (Willcox et al., 2005; Kandow et al., 2007). However, these crosslinkers have not been widely accepted as sulfo-SANPAH alternatives because they require long reaction times or are commercially unavailable.
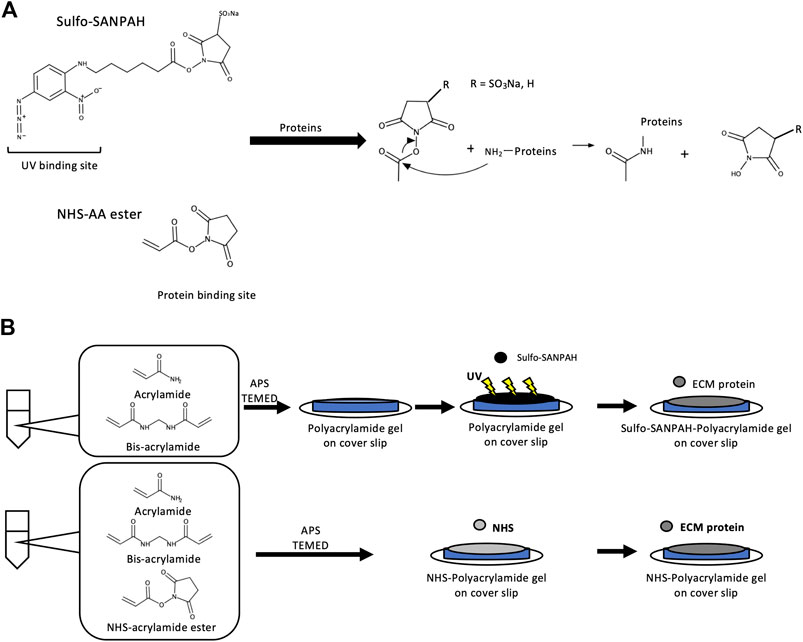
FIGURE 1. Overview of protein crosslinker action and PA gel preparation (A) When sulfo-SANPAH is used as a protein crosslinker, the ECM protein forms an amide bond with the reactive site of sulfo-SANPAH and indirectly binds to PA. When NHS-AA ester is used as a protein crosslinker, the ECM protein forms an amide bond directly with PA. (B) Outline of the PA gel preparation method. When sulfo-SANPAH is used as a protein crosslinker, 0.5 mL of sulfo-SANPAH solution (0.2 mg/mL) was applied to the gel surface under 365 nm UV light after polymerization of AA and bis-AA (top). When NHS-AA ester is used as a protein crosslinker, it is mixed with AA and bis-AA before polymerization (bottom).
NHS has a long history of use as an easy-to-dissolve and economical protein crosslinker and is used in the ACA and N6 protocols to initiate substitution reactions with ECM proteins. However, direct conjugation of NHS to acrylamide (AA) would simplify the protocol, as ECM proteins could be covalently bound directly to AA through a nucleophilic acyl substitution reaction (Figure 1A, bottom). Only one study has reported a protocol for the preparation method of PA gels using NHS, in which NHS was mixed with an AA/bis-AA mixture before polymerization (Cretu et al., 2010). However, this protocol is practically difficult, because NHS binding to AA requires esterification of the amide group, which only occurs under nonphysiological conditions involving strong acids or bases in high temperatures (Greenberg et al., 2000). NHS-AA ester is a commercially available alternative that avoids this problem. NHS-AA ester, dissolved in toluene, is applied to an AA/bis-AA mixture prior to polymerization (Schnaar and Lee, 1975; Schnaar et al., 1978; Kandow et al., 2007); however, it is difficult to uniformly distribute NHS on the gel surface by pouring liquid on liquid, and thus impractical. A practical method would require mixing NHS-AA ester with AA and bis-AA before polymerization (Figure 1B, bottom).
In this study, we developed a new preparation method for PA gels using NHS-AA ester, and demonstrated its utility in studying the effects of ECM stiffness on cellular behavior. We optimized the ratios of NHS-AA ester and AA to achieve similar stiffness to sulfo-SANPAH gels and accomplished uniform ECM protein coating efficacy on gels on various stiffness. In addition, we compared cellular behaviors on gels containing NHS-AA ester and sulfo-SANPAH. The results suggest that NHS-AA ester-containing PA gels can be used as an inexpensive and reproducible alternative to sulfo-SANPAH-containing gels.
Materials and Methods
Cell Culture
MCF7 cells were obtained from the Health Science Research Resources Bank (Osaka, Japan). The cells were maintained in Dulbecco’s modified Eagle’s medium (DMEM) containing 10% fetal bovine serum, 100 U/mL penicillin, and 100 μg/mL streptomycin (Fujifilm Wako Pure Chemical, Osaka, Japan). MCF10A cells were obtained from American Type Culture Collection (Manassas, VA, United States), and maintained in DMEM/F12 (Nakarai Tesque Inc., Kyoto, Japan) supplemented with 20 ng/mL of epidermal growth factor (EGF) (Peptide Institute Inc., Osaka, Japan), 100 ng/mL of cholera toxin (Sigma, St. Louis, MO, United States), 0.01 mg/mL of insulin (Sigma), 500 ng/mL of hydrocortisone (Fujifilm Wako Pure Chemical), 5% horse serum (Thermo Fisher Scientific, Waltham, MA, United States), 100 U/mL penicillin, and 100 μg/mL streptomycin (Debnath et al., 2003). All cells were maintained at 37°C in a humidified 5% CO2/95% air atmosphere.
Antibodies
Anti-p-FAK antibodies (Tyr397; ab81298), Alexa Fluor® 488 Donkey Anti-Rabbit IgG (ab150062), and Alexa Fluor® 488 Goat Anti-Mouse IgG (ab150113) were purchased from Abcam (Cambridge, United Kingdom). Anti-YAP antibodies (sc-101199) were purchased from Santa Cruz Biotechnology, Inc. (Dallas, TX, United States).
Preparation of Aminosilanated (Bottom) and Chlorosilanated (Top) Coverslips
For the bottom coverslips, 1 mL of 0.1 M NaOH (Fujifilm Wako Pure Chemical) was applied to a 22 mm coverslip (Matsunami Glass Ind., Ltd., Osaka, Japan) for 3 min to increase their reactivity with aminosilane. The NaOH was aspirated, and the coverslips were dried. Next, 300 μL of 3-aminopropyltriethoxysilane (APTES; Tokyo Chemical Industry Co., Ltd, Tokyo, Japan) was applied to the coverslip for 3 min. Then, the solution was aspirated, and the coverslip was washed three times with deionized water (DW) for 10 min each, dried, and 500 μL of 0.5% glutaraldehyde (Tokyo Chemical Industry Co., Ltd) was applied for 30 min to activate the APTES. After incubation, the solution was aspirated, and the coverslip was washed three times with DW for 10 min each. The dried coverslips could be stored at room temperature (RT) for several days. For the top coverslips, 120 μL of dichlorodimethylsilane (DCDMS) (Tokyo Chemical Industry Co., Ltd) was added to the coverslip and allowed to react for 5 min. Then, the solution was aspirated and the coverslip was washed with DW for 1 min.
Preparation of PA Gel Containing NHS-AA Ester
The amounts of NHS-AA ester and AA used were as previously described (Tse and Engler, 2010). We prepared the gel by mixing 40% AA (Fujifilm Wako Pure Chemical) and 1% bis-AA (Bio-Rad Laboratories, Inc., Hercules, CA, United States) in Milli-Q water and 20% NHS-AA ester (Tokyo Chemical Industry Co., Ltd.) in toluene (Table 2). The NHS-AA mixture was incubated for 5 min to transfer NHS-AA ester from the organic phase to the aqueous phase. After incubation, the solution was centrifuged for 5 min at 500 g and the toluene was removed. The soft gel solutions were degassed for 30 min in vacuum. To activate polymerization, 10 μL of 10% ammonium persulfate (APS) and 1 μL of tetramethylethylenediamine (TEMED) (1% and 0.1% of total volume, respectively) (both from Fujifilm Wako Pure Chemical) were added and briefly mixed. Then, 25 μL of the mixture was placed on a bottom coverslip and covered with a top coverslip. The sandwiched gels were incubated for 30 min (PA1), 20 min (PA2 and PA3), and 10 min (PA4) at RT, and then the top coverslips were removed. The polymerized gels were transferred to 6-well plates and washed three times for 5 min with 2 mL of phosphate-buffered saline (PBS) to remove unreacted AA. Gels were incubated with coating proteins overnight at 4°C. Unreacted NHS in the wells was blocked with 0.1% bovine serum albumin (BSA, Fujifilm Wako Pure Chemical) in DMEM for 30 min. Preparation process of PA gel containing NHS-AA ester is summarized as below.
(Preparation of bottom coverslips)
1. 1 mL of 0.1 M NaOH Is Applied to Coverslips for 3 min.
2. The NaOH was aspirated, and the coverslips are dried.
3. Next, 300 μL of APTES is applied to the coverslip for 3 min, and then is aspirated.
4. The coverslip is washed three times with DW for 10 min each, and then is dried.
5. 500 μL of 0.5% Glutaraldehyde Is Applied for 30 min.
6. After incubation, the solution is aspirated, and the coverslip is washed three times with DW for 10 min each. (The dried coverslips can be stored at RT for several days.)
(Preparation of top coverslips)
7. 120 μL of DCDMS Is Added to the Coverslip and Allowed to React for 5 min.
8. Then, the solution was aspirated, and the coverslip is washed with DW for 1 min.
(Gel Preparation)
9. Mixing solutions are prepared by mixing 40% AA and 1% bis-AA in Milli-Q water and 20% NHS-AA ester in toluene (Table 2).
10. The Solutions Are Incubated for 5 min.
11. After incubation, the solutions are centrifuged for 5 min at 500 g and then the toluene is removed.
12. The Solutions Are Degassed for 30 min Under Vacuum Conditions.
13. To activate polymerization, 10 μL of 10% APS and 1 μL of TEMED are added and briefly mixed.
14. Then, 25 μL of the mixture is placed on bottom coverslip and coved with a top coverslip.
15. The sandwiched gels are incubated for 30 min for PA1, 20 min for PA2 and PA3, and 10 min for PA4 at RT, and then the top coverslips are removed.
16. The polymerized gels are transferred to 6-well plates and washed three times for 5 min with 2 mL of PBS.
17. The Gels Are Incubated With Coating Proteins Overnight at 4°C.
Preparation of PA Gels Using Sulfo-SANPAH
Gels containing sulfo-SANPAH were prepared to contain equivalent amounts of acryloyl groups in NHS-AA ester gels (Table 2). After polymerization of AA and bis-AA, 0.5 mL of 0.2 mg/mL sulfo-SANPAH (Thermo Fisher Scientific) was applied to the gel surface under 365 nm UV light at RT for 10 min. After the binding reaction with sulfo-SANPAH, the gel was washed three times with 2 mL PBS. These gels were protein-coated similar to NHS-AA esters gels.
Measurement of PA Gel Stiffness by Atomic Force Microscopy (AFM)
The Young’s modulus of elasticity, which represents the stiffness of PA gels, was measured with AFM for each type of gel. The prepared PA gels were washed twice with 2 mL PBS and placed in a 35-mm dish. To measure their actual stiffness, the gels were immersed in PBS and force was measured on a NanoWizard three NanoOptics atomic force microscope (JPK Instruments, Berlin, Germany). Young’s modulus was calculated for each force curve using JPK DP Data Processing Software (JPK Instruments), which uses a Hertzian contact model.
Cloning, Expression, and Purification of EGFP
A cDNA encoding EGFP was amplified by polymerase chain reaction (PCR) (KOD FX Neo; TOYOBO Co., Ltd, Osaka, Japan) using primers containing the BamHI (forward, 5′-cgggatccATGGTGAGCAAGGGCGAGGAGCTG-3′) and the EcoRI (reverse, 5′-cggaattcTTACTTGTACAGCTCGTCCATGCCGAGAGTG-3′) restriction sites of pGEX-6P-1 (GE Healthcare, Chicago, IL, United States). The vector and PCR products were digested with BamHI/EcoRI and ligated together using T4 DNA ligase (TAKARA BIO Inc., Shiga, Japan). After plasmid purification and sequencing, the pGEX-6P-EGFP expression vector was transformed into BL21 (DE3) competent Escherichia coli (TAKARA BIO Inc.). Colonies were inoculated in 2 L of Luria-Bertani broth for large-scale culture, and GST-EGFP expression was induced with 0.5 mM isopropyl β-d-1-thiogalactopyranoside for 4 h. The bacterial pellet was resuspended in 20 mL of lysis buffer (50 mM Tris, 1% TritonX-100, 150 mM NaCl, 5 mM MgCl2, 1 mM DTT, pH 7.5) and disrupted by sonication. Then, the lysate was added to a 50% glutathione Sepharose (Nakarai Tesque Inc.) slurry in 4 mL PBS and incubated at 4°C for 1 h, and then washed with PBS. To elute EGFP, the beads were mixed with 2 mL of elution buffer (50 mM Tris-HCl, 150 mM NaCl, 1 mM EDTA, 1 mM DTT, pH 7.5) containing 360 U of PreScission protease (GE Healthcare) and incubated at 4°C for 5 h. The supernatant was collected and EGFP concentration was measured using a BCA Protein Assay Kit (Thermo Fisher Scientific).
Quantitation of EGFP and Rhodamine-Fibronectin Coating of PA Gels
EGFP and rhodamine-fibronectin (Cytoskeleton Inc., Denver, CO, United States) were conjugated to gels overnight. The gels were then mounted with VECTASHIELD® Antifade Mounting Medium (Novus Biologicals, Littleton, CO, United States) and examined on an Eclipse Ti microscope (Nikon Instruments Inc., Melville, NY, United States) fitted with a Plan Fluor 10× objective lens (numerical aperture (NA) 0.3; Nikon Instruments Inc.). The fluorescent intensities of EGFP and rhodamine-fibronectin were quantified using ImageJ v. 2.0.0-rc-69/1.52p (National Institutes of Health, Bethesda, MD, United States). Fluorescent intensities were calculated as the difference between the average fluorescent intensity of the proteins in each field and the background fluorescent intensity. Fluorescence from five randomly selected fields was measured.
Cell Proliferation and Adhesion Assays
The gels were coated with 100 µg/mL collagen I (Corning Inc, Corning, NY, United States). For proliferation assays, 1.0 × 105 cells were added to each well and incubated at 37°C for 3 days. For cell adhesion assays, 5.0 × 105 cells were added to each well and incubated at 37°C for 1 h. In both assays, the cells were counted using cell counting kit-8 (CCK-8, Dojindo Molecular Technologies, Inc., Kumamoto, Japan). Absorbance was measured at 450 nm using a Sunrise microplate reader (Tecan Japan Co. Ltd., Kawasaki, Japan).
Cell Area and Circularity
Cells were examined on an Eclipse Ti microscope fitted with a Plan Fluor 20× objective lens (NA 0.45; Nikon Instruments Inc.). After binary image processing, the cell area and circularity were measured using ImageJ. Five randomly selected fields were considered.
Immunofluorescence
MCF10A cells (5 × 104) were cultured on collagen-coated PA gels for 24 h. The cells were fixed with 4% paraformaldehyde for 20 min and permeabilized with 1% (for p-FAK) and 0.1% (for YAP) Triton X-100 in PBS for 10 min, and then blocked with 3% BSA in PBS for 30 min at RT. The cells were incubated with 1:50 solution of primary antibodies, for p-FAK and YAP, overnight at 4°C, and then incubated with secondary antibodies and Hoechst 33,342 (Thermo Fisher Scientific) at RT for 30 min. Finally, the cells were mounted with VECTASHIELD® and examined on an Eclipse Ti microscope fitted with a Plan Fluor 20× objective lens (NA 0.45; Nikon Instruments Inc.).
Matrigel Overlay Culture on PA Gel
Matrigel overlay culture on PA gel was performed as previously described, with slight modifications (Debnath et al., 2003). MCF10A cells were detached with trypsin at 37°C for 5 min to ensure complete collection of the cells, and then resuspended in culture medium (DMEM/F12 supplemented with 20 ng/mL of EGF, 100 ng/mL of cholera toxin, 0.01 mg/mL of insulin, 500 ng/mL of hydrocortisone, 5% horse serum, 100 U/mL penicillin, and 100 μg/mL streptomycin). The cells were washed with assay medium (DMEM/F12 supplemented with 100 ng/mL of cholera toxin, 0.01 mg/mL of insulin, 500 ng/mL of hydrocortisone, 2% horse serum, 100 U/mL penicillin and 100 μg/mL streptomycin), and then counted and diluted to 1 × 104 cells/mL in assay medium at RT. We prepared 4% Matrigel solution in assay medium at 4°C. Then, the cells and Matrigel solution were combined in 1:1 ratio. PA gels were transferred to 12-well plates, washed with assay medium three times, and 1 mL of the cell-Matrigel mixture (5 × 103 cells/well) was applied to the PA gel. The cells were refed assay medium containing 2% Matrigel every 4 days. After 10 days, the cells were examined on an Eclipse Ti microscope fitted with a Plan Fluor 20× objective lens (NA 0.45; Nikon Instruments Inc.).
Statistical Analysis
Differences between pairs of groups were analyzed by two-tailed unpaired Student’s t-test. p values <0.05 were considered statistically significant. Error bars in all figures represent the mean of three independent experiments ± the standard deviation (SD). Data for in vitro experiments represent the results of three independent biological replicates.
Results
Determination of the Optimal NHS-AA ester:AA Mixing Ratio
In the development of a new PA gel preparation method, we aimed to create gels that simulate the stiffness of various tissues. We used previously described AA:bis-AA mixing ratios of PA gels using sulfo-SANPAH that resulted in four different stiffness levels (0.48, 1, 3.24, and 34.88 kPa) (Tse and Engler, 2010). We hypothesized that if we use the published amount of bis-AA and ensure that the total amount of acryloyl groups in the gel came from NHS-AA ester and AA together, the stiffness of the NHS-AA ester gels would stay the same as the reference gels, as NHS would be hydrolyzed and dissolved in water. According to this hypothesis, we determined the optimal NHS-AA ester:AA ratio at the four stiffness levels mentioned above.
With the optimal mixing ratio, the ECM proteins should uniformly coat gels of any stiffness when equal amounts of ECM proteins are applied. Excess surface crosslinker permits the saturated binding of a constant amount of ECM protein, and the amount of protein coating the surface is proportional to the amount of applied ECM protein (Beer et al., 2015). Therefore, we aimed to identify a mixing ratio that provided an excess of surface NHS and used it to confirm uniform ECM protein coating of different stiffness gels.
First, we optimized the highest NHS-AA ester:AA ratio that solidified the gel, since NHS-AA ester was presumed to inhibit the polymerization of AA and bis-AA. At a 1:5 ratio, the gel did not adequately solidify (data not shown), whereas polymerization was successful at ratios of 1:6 and lower. We examined the adhesion and proliferation of MCF7 cells on collagen I-coated gels of the softest stiffness (Table 2) at various mixing ratios. Adherent cells were evenly distributed on the gel at a mixing ratio of 1:6. The distribution of adherent cells became uneven (Figure 2A) and the proliferation significantly decreased (Figure 2B) as the proportion of NHS-AA ester decreased. Next, we examined the uniformity of cell adhesion on gels of different stiffness (PA1-4, with PA1 being the softest and PA4 being the stiffest; Table 2) at the mixing ratio of 1:6. Adhesion was uniform on gels of all stiffness levels (Figure 2C), while proliferation was significantly reduced on soft gels compared to stiff gels (Figure 2D). These results suggest that a mixing ratio of 1:6 enables adequate gel solidification and uniform ECM protein coating of gels of various stiffness.
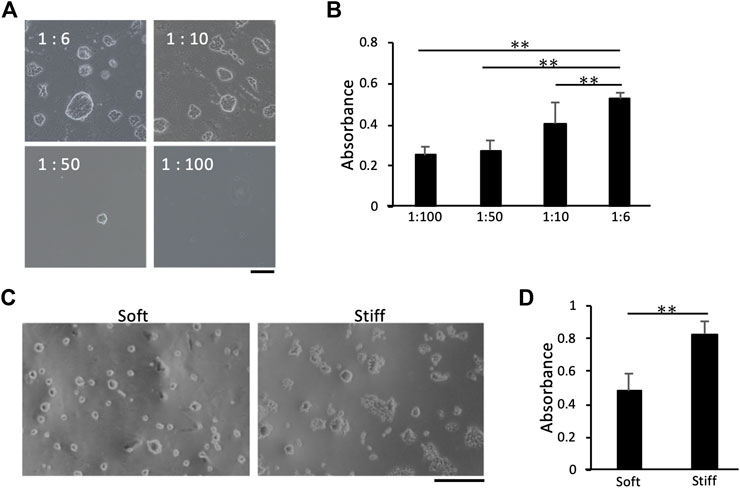
FIGURE 2. Effects of NHS-AA ester:AA mixing ratios on cell proliferation and adhesion. Cell distribution and proliferation increased with a higher mixing ratio of NHS-AA ester:AA (A) Cells adhered to softer gels (estimated stiffness: 0.48 kPa) made with different NHS-AA ester:AA mixing ratios. Scale bar: 100 µm (B) Cell proliferation on gels made with different NHS-AA ester:AA mixing ratios. (C) The distribution of adherent cells was uniform on both soft and stiff gels (estimated stiffness: 0.48 and 34.88 kPa, respectively) at a mixing ratio of 1:6. Scale bar: 500 µm. (D) Cell proliferation rate was higher on stiffer gels than on softer ones with 1:6 mixing ratios. Each value represents the mean of three independent replicates ± the SD. **p < 0.01, *p < 0.05.
Elastic Moduli of PA Gels Made With NHS-AA Ester
We measured the elastic moduli of 1:6 gels of four different stiffness levels (Table 2) by AFM and compared them with reference values (Tse and Engler, 2010). The elastic modulus values of each gel (3.84, 7.98, 20.76, and 142.8 kPa) were approximately three times higher than the estimated values (0.48, 1, 3.24 and 34.88 kPa), but the rates of changes in stiffness between the gels were equivalent to the estimated values (Figure 3).
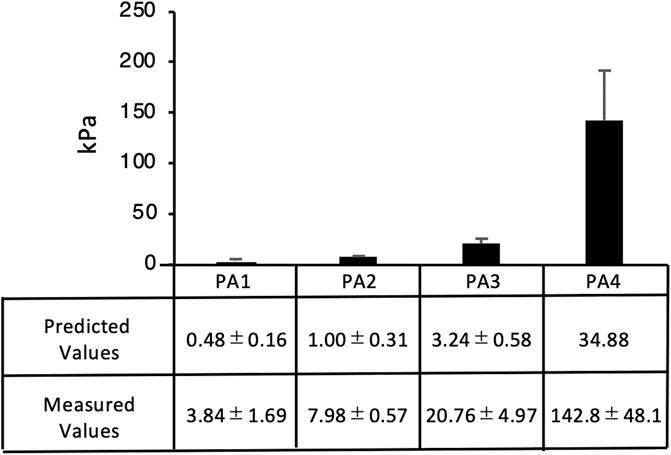
FIGURE 3. Elastic moduli of PA gels. Average elastic modulus values were measured by AFM. The elastic modulus of the four gels (PA1-4) was 3.84, 7.98, 20.76, and 142.8 kPa, respectively. Each value represents the mean of three independent replicates ± the SD.
Confirmation of Protein Coating Efficacy on PA Gels Containing NHS-AA Ester
We examined whether surface NHS was in sufficient excess for uniform ECM protein coating of gels at each stiffness level. The fluorescent intensity of enhanced green fluorescent protein (EGFP) reached saturation on both, the softest and the stiffest gels (PA1 and PA4, respectively) at mixing ratios above 1:8, indicating that excess NHS was present on the gel surface at these ratios (Figure 4A). There were no differences in EGFP coating efficacy among the gels of different stiffness, and the amount of EGFP coating the gels was proportional to the amount applied (Figure 4B). Equivalent ECM protein coating efficacy among gels of different stiffness was also confirmed using rhodamine-fibronectin (Figure 4C). Since the 1:6 mixing ratio was the maximum ratio that displayed equal protein coating efficacy at different stiffness levels, it was used in subsequent experiments.
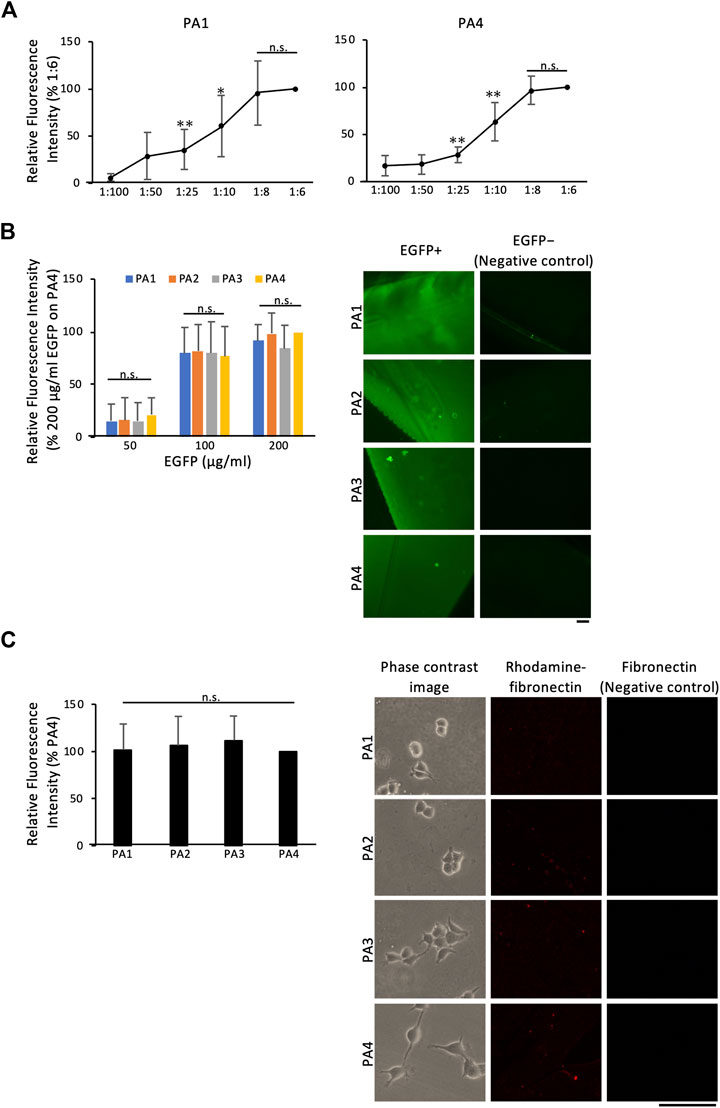
FIGURE 4. Protein coating efficacy of PA gels containing NHS-AA ester. Protein coating efficacy was determined using EGFP and rhodamine-fibronectin (A) The fluorescent intensity of EGFP (200 µg/mL) reached saturation on both soft and stiff gels (PA1 and PA4, respectively) at mixing ratios above 1:8 (B) The amount of EGFP coating the gels was proportional to the amount applied (left), and EGFP coating efficacy was similar among gels of different stiffness (right)(C) Rhodamine-fibronectin coating was similar among gels of different stiffness. The mean of three independent replicates ± the SD (left) are shown. Scale bar: 100 µm. **p < 0.01, *p < 0.05, n.s., not significant.
Comparison of Cell Behavior on PA Gels Containing NHS-AA Ester and Sulfo-SANPAH
To confirm that the NHS-AA ester-containing PA gels could reproduce the results of previous biological experiments conducted with PA gels containing sulfo-SANPAH, we first compared cell adhesion, proliferation, and morphology on gels made with NHS-AA ester and sulfo-SANPAH. There were no significant differences in the adhesion of MCF7 and MCF10A cells on NHS-AA ester and sulfo-SANPAH gels of any stiffness (Figure 5A). However, both cell lines displayed increased proliferation on NHS-AA ester gels than on sulfo-SANPAH gels (Figure 5B). With both crosslinkers, proliferation increased with increase in gel stiffness, while adhesion was unaffected by stiffness (Figures 5A,B). The morphology of MCF7 cells changed from circular to spreading with increasing stiffness, and similar changes observed on gels with either of the crosslinkers (Figure 5C). The cell area (Figure 5D) and circularity (Figure 5E) were similar in both cell lines on both gel types. These results indicate that gels containing NHS-AA ester induce cellular responses comparable to those containing sulfo-SANPAH.
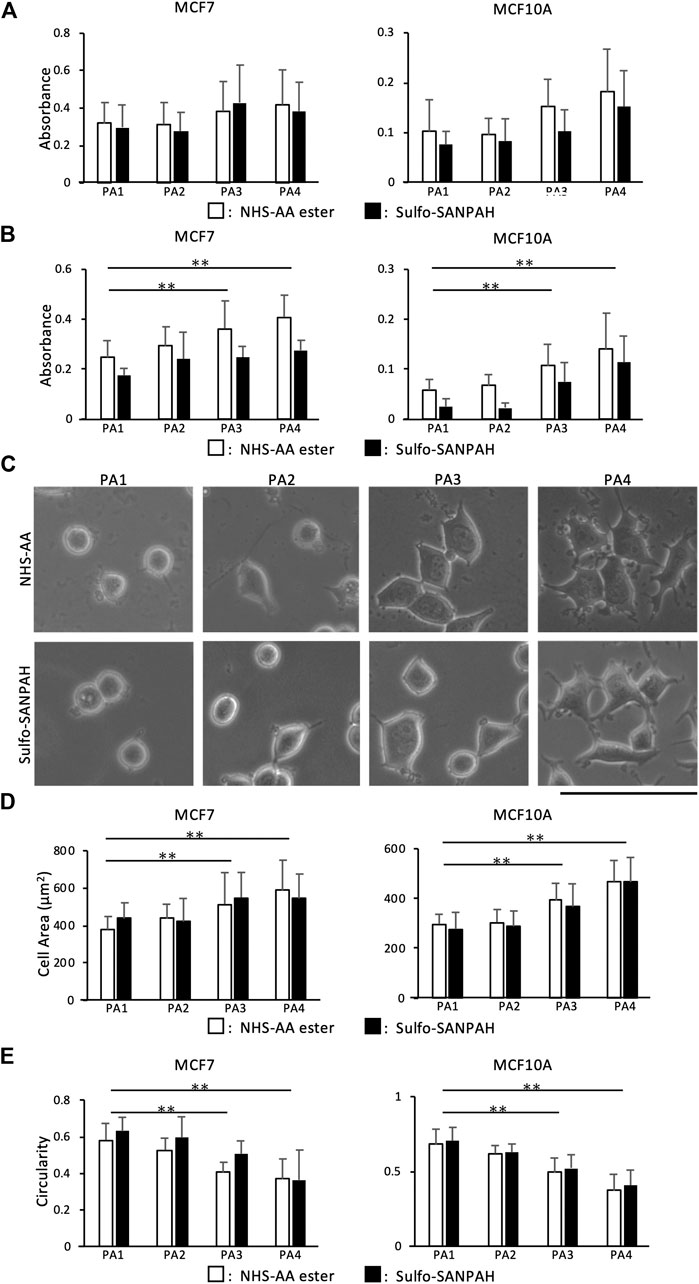
FIGURE 5. Comparison of cell adhesion, proliferation, and morphology on PA gels containing NHS-AA ester and sulfo-SANPAH. (A) Cell adhesion of MCF7 (left) and MCF10A (right) cells showed no difference on gels of different stiffness containing NHS-AA ester (white) and sulfo-SANPAH (black) (B) The proliferation rate of MCF7 (left) and MCF10A (right) cells was higher on NHS-AA ester gels (white) than on sulfo-SANPAH gels (black), irrespective of stiffness. The proliferation increased on stiffer gels (C) No difference in MCF7 cell morphology was observed between the two gels at any stiffness. Scale bar: 100 µm. (D–E) Cell area (D) and circularity (E) of MCF7 (left) and MCF10A (right) cells showed no difference on gels of different stiffness containing NHS-AA ester (white) and sulfo-SANPAH (black). The morphology of both cells changed from circular to spreading with increased stiffness. Values represent the mean of three independent replicates ± the SD. **p < 0.01, *p < 0.05.
Immunostaining and Matrigel Overlay Culture on PA Gels Containing NHS-AA Ester
To evaluate cellular responses to stiffness changes, we immunostained cells adhered to NHS-AA ester-containing PA gels for phosphorylated focal adhesion kinase (p-FAK) and Yes1 associated transcriptional regulator (YAP). In MCF10A cells, focal adhesions stained with p-FAK increased in both size and number on stiff gels than on soft gels (Figure 6A). YAP localized to the cytoplasm of MCF10A cells adhered to soft gels but was translocated to the nucleus in cells on stiff gels (Figure 6B). Furthermore, in Matrigel overlay culture, MCF10A cells formed acini on soft gels and displayed a spread morphology on the surface of stiff gels (Figure 6C). These results indicate that PA gels using NHS-AA ester can reproduce the typical biological behaviors of cells on PA gels using sulfo-SANPAH.
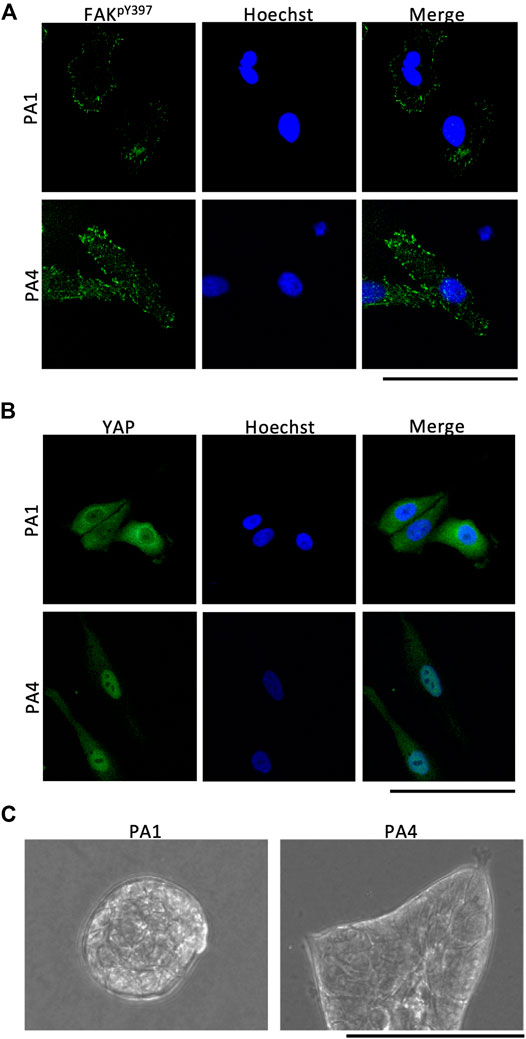
FIGURE 6. Immunostaining and Matrigel overlay culture of PA gels containing NHS-AA ester. (A) Focal adhesions stained with p-FAK increased more in size and number on stiff gels (bottom) than on soft gels (top). Scale bar: 100 µm (B) YAP protein in MCF10A cells was localized in the cytoplasm on soft gels (top) and in the nucleus on stiff gels (bottom). Scale bar: 100 µm. (C) In Matrigel overlay culture, MCF10A cells formed acini on soft gels and spread on stiff gels.
Discussion
In this study, we have developed a new preparation method for PA gels using NHS-AA ester. These gels overcome the disadvantages of sulfo-SANPAH and can be produced for less than 1% the cost of sulfo-SANPAH gels using a simpler preparation procedure than other alternative methods. Due to the excess surface NHS and the covalent nature of the bonds formed, the ECM protein on gels containing NHS-AA ester is uniform and stable, and efficiently transmits the stiffness of gels to cells. Importantly, cellular responses to changes in gel stiffness efficiently replicated those reported for sulfo-SANPAH gels.
With the goal of a simple preparation method, we mixed NHS-AA ester with AA and bis-AA before polymerization. When compared with gels containing sulfo-SANPAH or other alternative protein crosslinkers like ACA or N6, the preparation of PA gels containing NHS-AA ester is simpler. Since it is difficult to have equal number of crosslinking sites on gels of every stiffness, we provided an excess of surface NHS to ensure equivalent ECM protein coating. Therefore, the amounts of NHS contained in gels of each stiffness may be nonuniform, but the excess NHS enables ligand saturation, resulting in uniform ECM protein coating at all stiffness levels. Mixing NHS-AA ester into the gel results in NHS hydrolysis, resulting in negatively charged carboxyl groups that could impair gel durability (Kandow et al., 2007; Lee et al., 2009). However, in our experience, the durability of the gels containing NHS-AA ester was not noticeably different from that of gels containing sulfo-SANPAH.
We estimated that the gel stiffness levels established for sulfo-SANPAH-containing gels would be maintained in NHS-AA ester-containing gels if equal amounts of acryloyl groups were incorporated. However, the elastic modulus values of NHS-AA ester-containing PA gels of respective stiffness were approximately three times the reference values of gels containing sulfo-SANPAH. Nonetheless, the rates of increases in stiffness among the gels were equivalent to the estimated values (Table 2) and cellular responses on gels with NHS-AA ester were similar to those with sulfo-SANPAH. This suggests that the observed differences between the measured and estimated values may be due to differences in the AFM systems used.
Despite their advantages, NHS-AA ester-containing PA gels do have some limitations. ECM proteins must be coated immediately after polymerization, because NHS-AA ester is quickly hydrolyzed, whereas gels for sulfo-SANPAH experiments can be stored for approximately 3 days prior to sulfo-SANPAH conjugation before the stiffness changes (Denisin and Pruitt, 2016). In this study, we used glass coverslips with a diameter of 22 mm, as we found that the surface tension of the acrylamide mixture hindered the generation of larger or smaller gels by the described sandwich method. However, a modified preparation method for smaller scale gels used in drug screening studies has been reported (Medina et al., 2019). To investigate the effects of ECM stiffness on cellular responses, several 3D culture systems have recently been developed (Chaudhuri et al., 2014; Neves et al., 2020; Yamada et al., 2020). Although 3D culture systems are close to the biological environment, they are unable to separate the effects of structure and stiffness on cellular behavior. Thus, the PA gel culture will continue to be a useful experimental system for analyzing the effects of stiffness on cellular behavior.
In this study, we have developed a novel preparation method for PA gels using NHS-AA ester as a protein crosslinker. ECM proteins covalently bind to PA on these gels which efficiently transmit the stiffness of gels to cells. These economical gels are simple to make, highly reproducible, and allow experiments with large number of PA gels. The PA gels using NHS-AA ester is a practical and inexpensive alternative to sulfo-SANPAH gels in the evaluation of cell-ECM mechanical interactions. They may also contribute to advancements in various research areas, such as cancer biology, regenerative medicine, and embryology.
Data Availability Statement
The original contributions presented in the study are included in the article/Supplementary Material, further inquiries can be directed to the corresponding author.
Author Contributions
YY designed the experiments; JK and YY performed the experiments, analyzed data, and wrote the manuscript; MH measured elastic moduli of PA gels with AFM; SS cloned EGFP; all authors reviewed the manuscript.
Funding
This work was supported by JSPS KAKENHI (Grant No. 19K18482 and 18K16641). This funding supported our study design, data collection, analysis and interpretation, and writing the manuscript. This work was also supported by JSPS KAKENHI (Grant No. 18K14063) and the Nanotech Career-up Alliance and the Kyoto University Nano Technology Hub “Nanotechnology Platform Project” sponsored by the Ministry of Education, Culture, Sports, Science and Technology, Japan. It also had an important role in AFM measurement.
Conflict of Interest
The authors declare that the research was conducted in the absence of any commercial or financial relationships that could be construed as a potential conflict of interest.
Acknowledgments
The authors are grateful to Hidemitsu Nakagawa, Tomoyuki Yamaguchi, Toshie Shinagawa, and Masashi Kishi for critical discussion. We would like to thank Nozaki Tokushukai Hospital for financial support and Editage (www.editage.com) for English language editing.
Abbreviations
ECM, extracellular matrix; PA, polyacrylamide; AA, acrylamide; sulfo-SANPAH, sulfosuccinimidyl 6-(4′-azido-2′-nitrophenylamino)hexanoate; NHS-AA: N-hydroxysuccinimide-acrylamide, PEG, polyethylene glycol; UV, ultraviolet; ACA, N-acryloyl-6-aminocaproic acid; N6, N-succinimidyl ester of acrylamidohexanoic acid; EGF, epidermal growth factor; AFM, atomic force microscopy; EGFP, enhanced green fluorescent protein; p-FAK, phosphorylated focal adhesion kinase; YAP, Yes1 associated transcriptional regulator; DMEM, Dulbecco’s modified Eagle’s medium; APTES, 3-aminopropyltriethoxysilane; DCDMS, dichlorodimethylsilane; DW, deionized water; RT, room temperature; PBS, phosphate-buffered saline; BSA, bovine serum albumin; PCR, polymerase chain reaction; SD, standard deviation; 3D, three-dimensional; MCF7 and MCF10A, Michigan Cancer Foundation 7 and 10A, respectively.
References
Baldwin, A. D., and Kiick, K. L. (2010). Polysaccharide-modified synthetic polymeric biomaterials. Biopolymers. 94, 128–140. doi:10.1002/bip.21334
Beer, M. V., Hahn, K., Diederichs, S., Fabry, M., Singh, S., Spencer, S. J., et al. (2015). Quantifying ligand-cell interactions and determination of the surface concentrations of ligands on hydrogel films: the measurement challenge. Biointerphases, 10, 021007. doi:10.1116/1.4919015
Beningo, K. A., and Wang, Y. (2002). Fc-receptor-mediated phagocytosis is regulated by mechanical properties of the target. J. Cell Sci. 115, 849–856.
Butcher, D. T., Alliston, T., and Weaver, V. M. (2009). A tense situation: forcing tumour progression. Nat. Rev. Cancer. 9, 108–122. doi:10.1038/nrc2544
Chaudhuri, O., Koshy, S. T., Branco Da Cunha, C., Shin, J. W., Verbeke, C. S., Allison, K. H., et al. (2014). Extracellular matrix stiffness and composition jointly regulate the induction of malignant phenotypes in mammary epithelium. Nat. Mater. 13, 970–978. doi:10.1038/nmat4009
Cretu, A., Castagnino, P., and Assoian, R. (2010). Studying the effects of matrix stiffness on cellular function using acrylamide-based hydrogels. J. Vis. Exp. 10 (42), 2089. doi:10.3791/2089
Debnath, J., Muthuswamy, S. K., and Brugge, J. S. (2003). Morphogenesis and oncogenesis of MCF-10A mammary epithelial acini grown in three-dimensional basement membrane cultures. Methods. 30, 256–268. doi:10.1016/S1046-2023(03)00032-X
Denisin, A. K., and Pruitt, B. L. (2016). Tuning the range of polyacrylamide gel stiffness for mechanobiology applications. ACS Appl. Mater. Interfaces. 8, 21893–21902. doi:10.1021/acsami.5b09344
Domura, R., Sasaki, R., Ishikawa, Y., and Okamoto, M. (2017). Cellular morphology-mediated proliferation and drug sensitivity of breast cancer cells. J. Funct. Biomater. 8, 18. doi:10.3390/jfb8020018
Dupont, S., Morsut, L., Aragona, M., Enzo, E., Giulitti, S., Cordenonsi, M., et al. (2011). Role of YAP/TAZ in mechanotransduction. Nature. 474, 179–183. doi:10.1038/nature10137
Engler, A. J., Sen, S., Sweeney, H. L., and Discher, D. E. (2006). Matrix elasticity directs stem cell lineage specification. Cell. 126, 677–689. doi:10.1016/j.cell.2006.06.044
A. Greenberg, C. M. Breneman, and J. F. Liebman (Editors) (2000). The amide linkage: structural significance in chemistry, biochemistry, and materials science. Hoboken, NJ: John Wiley and Sons.
Kandow, C. E., Georges, P. C., Janmey, P. A., and Beningo, K. A. (2007). Polyacrylamide hydrogels for cell mechanics: steps toward optimization and alternative uses. Methods Cell Biol. 83, 29–46. doi:10.1016/S0091-679X(07)83002-0
Kleinman, H. K., Mcgarvey, M. L., Hassell, J. R., Star, V. L., Cannon, F. B., Laurie, G. W., et al. (1986). Basement membrane complexes with biological activity. Biochemistry. 25, 312–318. doi:10.1021/bi00350a005
Kolahi, K. S., Donjacour, A., Liu, X., Lin, W., Simbulan, R. K., Bloise, E., et al. (2012). Effect of substrate stiffness on early mouse embryo development. PloS One. 7, e41717. doi:10.1371/journal.pone.0041717
Koutsopoulos, S. (2016). Self-assembling peptide nanofiber hydrogels in tissue engineering and regenerative medicine: progress, design guidelines, and applications. J. Biomed. Mater. Res. 104, 1002–1016. doi:10.1002/jbm.a.35638
Lee, H., Rho, J., and Messersmith, P. B. (2009). Facile conjugation of biomolecules onto surfaces via mussel adhesive protein inspired coatings. Adv. Mater. 21, 431–434. doi:10.1002/adma.200801222
Levental, I., Georges, P. C., and Janmey, P. A. (2007). Soft biological materials and their impact on cell function. Soft Matter. 3, 299–306. doi:10.1039/b610522j
Levental, K. R., Yu, H., Kass, L., Lakins, J. N., Egeblad, M., Erler, J. T., et al. (2009). Matrix crosslinking forces tumor progression by enhancing integrin signaling. Cell. 139, 891–906. doi:10.1016/j.cell.2009.10.027
Martín, C., Merino, S., González-Domínguez, J. M., Rauti, R., Ballerini, L., Prato, M., et al. (2017). Graphene improves the biocompatibility of polyacrylamide hydrogels: 3D polymeric scaffolds for neuronal growth. Sci. Rep. 7. doi:10.1038/s41598-017-11359-x
Medina, S. H., Bush, B., Cam, M., Sevcik, E., Delrio, F. W., Nandy, K., et al. (2019). Identification of a mechanogenetic link between substrate stiffness and chemotherapeutic response in breast cancer. Biomaterials, 202, 1–11. doi:10.1016/j.biomaterials.2019.02.018
Neves, M. I., Moroni, L., and Barrias, C. C. (2020). Modulating alginate hydrogels for improved biological performance as cellular 3D microenvironments. Front. Bioeng. Biotechnol. 8, 665. doi:10.3389/fbioe.2020.00665
Parenteau-Bareil, R., Gauvin, R., and Berthod, F. (2010). Collagen-Based biomaterials for tissue engineering applications. Materials. 3, 1863–1887. doi:10.3390/ma3031863
Paszek, M. J., and Weaver, V. M. (2004). The tension mounts: mechanics meets morphogenesis and malignancy. J. Mammary Gland Biol. Neoplasia. 9, 325–342. doi:10.1007/s10911-004-1404-x
Paszek, M. J., Zahir, N., Johnson, K. R., Lakins, J. N., Rozenberg, G. I., Gefen, A., et al. (2005). Tensional homeostasis and the malignant phenotype. Cancer Cell. 8, 241–254. doi:10.1016/j.ccr.2005.08.010
Pelham, R. J., and Wang, Y. (1997). Cell locomotion and focal adhesions are regulated by substrate flexibility. Proc. Natl. Acad. Sci. U.S.A. 94, 13661–13665. doi:10.1073/pnas.94.25.13661
Schnaar, R. L., and Lee, Y. C. (1975). Polyacrylamide gels copolymerized with active esters. A new medium for affinity systems Biochemistry. 14, 1535–1541. doi:10.1021/bi00678a030
Schnaar, R. L., Weigel, P. H., Kuhlenschmidt, M. S., Lee, Y. C., and Roseman, S. 1978). Adhesion of chicken hepatocytes to polyacrylamide gels derivatized with N-acetylglucosamine. J. Biol. Chem. 253, 7940–7951. doi:10.1016/S0021-9258(17)34462-9
Tilghman, R. W., Cowan, C. R., Mih, J. D., Koryakina, Y., Gioeli, D., Slack-davis, J. K., et al. (2010). Matrix rigidity regulates cancer cell growth and cellular phenotype. PloS One. 5, e12905. doi:10.1371/journal.pone.0012905
Tse, J. R., and Engler, A. J. (2010). Preparation of hydrogel substrates with tunable mechanical properties. Curr. Protoc. Cell Biol. Chapter 10, Unit 10.16. doi:10.1002/0471143030.cb1016s47
Tsou, Y. H., Khoneisser, J., Huang, P. C., and Xu, X. (2016). Hydrogel as a bioactive material to regulate stem cell fate. Bioact. Mater. 1, 39–55. doi:10.1016/j.bioactmat.2016.05.001
Wen, J. H., Vincent, L. G., Fuhrmann, A., Choi, Y. S., Hribar, K. C., Taylor-weiner, H., et al. (2014). Interplay of matrix stiffness and protein tethering in stem cell differentiation. Nat. Mater. 13, 979–987. doi:10.1038/NMAT4051
Willcox, P. J., Reinhart-king, C. A., Lahr, S. J., Degrado, W. F., and Hammer, D. A. (2005). Dynamic heterodimer-functionalized surfaces for endothelial cell adhesion. Biomaterials. 26, 4757–4766. doi:10.1016/j.biomaterials.2004.11.060
Yamada, Y., Yoshida, C., Hamada, K., Kikkawa, Y., and Nomizu, M. (2020). Development of three-dimensional cell culture scaffolds using laminin peptide-conjugated agarose microgels. Biomacromolecules. 21, 3765–3771. doi:10.1021/acs.biomac.0c00871
Yip, A. K., Iwasaki, K., Ursekar, C., MacHiyama, H., Saxena, M., Chen, H., et al. (2013). Cellular response to substrate rigidity is governed by either stress or strain. Biophys. J. 104, 19–29. doi:10.1016/j.bpj.2012.11.3805
Keywords: polyacrylamide gel, N-hydroxysuccinimide-acrylamide ester, extracellular matrix, sulfosuccinimidyl 6-(4'-azido-2'-nitrophenylamino)hexanoate, mechanical stimulation
Citation: Kumai J, Sasagawa S, Horie M and Yui Y (2021) A Novel Method for Polyacrylamide Gel Preparation Using N-hydroxysuccinimide-acrylamide Ester to Study Cell-Extracellular Matrix Mechanical Interactions. Front. Mater. 8:637278. doi: 10.3389/fmats.2021.637278
Received: 03 December 2020; Accepted: 12 January 2021;
Published: 24 February 2021.
Edited by:
Elisabetta Ada Cavalcanti-Adam, Max-Planck-Gesellschaft, GermanyReviewed by:
Adil Denizli, Hacettepe University, TurkeyAndre D. R. Sliva, Brazilian Air Force Academy, Brazil
Copyright © 2021 Kumai, Sasagawa, Horie and Yui. This is an open-access article distributed under the terms of the Creative Commons Attribution License (CC BY). The use, distribution or reproduction in other forums is permitted, provided the original author(s) and the copyright owner(s) are credited and that the original publication in this journal is cited, in accordance with accepted academic practice. No use, distribution or reproduction is permitted which does not comply with these terms.
*Correspondence: Yoshihiro Yui, eW9zaGloaXJvLnl1aUB0b2t1c2h1a2FpLmpw