- 1Xi’an Herong New Energy Technology Co., Ltd., Xi’an, China
- 2School of Materials Science and Engineering, Xi’an University of Science and Technology, Xi’an, China
A modified ionic liquid (IL)-based electrolyte, with conventional carbonates as the support solvent and lithium salt as the additive, was designed for a high-voltage electrochemical double-layer capacitor (EDLC). It is found that the employment of carbonate solvents enhances the ionic conductivity and decreases the viscosity of the electrolyte. At the same time, the addition of lithium salt plays a key role in the stabilization of the operating potential window and the modification of the passivation layer on the electrode. As a result, increased specific capacitance, improved cycle stability, and rate capability are demonstrated in the EDLC with an EMI-BF4/LiPF6/PC/DMC electrolyte at the upper voltage of 3 V, in contrast to that with pure IL and IL/carbonate mixture. This study primarily stresses the combination effect of Li salt and organic solvent in an IL electrolyte system for constructing advanced 3 V-class EDLC with excellent overall performance.
Introduction
The development of electronic devices and the utilization of various renewable energies have created an urgent demand for advanced energy storage devices (Huang et al., 2020; Yuan et al., 2021; Lu et al., 2021). Electric double-layer capacitors (EDLCs) (Zhang et al., 2018), also known as super capacitors, have received much attention in recent years due to their high power density and extremely long shelf-life. However, their low energy density greatly hinders the practical application. An important approach to tackle the problem is to further expand the working voltage of the capacitor since the safe operating voltage range for a conventional organic electrolyte based on quaternary ammonium salt dissolved in acetonitrile (AN) or propylene carbonate (PC) is generally lower than 2.7 V (Zhong et al., 2015).
Great efforts to develop advanced electrolytes with a wider stable potential window have been made (Balducci, 2016; Martins et al., 2018; Liu et al., 2019). Ionic liquids (ILs) (Armand et al., 2009) seem to be a promising volunteer for constructing high-voltage EDLCs due to their high electrochemical and chemical stability. For example, it has been reported that EMI-BF4 can solely act as the electrolyte of EDLCs with an operative voltage of more than 3 V, even 4 V (Brandt et al., 2013; Yu et al., 2014). However, most of the ILs suffer from low ionic conductivity and high viscosity, which usually leads to poor cyclability and rate performance of the EDLC. For this reason, some low-viscous organic solvents (such as PC, AN, r-butyrolactone) have been used in combination with ILs (Palm et al., 2013; Tian et al., 2018; Scalia et al., 2019). As a result, the power characteristic of the EDLC was improved at the expense of shrinking the potential window because these support solvents inevitably participated in electrolyte decomposition, and thus stable cycling at the limited voltage beyond 3 V is hard to obtain.
Recently, there have been reports demonstrating that an EDLC with a Li+ -containing IL provides increased double-layer capacitance and especially enhanced electrochemical stability compared to pure IL without Li salt (Murayama et al., 2005). These studies revealed that the cathodic potential limit can shift favorably in a more negative direction after the addition of Li salt in the IL because the “inhibiting effect” induced by Li+ prevents the reduction of cations in IL (Matsumoto et al., 2003; Fukuda et al., 2007). An expanded operating potential window (OPW) and improved capacitor properties were therefore obtained. However, up to now, research on a mixed system consisting of ionic liquid, Li salt, and carbonate solvent in EDLCs has been rarely reported. Herein, this work aims to design a novel IL-based electrolyte employing conventional carbonates (PC, DMC) as the support solvent and Li salt LiPF6 as the additive for high-voltage EDLCs. The combination effects of Li salt and organic solvent on the electrolyte property and EDLC performance were investigated, and the related mechanism was discussed intensively.
Experimental
Electrolyte Preparation
1-Ethyl-3-methylimidazolium tetrafluoroborate (EMI-BF4, >99%, H2O < 70 ppm), lithium hexafluorophosphate (LiPF6, >99%, H2O < 50 ppm), propylene carbonate (PC, >99%, H2O < 10 ppm), and dimethyl carbonate (DMC, >99%, H2O < 10 ppm) were purchased from Guotai Super Power Co. Ltd. and used as received without further purification. The detailed compositions of the investigated electrolytes prepared in an argon-filled glove box are listed in Table 1.
The ionic conductivity and viscosity of the electrolyte at room temperature were measured by a conductivity meter (Mettler-Toledo) and viscosimeter (Brookfield), respectively. The moisture contents of the electrolytes were measured by the Karl Fisher titration method (Mettler-Toledo). The linear sweep voltammetry (LSV) of a stainless steel (SS)/Li cell was used to measure the potential threshold of the electrolyte with a scanning rate of 5 mV s−1.
Electrode and Cell Fabrication
Carbon electrode was prepared by mixing 85wt% active carbon (AC, Kuraray, YP-50F), 9wt% carbon black (CB, TIMCAL, Super P), 4wt% carboxymethylcellulose sodium (CMC, Nippon Paper Industries Co., Ltd., MAC500LC), and 2wt% styrene butadiene rubber (SBR, LG Chem, ADB81) on a piece of corrosive Al foil. The areal density of the electrode was around 7 mg cm−2, with the rectangular size of 45 mm × 40 mm, as exhibited in Figure 1A. The pouch-type EDLC (as shown in Figure 1B) was assembled by two identical carbon electrodes, a cellulose separator (NKK, TF4030), and the as-prepared electrolyte in the glove box.
Electrochemical Test
The cycle performance of the pouch-type EDLC was measured in a voltage range of 0.01–3 V, beginning with ten pre-cycles at 0.05 A g−1, and then performed at a constant current of 1 A g−1 on a LAND tester. The rate capability of the EDLC was also conducted at a constant current density from 1 to 10 A g−1 with an upper voltage of 3 V. The specific capacitance of the active material (Cs), energy density (E), and power density (P) of the EDLC was calculated according to the formulas below, respectively (Zhi et al., 2017):
where I is the discharge current (A), △t is the discharge time (s), △V is the voltage window (V) excluding the IR drop during the discharge process, and m is the total mass of the active material on both electrodes (g).
Cyclic voltammetry (CV) of the EDLC was carried out at varied voltage windows or scan rates on a CHI660E electrochemical workstation. Electrochemical impedance spectroscopy (EIS) was measured from 100 kHz to 0.01 Hz with an AC perturbation of 5 mV. The surface morphology and elemental constitution of the carbon electrode after 3 V cycling were investigated by scanning electron microscopy (SEM, Gemini 300) and energy-dispersive x-ray spectroscopy (EDS), respectively.
Results and Discussion
The conductivity and the viscosity of the various electrolytes are summarized in Table 2. The introduction of carbonate solvents in the EMI-BF4 increases the electrolyte conductivity due to distinctly decreased viscosity, especially for the PC-DMC mixture. Adding a small amount of LiPF6 into the IL/carbonate system leads to further improvement of the electrolyte ionic transport capability despite slightly increased viscosity, probably ascribed to the contribution of more mobile ions provided by the conductive salt. Ionic conductivity as high as 20.72 mS cm−1 is exhibited in the EMI-BF4/LiPF6/PC/DMC electrolyte. It should be noted that a certain amount of residual adsorbed/crystallized water in the IL product is always inevitable due to difficult purification, but the moisture content of the electrolyte is obviously reduced after utilizing these battery-grade support solvents, which can be regarded as the result of the “diluting effect.”
The LSV curves of the SS/Li cell in various electrolytes are shown in Figure 2. It can be seen that the cathodic potential threshold is almost similar among different electrolyte cases, and the introduction of carbonate solvents seems to have a minor influence on the anodic potential limit of the electrolyte as well. However, further addition of Li salt in the IL/carbonate mixture brings about obviously higher oxidation stability than its counterpart. The EMI-BF4/LiPF6/PC/DMC electrolyte is electrochemically stable up to ∼6.8 V vs. Li/Li+ in the test. It is considered that introducing Li salt into IL could balance the charge storage on the two electrodes and hence create an even distribution of the total window, which is favorable for broadening the OPW of the EDLC. The result of Li salt-contained IL is similar to a previous report where an IL mixture resulted in increased OPW (Van Aken et al., 2015).
The CV curves of the EDLCs based on various electrolytes are presented in Figure 3. It can be seen from Figure 3A that the pure EMI-BF4 provides a good rectangular shape in the CV curve when the voltage window is lower than 3.2 V. Increased upper voltage leads to a small quantity of Faraday reaction current, indicating that the IL tends to decompose. The existence of carbonate solvent usually affects the electrochemical stability of the IL electrolyte to a certain extent, but an oxidation peak was hardly observed in all of the investigated electrolytes at the limited voltage of 3 V, as shown in Figure 3B. Besides, the current responses (that is, specific capacitances) are almost identical at the low scan rate (5 mV s−1) among these cases. The result suggests that these IL/carbonates or IL/LiPF6/carbonate mixtures can favor normal operation at the upper voltage of 3 V.
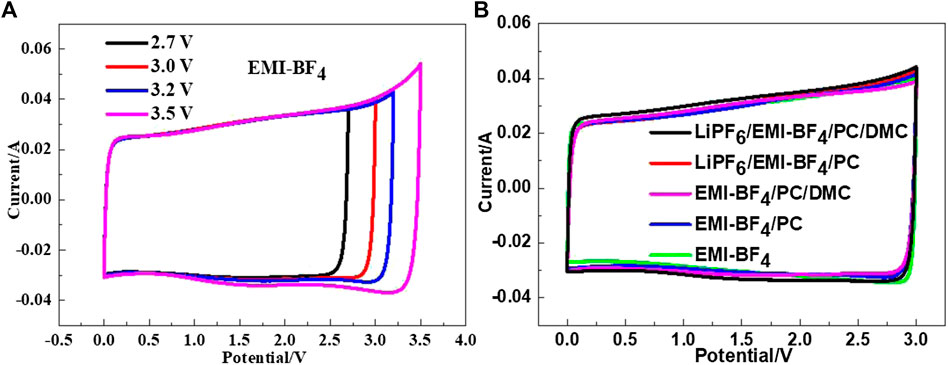
FIGURE 3. (A) CV curves of the EDLC in EMI-BF4 at the varied potential windows; (B) CV curves of the EDLCs in various electrolytes at a scan rate of 5 mV s−1.
The long-term cycle performances of the EDLCs in various electrolytes at the upper voltage of 3 V are compared in Figure 4A. The EDLC in EMI-BF4 delivers rapid capacitance decay during cycling, with capacitance retention of only 77.2% after 20,000 cycles. The employments of PC and PC/DMC in EMI-BF4 give rise to improved cycle capability. More notably, further addition of LiPF6 contributes to better cycle stability accompanied by stable Coulombic efficiency of over 99% compared to the counterparts. For instance, an initial specific capacitance of 128.5 F g−1 and capacitance retention as high as 95.8% after 20,000 cycles is exhibited in the modified electrolyte of EMI-BF4/LiPF6/PC/DMC. The superior high-voltage cycle stability can be mainly attributed to the fact that an addition of Li salt expands the OPW of the EDLC (as confirmed by the LSV test). Besides, introducing support solvents into the IL decreased the moisture content of the electrolyte, which should be another non-negligible reason for stable cycling operation.
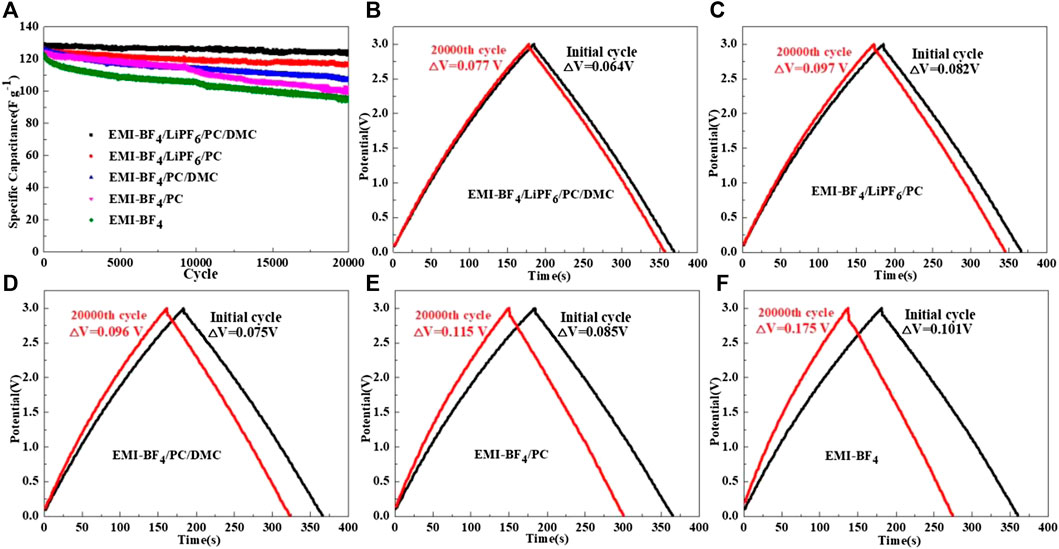
FIGURE 4. (A) Cycle performances of the EDLCs in various electrolytes at a current density of 1 A g−1; Charge-discharge profiles of the EDLCs in (B) EMI-BF4/LiPF6/PC/DMC, (C) EMI-BF4/LiPF6/PC, (D) EMI-BF4/PC/DMC, (E) EMI-BF4/PC, and (F) EMI-BF4 during long-term cycling.
The galvanostatic charge-discharge profiles of EDLCs in various electrolytes are shown in Figures 4B–F. When the charge cut-off voltage is set to 3.0 V, all of the cases show a linear and symmetrical triangle relationship no matter which electrolyte was employed, suggesting good electrochemical reversibility and typical double-layer capacitance behavior. However, a smaller IR drop at the charge/discharge switch (Chen et al., 2019) is observed for those solvent-contained electrolytes in contrast to pure IL, mainly attributed to the improved ionic conductivity. The addition of Li salt in a solvent-contained electrolyte contributes to a further reduction in IR drop. Significantly, the increase of IR drop in EMI-BF4/LiPF6/PC/DMC is almost negligible during 20,000 cycles, which is due to stable equivalent series resistance owing to minor irreversible Faraday reactions and fewer decomposed byproducts (Zhang et al., 2016).
Figure 5 displays the rate performance of the EDLCs in three selected electrolytes. The discharge specific capacitance descends gradually with the increased current density. But the variation trend is much smaller in the two Li salt-added electrolytes than that of the pure IL. Especially, the electrolyte EMI-BF4/LiPF6/PC/DMC provides the highest specific capacitances at different current densities compared to the other two cases. Even the charge-discharge current reaches 10 A g−1, and the specific capacitance values still stay at ∼114 F g−1. The rate capability of the EDLC in EMI-BF4/LiPF6/PC/DMC is also well demonstrated in the CV measurement at varied scan rates (Figure 6), where good rectangular shape can be maintained, and even the scan rate is up to 50 mV s−1. The excellent rate performance profits from a less viscous and more conductive advantage when carbonate solvent and LiPF6 were simultaneously introduced into the IL electrolyte: 1) highly dielectric PC can break the ion pair in IL, absorbing more ions on the surface of the porous electrode (Lu et al., 2019); 2) low viscous DMC (Marcinek et al., 2015) reduce ion movement resistance and benefit ion transport in the electrolyte and porous electrode; 3) dissociative Li salt also provides more mobile ions for conduction in the electrolyte. Besides, it is likely to involve the conversion of the double-layer structure (Yamagata et al., 2013); for example, Li+ may occupy the electrode/electrolyte interface preferentially due to a strong interaction between Li+ and the negatively polarized electrode at a high charge density, which decreases the thickness of the Helmholtz layer and leads to the increase in the electric double-layer capacitance.
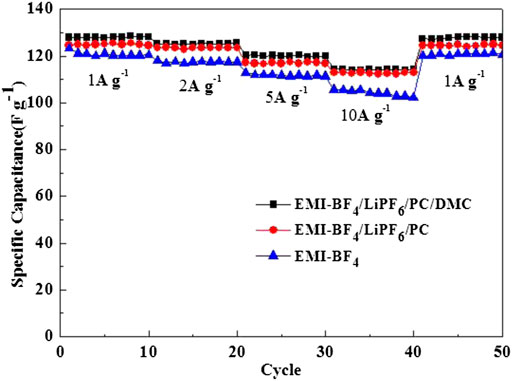
FIGURE 5. Rate performances of the EDLCs in various electrolytes at current densities ranging from 1 A g−1–10 A g−1.
Figure 7 gives the Ragone plots of the EDLCs based on formula calculation. The EMI-BF4/LiPF6/PC/DMC electrolyte offers superior specific energy and specific power values at each current density, e.g., the calculated energy density and the corresponding power density reaches 37.94 Wh kg−1 and 843 W kg−1 at 1 A g−1, respectively. Moreover, a high energy density of 26.35 Wh kg−1 can still be sustained even the applied current is increased to 10 A g−1, which is significantly higher than that measured in EMI-BF4/LiPF6/PC (22.03 Wh kg−1) and pure EMI-BF4 (15.93 Wh kg−1). The EDLC performance based on the designed EMI-BF4/LiPF6/PC/DMC electrolyte is comparable and even superior to previous reports with other 3 V-class electrolytes or those adopting asymmetric design (Lu et al., 2019; Vijayakumar et al., 2019).
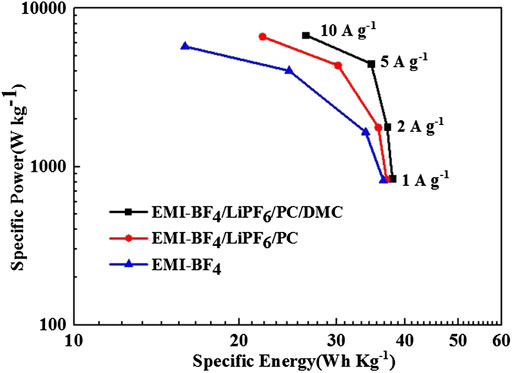
FIGURE 7. Ragone plots for the EDLCs in various electrolytes. The indicated specific energy and specific power refer to the total mass of the active materials on both electrodes.
The Nyquist plots of EDLCs based on various electrolytes after the initial cycle and the 20,000th cycle are presented in Figures 8A,B, respectively. Generally, the intercept on the X-axis is the series resistance (Rs), which is the sum of intrinsic resistance of the electrode, electrolyte, and separator, etc. The semi-circle in the high-frequency range refers to the charge transfer resistance (Rct) at the interface between the electrolyte and electrode in parallel with a constant phase element (CPE) (Lu et al., 2020). The intermediate frequency region with a linear slope is associated with the ion diffusion in the porous electrodes described by a Warburg diffusion element (W0) (Li et al., 2016). After the Warburg line, the impedance spectrum begins to be dominated by double-layer capacitive behavior (Cdl) in the very low-frequency range (Li et al., 2016). By fitting the inserted equivalent circuit, it is found that the Rs and Rct for two electrolytes after the initial cycle (0.34 and 0.091 Ω for EMI-BF4/LiPF6/PC/DMC, 0.37 and 0.095 Ω for EMI-BF4/LiPF6/PC) are obviously lower than the corresponding values for the EMI-BF4 electrolyte (0.42 and 0.141 Ω) owing to improved ionic conductivity. The decreased viscosity is beneficial to the permeability of the electrolyte in the electrode as well, and therefore a facilitated transfer process can be easily achieved at the interface (Wang et al., 2017). Besides, the overall resistance (especially Rs) of pure IL electrolyte increases markedly after 20,000 cycles, while a nearly constant resistance response exists in the two modified electrolytes. The above results clearly reveal an accelerated ion transport property and stable interface property in the IL electrolyte containing Li salt and support solvents, especially in the EMI-BF4/LiPF6/PC/DMC system.
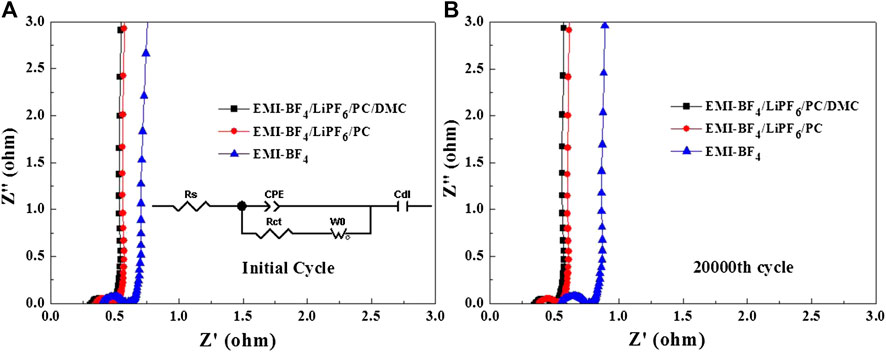
FIGURE 8. Nyquist plots of the EDLCs in three electrolytes after (A) the initial cycle and (B) the 20,000th cycle.
The surface chemistry difference of the cycled carbon electrodes in two selected electrolytes was further examined by SEM and EDS analyses. As shown in Figures 9A,C, the irregular AC particles are closely surrounded by abundant nano-sized carbon black, and a finely porous morphology is well maintained after 20,000 cycles. However, the EDS spectra in Figure 9D reveals the presence of high F-containing species on the electrode surface after the EDLC was cycled in EMI-BF4, which should mainly originate from the anion decomposition. In contrast, the cycled electrode in EMI-BF4/LiPF6/PC/DMC presents a surface composition of rich P element with less fluorine-related species (Figure 9B), suggesting that the Li salt is likely to participate in the formation of the surface passivation layer. The modified passivation effect could restrict the interactivity of the carbon electrode with the IL electrolyte and side reaction between them, and hence offers a favorable interface environment and high-voltage operation of the EDLC.
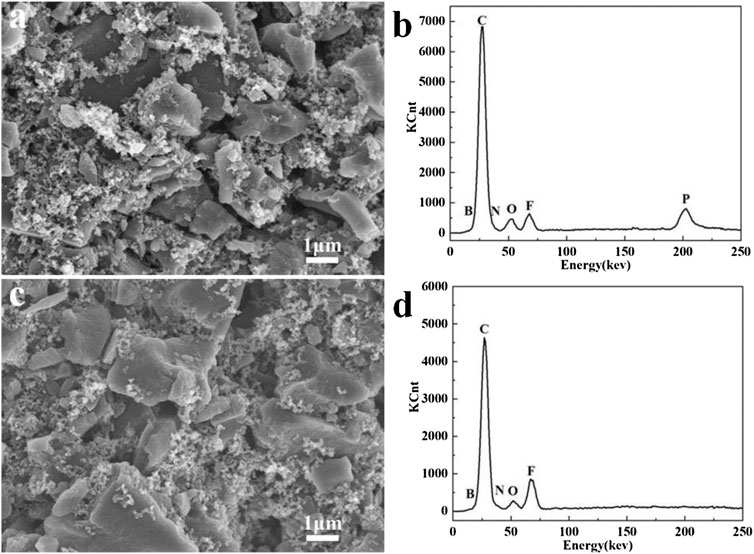
FIGURE 9. (A,C) SEM images and (B,D) EDS spectra of the cycled carbon electrode in (A,B) EMI-BF4/LiPF6/PC/DMC and (C,D) EMI-BF4.
Conclusion
In summary, a novel electrolyte consisting of ionic liquid, Li salt, and carbonate solvent is proposed for high voltage application of the EDLC. The EMI-BF4/LiPF6/PC/DMC electrolyte exhibits high ionic conductivity (20.72 mS cm−1) low viscosity (2.89 mPa s), and high electrochemical stability. The active carbon electrode in the modified electrolyte delivers an initial specific capacitance of as high as 128.5 F g−1 and a specific energy of 37.94 Wh kg−1 at 1 A g−1, with capacitance retention of ∼95.8% after 20,000 cycles at the limited voltage of 3.0 V, which is obviously superior to pure IL or the mixture of IL/carbonate. The enhanced ionic transport capability, balanced charge storage on both electrodes, and modified electrode surface passivation on account of the joint contribution of Li salt and carbonate solvents are considered to be important reasons for the improved overall performance, which guides a promising direction for the construction of advanced EDLC devices with high energy density, high power density, and long life.
Data Availability Statement
The original contributions presented in the study are included in the article/Supplementary Material, further inquiries can be directed to the corresponding authors.
Author Contributions
XL: Conceptualization, Writing - review and amp; editing, Supervision. HL: Investigation, Formal analysis, Writing - original draft. RF: Investigation. LH: Investigation. HL: Conceptualization, Formal analysis, Writing - review and amp; editing, Funding acquisition.
Funding
This work was supported by the Natural Science Foundation of China (No. 51604221) and Xi’an Science and Technology Plan (2020KJRC0065).
Conflict of Interest
XL and HL were employed by Xi’an Herong New Energy Technology Co., Ltd.
The remaining authors declare that the research was conducted in the absence of any commercial or financial relationships that could be construed as a potential conflict of interest.
References
Armand, M., Endres, F., MacFarlane, D. R., Ohno, H., and Scrosati, B. (2009). Ionic-liquid materials for the electrochemical challenges of the future. Nat. Mater. 8, 621–629. doi:10.1038/nmat2448
Balducci, A. (2016). Electrolytes for high voltage electrochemical double layer capacitors: a perspective article. J. Power Sourc. 326, 534–540. doi:10.1016/j.jpowsour.2016.05.029
Brandt, A., Pohlmann, S., Varzi, A., Balducci, A., and Passerini, S. (2013). Ionic liquids in supercapacitors. MRS Bull. 38, 554–559. doi:10.1557/mrs.2013.151
Chen, Z., Li, Z., Ma, X., Wang, Y., Zhou, Q., and Zhang, S. (2019). A new DMF-derived ionic liquid with ultra-high conductivity for high-capacitance electrolyte in electric double-layer capacitor. Electrochimica Acta 319, 843–848. doi:10.1016/j.electacta.2019.07.015
Fukuda, Y., Tanaka, R., and Ishikawa, M. (2007). Beneficial effects of a Li salt on electrode behavior in an ionic liquid for electric double layer capacitors. Electrochemistry 75, 589–591. doi:10.5796/electrochemistry.75.589
Huang, Y., Yu, R., Mao, G., Yu, W., Ding, Z., and Cao, Y., et al. (2020). Unique FeP@C with polyhedral structure in-situ coated with reduced graphene oxide as an anode material for lithium ion batteries. J. Alloys Compd. 841, 155670. doi:10.1016/j.jallcom.2020.155670
Li, Z., Liu, J., Jiang, K., and Thundat, T. (2016). Carbonized nanocellulose sustainably boosts the performance of activated carbon in ionic liquid supercapacitors. Nano. Energy 25, 161–169. doi:10.1016/j.nanoen.2016.04.036
Liu, C. F., Liu, Y. C., Yi, T. Y., and Hu, C. C. (2019). Carbon materials for high-voltage supercapacitors. Carbon 145, 529–548. doi:10.1016/j.carbon.2018.12.009
Lu, H., He, L., Li, X. Y., Zhang, W., Che, J. Y., Liu, X., et al. (2019). Ionic liquid-solvent mixture of propylene carbonate and 1,2-dimethoxyethane as electrolyte for electric double-layer capacitor. J. Mater. Sci. Mater. Electron. 30, 13933–13938. doi:10.1007/s10854-019-01737-8
Lu, H., Zhu, Y., Yuan, Y., He, L., Zheng, B., and Zheng, X., et al. (2021). LiFSI as a functional additive of the fluorinated electrolyte for rechargeable Li-S batteries. J. Mater. Sci.: Mater. Electron. 32, 5898–5906. doi:10.1007/s10854-021-05310-0
Lu, H., Zhu, Y., Zheng, B., Du, H. L., Zheng, X. Z., Liu, C. C., et al. (2020). A hybrid ionic liquid-based electrolyte for high-performance lithium-sulfur batteries. New J. Chem. 44, 361–368. doi:10.1039/c9nj03790j
Marcinek, M., Syzdek, J., Marczewski, M., Piszcz, M., Niedzicki, L., and Kalita, M., et al. (2015). Electrolytes for Li-ion transport-Review. Solid State Ionics 276, 107–126. doi:10.1016/j.ssi.2015.02.006
Martins, V. L., Torresi, R. M., and Rennie, A. J. R. (2018). Design considerations for ionic liquid based electrochemical double layer capacitors. Electrochimica Acta 270, 453–460. doi:10.1016/j.electacta.2018.03.094
Matsumoto, H., Kageyama, H., and Miyazaki, Y. (2003). Effect of ionic additives on the limiting cathodic potential of EMI-based room temperature ionic liquids. Electrochemistry 71, 1058–1060. doi:10.5796/electrochemistry.71.1058
Murayama, I., Yoshimoto, N., Egashira, M., Morita, M., Kobayashi, Y., and Ishikawa, M. (2005). Characteristics of electric double layer capacitors with an ionic liquid electrolyte containing Li ion. Electrochem. 73, 600–602. doi:10.5796/electrochemistry.73.600
Palm, R., Kurig, H., Tõnurist, K., Jänes, A., and Lust, E. (2013). Influence of different organic solvent additives on 1-ethyl-3-methylimidazolium tetrafluoroborate electrolyte based electrical double layer capacitors. J. Electrochem. Soc. 160, A1741–A1745. doi:10.1149/2.046310jes
Scalia, A., Varzi, A., Moretti, A., Ruschhaupt, P., Lamberti, A., and Tresso, E. (2019). Electrolytes based on N-butyl-N-Methyl-Pyrrolidinium 4,5-dicyano-2-(trifluoromethyl) imidazole for high voltage electrochemical double layer capacitors. ChemElectroChem 6, 552–557. doi:10.1002/celc.201801172
Tian, J. R., Cui, C. J., Xie, Q., Qian, W. Z., Xue, C., and Miao, Y. H., et al. (2018). EMIMBF4–GBL binary electrolyte working at -70 oC and 3.7 V for a high performance graphene-based capacitor. J. Mater. Chem. A 6, 3593–3601. doi:10.1039/c7ta10474j
Van Aken, K. L., Beidaghi, M., and Gogotsi, Y. (2015). Formulation of ionic-liquid electrolyte to expand the voltage window of supercapacitors. Angew. Chem. Int. Ed. 127, 4888–4891. doi:10.1002/anie.201412257
Vijayakumar, M., Rohita, D. S., Rao, T. N., and Karthik, M. (2019). Electrode mass ratio impact on electrochemical capacitor performance. Electrochimica Acta 298, 347–359. doi:10.1016/j.electacta.2018.12.034
Wang, B. H., Qin, Y., Tan, W. S, Tao, Y. X., and Kong, Y. (2017). Smartly designed 3D N-doped mesoporous graphene for high-performance supercapacitor electrodes. Electrochimica Acta 241, 1–9. doi:10.1016/j.electacta.2017.04.120
Yamagata, M., Hiryama, M., Nishishita, S., Horikawa, D., and Ishikawa, M. (2013). Impact of lithium salt addition to ionic liquid electrolytes for high-performance Electric Double-layer Capacitors. Electrochem. 81, 857–862. doi:10.5796/electrochemistry.81.857
Yuan, Y., Li, Z., Peng, X., Xue, K., Zheng, D., and Lu, H. (2021). Advanced sulfur cathode with polymer gel coating absorbing ionic liquid-containing electrolyte. J. Solid State Electrochem. 25, 1393–1399. doi:10.1007/s10008-021-04917-x
Yu, Y., Cui, C., Qian, W., and Wei, F. (2014). Full capacitance potential of SWCNT electrode in ionic liquids at 4 V. J. Mater. Chem. A. 2, 19897–19902. doi:10.1039/c4ta04773g
Zhang, L., Hu, X. S., Wang, Z. P., Sun, F. C., and Dorrell, D. G. A. (2018). Review of Supercapacitor Modeling, estimation, and Applications: A Control/management Perspective. Renew. Sust. Energ. Rev. 81, 1868–1878. doi:10.1016/j.rser.2017.05.283
Zhang, T., Fuchs, B., Secchiaroli, M., Wohlfahrt-Mehrens, M., and Dsoke, S. (2016). Electrochemical behavior and stability of a commercial activated carbon in various organic electrolyte combinations containing Li-salts. Electrochimica Acta 218, 163–173. doi:10.1016/j.electacta.2016.09.126
Zhi, L., Li, T., Yu, H., Chen, S., Dang, L., and Xu, H. (2017). Hierarchical graphene network sandwiched by a thin carbon layer for capacitive energy storage. Carbon 113, 100–107. doi:10.1016/j.carbon.2016.11.036
Keywords: electrochemical double layer capacitor, ionic liquid, lithium salt, capacitance, carbonate solvent, potential window
Citation: Li X, Li H, Feng R, He L and Lu H (2021) Electrochemical Double-Layer Capacitor Containing Mixtures of Ionic Liquid, Lithium Salt, and Organic Solvent as an Electrolyte. Front. Mater. 8:633460. doi: 10.3389/fmats.2021.633460
Received: 25 November 2020; Accepted: 06 January 2021;
Published: 14 April 2021.
Edited by:
Dewei Chu, University of New South Wales, AustraliaReviewed by:
Wei Kong Pang, University of Wollongong, AustraliaZhaoyong Chen, Changsha University of Science and Technology, China
Copyright © 2021 Li, Li, Feng, He and Lu. This is an open-access article distributed under the terms of the Creative Commons Attribution License (CC BY). The use, distribution or reproduction in other forums is permitted, provided the original author(s) and the copyright owner(s) are credited and that the original publication in this journal is cited, in accordance with accepted academic practice. No use, distribution or reproduction is permitted which does not comply with these terms.
*Correspondence: Hao Li, bGloYW8yNTMyNUAxNjMuY29t; Hai Lu, bGh4dXN0QDEyNi5jb20=