- 1Departamento de Física, Universidad Autónoma Metropolitana, Unidad Iztapalapa, Mexico City, Mexico
- 2Centro de Física Aplicada y Tecnología Avanzada, Universidad Nacional Autónoma de México, Santiago de Querétaro, México
- 3Laboratorio de Biotecnología, Instituto Nacional de Rehabilitación Luís Guillermo Ibarra Ibarra, Ciudad de México, México
- 4Departamento de Ingeniería Eléctrica, Universidad Autónoma Metropolitana, Unidad Iztapalapa, Mexico City, Mexico
Fibrous scaffolds based on biodegradable and biocompatible polyhydroxybutyrate (PHB) were produced by electrospinning. The scaffolds were coated by a thin solid film of polypyrrole, PPyI, deposited by plasma polymerization technique. FTIR and Raman spectroscopy results confirm the presence of PPyI on the PHB fibers, in FTIR can be show the signal of the NH2 groups characteristic of the polypyrrole, so Raman show a broad band due to fluorescence from the PPyI coating. The morphology of the scaffolds was characterized by scanning electron microscopy, and the average fiber diameter of PHB is (2.68 ± 1.69) μm, also the average fibers diameter of PHB-PPyI is (3.57 ± 1.36) μm, the comparation of the average fibers diameter of coated and uncoated indicates that the average PPyI coated thickness is 0.45 μm. Crystallinity of the PHB fibers was characterized by X-ray diffraction and differential scanning calorimetry, the degree of crystallinity is estimated at 70%. Pancreatic beta cells (from the RIN-M cell line) were cultured on PHB and PHB-PPyI scaffolds. Cell viability results showed that the surface modified material is a good cell culture substrate for beta cells.
Introduction
Diabetes mellitus (DM) is a health problem that affects a substantial number of people all over the world. In 2019, 463 million people were estimated to have this disease. An important aspect in the development of this illness is the reduction of insulin producing pancreatic beta cells and their inability to produce enough insulin to control the glucose in blood (World Health Organization and International Diabetes Federation, 2006; De Fronzo et al., 2015; Ogurtsova et al., 2018). DM represents a serious public health problem with significant social and economic impact. For this reason, it is the subject of wide research. In search of an alternative to control and prevent DM, tissue engineering (TE) makes use of polymeric biomaterials combined with cells. One of the most important goals in this research field is the design of biomaterials that support cells and carry signaling molecules that allow tissue regeneration (O’Brien, 2011). TE scaffolds have been prepared from different biocompatible and biodegradable polymers because of their easy processing. These polymers can be natural or synthetic, and scaffolds have been prepared from single polymers or combinations with other materials.
Polyhydroxyalkanoates are a family of biodegradable and natural polymers produced by microorganisms, used for biomedical applications. A member of this family of polymers is poly(3-hydroxybutyrate) (PHB). PHB exhibits biocompatibility, biodegradability, abrasion resistance, good mechanical strength, and adequate dimensional stability. Studies indicate that biodegradation of PHB is slower than poly(lactic acid), a commonly used biodegradable polymer (Zhuikov et al., 2021). PHB is a hydrophobic polymer with low cellular interaction, which limits its use in TE. PHB oligomers produced during degradation are non-toxic. The main disadvantage of PHB is its hydrophobic nature and lack of functional groups which results in low cell affinity, this limits its applications in TE. To solve the problem, PHB has been functionalized or mixed with other materials to create scaffolds that have good cellular interaction (Nahideh et al., 2019; Toloue et al., 2019; Naderi et al., 2020).
Various methods have been used for scaffold preparation, such as solvent casting, leaching, and electrospinning. One of the most widely used techniques is electrospinning, which can produce fibrous scaffolds with fiber diameters in the range from micrometers to nanometers. The fiber diameter can be controlled through the electrospinning process variables such as solution viscosity, applied voltage, injection flow rate, and needle-collector distance (Michael et al., 2001). The electrospun fibers have advantages for TE, such as high surface area, high porosity with interconnected pores that allow cell growth, proliferation, and differentiation in three dimensions (Sadeghi et al., 2016).
To improve biocompatibility, PHB has been electrospun with other materials, such as zinc oxide, (Zviagin Andrei et al., 2019), chitosan (Tolouea et al., 2019), carbon nanotubes, (Maryam and Saeed, 2020), and alumina nanowires (Tolouea et al., 2019). Degli Esposti and Chiellini, made bioactive and bioresorbable porous scaffolds for bone tissue regeneration, based on PHB and the incorporation of hydroxyapatite (Degli Esposti et al., 2019). Chondrocytes have been cultured on scaffolds of PHB/poly(hydroxybutyrate-co-hydroxyhexanoate) copolymer for cartilage regeneration (Ba et al., 2019). In the treatment of diabetic wounds, scaffolds of PHB with graphene oxide improved healing of normal and diabetic wounds (Daisy et al., 2020). PHB has also been combined with gelatin in electrospun scaffolds to evaluate their capability to treat diabetic wounds (Claudia et al., 2020).
One technique that has been shown to be effective in modifying the surface of different polymers is plasma polymerization. Surface modification can be accomplished by depositing a polymeric thin film that has functional groups that allow biomolecules to anchor to the surface (Ramírez-Fernández et al., 2012; Zuñiga Aguilar et al., 2014). Plasma polymerization using pyrrole as monomer has shown reliable performance in producing surfaces that promote cell adhesion and proliferation, especially in in vivo experiments (Ramírez-Fernández et al., 2015; Ruiz Velasco et al., 2017). Electrospun PHB has been treated by plasma to improve its biocompatibility. Electrospun PHB scaffolds have been exposed to oxygen plasma discharge and polyaniline nanoparticles have been synthesized on the treated surface of the scaffolds. The results indicated that the modification by oxygen plasma and nanoparticles of polyaniline had a favorable impact on the cell adhesion and direction of cell growth (Zamanifard et al., 2020).
In this study PHB scaffolds were prepared by electrospinning. The diameter of the fibers in the scaffolds have nano and micrometer size, with a high area-to-volume ratio and pores with sizes in the micrometer range. The surfaces of the PHB fibers were modified by coating with a thin polymeric film of polypyrrole deposited by the plasma polymerization technique and doped in situ with iodine, PPyI. This film has the objective of improving the biocompatibility of the fiber. Structural analyses confirm the presence of PHB and PPyI in the coated scaffolds. The scaffolds were placed in beta cell culture and the results show that the PPyI coating produces a biologically active surface on the scaffolds.
Experimental
Materials
Poly(3-hydroxybutyrate) (product #363502, natural origin), pyrrole (product #131709, reagent grade, 98%), ethanol (product #459844, 200 proof, ACS reagent. ≥99.5%) and iodine (product #207772, ACS reagent, ≥99.8%) were purchased from Sigma-Aldrich, reactive grade chloroform (product #AC423550250, ACS reagent, ≥99.8%, Acros Organics) was purchased from Fisher Scientific, all substances were used without further purification.
PHB Electrospinning
PHB scaffolds were prepared in a NaBond Electrospinning United model TL-01 of Tong Li Tech (Shenzhen, China). The machine is equipped with a 2-channel injection pump, a variable high-voltage source (0–30 kV) and a rotating cylinder collector 10 cm in diameter, with variable angular speed. In a typical experiment, PHB (1.2 g) was dissolved in 10 ml of a chloroform/ethanol mixture (9/1 v/v). The solution was heated to 50°C with stirring using a magnetic stirrer for 1 h. The solution was then introduced into a disposable syringe (20 ml) and placed in the injection pump. The injection flow rate was set to 3.0 ml/h. The solution was pushed through a food grade Teflon hose and reached a 0.6 mm ID needle. The collecting cylinder was covered with aluminum foil. A voltage of 15.0 kV was applied between the needle tip and the collector, and the needle-collector distance was set to 12 cm. PHB fibers were deposited on the collector, which was rotating at 1700 rpm. The electrospinning total time was 6.5 h.
PHB Surface Modification by Plasma Polymerization
Samples of PHB electrospun material were cut (approx. area of 1.0 cm2) and placed inside a plasma reactor. The plasma reactor (Figure 1) consists of a Pyrex glass tube of 90 mm external diameter and 250 mm long. Each end of the tube was sealed with a stainless-steel flange. Stainless-steel rods fitted with a 70 mm plate on their end, were inserted through the center of each flange and placed 5 cm apart. Each flange has two access ports. One port was used to introduce the pyrrole monomer and the iodine dopant. Pyrrole and iodine were in separate reservoirs and were introduced into the plasma reactor in vapor form. A pirani pressure gauge (Edwards) and a vacuum system consisting of a cold trap and a mechanical vacuum pump (Edwards) were fitted on the other flange. Plasma polymerization was performed using 20 W of power, a pressure of 2 × 10−2 Torr, a total synthesis time of 30 min and rf of 13.56 MHz.
Characterization
Raman Spectroscopy
Raman spectra of the scaffolds were obtained with a WiTec Raman System Alpha 300 RA confocal microscope. The samples were exposed to light from a 532 nm wavelength and 75 mW laser. Spectra were obtained from 32 replicate scans recorded between 3,700 cm−1 and 200 cm−1 with 4 cm−1 resolution. Samples were placed on the Raman System stage without further treatment.
Infrared Spectroscopy
The PHB and PHB-PPyI scaffolds were characterized by Fourier transform infrared spectroscopy (Perkin-Elmer GX-2000 System) with an attenuated total reflectance unit (Smith diamond DuraSample II). Spectra was recorded with 64 scans between 4,000 cm−1 and 400 cm−1 with 4 cm−1 resolution.
X-Ray Diffraction
The crystalline structure of PHB and PHB-PPyI scaffolds were studied by X-ray diffraction with a Bruker D8 Advance ECO diffractometer equipped with SSD160 detector, and Cu Kα X-ray source (0.15406 nm wavelength). Scaffold samples of 1 cm2 were placed in the sample holder and the diffractograms were acquired in a 2θ range of 2°–50°.
Differential Scanning Calorimetry
Differential scanning calorimetry was performed in a DSC instrument (DSC 2820 Modulated DSC, TA Instruments). Scaffold samples were placed in sealed aluminum trays. The samples were heated from 20 to 200°C using a 10°C/min heating ramp and were then cooled at 10°C/min from 200 to 20°C. The degree of crystallinity was calculated from the ratio of the enthalpy of fusion (∆Hm) of the sample to the enthalpy of fusion of 100% crystalline PHB, ∆H0m = 146 J/g (Barham et al., 1984).
Scanning Electron Microscopy
The morphology of the scaffolds was characterized from images obtained using a high-resolution scanning electron microscope (HRSEM JEOL JSM-7600F), at an accelerating voltage of 30 kV. The samples were coated by sputtering with a thin film of gold with 5 nm thickness, prior to SEM analysis. The fiber diameters were measured from SEM images using ImageJ software (National Institutes of Health, United States, version 1.53b). Histograms of fiber diameters were prepared and best fits to normal distribution were made using Origin software (OriginLab, version 8.1).
Cell Culture
Scaffold samples were sterilized overnight under UV light. Cells from the RIN-M cell line were cultured on the sterilized scaffolds. This cell line comes from a male rat’s insulinoma of the Rattus Norvegicus species. The cells secrete insulin and somatostatin. They have an epithelial morphology and an approximate size of 10 μm. Cells were proliferated following the ATCC® protocol using RPMI-1640 (ATCC® 30-2001) as the base culture medium and supplementing with fetal bovine serum and antibiotic with antimycotic at 10 and 1% of the total volume, respectively. The cells were incubated in a moistened environment with 5% CO2 at 37°C, using 100 × 20 mm culture petri dishes (product # CLS430167, Sigma Aldrich) and changing the culture medium every 3 days. The number of cells seeded on each scaffold was 4 × 104.
Cell Viability
To determine cell viability, a Calcein-AM and Ethidium-1 Homodimer kit purchased from AccesoLab was used. The scaffolds were washed with PBS buffer for 5 min and the supernatant was removed, this process was repeated two times. A PBS solution with Calcein-AM and Ethidium Homodimer-1 was prepared with a concentration of 1 and 2 μM, respectively. A volume of 500 μl of the solution was added to the scaffold samples. The samples were covered to protect them from light and allowed to stand before being viewed under the confocal microscope. With this technique, viable cells fluoresce green while dead cells fluoresce red.
Results and Discussion
FTIR Analysis
The infrared spectra of uncoated PHB and surface-modified PHB-PPyI scaffolds are show in Figure 2. The most intense band of the PHB is found at 1724 cm−1, corresponding to the characteristic stretch of the carbonyl bond (ester carbonyl stretch). Peaks at 2,972 cm−1 and 2,927 cm−1 are characteristic of aliphatic CH3 and CH2 groups. The band at 1,442 cm−1 corresponds to the asymmetric deformation of C-H bonds, and the signal corresponding to stretching of these bonds is also observed at 1,378 cm−1. The series of intense bands located between 900 and 1,300 cm−1 correspond to the stretching of the C-O bond of the ester group. The spectrum of PHB-PPyI shows a broad and intense band between 3,100 and 3,700 cm−1, this signal is due to amine groups along with hydroxy groups. Since this band is not present in the PHB spectrum, it is produced by vibrations of chemical groups in the PPyI coating. Also, in the PHB-PPyI spectrum, the band at 1724 cm−1 shows a shoulder around 1,627 cm−1 which is due to amine groups in the PPyI coating (Zuñiga Aguilar et al., 2014).
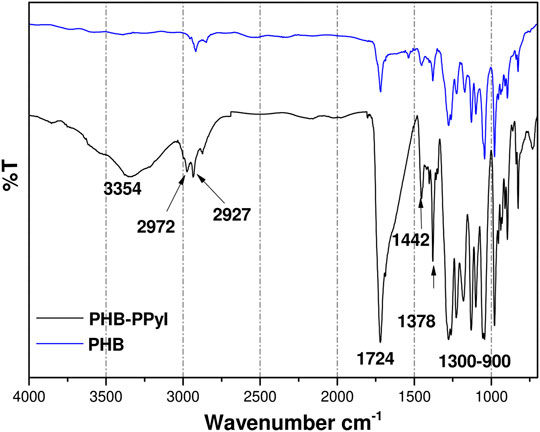
FIGURE 2. FTIR spectra of PHB and PHB-PPyI scaffolds. Amine groups in PPyI produce the band at 3,354 cm−1.
Raman Spectroscopy
Raman spectra obtained with a Raman confocal microscope are shown in Figure 3. The Raman spectrum of the PHB scaffold shows an intense band at 2,920 cm−1 that corresponds to aliphatic carbons. The spectrum is consistent with other reports (Furukawa et al., 2006; Tsuyoshi et al., 2006). In contrast, the Raman spectrum of PHB-PPyI shows a broad band between 1,600 and 3,600 cm−1 which masks most other signals. This broad band is due to fluorescence from the PPyI coating (Vazquez Ortega et al., 2014). Two other bands can be observed at 1,357 and 1,588 cm−1. The first of these bands is likely due to aliphatic CH3 groups and is also present in the PHB spectrum. The second band is only present in the PHB-PPyI spectrum and is likely due to the presence of iodine (Vazquez Ortega et al., 2014).
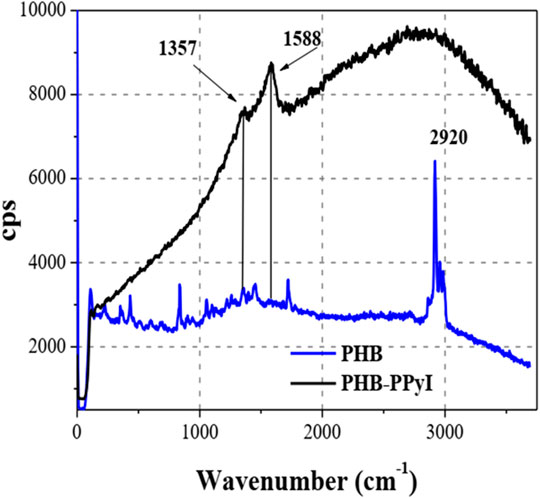
FIGURE 3. Raman spectra of PHB and PHB-PPyI scaffolds. The broad signal in the PPyI spectrum is due to fluorescence.
Crystalline Structure
The X-ray diffractograms of PHB and PHB-PPyI were obtained at room temperature and are shown in Figure 4. Both samples present the same diffraction signals, showing that the PPyI surface coating did not affect the PHB crystallinity (Socrates, 2001). The figure shows two very intense diffraction peaks centered at 2θ = 13° and 17° corresponding to the (020) and (110) planes of the orthorhombic unit cell of PHB crystals. Less intense diffractions are located around 2θ = 23° and 26°, corresponding to (101) and (121) planes, respectively (Tolouea et al., 2019).
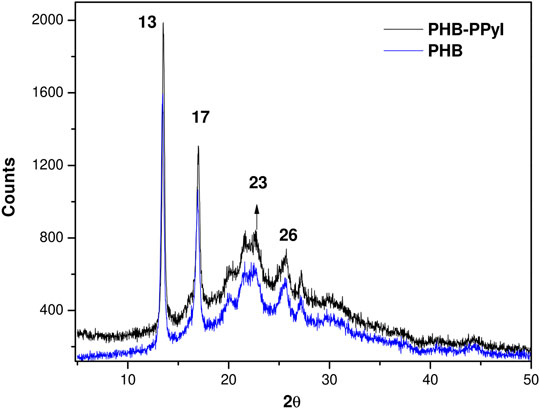
FIGURE 4. X-ray diffractograms of PHB and PHB-PPyI. The lattice parameters of PHB and PHB-PPyI scaffold were a = 5.65 Å, b = 13.61 Å and c = 5.86 Å.
PHB crystals have an orthorhombic unit cell with dimensions a = 5.76 Å, b = 13.20 Å and c = 5.96 Å (Wang and Tashiro, 2016). The lattice parameters from the diffractograms of PHB and PHB-PPyI samples were calculated to be a = 5.65 Å, b = 13.61 Å and c = 5.86 Å. The crystal size was estimated from the main diffraction peak to be 300 Å; and the percentage of crystallinity of both samples is 70%. Table 1 shows the crystal planes, the angular position of the diffraction peaks and the interplanar distance of the PHB crystals.
DSC Analysis
The thermal analysis of the scaffolds was carried out by means of differential scanning calorimetry. The DSC thermograms are shown in Figure 5. The melting temperature of the PHB fibers was 176°C and ∆Hm of 102.3 J/g. The degree of crystallinity is estimated at 70%, consistent with XRD results. The PHB-PPyI scaffold, in contrast, had a melting point of 172°C, lower than that of PHB. During the plasma polymerization procedure, the electric field is turned on for a few minutes before pyrrole is allowed into the reactor. The air that remains in the reactor forms a plasma which can damage the fibers. Previous studies have shown that during plasma polymerization of electrospun materials, very thin fibers disappear due to this etching (Cruz et al., 2019). This process also results in partial degradation of PHB molecules, therefore reducing the molecular weight of the PHB molecules. This is possibly the reason for the lower melting temperature that was measured in PHB-PPyI.
The DSC cooling curve (Figure 5B) also shows a difference in the crystallization temperature of pure PHB and PHB-PPyI. After heating to 200°C, the materials are no longer in fiber form since melting has occurred. On cooling, the PHB-PPyI sample crystallizes at a higher temperature than the PHB sample likely because PPyI fragments act as nucleation agents.
SEM Analysis
Figures 6A–C show SEM images of the electrospun PHB scaffold. The fibers are distributed over the entire observation region. Figures 6A,B show that the fibers were aligned by the rotation of the collecting drum, they also show the formation of beads along the fibers. The fibers alignment and a wide size distribution are more evident in Figure 6B. In Figure 6C a thick fiber with rough surface can be observed. The roughness is likely due to the interaction of the fiber with the air during travel to the collecting cylinder as the solvents were evaporating. The fiber size distribution is shown in Figure 6D. The average fiber diameter is (2.68 ± 1.69) µm.
Figures 7A–C show SEM images of the PHB-PPyI scaffold. Some fibers are fractured possibly due to their interaction with the high energy plasma. Figure 7A shows a panoramic view of the scaffold, and higher magnification images are shown in Figures 7B,C. The roughness of the coating could promote the anchorage of the cells on the fiber’s surface and contribute to better cellular interaction. The PPyI coating resulted in an increase in the diameter of the fibers; the average fiber diameter was (3.57 ± 1.36) μm. Comparing the average fiber diameter of the coated and uncoated samples indicates that the average PPyI coating thickness was 0.45 μm.
Cell Culture
Figure 8 shows optical photographs of RIN-M cells after 10 days of culture on PHB and PHB-PPyI scaffolds. The images show the frameworks of the PHB fibers and anchoring of cells on the scaffold. RIN-M cells can be seen forming cell colonies on the scaffold. Cells have also entered the scaffolding forming a 3D culture. In contrast, it is not possible to observe the internal structure of the PPyI-coated scaffold (Figure 8B). A monolayer of RIN-M cells can be seen growing on the edge of the PHB-PPyI scaffold. This confirms the biocompatibility of this material.
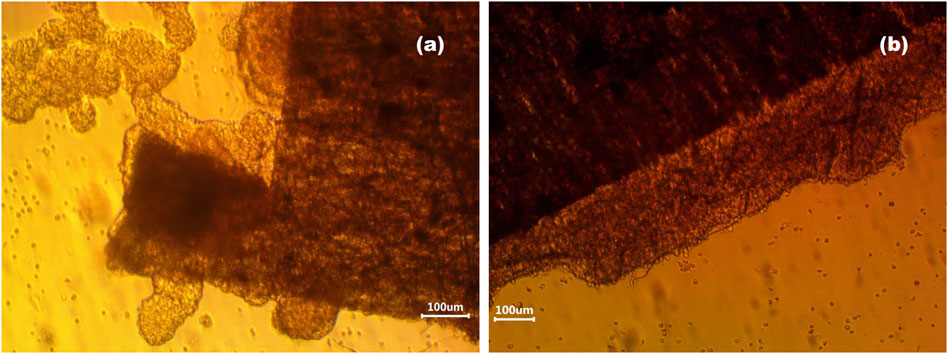
FIGURE 8. Optical photographs of RIN-M cells on the 10th day of culture on (A) PHB and (B) PHB-PPyI scaffolds.
Cellular Viability
Figure 9 shows the results of the Calcein and EthD-1 cell viability assay for RIN-M cells cultured for 10 days on PHB and PHB-PPyI. Figure 9A corresponds to the PHB scaffold. It is possible to identify both living and dead cells on the image. Some cell colonies are also appreciated. There are more living cells (green) than dead cells (red).
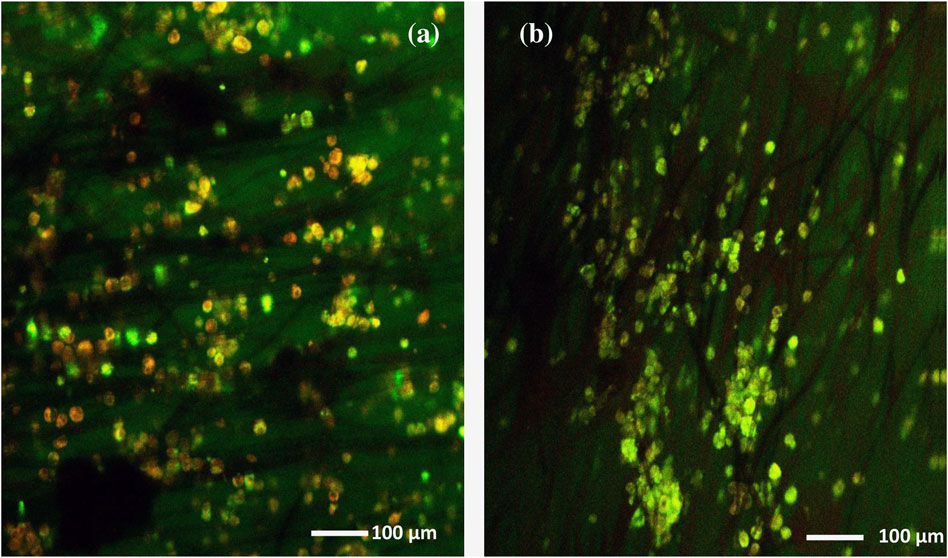
FIGURE 9. Cell viability assay after 10 days of culture resulting images for (A) PHB and (B) PHB-PPyI scaffolds.
In Figure 9B, viable cells are observed after 10 days of cell culture on the PHB-PPyI scaffold. A large number of living cells are observed on the scaffold fibers. The cells seen in green are viable and form colonies of cells spread throughout the observation area. It is also observed that some cells anchored to the scaffold fibers. This shows that this type of scaffold allows the anchorage, growth, proliferation, and viability of this type of cells.
Conclusions
PHB electrospun scaffolds allow the anchorage, differentiation, and proliferation of RIN-M beta cells. The scaffold coated with PPyI shows a better response in cell culture. It is possible to grow RIN-M cells on these scaffolds.
In vitro cell culture tests with the RIN-M cell line showed that PHB electrospun scaffolds are biocompatible since cell adhesion and proliferation was observed. The cell viability test showed that the scaffold with best suited for beta cell culture was PHB-PPyI. This coated scaffold showed a greater number of viable cells. The materials presented in this work have the potential to be used for treatment of diabetes mellitus in the form, for example, of an insulin producing subcutaneous patch.
Data Availability Statement
The raw data supporting the conclusion of this article will be made available by the authors, without undue reservation.
Author Contributions
EC-O participated in scaffold preparation and characterization, cell studies and wrote the manuscript. RO-V interpreted X-ray characterization. RR-T, MG-T, and SV-M aided in the preparation of the scaffolds. RG-F and OJ aided in cell culture and cell viability studies. RO and JM-C conceived the project. RO-V, RO, and JM-C were involved in writing and reviewing the manuscript.
Conflict of Interest
The authors declare that the research was conducted in the absence of any commercial or financial relationships that could be construed as a potential conflict of interest.
Acknowledgments
The authors thank Patricia Castillo from the UAM Iztapalapa Electron Microscopy Laboratory for electron microscopy images. JMC thanks PROMEP-SEP for the support through the “Estancias Cortas de Investigación de Integrantes de Cuerpos Académicos” program.
References
Ba, K, Wei, X, Ni, D, Li, N, Du, T, Wang, X, and Pan, W (2019). Chondrocyte Co-cultures with the Stromal Vascular Fraction of Adipose Tissue in Polyhydroxybutyrate/Poly-(hydroxybutyrate-co-hydroxyhexanoate) Scaffolds: Evaluation of Cartilage Repair in Rabbit. Cell Transplant. 28 (11), 1432–1438. doi:10.1177/0963689719861275
Barham, P. J., Keller, A., Otun, E. L., and Holmes, P. A. (1984). Crystallization and morphology of a bacterial thermoplastic: poly-3-hydroxybutyrate. J. Mater. Sci. 19, 2781–2794. doi:10.1007/BF01026954
Claudia, S., Jeyson, H., Bugallo-Casal, A., Da Silva-Candal, A., Cristina, T., Rosendo, M., et al. (2020). One-step electrospun scaffold of dual-sized gelatin/poly-3-hydroxybutyrate nano/microfibers for skin regeneration in diabetic wound. Mater. Sci. Eng. C. 119, 111602. doi:10.1016/j.msec.2020.111602
Cruz, Y., Muñoz, E., Gomez-Pachón, E. Y., Morales-Corona, J., Olayo-Lortia, J., Olayo, R., et al. (2019). Electrospun PCL-protein scaffolds coated by pyrrole plasma polymerization. J. Biomater. Sci. Polym. Ed. 30 (10), 832–845. doi:10.1080/09205063.2019.1603338
Daisy, E. R. A. C., Rajendran, N. K., Houreld, N. N., Marraiki, N., Elgorban, A. M., and Rajan, M. (2020). Curcumin and Gymnema sylvestre extract loaded graphene oxide-polyhydroxybutyrate-sodium alginate composite for diabetic wound regeneration. Reactive Funct. Polym. 154, 104671. doi:10.1016/j.reactfunctpolym.2020.104671
De Fronzo, R. A., Ferrannini, E., Zimmet, P., and Alberti, G. (2015). International Textbook of Diabetes Mellitus. Oxford, United Kingdom: Wiley-Blackwell.
Degli Esposti, M., Federica, C., Federica, B., and Paola Fabbri, M. (2019). Highly porus PHB-based bioactive scaffolds for bone tissue engineering by in situ synthesis of hydroxyapatite. Mater. Sci. Eng. C. 100, 286–296. doi:10.1016/j.msec.2019.03.014
Furukawa, T., Sato, H., Murakami, R., Zhang, J., Noda, I., Ochiai, S., and Ozaki, Y. (2006). Raman microspectroscopy study of structure, dispersibility, and crystallinity of poly(hydroxybutyrate)/poly(l-lactic acid) blends. Polymer 47, 3132–3140. doi:10.1016/j.polymer.2006.03.010
Maryam, M, and Saeed, S (2020). Physical, mechanical and biological performance of PHB-Chitosan/MWCNTs nanocomposite coating deposited on bioglass based scaffold: Potential application in bone tissue engineering. Int. J. Biol. Macromol. 152, 645–662. doi:10.1016/j.ijbiomac.2020.02.266
Michael, B., Czado, W., Thomas, F., Andreas, S., Michael, H., Martin, S., et al. (2001). Nanostructured fibers via electrospinning. Adv. Mater. 13 (1), 70–72. doi:10.1002/1521-4095(200101)13:1<70::AID-ADMA70>3.0.CO;2-H
Naderi, P., Zarei, M., Karbasi, S., and Salehi, H. (2020). Evaluation of the Effects of keratin on Physical, Mechanical and Biological Properties of Poly (3-hydroxybutyrate) Electrospun Scaffold: Potential Application in Bone Tissue Engineering. Eur. Polym. J. 124, 109502. doi:10.1016/j.eurpolymj.2020.109502
Nahideh, A., Del Bakhshayesh, A. R., Soodabeh, D., and Akbarzadeh, A. (2019). Common biocompatible polymeric materials for tissue engineering and regenerative medicine. Mater. Chem. Phys. 242, 122528. doi:10.1016/j.matchemphys.2019.122528
O’Brien, F. J. (2011). Biomaterials and scaffolds for tissue engineering. Materials Today 14, 88–95. doi:10.1016/S1369-7021(11)70058-X
Ogurtsova, K., da Rocha Fernandes, J.D., Huang, Y., Linnenkamp, U., Guariguata, L., Cho, N. H., et al. (2018). IDF diabetes atlas: Global estimates of diabetes prevalence for 2017 and projections for 2045. Diabetes Res. Clin. Pract. 138, 271–281. doi:10.1016/j.diabres.2018.02.023
Ramírez‐Fernández, O., Godinez, R., Morales, J., Gómez-Quiroz, L., Gutiérrez-Ruiz, M. C., Zuñiga-Aguilar, E., and Olayo, R. (2012). Glow discharge plasma modified surfaces for hepatic co-culture models. Rev. Mex. Ing. Biomed. 33, 127–135.
Ramírez-Fernández, O., Godinez, R., Morales, J., Gómez-Quiroz, L., Gutiérrez-Ruiz, M. C., Zuñiga-Aguilar, E., and Olayo, R., et al. (2015). Hepatocyte culture in a radial-flow bioreactor with plasma polypyrrole coated scaffolds. Biocell 39, 9–14. doi:10.32604/biocell.2015.39.009
Ruiz Velasco, G., Martínez-Flores, F., Morales-Corona, J., Olayo-Valles, R., and Olayo, R. (2017). Polymeric scaffolds for skin. Macromol. Symp. 374, 1600133. doi:10.1002/MASY.201600133
Sadeghi, D., Karbasi, S., Razavi, S., Mohammadi, S., Shokrgozar, M. A., and Bonakdar, S. (2016). Electrospun poly(hydroxybutyrate)/chitosan blend fibrous scaffolds for cartilage tissue engineering. J. Appl. Polym. Sci. 133, 44171. doi:10.1002/app.44171
Socrates, G. (2001). Infrared and Raman characteristic group frequencies; tables and charts. 3rd Edn. Chichester, United Kingdom: John Wiley and Sons.
Toloue, E. B., Karbasi, S., Salehi, H., and Rafienia, M. (2019). Evaluation of mechanical properties and cell viability of poly (3-hydroxybutyrate)-chitosan/Al2O3 nanocomposite scaffold for cartilage tissue engineering. J. Med. Signals Sens 9 (2), 111–116. doi:10.4103/jmss.JMSS_56_18
Tolouea, E. B., Karbasi, S., Salehi, H., and Rafienia, M. (2019). Potential of an electrospun composite scaffold of poly (3-hydroxybutyrate)-chitosan/alumina nanowires in bone tissue engineering applications. Mater. Sci. Eng. C. 99, 1075–1091. doi:10.1016/j.msec.2019.02.062
Tsuyoshi, F., Sato, H., Murakami, R., Zhang, J., Noda, I., Shukichi, O., et al. (2006). Raman microspectroscopy study of structure, dispersibility, and crystallinity of poly(hydroxybutyrate)/poly(l-latic acid) blends. Polymer 47 (9), 3132–3140. doi:10.1016/j.polymer.2006.03.010
Vazquez Ortega, M., Ortega, M., Morales, J., Olayo, M. G., Cruz, G. J., and Olayo, R. (2014). Core–shell polypyrrole nanoparticles obtained by atmospheric pressure plasma polymerization. Polym. Int. 63, 2023–2029. doi:10.1002/PI.4756
Wang, H., and Tashiro, K. (2016). Reinvestigation of crystal structure and intermolecular interactions of biodegradable poly(3-hydroxybutyrate) α-form and the prediction of its mechanical property. Macromolecules 49, 581. doi:10.1021/acs.macromol.5b02310
World Health Organization and International Diabetes Federation(2006). Definition and diagnosis of diabetes mellitus and intermediate hyperglycaemia. Geneva, Switzerland: World Health Organization and International Diabetes Federation.
Zamanifard, M., Khorasani, M. T., Daliri, M., and Parvazinia, M. (2020). Preparation and modeling of electrospun polyhydroxybutyrate/polyaniline composite scaffold modified by plasma and printed by an inkjet method and its cellular study. J. Biomater. Sci. Polym. Edition 31 (12), 1515–1537. doi:10.1080/09205063.2020.1764162
Zhuikov, V. A., Akoulina, E. A., Chesnokova, D. V., Wenhao, Y., Makhina, T. K., Demyanova, I. V., et al. (2021). The growth of 3T3 fibroblasts on PHB, PLA and PHB/PLA blend films at different stages of their biodegradation in vitro. Polymers 13, 108. doi:10.3390/polym13010108
Zuñiga Aguilar, E., Olayo, R., Ramírez-Fernández, O., Morales, J., and Godínez, R. (2014). Nerve cells culture from lumbar spinal cord on surfaces modified by plasma pyrrole polymerization. J. Biomater. Sci. Polym. Edition 25, 729–747. doi:10.1080/09205063.2014.898124
Zviagin, A. S., Chernozem, R. V., Surmeneva, M. A., Pyeon, M., Frank, M., Ludwig, T., et al. (2019). Enhanced piezoelectric response of hybrid biodegradable 3D poly(3-hydroxybutyrate) scaffolds coated with hydrothermally deposited ZnO for biomedical applications. Eur. Polym. J. 117, 272–279. doi:10.1016/j.eurpolymj.2019.05.016
Keywords: plasma polymerization, polyhydroxyburyrate, electrospinning, scaffold, beta cells, tissue engineering
Citation: Cortés-Ortiz E, Olayo-Valles R, Rodríguez-Talavera R, González-Torres M, Vargas-Muñoz S, Olayo R, Godinez-Fernández R, Uribe Juárez OE and Morales-Corona J (2021) Plasma Functionalized Scaffolds of Polyhydroxybutyrate Electrospun Fibers for Pancreatic Beta Cell Cultures. Front. Mater. 8:600738. doi: 10.3389/fmats.2021.600738
Received: 31 August 2020; Accepted: 19 February 2021;
Published: 08 April 2021.
Edited by:
Ana M. Beltrán, University of Seville, SpainReviewed by:
Saeid Kargozar, Mashhad University of Medical Sciences, IranSônia Maria Malmonge, Federal University of ABC, Brazil
Paul Andrew Wieringa, Maastricht University, Netherlands
Copyright © 2021 Cortés-Ortiz, Olayo-Valles, Rodríguez-Talavera, González-Torres, Vargas-Muñoz, Olayo, Godinez-Fernández, Uribe Juárez and Morales-Corona. This is an open-access article distributed under the terms of the Creative Commons Attribution License (CC BY). The use, distribution or reproduction in other forums is permitted, provided the original author(s) and the copyright owner(s) are credited and that the original publication in this journal is cited, in accordance with accepted academic practice. No use, distribution or reproduction is permitted which does not comply with these terms.
*Correspondence: Juan Morales-Corona, am1vckB4YW51bS51YW0ubXg=