- 1Department of Orthopaedics, Wuhan University, Renmin Hospital, Wuhan, China
- 2Department of Mechanical Engineering, San Diego State University, San Diego, CA, United States
- 3Mork Family Department of Chemical Engineering and Materials Science, Viterbi School of Engineering, University of Southern California, Los Angeles, CA, United States
- 4Keck School of Medicine, Roski Eye Institute, University of Southern California, Los Angeles, CA, United States
Nature has developed a wide range of functional microstructures with optimized mechanical properties over millions of years of evolution. By learning from nature’s excellent models and principles, biomimicry provides a practicable strategy for designing and fabricating the next smart materials with enhanced properties. Nevertheless, the complicated micro-structural constructions in nature models are beyond the ability of conventional processes, hindering the developments of biomimetic research and its forthputting in engineering systems. Additive manufacturing (AM) or 3D printing processes have revolutionized manufacturing via their ability to manufacture complex micro/mesostructures, increase design freedom, provide mass customization, and waste minimization, as well as rapid prototyping. Here, a review of recent advances in biomimetic 3D printing materials with enhanced mechanical properties is provided. The design and fabrication were inspired by various natural structures, such as balsa wood, honeycomb, nacre, lobster claw, etc., which are presented and discussed. Finally, future challenges and perspectives are given.
Introduction
There are numerous biological systems on earth that have experienced thousands of years of evolution to refine their structures, each which enormously take advantage of different environments (Catania, 2012; Yang et al., 2018b). Moreover, biological structures may generally evolve into certain function-related models. As for the applications of bioinspiration, biomimetic models are often used as experimental agents of the natural designs. This is done so that unique individual characteristics can be analyzed independently from their evolutionary constraints, artificially modified, and systematically tested under accessible control (Zhao et al., 2013; Speck et al., 2017). However, the complicated micro-structural constructions in nature models are beyond the fabrication capability of traditional manufacturing methods (Feilden et al., 2017; Jiang et al., 2018, 2019a). This limitation hinders further studies in bioinspired design and exploring their applications.
Additive manufacturing (AM), or 3D printing processes, have revolutionized manufacturing by allowing greater design freedom, waste minimization, mass customization, geometrically-intricate capabilities, rapid prototyping, and the capability to manufacture complex micro/mesostructures (Herzog et al., 2016; Ngo et al., 2018). Additionally, bioinspired structures, which range from imitating plants (Kim et al., 2018; Yang et al., 2018a) to animals (Cully et al., 2015; Cui et al., 2018), and insects (Rogóż et al., 2016; Chung et al., 2018), have been studied to investigate multiple properties using 3D printing technology (Figure 1). The Bouligand structure in the lobster claw will effectively increase the toughness and impact resistance of the material by increasing the difficulty of crack propagation. The aligned fiber in a balsa wood structure will reinforce the strength, which leads to a higher capability of wind resistance. The brick-and-mortar structure in natural nacre leads to increased impact resistance by crack deflection and energy dissipation. For example, Brett Compton et al. prepared a biomimetic structure of balsa wood produced by 3D direct ink writing, which oriented milled carbon fiber in epoxy resin along the print direction (Compton and Lewis, 2014). Nature provides excellent models that can also be further adjusted to develop related products that best suit real-life applications.

Figure 1. Schematic diagram shows the biomimetic structures. (A) Bouligand structure from lobster claw (Yang et al., 2017); (B) aligned fiber in balsa wood; (C) brick-and-mortar structure in natural nacre (Tran et al., 2017).
Herein, in this review, we focus on the latest developments of biomimetic 3D printing materials with enhanced properties, especially mechanical properties. The main content of the paper is divided into two parts: (1) Single material– bioinspired reinforced structures that are inspired by creatures such as shrimp and honeycomb. These were manufactured by 3D printing using a single material, such as polymer, metal, graphene, and so on. (2) Multi-materials– numerous 3D-printed constructions such as those inspired by the Bouligand structure, balsa wood structure, nacre structure as well as shape changing structures. These have been constructed to investigate their enhanced functionalities. Challenges and opportunities will be discussed, and perspectives on future research will be given in the conclusion.
Bioinspired Reinforced Structures by Additive Manufacturing
Single Material
The 3D printing of bioinspired structures using single materials is widely studied. The materials can be different types of polymers, metals, ceramics, and so on (Wang et al., 2017). Bioinspired structures using 3D printing play an important role in strengthening the single materials’ mechanical properties (Meyers et al., 2008).
Honeycomb Structure
Bioinspired structures derived from hexagonal honeycomb have generally received tremendous attention from the public, and have been applied in several engineering-related fields. Hedayati et al. (2016) used polylactic acid (PLA) as a single raw material to manufacture thick honeycomb structures by fused deposition modeling (FDM), a process accomplished by directly extruding out the materials with a heated printing nozzle (Figure 2A). By using both Euler-Bernoulli and Timoshenko beam principles, this research shows accurate analysis solutions for the stiffness matrix of thick hexagonal honeycombs. Finite element analysis reveals that the solutions are consistent with experimental and calculated values for honeycombs with very thick cell walls. However, the finite element models and experimental observations begin to deviate from each other when the relative density increases. Bulk cylinders (100% infill) with nominal diameters of 12.7 mm and nominal lengths of 25.4 mm were made and tested under compression, using a methodology like that used for the honeycombs. The measured elastic modulus and yield stress of the bulk material were 1.962 ± 0.069 GPa and 56.204 ± 1.213 MPa, respectively. This research contributes to the performed calculations for the elastic actions of hexagonal honeycomb configurations in the two main in-plane directions. It has also been found that as the relative density increases, the flexural stiffness of the cell-wall rises dramatically in comparison to the axial stiffness of the cell-wall until reaching a critical value. The results can be used to predict and optimize the mechanical properties of honeycomb produced by various designs. Another study on honeycomb-inspired structure is performed by using the same PLA material and FDM technology (Figure 2B). This study shows that the elastic modulus, compressive strength, and energy absorption per unit volume increase from 71.77, 2.16 MPa, 341 KJ/m3 for the L-EH sample with low density to 496.97, 5.96 MPa, 2,132 KJ/m3 for the L-FH-1 sample with high density (Yan et al., 2020).
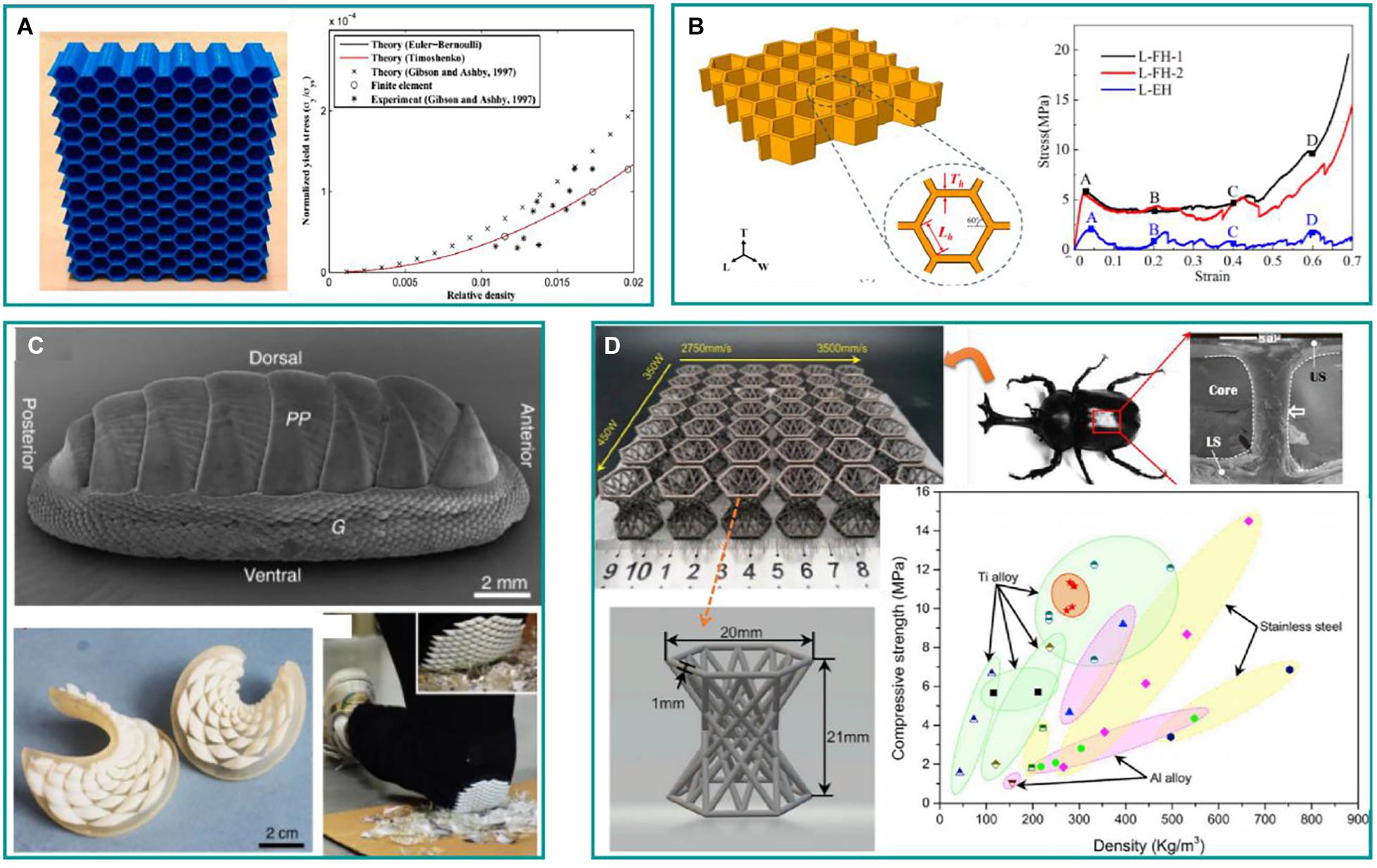
Figure 2. The 3D printing bioinspired reinforced structures. (A) Mechanical properties of 3D printed thick honeycombs (Hedayati et al., 2016); (B) honeycomb-inspired structures and the in-plane compressive stress-strain curves for different layer thicknesses (Yan et al., 2020); (C) chiton scale-inspired flexible armor using rigid polymers (Connors et al., 2019); (D) the adult Allomyrina dichotoma beetle-inspired structure with the metal lattice structure fabricated by selective laser melting (SLM) (Du et al., 2020).
Scale and Shell Inspired Structures
The studies on biomimetic 3D printing do not merely focus on bioinspired microstructures or microarchitectures, but also provide value by focusing on the unique phenomenon some creatures can generate (Baik et al., 2019).
Martini et al. received inspiration from fish scales to build flexible armor (Martini et al., 2017). They used 3D printing methods to build different acrylonitrile butadiene styrene (ABS) scale structures on polyurethane membranes. They built fish scale-inspired structures with simplified elasmoid, simplified ganoid, overlapped staggered, full ganoid, overlapped, and topologically interlocked structures. The results show that the average puncture resistance was the largest (13N) for the simplified elasmoid structures, but its flexural compliance was low. In comparison, the bioinspired full ganoid structure possessed both reinforced average puncture resistance (8.7 N) and flexural compliance (1–3.3 mm.N–1). The puncture resistance was reinforced by a factor of 16 for a bioinspired array of scales, compared to the isolated scales. But the flexural compliance decreased by a factor of 20. This result indicates that scale-scale interactions for 3D printed bioinspired structures, as in natural fish scale, significantly increases puncture resistance and decreases flexural compliance. Within the bioinspired designs of scale arrays, the geometries and arrangement of 3D printed fish scale structures show a better combination of mechanical properties than simplified elasmoid and ganoid structures. This study offers us an interesting way to improve the design of flexible armor with bioinspired design and reinforced mechanical properties.
Chiton scale-inspired flexible armor was studied to accurately mimic natural structures by using 3D printed polymer materials with a modulus of elasticity of 2 GPa (Connors et al., 2019). The 3D printed structure showed good flexibility, protection, could attach well to the human body, and demonstrated potential applications as a military or sports armor (Figure 2C). For 3D printing of bioinspired structures using metals, AlSi10Mg powder material was fabricated to mimic the beetle shell structures through selective laser melting (SLM) technology (Du et al., 2020). The effect on the microstructure and mechanical properties due to different laser powers were studied. The highest Fmax (2.95 kN) with the largest displacement value (1.18 mm) was achieved in the sample at 375 W. With the further increase of laser power to 450 W, the accumulated displacement drastically decreased. The 3D printed structure (with a low density of 270 kg/m3) showed a high compressive strength of 12 MPa, which was much higher than aluminum alloy and was comparable to titanium alloy (Figure 2D).
Multi-Materials
Currently, 3D printing can construct multi-material or composite systems and improve performance at user-defined locations (Ready et al., 2014; Tibbits, 2014; Bandyopadhyay and Heer, 2018). In a single component inspired by the natural structure, properties such as hardness, corrosion resistance, and environmental adaptability can be optimized in the areas where it is most needed. These new technologies can produce exciting multifunctional components, which is not possible with traditional single-material 3D printing (Truby and Lewis, 2016).
Bouligand Structure Materials
A Bouligand-type structure is a specific hierarchical arrangement that can achieve excellent mechanical properties while maintaining a small amount of mass (Zimmermann et al., 2013). The Bouligand-type arrangement found in Arapaima gigas is composed of fibril lamellae, each one is made from mineralized collagen fibrils with a dominated alignment. One challenge in manufacturing this arrangement is the difficulty in aligning the reinforcement phase in the matrix during fabrication. Through the study of small-angle XRD analysis while conducting mechanical tensile assessments of the Bouligand-type arrangement, the scale’s inner collagen layer can stretch and reorient the collagen lamellae mostly along the direction of tensile stress. One solution to this challenge is to apply additional fields to assist shape-changing anisotropy by 3D printing. In order to recreate such a unique reinforcement structure using 3D printing, several additional external fields will need to be applied. Sun et al., used a FDM method to build Bouligand-type structures with polylactic acid (PLA) by controlling the angles between different layers (Sun et al., 2020). The results show improved mechanical properties with the maximum ultimate strength of 57 MPa at 10 degrees and toughness of 1.4 N/mm2 at 15 degrees (Figure 3A). Moini et al. (2018), built Bouligand-type cement structures for applications in civil engineering and they found that it significantly improved performance over conventionally cast structures with equivalent density (Figure 3B). The structures possessed the highest modulus of rupture (MOR) of 4 MPa at a pitch angle of 8° attributed to the controllable failure through the interfaces and delocalization of cracks (Moini et al., 2018). The angle of rotation and the pitch angle tended to play an important role in shaping the fracture properties of Bouligand-structured materials. The results illustrate that deflections at maximum load of elements with Bouligand architectures were consistently higher than the deflections at maximum load of the cast elements. Specifically, the observed increases in the maximum load deflections were 5, 25, and 50% for architectures with γ = 8° (60% infill), γ = 45° (60% infill), and γ = 8° (solid, i.e., 100% infill), respectively. In general, the work of failure of the material increased with the rise in the angle of rotation and the exact modulus of rupture achieved a maximum peak at a pitch angle of 45°. Therefore, an optimum structure could be achieved by applying a Bouligand architecture while maintaining a moderate pitch angle. The pitch angles should be available to allow local hardening and support microcrack nucleation, while having the ability to avoid major crack propagation across the filaments.
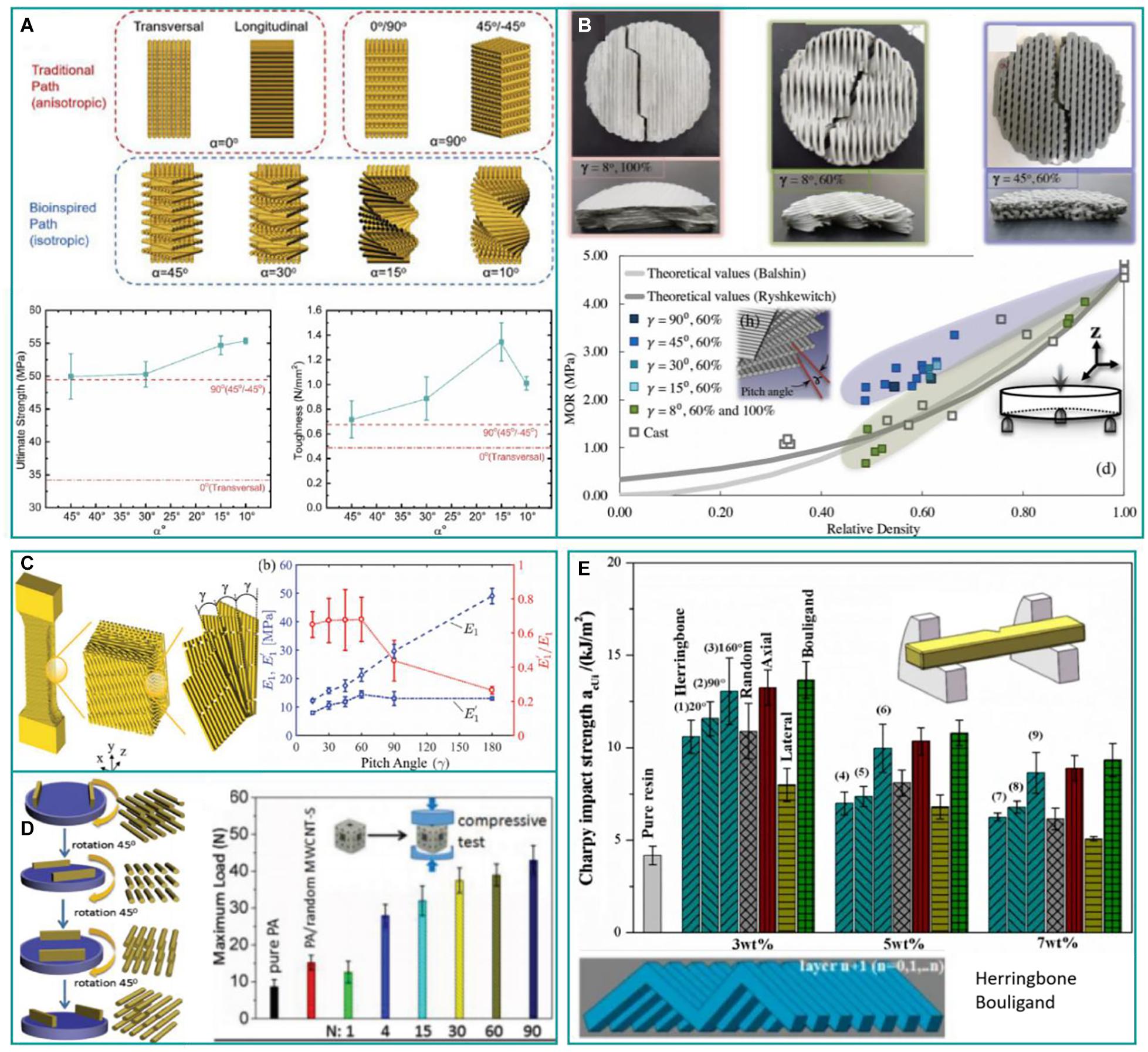
Figure 3. Schematic diagrams illustrate the study of 3D printing of Bouligand microstructures, (A) parallel-scan paths designed for tensile tests (Zimmermann et al., 2013; Sun et al., 2020); (B) modulus of rupture (MOR) versus relative density for Bouligand architectures with varying pitch angles and percentages of infill compared to MOR of cast control disks (Moini et al., 2018); (C) schematic representation of the helicoidal architecture (Zaheri et al., 2018); (D) electrically assisted 3D printing of biomimetic architectures with Bouligand-type MWCNT-S (Yang et al., 2017); (E) magnetic 3D printing of Bouligand structures and the comparison of impact strength of components with different ratios or embedded microstructures (Ren et al., 2018).
Similar to the Bouligand structure, the helicoid motif has also received tremendous interest in biomaterials. Zaheri et al. (2018), inspired by the helicoidal architectures of the cuticle of the Figeater beetle, studied the experimentation and analysis of the changes of the microstructure. This was achieved by conducting a mechanistic analysis and using 3D printing for the manufacture of fiber-reinforced synthetic helicoidal composites, which were made from a soft rubber-like polymer for the matrix and a rigid polymer for the fibers (Figure 3C; Zaheri et al., 2018). The stiffness degradation (E’1/E1) exhibited an inverse relation with pitch angles. The mechanical properties of the composite materials were inelastic, elastic, and destructive, and had a great dependence on the pitch angle. The flexibility of the spiral structure was confirmed further as the isotropy and fracture toughness were improved at lower pitch angles. The results showed that when the pitch angle increased, the overall stiffness significantly reduced during the inelastic deformation process. A smaller pitch angle also caused the fracture morphology to have a more clearly defined spiral pattern. This research could shed light on the mechanism of bioinspired design of discontinuous fibrous composite systems, and the optimization of its mechanical properties.
Yang et al. applied external electrical fields to control the alignment of carbon nanotubes (CNTs) in the resin matrix in order to fabricate Bouligand-type aligned surface functionalization MWCNT-S (Figure 3D; Yang et al., 2017). Moreover, Bouligand-type CNTs-reinforced composites can be used to fabricate several complex and functional materials by 3D printing. The electrically 3D printed artificial meniscus is one of them and possesses a slightly larger compressive modulus (0.79 MPa compared with 0.69 Mpa for natural meniscus) and better tensile modulus (176 MPa at rupture compared to natural meniscus’ 120 MPa at rupture). This has great possibility for application in mechanical, aerospace, and tissue engineering.
Ren et al. (2018) applied a magnetic field to the manufacturing process of 3D printing of biomimetic structural materials in order to achieve microstructures inspired from the “herringbone” helicoidal construction of mantis shrimp and Bouligand structure of Arapaima gigas (Figure 3E). The alignment of short carbon fibers as the reinforcement phase in a photocurable resin matrix relies on a rare earth magnet with an effective magnetic field that is placed on the xy-axis platform and moves over the layer to align the fiber into the designed architecture. While metal coatings and polymers have poor interface bonding characteristics, it can be improved by using a silane coupling treatment. Fiber-reinforced composites with herringbone and Bouligand microstructures have been successfully fabricated by 3D magnetic printing. With a smaller angle between the alignment fibers and the loading direction, the herringbone structure manifests a more prominent compressive resistance (compressive modulus of 27 MPa) while the Bouligand structure is the most salient for impact resistance (impact strength: 13 KJ/m2). Moreover, by designing the fiber alignment and distribution in a matrix, some customized properties and performances can be obtained. A Bouligand structure is not only achievable in metal polymer composites but also in ceramic polymer composites. Feilden et al. manufactured this structure by robocasting, which introduced shear forces by extrusion from a nozzle to align alumina platelets in a polymeric matrix (Feilden et al., 2017). One thing unique to this study was that the fabricated parts possessed a large concentration of alumina platelets (30 vol%) that is yet to be achieved by other manufacturing techniques. Inter-filament, trans-filament, and Bouligand structures were manufactured to study the mechanism of direct crack propagation and crack resistance in ceramic composites. Because of the strong network of alumina platelets with alumina powder due to sintering, the strength and toughness of trans-filament direction composites were comparatively higher than their counterparts while the three materials were relatively comparable in fracture toughness. This unique network not only rendered a high Young’s modulus, but also imparted a sufficiently higher hardness. These hierarchical structures can be manipulated by applying different velocity gradients on the rheology and extrusion of printed ink, including increasing the quantity of particles added. While enhancing mechanical properties and defect tolerance, the structure of the printed filaments simultaneously maintained higher specific strength, which can be applicable for the design of light-weight structural composites in aerospace engineering.
Balsa Wood Structures by Extrusion
Balsa wood-inspired structures are widely studied and fabricated by extrusion-based 3D printing methods due to the alignment of fibers by shear force during the extrusion process. Not only is the surface structure produced and managed to ameliorate the total mechanical properties of materials, but the performance could also be improved by creating unique inner layouts (Jiang et al., 2019b). Lightweight cellular composites are one high-performance structural material that possess a bright future toward applications in load carrying, energy absorption, vibration damping, and insulation (Al-Ketan et al., 2018). The structure of balsa wood is one of nature’s cellular materials that has outstanding strength-to-weight ratios and stiffness-to-weight, as well as excellent energy absorption (Da Silva and Kyriakides, 2007). Compton et al. prepared a biomimetic structure of balsa wood produced by 3D direct ink writing which oriented milled carbon fiber (220 μm length) in epoxy resin along the printing direction through shear force (Figure 4A; Compton and Lewis, 2014). The Young’s modulus of the tensile bars printed by this process was approximately 10 times (24.5 GPa for SiC/C-filled longitudinal specimens) higher than commercial 3D printed epoxy resins (2.66 GPa). Moreover, stiffness can be graded around fixture points or reinforcements can be aligned near geometric stress concentrators to minimize damage. This new emerging technology of 3D printing not only exhibits a novel time-saving manufacturing method, but also reduces unnecessary costs during production.
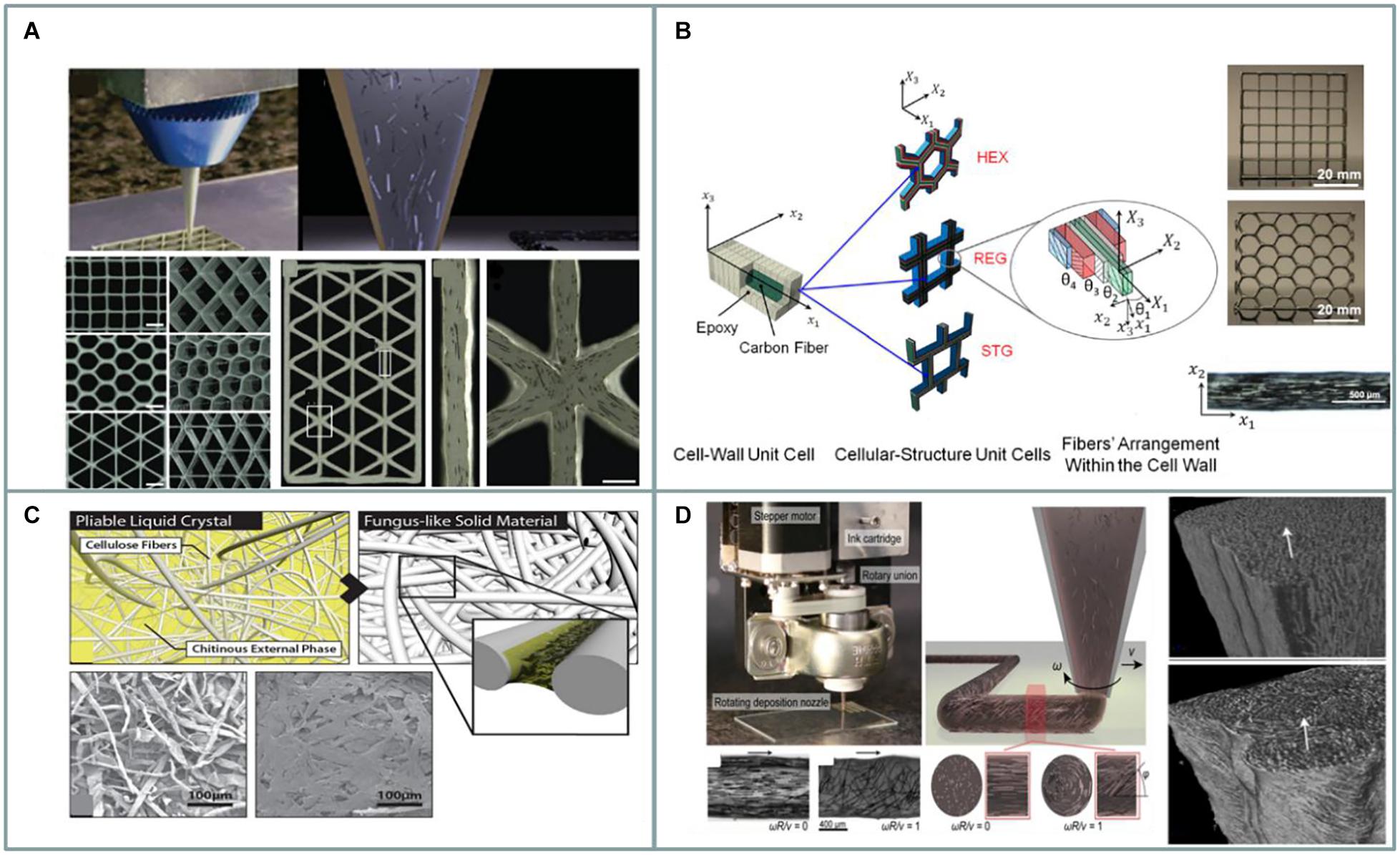
Figure 4. Schematic diagrams illustrate the aligned fiber structures in balsa wood. (A) Optical image of a 3D printed triangular honeycomb composite with aligned SiC/C-fiber (Compton and Lewis, 2014); (B) schematic diagram of a multiscale method for modeling porous composite materials inspired by balsa wood. Different colors represent eight layers with different fiber orientations at the cellular structure level (Malek et al., 2017); (C) supramolecular organization of fungus-like mimetic materials (Sanandiya et al., 2018); (D) schematic diagram showing fiber orientation when rotating 3D printing by rotating a nozzle to obtain the helical pattern (Raney et al., 2018).
Malek et al. (2017) fabricated a light cellular configuration inspired from balsa wood that possessed fiber-reinforced composite cell walls and yielded improved mechanical properties (Figure 4B; Malek et al., 2017). Balsa wood is generally constituted from three types of cells: rays, fibers, and vessels. Fibers provide basic structural support to the trees. Ray cells have approximately hexagonal honeycombs and are responsible for sugar storage. Vessels which represent thin-walled cylindrical shapes assist with the conduct of fluids in the tree. The structures are prepared by 3D direct ink writing, starting with epoxy resin as ink, nano-clay as a rheological modifier, then dimethyl methylphosphonate and short carbon fibers are added as reinforcements. Focusing on the elastic properties of regular hexagonal cells, regular square cells, and staggered square cells, researchers can study the effect brought by these different parameters. The volume fraction, aspect ratio, the orientation and distribution of carbon fibers, and geometrical design of cellular material structures contribute to the elastic properties of printed structures. By arranging carbon fibers in the in-plane loading direction, the Young’s modulus of porous composite material with a regular square structure is six times greater than that of balsa wood. The printed materials with longitudinal Young’s modulus can be up to 57 GPa (exceeding the longitudinal modulus of wood cell wall material). Moreover, the same cellular structure possesses higher shear moduli than that of balsa when the fiber orientation is at θ = ± 45°. Meanwhile, a multi-scale computational model has been tested to design cellular materials with enhanced mechanical properties based on experimental data from the printed cellular structures fabricated from short carbon fiber and epoxy resin.
Another biomimetic cellulosic material that has received dramatic attention is fungal-like adhesive materials (FLAM). Inspired by the fungal oomycete wall, Sanandiya et al. (2018) invented a new approach to fabricate composites using chitin as a matrix with cellulose fibers and wood floor as reinforcements. This approach created a FLAM with the advantages of being strong, lightweight, and cost-saving (Figure 4C; Sanandiya et al., 2018). Since the raw materials used are abundant in nature and biodegradable, they are completely environmentally friendly. The results showed that under a small amount of chitosan (<8%), composites exhibited slender mechanical features. While composites generated larger internal forces and undermined the structural integrity with high amounts of chitosan (>17%) and gave a Young’s modulus of 0.26 GPa and a density of 0.37 g/cm3. This research also highlights a unique application of a direct ink writing (DIW)-based large-scale 3D printing devices for FLAM materials to fabricate a turbine blade and improve its surface finish and functionalities.
It is possible to use an external field in combination with a printing system to better adjust the orientation in the fiber structure and prefabricated materials (Kokkinis et al., 2015; Llewellyn-Jones et al., 2016). In addition to field-assisted 3D printing to fabricate metamaterials, Jordan et al. invented a rotating 3D printing approach, which allowed the spatially controlled alignment of short fibers within the resin matrix, together with adjusting the printing speed and nozzle rotating velocity to produce short carbon fiber-epoxy composites (Figure 4D; Raney et al., 2018). This approach led to an optimizable combination of stiffness, toughness, and damage tolerance in composites. One in three compositions of carbon fiber (18.5 vol% carbon fibers) and resin showed a lower failure strain and a much higher stiffness on curing (with elastic modulus of the longitudinal (EL∼10 GPa) and transverse (ET∼2 GPa) tensile bars). Materials with higher load carried, higher stiffness before fracture, and better energy absorption efficiency can be produced by rotation compared to samples printed without rotation. In addition, fiber-reinforced composites with high-performance and effectively isotropic elastic features can be obtained when sufficiently fast rotating rates (Rω /v ≥ 5) are applied. However, fiber alignment has less effect on materials when conducting tensile strength tests than it does on stiffness. The study also found that the mechanical features of printed materials were dramatically improved through internal local helical fiber arrangement, without any modifications to the print path, external geometry, or material ratio. As a result, rotational direct ink writing enabled the fabrication of short-fiber strengthened composites, which exhibited programmable failures in a controlled form. Due to its simplicity and attainability, rotation direct ink writing can be explored in broader matrix combinations and anisotropic fillers, wherein the controlled mechanical, thermal, optical, and electrical properties can be obtained by manipulating the arrangement of filler in composites.
Nacre Structure
The “brick-and-mortar” structure of nacre is the most seen biomimetic design in nature, this structure can be used as a lightweight and strong protective shelter for sports applications, aerospace, and other related fields. Tran et al. also presented a 3D printing approach of fabricating nacre-inspired Voronoi-based composite structures (Figure 5B; Tran et al., 2017), which enabled the fabrication of lightweight and strong structures that can be used for various potential applications. Yang et al. presented a novel way to fabricate nacre-inspired multifunctional devices by 3D printing (Figure 5C; Yang et al., 2019). Electrically assisted 3D printing was used to align graphene nanoplatelets (GNs), which act as bricks and the polymer in between as mortar. The mechanical/electrical properties of 3D printed nacre were studied and the nacre-inspired structures with aligned GNs and the structures with random GNs were compared. The 3D printed nacre-inspired structure showed a fracture toughness of 1.59 MPa m1/2, which was twice of that for pure resin. In the self-sensing test of anisotropic electrical properties, the resistance of the composite with aligned GNs (104 ohm) was 102 lower than that with randomly distributed GNs (106 ohm). The impact tests showed that the helmet produced with random GNs was completely damaged, but the one with aligned GNs still remained intact. With aligned GNs, the 3D printed structural materials showed improved fracture toughness, impact resistance, and electrical conductivity.
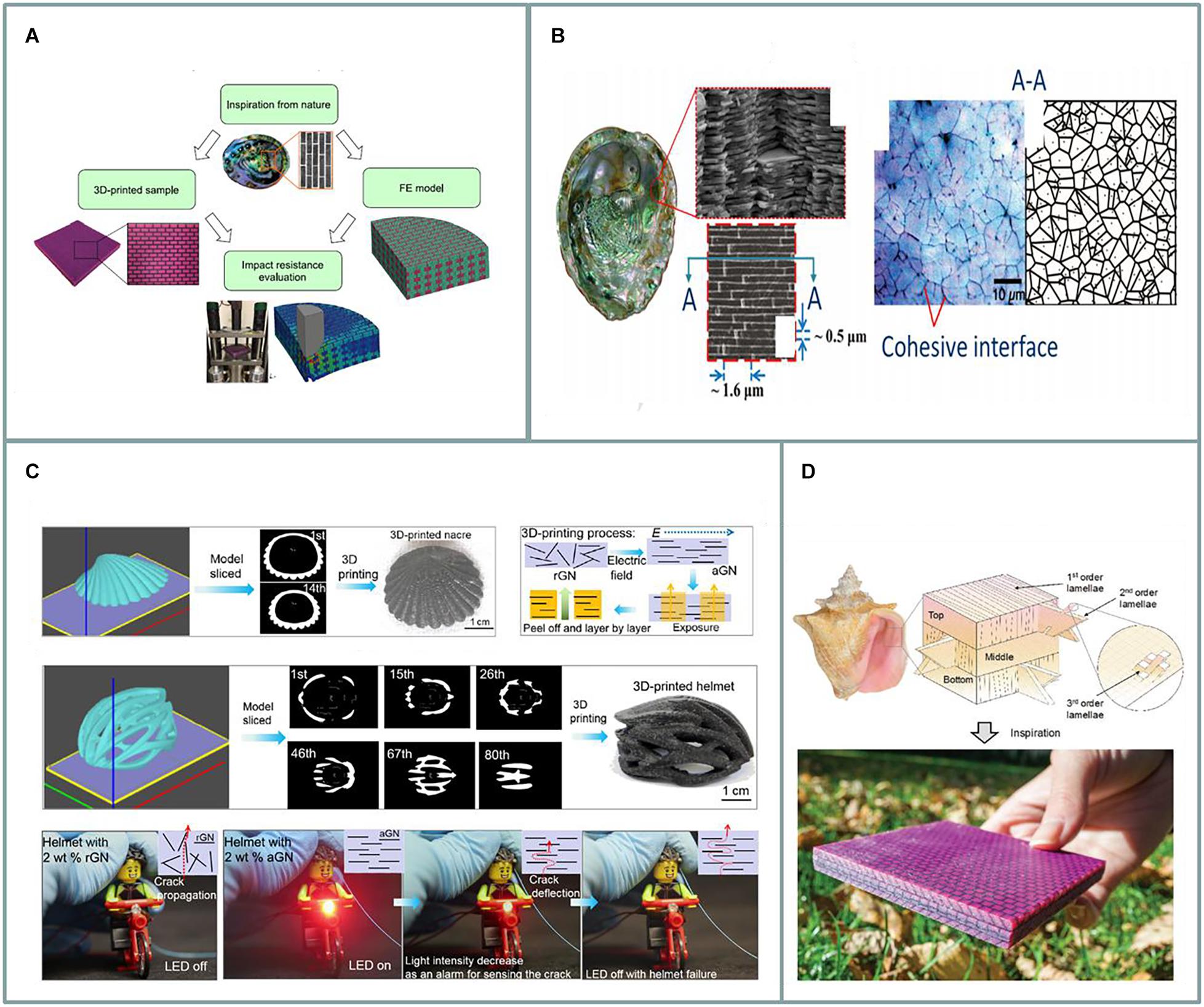
Figure 5. Schematic diagrams illustrate the microstructure of nacre. (A) 3D printing of nacre-inspired structures guided by a finite element (FE) model (Gu et al., 2016); (B) microstructures of abalone shell shows the brick and mortar structure (Tran et al., 2017); (C) nacre model and self-sensing smart helmet with anisotropic electrical property produced by electrically assisted 3D printing (Yang et al., 2019); (D) 3D printing of conch shell-inspired three-tier calcium carbonate cross-lamellar structure (Gu et al., 2017).
As the study of biomimetics deepens, it has been revealed that the toughness of conch shell surpasses nacre by an order of magnitude. Inspired by the multiscale architectures of seashells and conch shells, Gu et al. invented alternating cross-lamellar hierarchy packing structures and fabricated hierarchically enhanced impact resistance composites by applying stereolithography (Figure 5A; Gu et al., 2017). Research showed that the impact performance can be increased 70–85% by the design of a second level of cross-lamellar hierarchy rather than a single-level hierarchy and bulk stiff material. The structure of conch shells is composed of three layers, a first (5 μm in thickness), second (5–30 μm thickness, 5–60 μm wide), and third layer (60–100 nm thickness, 100–380 nm wide) of ordered aragonite lamellae. Drop tower impact testing was used to study the impact performance of printed architectures and analyze the energy dissipating mechanism of crack propagation. The research highlighted a complex architectural layout that can apply controllable crack propagation by depositing alternating layers of lamellae for cracks to propagate through the interfaces. This was done through a prolix zig-zag path which increased the energy dissipation. In addition, the study found that weaker interfacial strength led to larger angles of deflection, while early delamination could occur when interfacial strength became weaker. Therefore, there is a balance between the interfacial bonding of layers. This analysis also suggests that applying an angle of about 50° could greatly inhibit crack deflection and achieve anti-perforation. In summary, the hierarchical architecture of conch shells enables a novel approach for the design of high-performance body armor and helmets. However, most of the research on nacre-inspired structures are restricted to tension/compression tests. Gu et al. further developed multi-material nacre-inspired designs with distinctive performances and assembled them in a ply to mimic the structure of nacres and then 3D printed for impact testing (Figure 5D; Gu et al., 2016). Again, the impact testing was conducted by a drop tower and the result showed that nacre-like materials outperformed each of its constituent materials in impact resistance. Moreover, the force-displacement test results were consistent with finite element modeling. The dynamic mechanical properties of nacre-inspired 3D printed structures showed a capability to prevent perforation of the dropped material with the brittle failure for bulk stiff material upon impact testing. This study demonstrated that a combination of 3D printing and finite element simulation is critical in understanding the mechanism of the key contribution to novel impact resistant materials.
Apart from the structure of nacre and conch shells, the ocean also provides us with a lesson in biomimetic design from its many creatures. The hierarchical structure of whale baleen contributes to the study of fracture toughness in unique performance requirements. Whale baleen is a keratin-based anisotropic biomaterial which exhibits high fracture toughness when subjected to different loading orientations and rates. Wang et al. created a 3D printing prototype and it was applied to fabricate three principal structures of the baleen plate (hollow medulla, sandwich-tubular, and mineralized tubules), and the mechanical properties of these three distinctive structures were also analyzed, respectively (Wang et al., 2019). The anisotropic and hierarchical structure provided an efficient approach to study how structure interacts with competing factors of hydration and loading conditions in fracture. The results showed that incorporating the stiff “mineral” component can effectively increase the stiffness and strength. It was indicated that by applying advanced multi-material 3D printed prototypes, the hierarchical structure manifests its significance on the mechanical properties. A better response to a mechanical stimulus can be further achieved by designing a solid shell and hollow tubules with filament-matrix lamellae and mineralization.
Shape-Changing Materials
Bioinspired structures and bioinspired motion can benefit the mechanical properties of artificial materials (Singh et al., 2012; Kim et al., 2013). Arslan et al. (2019) discovered a bioinspired design that applies linear hydrogel actuators fabricated from a temperature-unresponsive filler [poly (ethylene glycol) (PEG)] and a reinforced temperature-responsive polymer [poly(N-isopropylacrylamide) (PNIPAM)] (Figure 6B). The actuator introduced a saddle-like shape change and other motions by the manipulation of the geometrical design and the orientation of the components under certain temperatures. Moreover, the 3D structures with anisotropic PEG alignments could achieve linear actuation to approximately 210% with around 110% strain in the direction perpendicular and longitudinal to the PEG reinforcement direction. The major direction of actuation, from shrinking to swelling state was about sixfold higher than that in the transverse direction (nearly 20% strain), and the motion could be manipulated by the alignment of PEG patterns. This research also discovered a lower limit of critical modulus (15 kPa) sufficient for printing self-supporting 3D structures. Inspired by natural hygromorphs and seed pods due to their shape transformations, the reinforcement structure induced bending and twisting motions by responding to the stimulus of humidity to mimic the motions of biological organisms. The results showed that the bioinspired shape changing from the modular assembly of functional components has huge potential in fabricating soft devices with bioinspired motions due to stimulus-induced control mechanisms.
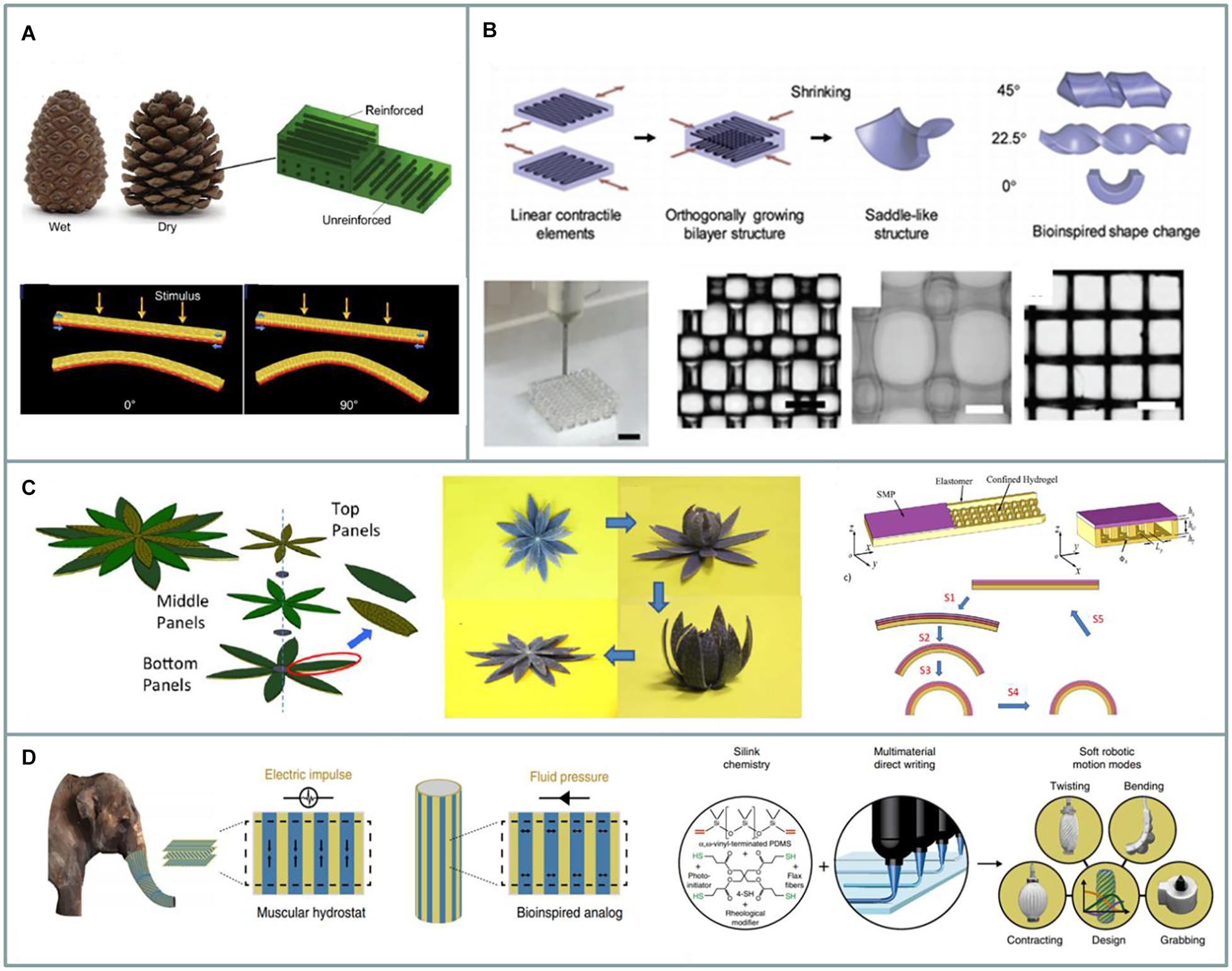
Figure 6. Schematic diagrams representing shape-changing material structures via 3D printing. (A) Schematic of the architecture of pine cones and the shape-changing behavior under stimulus (Ren et al., 2019); (B) programmed motions of bilayer structures of hydrogels by 3D printing (Arslan et al., 2019); (C) two activated shape memory petal-like structures and the design principle of the reversible actuator (Mao et al., 2016); (D) 3D printed bioinspired soft actuators drawing inspiration from elephant trunks (Schaffner et al., 2018).
Mao et al. studied a reversible shape-changing component designed by 3D printing of shape memory polymers and hydrogels (Figure 6C; Mao et al., 2016). The driving force of shape changing comes from the swelling of a hydrogel. The regulation of shape-changing time is attributed to the temperature-dependent modulus of the shape memory polymer. The switch between stable configurations is achieved by temperature and the aqueous environment. The design of 3D printed architectures and its interaction with multi-materials can generate controllable shape-changing behavior, such as bending and twisting. Various kinds of 2D and 3D shape-changing components were fabricated in this study. Mechanisms with different parameters were studied, demonstrating potential applications as robotic arms or in drug delivery. Additionally, bioinspired soft actuators have attracted attention because of the safety involved in their interaction with humans and their surroundings (Figure 6D; Schaffner et al., 2018). Research progress on soft actuators has been hindered by current manufacturing technology’s difficulty in fabricating complex shapes and architectures with multi-materials. Drawing inspiration from the elephant trunk, which is an effective bio-actuator in nature, Schaffner et al. reported on a bioinspired trunk by 3D printing. This silicon actuator exhibited programmable bioinspired architectures and motions. Inspired by the fibrous structures in muscular hydrostats in elephant trunks, the surface of the silicon actuator was decorated with reinforcing stripes at a well-defined lead angle. The specially designed lead angle could be controlled to achieve different motions including elongation, contraction, and twisting. This study furthers the progress of soft actuators with accurately controlled motion, which have potential applications in fields that need safe robot to human interaction.
Liquid resin curing has been extensively applied in 3D printing because of its simplicity and accessibility (Manapat et al., 2017). However, the fabrication resolution and printing speed are restricted by the adhesion of in situ cured resin with previous layers at the curing interface. In order to solve this problem, Wu et al. (2018) fabricated a pitcher plant-inspired slippery surface by DLP 3D printing to decrease both the vertical and horizontal adhesion. This research presented a way of producing a slippery surface by 3D printing to effectively increase the printing speed with ultra-low adhesive energy at the curing interface and increment the refilling speed of liquid resin. Parameters including lifting velocities of the supporting plate, light intensities, and switching speed of the UV projector were studied. Additionally, the time for fabrication was determined by the printing height. This bioinspired 2D slippery interface was effective in producing 3D structures with detailed features with a fast speed and low-cost.
Some novel technologies of 3D printing were invented by introducing external fields in assisting the fabrication of various metamaterials (Samuel et al., 2015; Huber et al., 2016). One of the external fields we mentioned before was a nacre-inspired structure nanocomposite fabricated with an electrical field (Yang et al., 2019). Discontinuous fiber composites have excellent mechanical properties and are lightweight because the microstructure of composites plays a significant role in determining the fracture mechanism. Martin et al. applied the technology of 3D magnetic printing based on an SLA framework to design and recreate complex bioinspired reinforcement architectures of discontinuous fiber composites (Martin et al., 2015). The reinforcement architectures were achieved by applying magnetic fields that align the anisotropic reinforcement phase (such as magnetic responsive alumina, silica, and calcium phosphate coated with iron oxide nanoparticles) during the process of printing. This technique enabled the designed orientation of discontinuous fiber to be fulfilled for several biomimetic structural systems. Microstructures play an important role in explaining fracture mechanics in heterogenous natural composites. The fracture mechanisms of 3D magnetic printed architectures represented crack steering for when the crack propagated and different orientations of reinforcement phase would result in tremendous outcomes. Crack steering offers a plausible solution to explain the fracture toughening mechanisms in composites designs. These results indicated that magnetic field-assisted 3D printing allows for the formation of microstructures that increase the crack deflection path. Therefore, they predict and manipulate the propagation pathways of cracks to avoid critical failure in composites. Similarly, Ren et al. used magnetic field assembly and slurry-based 3D printing to fabricate shape memory composites with bioinspired fiber architecture designs (Figure 6A; Ren et al., 2019). First, the sieved short steel fibers were mixed with clear resin and flexible resin for 3D printing. The 3D printed structure showed a bending behavior toward the opposite direction of illuminated UV light by the release of stress. Most importantly, the printed samples could be heated to 60°C, molded, and then cooled to 20°C to keep the temporary shape. Then the samples could be molded to recover the permanent shape by reheating to 60°C. The produced fiber-regulated shape memory composites could be fabricated into a self-folded flower structure which deployed smart robotics and inspires promising applications for 4D printing in soft robotics.
Conclusion and Perspective
After millions of years of evolution, natural structures and materials have evolved excellent mechanical properties. But these natural structures are often too complicated and far out of reach of traditional manufacturing technology. Additive manufacturing (3D printing) has shown a great advantage in the design and building of bioinspired structures due to its capability in fabricating complex structures. Further development of bioinspired 3D printing of mechanical reinforced structures will depend on the development of materials and structures to further improve the tensile modulus, impact resistance, and toughness. Also new 3D printing processes need to be developed such as higher resolution, multi-material capability, larger printing area, and lower cost fabrication. Electric/magnetic/acoustic field-assisted 3D printing has limitations in low loading of fillers, increasing the filler content, and controlling the alignment in high viscosity slurry. Most studies are focused on the reinforcement of single mechanical properties by using bioinspired designs. Recently, the research of bioinspired 3D printing has changed from the study of a single functional property to multi-functional properties because most natural structures possess multi-functional properties (such as the combination of mechanical/electrical/thermal properties). As shown in the research presented in section “Nacre Structure,” the replication of nacre-inspired structures by multi-materials still has limitations due to the scale difference and material difference. Multi-type polymers are used while natural nacre developed excellent structures by combining ceramic and polymers. This as well as the interlocking bond between each layer is difficult to achieve right now. This difficulty remains as a principal challenge in building bioinspired multi-material structures with polymers, ceramic, and metals. External field-assisted 3D printing technology shows excellent capability in building bioinspired structures, but currently the size of the printed sample is limited to centimeters. Building large size structures for practical applications in vehicles, armor, and aerospace engineering is still challenging. In addition, some of the structures in nature include a different alignment of interlock fillers in a single layer and further study on this topic is needed. Further study on bioinspired 3D printing lies in the development of multi-material printing technology, high-resolution printing as well as using 3D printing to assist with traditional technologies (Gräfe et al., 2018; Emon et al., 2019; Reiser et al., 2019). Fortunately, the rapid development of today’s manufacturing and technology environments are fueling progress in bioinspired 3D printing technology. To achieve this goal, future developments in next-generation nature-inspired structures using new materials and new 3D printing processes will need to be eco-friendly, reduce costs, employ green energy, and have advanced structural design improvements. Overall, understanding the mechanisms of natural structures can inspire the development of 3D printing processes that will play important roles in future engineering applications such as body armor, robotic arms, drug delivery, etc.
Author Contributions
All authors contribute to the writing and revision of this review.
Conflict of Interest
The authors declare that the research was conducted in the absence of any commercial or financial relationships that could be construed as a potential conflict of interest.
References
Al-Ketan, O., Soliman, A., Alqubaisi, A. M., and Abu Al-Rub, R. K. (2018). Nature-inspired lightweight cellular co-continuous composites with architected periodic gyroidal structures. Adv. Eng. Mater. 20:1700549. doi: 10.1002/adem.201700549
Arslan, H., Nojoomi, A., Jeon, J., and Yum, K. (2019). 3D printing of anisotropic hydrogels with bioinspired motion. Adv. Sci. 6:1800703. doi: 10.1002/advs.201800703
Baik, S., Lee, H. J., Kim, D. W., Kim, J. W., Lee, Y., and Pang, C. (2019). Bioinspired adhesive architectures: from skin patch to integrated bioelectronics. Adv. Mater. 31:1803309. doi: 10.1002/adma.201803309
Bandyopadhyay, A., and Heer, B. (2018). Additive manufacturing of multi-material structures. Mater. Sci. Eng. R Rep. 129, 1–16.
Catania, K. C. (2012). Evolution of brains and behavior for optimal foraging: a tale of two predators. Proc. Natl. Acad. Sci. U.S.A. 109, 10701–10708. doi: 10.1073/pnas.1201885109
Chung, T., Lee, Y., Yang, S. P., Kim, K., Kang, B. H., and Jeong, K. H. (2018). Mining the smartness of insect ultrastructures for advanced imaging and illumination. Adv. Funct. Mater. 28:1705912. doi: 10.1002/adfm.201705912
Compton, B. G., and Lewis, J. A. (2014). 3D−printing of lightweight cellular composites. Adv. Mater. 26, 5930–5935. doi: 10.1002/adma.201401804
Connors, M., Yang, T., Hosny, A., Deng, Z., Yazdandoost, F., Massaadi, H., et al. (2019). Bioinspired design of flexible armor based on chiton scales. Nat. Commun. 10:5413.
Cui, Y., Gong, H., Wang, Y., Li, D., and Bai, H. (2018). A thermally insulating textile inspired by polar bear hair. Adv. Mater. 30:1706807. doi: 10.1002/adma.201706807
Cully, A., Clune, J., Tarapore, D., and Mouret, J.-B. (2015). Robots that can adapt like animals. Nature 521, 503–507. doi: 10.1038/nature14422
Da Silva, A., and Kyriakides, S. (2007). Compressive response and failure of balsa wood. Inter. J. Solids Struct. 44, 8685–8717. doi: 10.1016/j.ijsolstr.2007.07.003
Du, Y., Gu, D., Xi, L., Dai, D., Gao, T., Zhu, J., et al. (2020). Laser additive manufacturing of bio-inspired lattice structure: forming quality, microstructure and energy absorption behavior. Mater. Sci. Eng. A 773:138857. doi: 10.1016/j.msea.2019.138857
Emon, M. O. F., Alkadi, F., Philip, D. G., Kim, D.-H., Lee, K.-C., and Choi, J.-W. (2019). Multi-material 3D printing of a soft pressure sensor. Addit. Manuf. 28, 629–638. doi: 10.1016/j.addma.2019.06.001
Feilden, E., Ferraro, C., Zhang, Q., García-Tuñón, E., D’elia, E., Giuliani, F., et al. (2017). 3D printing bioinspired ceramic composites. Sci. Rep. 7:13759.
Gräfe, D., Wickberg, A., Zieger, M. M., Wegener, M., Blasco, E., and Barner-Kowollik, C. (2018). Adding chemically selective subtraction to multi-material 3D additive manufacturing. Nat. Commun. 9:2788.
Gu, G. X., Takaffoli, M., and Buehler, M. J. (2017). Hierarchically enhanced impact resistance of bioinspired composites. Adv. Mater. 29:1700060. doi: 10.1002/adma.201700060
Gu, G. X., Takaffoli, M., Hsieh, A. J., and Buehler, M. J. (2016). Biomimetic additive manufactured polymer composites for improved impact resistance. Extreme Mech. Lett. 9, 317–323. doi: 10.1016/j.eml.2016.09.006
Hedayati, R., Sadighi, M., Mohammadi Aghdam, M., and Zadpoor, A. (2016). Mechanical properties of additively manufactured thick honeycombs. Materials 9:613. doi: 10.3390/ma9080613
Herzog, D., Seyda, V., Wycisk, E., and Emmelmann, C. (2016). Additive manufacturing of metals. Acta Mater. 117, 371–392. doi: 10.1016/j.actamat.2016.07.019
Huber, C., Abert, C., Bruckner, F., Groenefeld, M., Muthsam, O., Schuschnigg, S., et al. (2016). 3D print of polymer bonded rare-earth magnets, and 3D magnetic field scanning with an end-user 3D printer. Appl. Phys. Lett. 109:162401. doi: 10.1063/1.4964856
Jiang, L., Tan, Z., Xie, L., Li, Y., Xing, J., Wu, J., et al. (2018). Study of the relationships among the crystal structure, phase transition behavior and macroscopic properties of modified (K, Na) NbO3-based lead-free piezoceramics. J. Eur. Ceram. Soc. 38, 2335–2343. doi: 10.1016/j.jeurceramsoc.2017.12.062
Jiang, L., Yang, Y., Chen, R., Lu, G., Li, R., Li, D., et al. (2019a). Flexible piezoelectric ultrasonic energy harvester array for bio-implantable wireless generator. Nano Energy 56, 216–224. doi: 10.1016/j.nanoen.2018.11.052
Jiang, L., Yang, Y., Chen, R., Lu, G., Li, R., Xing, J., et al. (2019b). Ultrasound−induced wireless energy harvesting for potential retinal electrical stimulation application. Adv. Funct. Mater. 29:1902522. doi: 10.1002/adfm.201902522
Kim, H., Lee, H., Ha, I., Jung, J., Won, P., Cho, H., et al. (2018). Biomimetic color changing anisotropic soft actuators with integrated metal nanowire percolation network transparent heaters for soft robotics. Adv. Funct. Mater. 28:1801847. doi: 10.1002/adfm.201801847
Kim, S., Laschi, C., and Trimmer, B. (2013). Soft robotics: a bioinspired evolution in robotics. Trends Biotechnol. 31, 287–294. doi: 10.1016/j.tibtech.2013.03.002
Kokkinis, D., Schaffner, M., and Studart, A. R. (2015). Multimaterial magnetically assisted 3D printing of composite materials. Nat. Commun. 6:8643.
Llewellyn-Jones, T. M., Drinkwater, B. W., and Trask, R. S. (2016). 3D printed components with ultrasonically arranged microscale structure. Smart Mater. Struct. 25:02LT01. doi: 10.1088/0964-1726/25/2/02lt01
Malek, S., Raney, J. R., Lewis, J. A., and Gibson, L. J. (2017). Lightweight 3D cellular composites inspired by balsa. Bioinspir. Biomim. 12:026014. doi: 10.1088/1748-3190/aa6028
Manapat, J. Z., Chen, Q., Ye, P., and Advincula, R. C. (2017). 3D printing of polymer nanocomposites via stereolithography. Macromol. Mater. Eng. 302:1600553. doi: 10.1002/mame.201600553
Mao, Y., Ding, Z., Yuan, C., Ai, S., Isakov, M., Wu, J., et al. (2016). 3D printed reversible shape changing components with stimuli responsive materials. Sci. Rep. 6:24761.
Martin, J. J., Fiore, B. E., and Erb, R. M. (2015). Designing bioinspired composite reinforcement architectures via 3D magnetic printing. Nat. Commun. 6:8641.
Martini, R., Balit, Y., and Barthelat, F. (2017). A comparative study of bio-inspired protective scales using 3D printing and mechanical testing. Acta Biomater. 55, 360–372. doi: 10.1016/j.actbio.2017.03.025
Meyers, M. A., Chen, P.-Y., Lin, A. Y.-M., and Seki, Y. (2008). Biological materials: structure and mechanical properties. Prog. Mater. Sci. 53, 1–206.
Moini, M., Olek, J., Youngblood, J. P., Magee, B., and Zavattieri, P. D. (2018). Additive manufacturing and performance of architectured cement−based materials. Adv. Mater. 30:e1802123.
Ngo, T. D., Kashani, A., Imbalzano, G., Nguyen, K. T., and Hui, D. (2018). Additive manufacturing (3D printing): a review of materials, methods, applications and challenges. Compos. B Eng. 143, 172–196. doi: 10.1016/j.compositesb.2018.02.012
Raney, J. R., Compton, B. G., Mueller, J., Ober, T. J., Shea, K., and Lewis, J. A. (2018). Rotational 3D printing of damage-tolerant composites with programmable mechanics. Proc. Natl. Acad. Sci. U.S.A. 115, 1198–1203. doi: 10.1073/pnas.1715157115
Ready, S., Whiting, G., and Ng, T. N. (2014). “Multi-material 3D printing,” in Proceedings of the NIP & Digital Fabrication Conference: Society for Imaging Science and Technology, Žilina, 120–123.
Reiser, A., Lindén, M., Rohner, P., Marchand, A., Galinski, H., Sologubenko, A. S., et al. (2019). Multi-metal electrohydrodynamic redox 3D printing at the submicron scale. Nat. Commun. 10:1234567890.
Ren, L., Li, B., Song, Z., Liu, Q., Ren, L., and Zhou, X. (2019). Bioinspired fiber-regulated composite with tunable permanent shape and shape memory properties via 3d magnetic printing. Compos. B Eng. 164, 458–466. doi: 10.1016/j.compositesb.2019.01.061
Ren, L., Zhou, X., Liu, Q., Liang, Y., Song, Z., Zhang, B., et al. (2018). 3D magnetic printing of bio-inspired composites with tunable mechanical properties. J. Mater. Sci. 53, 14274–14286. doi: 10.1007/s10853-018-2447-5
Rogóż, M., Zeng, H., Xuan, C., Wiersma, D. S., and Wasylczyk, P. (2016). Light−driven soft robot mimics caterpillar locomotion in natural scale. Adv. Opt. Mater. 4, 1689–1694. doi: 10.1002/adom.201600503
Samuel, B. P., Pinto, C., Pietila, T., and Vettukattil, J. J. (2015). Ultrasound-derived three-dimensional printing in congenital heart disease. J. Digit. Imaging 28, 459–461. doi: 10.1007/s10278-014-9761-5
Sanandiya, N. D., Vijay, Y., Dimopoulou, M., Dritsas, S., and Fernandez, J. G. (2018). Large-scale additive manufacturing with bioinspired cellulosic materials. Sci. Rep. 8:8642.
Schaffner, M., Faber, J. A., Pianegonda, L., Rühs, P. A., Coulter, F., and Studart, A. R. (2018). 3D printing of robotic soft actuators with programmable bioinspired architectures. Nat. Commun. 9:878.
Singh, A. V., Rahman, A., Kumar, N. S., Aditi, A., Galluzzi, M., Bovio, S., et al. (2012). Bio-inspired approaches to design smart fabrics. Mater. Des. 36, 829–839.
Speck, O., Speck, D., Horn, R., Gantner, J., and Sedlbauer, K. P. (2017). Biomimetic bio-inspired biomorph sustainable? An attempt to classify and clarify biology-derived technical developments. Bioinspir. Biomim. 12:011004. doi: 10.1088/1748-3190/12/1/011004
Sun, Y., Tian, W., Zhang, T., Chen, P., and Li, M. (2020). Strength and toughness enhancement in 3d printing via bioinspired tool path. Mater. Des. 185:108239. doi: 10.1016/j.matdes.2019.108239
Tibbits, S. (2014). 4D printing: multi−material shape change. Archit. Des. 84, 116–121. doi: 10.1002/ad.1710
Tran, P., Ngo, T. D., Ghazlan, A., and Hui, D. (2017). Bimaterial 3D printing and numerical analysis of bio-inspired composite structures under in-plane and transverse loadings. Compos. Eng. 108, 210–223. doi: 10.1016/j.compositesb.2016.09.083
Truby, R. L., and Lewis, J. A. (2016). Printing soft matter in three dimensions. Nature 540, 371–378. doi: 10.1038/nature21003
Wang, B., Sullivan, T. N., Pissarenko, A., Zaheri, A., Espinosa, H. D., and Meyers, M. A. (2019). Lessons from the ocean: whale baleen fracture resistance. Adv. Mater. 31:1804574. doi: 10.1002/adma.201804574
Wang, X., Jiang, M., Zhou, Z., Gou, J., and Hui, D. (2017). 3D printing of polymer matrix composites: a review and prospective. Compos. B Eng. 110, 442–458. doi: 10.1016/j.compositesb.2016.11.034
Wu, L., Dong, Z., Du, H., Li, C., Fang, N., and Song, Y. (2018). Bioinspired ultra-low adhesive energy interface for continuous 3d printing: reducing curing induced adhesion. Research 2018:4795604.
Yan, L., Zhu, K., Zhang, Y., Zhang, C., and Zheng, X. (2020). Effect of absorbent foam filling on mechanical behaviors of 3d-printed honeycombs. Polymers 12:2059. doi: 10.3390/polym12092059
Yang, Y., Chen, Z., Song, X., Zhang, Z., Zhang, J., Shung, K. K., et al. (2017). Biomimetic anisotropic reinforcement architectures by electrically assisted nanocomposite 3D printing. Adv. Mater. 29:1605750. doi: 10.1002/adma.201605750
Yang, Y., Li, X., Chu, M., Sun, H., Jin, J., Yu, K., et al. (2019). Electrically assisted 3D printing of nacre-inspired structures with self-sensing capability. Sci. Adv. 5:eaau9490. doi: 10.1126/sciadv.aau9490
Yang, Y., Li, X., Zheng, X., Chen, Z., Zhou, Q., and Chen, Y. (2018a). 3D−printed biomimetic super−hydrophobic structure for microdroplet manipulation and oil/water separation. Adv. Mater. 30:1704912. doi: 10.1002/adma.201704912
Yang, Y., Song, X., Li, X., Chen, Z., Zhou, C., Zhou, Q., et al. (2018b). Recent progress in biomimetic additive manufacturing technology: from materials to functional structures. Adv. Mater. 30:1706539. doi: 10.1002/adma.201706539
Zaheri, A., Fenner, J. S., Russell, B. P., Restrepo, D., Daly, M., Wang, D., et al. (2018). Revealing the mechanics of helicoidal composites through additive manufacturing and beetle developmental stage analysis. Adv. Funct. Mater. 28:1803073. doi: 10.1002/adfm.201803073
Zhao, Y., Sakai, F., Su, L., Liu, Y., Wei, K., Chen, G., et al. (2013). Progressive macromolecular self−assembly: from biomimetic chemistry to bio−inspired materials. Adv. Mater. 25, 5215–5256. doi: 10.1002/adma.201302215
Keywords: mechanical properties, bioinspired design, additive manufacturing, 3D printing, biomimetic
Citation: Yan X, Bethers B, Chen H, Xiao S, Lin S, Tran B, Jiang L and Yang Y (2021) Recent Advancements in Biomimetic 3D Printing Materials With Enhanced Mechanical Properties. Front. Mater. 8:518886. doi: 10.3389/fmats.2021.518886
Received: 29 December 2019; Accepted: 15 April 2021;
Published: 28 May 2021.
Edited by:
Alfonso Maffezzoli, University of Salento, ItalyReviewed by:
Leire Ruiz Rubio, University of the Basque Country, SpainLarissa Gorbatikh, KU Leuven, Belgium
Copyright © 2021 Yan, Bethers, Chen, Xiao, Lin, Tran, Jiang and Yang. This is an open-access article distributed under the terms of the Creative Commons Attribution License (CC BY). The use, distribution or reproduction in other forums is permitted, provided the original author(s) and the copyright owner(s) are credited and that the original publication in this journal is cited, in accordance with accepted academic practice. No use, distribution or reproduction is permitted which does not comply with these terms.
*Correspondence: Laiming Jiang, bGFpbWluZ19qaWFuZ0Bmb3htYWlsLmNvbQ==; Yang Yang, eXlhbmcxMEBzZHN1LmVkdQ==
†These authors have contributed equally to this work