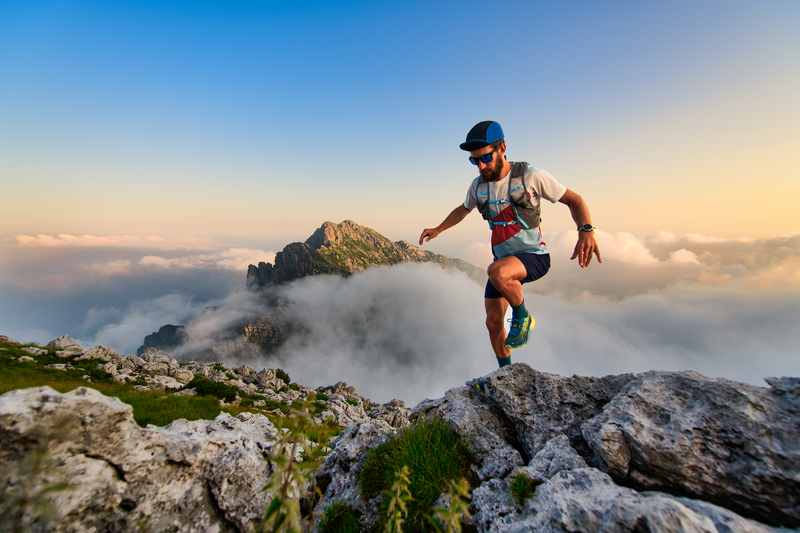
95% of researchers rate our articles as excellent or good
Learn more about the work of our research integrity team to safeguard the quality of each article we publish.
Find out more
ORIGINAL RESEARCH article
Front. Mater. , 10 December 2020
Sec. Structural Materials
Volume 7 - 2020 | https://doi.org/10.3389/fmats.2020.572955
This article is part of the Research Topic Women in Science: Materials View all 34 articles
The concept of sustainability in the road construction sector is a complex issue because of the various steps that contribute to the production and release of greenhouse gas (GHG) emissions. Addressing this issue, the European Commission has put various policy initiatives in place to encourage the construction industry to adopt circular economy (CE) and industrial symbiosis (IS) principles e.g., the use of recycled materials. Cooperativa Trasporti Imola (CTI), a company located in the Emilia-Romagna region (Italy), has been chosen for the current case study to examine practices, management, and the industrial symbiosis network among various companies in the road construction and rehabilitation sector. In this regard, the use of steel slags, obtained by an electric arc furnace (EAF), and reclaimed asphalt pavement (RAP), obtained by the deconstruction and milling of old asphalt pavement have been investigated. Two mixtures of recycled hot Mix Asphalt (HMA) i) were prepared incorporating different recycled material percentages for the wearing and binder course, respectively, ii) were characterized in terms of size distribution, strength modulus and volumetric properties, iii) and finally were compared to the performances of two mixtures entirely designed by virgin materials for the wearing and binder course, respectively. Therefore, the Life Cycle Assessment (LCA) tool was chosen to evaluate the environmental impacts that affect the designed road life cycle. The results show that recycling RAP and EAF slags in a CTI batch plant provides benefits by reducing the consumption of virgin bitumen and aggregates and by reducing CO2eq emissions. Finally, practical implications on the use of recycled materials in new asphalt mixtures from a life cycle and industrial symbiosis perspective are provided.
Greenhouse gas (GHG) emissions in infrastructure projects are a key indicator when sustainability is being assessed (Gasparatos et al., 2008; Fernández-Sánchez and Rodríguez-López, 2010). The road sector, due to its characteristics (high energy consumption; use of resources, raw material, and surface; generation of high volumes of waste; quantity of linked transports and long service life) is one of the main sectors that contributes to global warming (GW) (Cass and Mukherjee, 2011). Significant GHG emissions result from many stages of the road life cycle. Santero and Horvath (2009) stated that GHG emissions could range from negligibly small values to 60,000 tons of equivalent carbon dioxide (CO2eq) per lane-kilometer over a service life of 50 years. Similarly, the emission factor per meter per year associated with the construction of road infrastructure has been estimated at 14.7 kg of CO2eq (Hill et al., 2012). In this sense, regarding road construction and maintenance, several steps contribute to the production and release of GHG emissions, i.e., site clearing, preparation of the sub-grade, production of construction materials (i.e., granular sub-base, base course, surfacing), site delivery, construction works, ongoing supervision, and maintenance activities. As European road infrastructure includes a growing network, with 4.8 million km at the end of 2013 (European Union Road Federation, 2017) and 5.5 million km at the end of 2016 (European Union Road Federation, 2019), this sector has broad margins for environmental improvement (Santero et al., 2011a; Santero et al., 2011b; JRC, 2016). For these reasons, this study is of particular interest.
Road construction is one of three main drivers of resource use in the European Union (Steger and Bleischwitz, 2011). Road construction not only requires large quantities of materials, but also their maintenance is highly material intensive. Construction works and regular maintenance of roads require materials that are produced through highly carbon-intensive and energy-demanding processes (Santos et al., 2015; Jiang and Wu, 2019). Previous studies have suggested that the life-cycle of GHG emissions associated with building roads can account for 10–20% of the emissions associated with the lifetime usage of the road by vehicles (Chester and Horvath, 2009; Hanson and Noland, 2015; Noland and Hanson, 2015). Previous research has also shown that the bulk of the emissions related to road construction and maintenance activities is often associated with the upstream emissions embodied in the materials used (Hanson and Noland, 2015; Huang et al., 2015; Noland and Hanson, 2015). Hence, the choice of materials impacts local pollution and environmental degradation. These materials primarily include asphalt, concrete, and steel (Hanson and Noland, 2015). Therefore, also considering the increase of landfilling restrictions on CDW, the use of alternative materials such as industrial by-products has gained greater significance and attention from academia and industrial sectors (Jamshidi et al., 2017).
The European Commission (EC) has put various policy initiatives in place to encourage the construction industry toward circular economy (CE) principles. The overall idea is to reconsider the whole life cycle of resources, to make the European Union (EU) a “circular economy” based on recycling, and the use of waste as a resource (EC, 2011).
The use of alternative materials for the construction and rehabilitation of roads would therefore be a strategy to be boosted, establishing regional industrial symbiosis (IS) agreements which can support companies to gain competitiveness and reduce the environmental impact associated to their day to day business activities (Martin-Portugues Montoliu et al., 2019). For that reason, the EC has recently launched an industry-led IS reporting and certification system (EC, 2020). In this sense, symbiotic activities can be applied at different levels. According to Roberts (Roberts, 2004), they can involve a single firm or organization (micro level); companies co-located in the same area (meso level); and finally the entire regional or national production system (macro level). The greatest benefits are achieved at the meso level, where the clustering of complementary companies provides a complexity of functions (Roberts, 2004; Taddeo, 2016).
Roads are built in layers and three main types of road pavements can be identified: flexible, semi-rigid, and rigid pavements. In Europe, the main pavement type is flexible (asphalt) (Sherwood, 2001; Garbarino et al., 2016). As shown in Figure 1, the main road layers for flexible pavement are:
• surface, binder, and base courses, which consist of bituminous mixtures;
• road base and sub-base courses, which consist of cement bound or unbound aggregates.
FIGURE 1. Flexible pavement layer system (Garbarino et al., 2016). The sizes of each course represented in the figure do not necessarily correspond to the actual ones.
Asphalt mixtures are typically composed of approximately 95% of mineral aggregates mixed with about 5% paving bitumen, with bitumen functioning as the glue that binds the mineral aggregates in a cohesive mix (EAPA, 2011). In general, three types of asphalt mixtures can be used: hot mix asphalt (HMA), warm mix asphalt (WMA), and cold mix asphalt (CMA).
Some aggregates can, usefully, be created by recycling processes.
In this study, the use of steel slags obtained by an electric arc furnace (EAF), and reclaimed asphalt pavement (RAP) obtained by the deconstruction and milling of old asphalt pavement have been investigated. The HMA technology was used in the production process.
While recycling HMA results in a reusable mixture of aggregates and aged asphalt binders known as RAP (Al-Qadi et al., 2007; Noferini et al., 2018), recycling steel slags produces artificial aggregates, containing 90% iron oxide and smaller quantities of other oxides (calcium, magnesium, silicon, etc.), derived from additives used in steel production. Moreover, on the basis of production technology, steel slags can be classified as basic oxygen furnace (BOF), electric arc furnace (EAF), and ladle refining (LF) slags (Meng and Liu, 2000; Gu et al., 2018).
As is widely known, steel slags, produced during the separation of molten steel from impurities in a steel-making furnace, are one of the most common industrial wastes and they can be used for several applications. Thanks to their high hardness and cementing properties, they are commonly used in the road sector (Rashad, 2019). In steel plants, high-grade steel is melted, obtaining a sinking heavy liquid metal fraction, which agglomerates into a metal phase, separating metals from some light fractions such as chromium by reducing agents and liberating chromium from its compounds. Slag composition can be optimized in relation with application requirements and then it can be cast. In some processes (i.e., RecArc project, http://www.recarc.bam.de) the high-grade steel slags can be converted into a chromium-rich metal phase, which can be used as a raw material in high-grade steel production.
As far as the recycling of RAP and of EAF steel slags are concerned, several studies have shown that the use of these materials is common in pavement construction because of their technical performances and economic value. Miliutenko et al. (2013) have shown that HMA mixes with RAP content have the same technical characteristics (stiffness, fatigue, and deformation resistance) as virgin ones. Thanks to the incorporation of RAP in new asphalt mixtures, the need of neat bitumen is reduced, making RAP recycling economically attractive (Noferini et al., 2018; Pantini et al., 2018).
Similarly, other studies have demonstrated that steel slags with proper pre-processing and sufficient in-place quality control procedures can perform credibly well as asphalt aggregates (Del Fabbro et al., 2001; Ahmedzade and Sengoz, 2009; Gu et al., 2018). In particular, EAF slags have been frequently used as pavement aggregates due to their excellent mechanical properties, which make them suitable for asphalt layers with any kind of traffic load (Santos et al., 2015).
Moreover, Pasetto and Baldo (2017) studied the stiffness and the fatigue performance of five different base-binder bituminous mixtures, made with RAP and EAF steel slag, up to 70% by weight of the aggregate. They reported that the resulting mixes with RAP and EAF slag were characterized by improved stiffness and fatigue performance compared to the control asphalt concrete, made exclusively with natural aggregate.
The Life Cycle Assessment (LCA) is a considerable method to evaluate the environmental impacts of a system, a product, or a process. All the inputs (such as energy and resources) are identified, with the aim of quantifying the relevant emissions, the consumed resources, and the related environmental impacts. Considering a product, the impacts do not only arise during the manufacturing stage, but along its entire life cycle, including the extraction and transportation of raw materials, use and maintenance, possible reuse, and end of life. Therefore, the approach encompasses the whole life cycle of a product, “from cradle to grave,” as the first definition stated (SETAC, 1993): from “cradle,” where raw materials are extracted, put into production, and used, to “grave,” i.e., waste disposal, with the aim to provide a comprehensive picture of the environmental impacts of the system.
According to a circularity perspective, a new philosophy, referred to as “from cradle to cradle”, is taking hold: at their end of life, materials are not considered as waste to be discarded, but as secondary raw material, thanks to an appropriate recycling process. In this way, a cradle-to-cradle closed loop is outlined.
According to the ISO14040 standard, the four steps to perform a Life Cycle Assessment (LCA) are: the definition of the goal and scope of the analysis, the inventory analysis, the impact assessment, and finally, the interpretation of the results (Figure 2).
FIGURE 2. LCA phases (EC et al., 2010).
The context of the study and its purpose are set. The goal of the LCA states the intended application and the reasons for carrying out the study, the intended audience, and whether the results are to be used for internal purpose or for disclosure to the public. The scope includes the following items: functional unit, system boundary, allocation procedure, data requirements, impact assessment method, assumption, and data quality. In particular, the functional unit, that defines the quantification of the identified function of the product, has the primary purpose to provide a reference to which the inputs and outputs are related, ensuring the comparability of the LCA results. The system boundary defines the unit processes to be included in the system. Criterions for the choice of the system boundaries are physical (description of the productive cycle), geographical (reference area), and temporal (reference period).
It lists all the inputs (materials and energy) and outputs (products, co-products, and emissions) to be used to compare standards and processes. Inventory analysis involves data collection and calculation procedures, aiming at quantifying the relevant inputs and outputs of a product system. The life cycle inventory uses both primary and specific as well as literature and secondary data from international databases.
The life cycle impact assessment (LCIA) includes the following mandatory elements: the selection of impact categories and characterization models; the assignment of LCI results to the selected impact categories (classification); and the calculation of category indicator results (characterization).
Finally, the life cycle interpretation aims at the identification of substantial issues, based on the results of the previous steps. The evaluation includes considerations about the completeness and the consistency of the study, conclusions, limitations, and recommendations.
In Figure 2, the two-way arrows highlight the iterative approach of an LCA. During the analysis, the results and the assumptions in subsequent stages might lead to the revision of what has been done in previous stages, in a process of continuous improvement. Information which was not available during the compilation of the previous phases can be added afterward.
Due to the high amount of GHG emissions generated during road construction, rehabilitation, and operation, the evaluation and reduction of the environmental impact related to the road sector have become an international challenge (Espinoza et al., 2019). In this sense, a systematic approach has emerged to assess the environmental impact of pavements. LCA is considered a relevant methodology to evaluate the environmental impacts that affect the road life-cycle (Espinoza et al., 2019) and the International Organization for Standardization (ISO) has established the principles, requirements, and guidelines to regulate the LCA analysis (ISO 14040, ISO 14044, ISO 14020, ISO 14024, and ISO 14025). Moreover, the Joint Research Centre (JRC) in 2016 proposed an LCA as an assessment methodology of road environmental performance, with reference to ISO 14067 or equivalents and ISO 14040/14044. Finally, according to Espinoza et al., 2019, the use of an LCA for evaluating the environmental performance of the construction of road projects allows construction companies to obtain information that can be used to predict the performance of their projects and to evaluate compliance with environmental requirements. Similarly, it allows the selection of optimal materials and construction processes, reducing the GHG emissions and permitting a more sustainable approach.
Therefore, LCA analysis performed by Espinoza et al., 2019 highlighted that HMA production generates the greatest environmental impact, considering the extraction of raw material and the construction of the HMA layers. Previous research has shown that HMA emits up to 18–22 kgCO2/t (Agentschap Wegen en Verkeer, 2012; JRC, 2016) and a recent LCA literature review for roads, carried out by JRC (2016), shows that the second largest source of environmental impact after the use phase is the production of construction materials. In addition, in low traffic roads, this can in fact be the most significant source of environmental impact (JRC, 2016). Moreover, the durability of road materials is a key factor that will influence the requirement for maintenance. The impacts of maintenance activities themselves are dominated by impacts from material production and transportation. Consequently, special attention to HMA production and construction materials is required in order to minimize GHG emissions. For these reasons, several studies have pointed out the environmental benefits of using recycled materials, such as RAP and EAF steel slags. Hasan et al. (2020) argued that RAP obtained after milling and screening existing asphalt pavements is a viable alternative to mitigate the high GHG burdens of bitumen and aggregates (Praticò et al., 2015; Guo et al., 2018) and transport agencies (AASHTO, 2012; Hasan et al., 2020). In particular, the use of steel slags in asphalt mixtures saves natural resources, by reducing the consumption of natural and non-renewable aggregates and the quantity of slag deposited on landfill sites (Ferreira et al., 2016) and the reduction of the landfill space requirements associated with the need to landfill industrial wastes and by-products (Carpenter et al., 2007; Huang et al., 2009; Miliutenko, et al., 2013, Mladenovic et al., 2015). The EAF is a less energy intensive process where electricity is used to melt steel into the end product. This could be a promising alternative that may have close to zero CO2 emissions, theoretically (Ferreira et al., 2016; Morfeldt et al., 2015). Finally, Giani et al. (2015) explored the replacement of virgin asphalt by 10% RAP in a HMA surface course and by 20% RAP in a HMA binder course of a 1 km asphalt pavement section in Italy. They found that the HMA RAP alternative exhibited 688 tons of CO2eq (6.8%) GHG emissions reductions, considering that the environmental burdens of asphalt significantly depend on the bitumen content (Häkkinen and Mäkelä, 1996).
The “Cooperativa trasporti Imola Scrl” (CTI) company has four plants for the production of asphalt mixes, three batch plants and one drum plant. In both the typologies, the mineral aggregates are dried and heated in a rotating drum. Nowadays, the predominant plant type in the U.S. and New Zealand is the drum-mix plant, while batch plants prevail in Europe, South Africa, and Australia (EAPA, 2018). While in batch plants aggregates are stored in hot bins to mix them with bitumen in discrete batches, in drum plants the mixing of aggregates with bitumen takes place in the same drum. After those processes, the mixtures are stored in silos or loaded into trucks for delivery. Afterwards, aggregates, temporarily stored in a silo, are transported by mechanical shovels, and loaded on hoppers for pre-dosage. The CTI plants have seven hoppers, five for aggregates and two for milled materials, with the possibility of introducing RAP. In the drum plant, the drum acts both as a dryer and a mixer, whereas in the batch plant the mixing of aggregates with bitumen takes place in different machines. The bitumen, heated to 130–150°C by an oil-fired oleothermal boiler, is kept at a constant temperature in the storage tanks. Considering hot in plant recycling, while the most conventional drum plants can accommodate up to 50% RAP, and the percentage of reusable RAP in batch plants ranges from 10 to 30% (Kandhal and Mallick, 1997; Noferini et al., 2018), nowadays multiple readily available for production technologies can accommodate up to 100% of recycled hot mix asphalt (Zaumanis et al., 2014; Noferini et al., 2018). The CTI batch plants might accommodate up to 45–50% RAP, while the percentage of reusable RAP in drum plants is approximately 50%. Hence, nowadays there is no technical limit on RAP content in new asphalt mixtures, as long as an adequate performance is achieved. However, it is a common practice to set a maximum value, to guarantee the durability of asphalt mixes in the long term (JRC, 2016), due to the possible compromising effect of the aged bitumen in RAP on the final mix. Moreover, the defined optimum content of RAP in asphalt mixtures varies widely from country to country, from 7 to 50% (up to 66%) by mass (Kalman et al., 2013; Garbarino et al., 2016). On average, western European countries have 40% RAP content in HMA and WMA mixtures, while Eastern European countries have 6% (BIOIS and EC, 2011; Blankendaal et al., 2014; Garbarino et al., 2016).
Moreover, according to the European Commission, steel slags can be used in road construction, meeting the requirements of European and national legislations and standards, although a specific recycling target is not set (JRC, 2016).
In Italy, the steel slags resulting from steelmaking are considered by-products, whereas RAP, as a result of the milling operations of existing road pavements at their end of life stage, is not considered to be waste, as long it is re-used within the domain of the asphalt sector (Italian M.D. 69/2018). Due to the fact that in Italy the use of steel slags and RAP for road construction is allowed, in this study, the content of RAP and EAF slags in the mixtures was designed to allow for the production of the mixtures in the CTI batch plant and to achieve acceptable values of physical and mechanical properties, in compliance with national legislations and technical standards.
This study aims at testing the use of EAF steel slags and RAP in two mixtures, for wearing and binder courses, respectively. The physical and mechanical properties and the environmental performances have been evaluated. The objectives of the research study are summarized below:
• define a standard characterization of mixtures in order to evaluate the physical and mechanical performances related to the use of virgin and recycled materials;
• assess the environmental impacts associated with the mixtures and model a best-case scenario for the CTI batch plant with the maximum percentages of steel slags and RAP;
• identify practical implications of the use of recycled materials in new asphalt mixtures, from a life cycle and industrial symbiosis perspective.
The research study is divided into two phases: in the first phase, the effects of recycled materials on asphalt mixture proprieties are investigated. Two specific types of asphalt mixtures are produced with different compositions:
(1) 35% RAP and 16% steel slags for the wearing course, by weight;
(2) 40% RAP and 15% steel slags for the binder course, by weight.
Asphalt materials are characterized in terms of size distribution, strength modulus (indirect tensile strength), and volumetric properties (air voids content).
The second phase aims at evaluating environmental impacts by applying LCA methodology to the geographical context of the CTI company. The novelty of this study is the integration of the technical analysis of material characterization, assessed by laboratory experiments, with the analysis of the environmental impacts.
Four mixtures were analyzed:
• A control mixture for the wearing course (MixW0)
• An experimental mixture for the wearing course (MixW1)
• A control mixture for the binder course (MixB0)
• An experimental mixture for the binder course (MixB1)
A description of the four mixtures can be found in Table 1.
The design of the aggregate distribution was based on gradation limits specified in the UNI 13108 Italian technical specification for bituminous layers, as shown in Figures 3, 4, with cumulative percentage passing on the y axis and logarithmic sieve size on the x axis. On the graphs, the sieve size scale (x axis) is logarithmic.
The experimental program can be divided into three different phases. In order to evaluate the physical and mechanical performances of the designed mixtures, MixW1 and MixB1 were characterized in terms of particle size distribution (1), volumetric properties (2), and strength modulus (3) according to the standard UNI EN 933-1 (2012), UNI EN 12697-12 (2018), UNI EN 12697-23 (2003), and UNI EN 12697-26 (2012). Asphalt mixtures were manufactured in a laboratory with design neat bitumen content of 6% for the wearing course and 5% for the binder course (these percentages include aged bitumen contained in RAP, 3.85% for the wearing course and 3.30% for the binder course, respectively). A neat binder was incorporated into the mixes, taking into account the presence of the aged binder in the RAP fractions. At the same time, the inclusion of recycled materials in the mixtures requires the addition of rejuvenating agents (ACF) to improve the adhesion properties, thermal susceptibility, viscosity, and workability of the mixes. The ACF is incorporated in the commercial bitumen. Both aggregates and bitumen can directly replace their equivalent virgin products in the new mixtures (MixW1 and MixB1) at a ratio of 1:1. Aggregates were heated at 160°C. The physical and mechanical characterizations were then carried out. Asphalt mixes were tested for particle size distribution (EN 933 – 1), air void content (EN 12697-8), and indirect tensile strength (EN 12697 – 23).
The sieve analysis was carried out in a laboratory to define the particle size distribution of MixW1 and MixB1. According to the EN 933-1 standard, a representative weighed sample for each mixture was separated on sieves of different sizes (Series 2). To find the percentage of the aggregate passing through each sieve, Eq. 1 was used:
where: WSieve is the mass of the aggregate in the sieve; WTotal is the total mass of the aggregate.
In order to find the cumulative percentage of the aggregate retained in each sieve, Eq. 2 was used. The total amount of the aggregate retained in each sieve and the amount in the previous sieves were added up. Then, the cumulative percentage passing of the aggregate was found by subtracting the percentage retained from 100%.
The % cumulative retained
where:
To solve Eq. 3, Eq. 4 was provided:
Once the mix design for MixW1 and MixB1 was defined, the following step in the research program considered their physical analysis. The compactability and workability properties of the HMAs were evaluated against gyratory compactor samples (EN 12697-31). For both mixtures, three specimens per MixW1 were compacted up to 180 times more than the gyratory compactor, and three specimens per MixB1 were compacted up to 210 times more than the gyratory compactor. The air voids content (v) of each specimen was evaluated according to the EN 12697-8 standard.
Finally, for each mixture, according to the EN 12697-23 standard, the indirect tensile strength (ITS) was performed at 25°C.
The present study has assessed the impacts arising from the hot-mix batch plant by applying an LCA methodology to the geographical context of the CTI plant, located in the Emilia-Romagna region. As previously described, an LCA study consists of four stages: 1) goal and scope definition, 2) inventory analysis, 3) impact assessment, and 4) results and interpretation.
Quantitative and comparative life cycle assessment results on road construction materials are essential first steps toward making informed decisions and toward more sustainable practices in road construction (Chowdhury et al., 2010). The present LCA study aims at evaluating the potential environmental impacts related to asphalt mixtures: 1) MixW0 compared to MixW1 and 2) MixB0 compared to MixB1. The final aim is to provide recommendations to the CTI for the improvement of technologies and regulations, based on environmental considerations. The functional unit (FU) of LCA is 1 km of secondary suburban road (with a width of 10.5 m, and a thickness of 4 cm for the wearing course, and 6 cm for the binder course). The system boundary includes all the treatment processes, starting from virgin material mining, and secondary and virgin materials entering the CTI batch plant (diesel, electricity), until when they leave the plant as an (solid, liquid, or gaseous) emission or as a new material. The final disposal or recycling processes are out of the boundary. Hence, this LCA is a cradle-to-gate analysis. As depicted in Figure 5, the system and processes involved in the present study are:
• - The raw material transportation from the mining site/quarry to the CTI plant;
• - The RAP transportation from road worksites to the CTI plant;
• - The RAP pre-processing, which includes crushing and screening;
• - The avoided production and transportation of natural aggregates (replaced by recycled aggregates), including extraction, processing, and transportation to the CTI batch plant;
• - The avoided production and transportation of virgin bitumen (replaced by recycled bitumen).
FIGURE 5. Diagram flow of recycling reclaimed asphalt pavement (RAP) and electric arc furnace (EAF) in the CTI batch plant.
The geographical scope is local. The study focuses on the conditions and CTI technologies used in 2018. The potential environmental impacts were evaluated using the software SimaPro®. This analytical tool works in accordance with the ISO 14040 standard (ISO, 2006a). The impact assessment baseline, performed by the Institute of Environmental Sciences of the Leiden University (CML) in version 3.05, was selected as a method for the environmental impact assessment, using the LCI “Ecoinvent 3.5” and “Europe & Denmark input output” databases. The following impact categories were evaluated: abiotic depletion, acidification, eutrophication, global warming potential, ozone layer depletion, and photochemical oxidation.
Data regarding the core processes, i.e., transportation, hot recycling, and energy consumption, are primary data. For analyzing the CTI HMA batch plant, data were collected directly from the CTI company. Data related to other foreground processes, i.e., bitumen production, extraction of natural mineral resources, and pre-processes of waste asphalt, were instead taken from the LCA software SimaPro databases (Ecoinvent and Europe & Denmark databases). Therefore, the avoided impacts, due to the avoided consumption of natural virgin aggregates because of the EAF steel slags and RAP addition into hot mixes, are modelled using secondary data on quarry activities in Europe.
Inventory data about the transportation of the raw materials, asphalt waste, and bitumen are modelled using the primary data on CTI transports, as shown in Table 2. Table 3 shows the inventory data on energy consumption in the CTI batch plant.
TABLE 2. Inventory data about the transportation of the asphalt waste, the by-products, and the primary materials to the plant.
In the LCIA, the CML impact assessment baseline calculation method was adopted. The consumption of materials and energy as well as the emissions to air, water, and soil were gathered according to the effects they can have on the environment. According to ISO 14044 (2006b), the LCIA consists of classifications into impact categories, normalization, and the weighting of impacts. In this standard, a distinction between mandatory elements (classification and characterization) and optional elements (normalization, grouping, ranking, and weighting) was pointed out. In the current LCA study, classification and characterization were performed to assess the environmental impacts of MixW1 compared to MixW0 and of MixB1 compared to MixB0. No optional elements were evaluated.
Therefore, this methodology aims to assess the environmental impacts of the processes identified in the inventory analysis. Hence, all substances were measured and assigned to an impact category. The results are represented by single midpoints.
In order to evaluate the physical and mechanical performances of the mixtures incorporating different recycled aggregate percentages for the wearing and binder courses, MixW1 and MixB1 were characterized in terms of air void content (v), indirect tensile strength (ITS), indirect tensile stiffness modulus (ITSM), and indirect tensile strength ratio (ITSR).
The determination of the air void content of MixW1 and MixB1 can be found in the Supplementary Material as well as the results of the determination of ITS, ITSR, and ITSM of MixW1 and of MixB1, respectively. According to the UNI EN 12 697 – 12 standard, the ITSR value represents the ratio of the indirect tensile strength of wet (water conditioned) specimens to that of dry specimens expressed as percentages, calculated by using the following equation (Eq. 5):
Where:
The LCA was chosen to evaluate the environmental impacts that affect the designed road life cycle (production and treatment processes and transportation of the involved materials). The overall environmental impacts related to the production of asphalt mixtures MixW1 and MixB1 in the CTI batch plant are shown in Table 4. The analysis was supported by the LCA in compliance with the ISO 14040 standard and the ISO 14044 standard.
To discuss the results of the standard characterization of the designed mixtures, a comparison of the performances of the designed mixtures and control mixtures was first performed.
Table 5, 6 show the results for the four mixtures, in terms of average indirect tensile strength (ITS) and average air void percentages.
The mechanical analysis was supported by the ITS test in compliance with the EN 12697-23 standard. For each mixture, three samples were prepared with a gyratory compactor (180 and 210 times) and then conditioned at 25°C for 4 h before testing. According to the scientific literature, an ITS test is generally used to assess the level of tenacity of the aggregate-filler-bitumen bond (Sangiorgi et al., 2019) and the ITS value strongly depends on the medium-high amount of aggregates, bitumen, and recycled materials. From the analysis of data, there was a difference in terms of indirect tensile resistance between the two experimental mixtures (MixW1 and MixB1) compared to the control ones (MixW0 and MixB0). It could be argued that the results indicate a hardening of the composite blend caused by the presence of the aged bitumen. Therefore, the two mixtures show different air void contents.
To note, the average value of ITS recorded for both experimental mixtures was considerably higher than the limit suggested by the Italian technical specifications (ANAS, 2019), which ranges between 0.72 and 1.60 MPa per wearing course, and between 0.72 and 1.40 MPa per binder course.
Similarly, if the Italian technical specification is taken into account, the air void content (v) was lower than the suggested one, which ranges between 3 and 8%.
The standard characterization of the mixtures evaluated the hardening effect of the old bitumen on the content blend. According to Noferini et al. (2018), the hardening effect becomes relevant when the RAP binder content is above 20% by weight of the mixture. In particular, the new bituminous mixtures per binder and wearing course (MixB1 and MixW1) were more rigid than the traditional ones (MixB0 and MixW0), but they were not excessively thickened because the final void value was less than 2%. Hence, the final void content of the two new mixtures was optimal.
Moreover, as the stiffness and the fatigue performance were not tested, further research might investigate these aspects.
Secondly, a comparison of LCA results between the two mixtures for the wearing course (MixW0 and MixW1) and the two mixtures for the binder course (MixB0 and MixB1) was performed. Hence, Figure 6 and Table 7 show the results of the contribution analysis related to the recycling of RAP and EAF slags in the CTI batch plant. Interestingly, significant differences were found between MixW0 and MixW1, and between MixB0 and MixB1. LCA allows the authors to evaluate the environmental benefits related to the use of recycled aggregates. Due to the reduction of emissions and natural resources used, MixW1 and MixB1 provide environmental benefits in all impact categories (abiotic depletion, abiotic depletion fossil fuels, global warming potential, ozone layer depletion, human toxicity, terrestrial ecotoxicity, photochemical oxidation, acidification, and eutrophication). The avoided impacts associated with the use of recycled material and with the reduction in the consumption of bitumen and aggregates overcome the impacts related to the waste transportation and the pre-treatment processes, resulting in a total reduction in environmental impact. Hence, it can be argued that recycling RAP and EAF steel slags in the CTI batch plant provides environmental benefits, besides reducing the consumption of virgin bitumen and natural aggregates.
FIGURE 6. Contribution analysis related to recycling of RAP and EAF slags in CTI batch plant for wearing course (above) and for binder course (below).
These results are supported by the ones obtained in a preliminary and more general study, previously undertaken (Degli Esposti et al., 2020), showing that the experimental mixtures have fewer environmental impacts than the control ones. According to the LCA results, a reduction in all impact categories occurred, and mainly in human toxicity (−30.5%) and eutrophication (−24%), related to the intensive energy consumption and the utilization of non-renewable sources, during both the extraction and transportation phases. In particular, a robust reduction in CO2eq emissions was demonstrated by the better performance of the category global warming potential (−21%), as it was estimated at 46 tons of CO2eq for the experimental mixtures (MixW1 and MixB1) and at 58 tons of CO2eq for the control mixtures (Mix W0 and MixB0). To summarize, the use of RAP and EAF steel slags in 1 km of suburban road allows the CTI to reduce the content of virgin bitumen by weight of the total mixture by 2.15% (by total weight) for the wearing course and by 1.70% (by total weight) for the binder course. Moreover, the use of recycled materials in 1 km of suburban road allows the company to save 438.0 tons of natural aggregates and 18.2 tons of virgin bitumen for the wearing course, 826.2 tons of natural aggregates and 22.1 tons of virgin bitumen for the binder course, as shown in Figure 7.
FIGURE 7. Diagram flow of recycling RAP and EAF in the CTI batch plant for hot-mix asphalt, with the inclusion of the avoided products for wearing course (MixW0 and MixW1) and binder course (MixB0 and MixB1).
The inert nature of RAP, and the excellent mechanical properties of the EAF slags make them two potentially useful materials in a wide variety of applications, including re-use or recycling in new asphalt pavements. This case study demonstrates the high potential for recycling RAP and EAF steel slags in the road construction sector, as a secondary raw material and a by-product, respectively.
As a result of testing the use of EAF steel slags and RAP in new bituminous mixtures, the physical and mechanical properties as well as the environmental performances of the two mixtures have been evaluated for wearing and binder courses, respectively. In order to maximize the environmental sustainability of the road pavement, the use of RAP and EAF steel slags can be recommended.
Moreover, the authors believe that LCA results and indicators are appropriate tools to compare and communicate the environmental performances of different asphalt mixtures in road construction.
By reducing the global environmental impact and recycling by-products, the CTI and the co-located companies are a real case study of industrial symbiosis at the meso-level.
The authors believe that the development of industrial symbiosis projects provides the opportunity to promote waste reduction, reuse, and recycling, while reducing the environmental impacts, as well as increasing companies’ competitiveness, in particular in countries like Italy, where there are already several large industrial clusters. Moreover, information sharing among stakeholders would facilitate the development of industrial symbiosis networks.
Future research efforts could focus on investigating other recycled materials, for the same applications as virgin ones, with the purpose of reaching the same quality level and performances. In this issue, no economic evaluation was carried out. As a future research direction, the economic sustainability will be evaluated.
The original contributions presented in the study are included in the article/Supplementary Material, further inquiries can be directed to the corresponding authors.
AB and AE designed the study. AE collected information and materials from the company. AB and AE conceived and planned the experiments for the characterization of asphalt mixtures. AE carried out the experiment. All the authors contributed to the interpretation of the results. AE and CM designed the LCA model and analysed the data. All the authors contributed to the interpretation of the LCA results. AB took the lead in writing the manuscript. All authors provided critical feedback and helped shape the research, analysis and manuscript.
The authors declare that the research was conducted in the absence of any commercial or financial relationships that could be construed as a potential conflict of interest.
The authors gratefully acknowledge contributions from the CTI company (Imola, Italy).
The Supplementary Material for this article can be found online at: https://www.frontiersin.org/articles/10.3389/fmats.2020.572955/full#supplementary-material.
AASHTO (2012). Standard specification for use of reclaimed asphalt shingles (RAS) as an additive in hot mix asphalt (HMA). Washington, DC: American Association of State Highway Transportation Officials. https://www.sciencedirect.com/science/article/pii/S0959652620305783#bib.
Agentschap Wegen en Verkeer (2012). Carbon free-ways. http://www.wegenenverkeer.be/parallelle-sessies/sessies-pm/carbon-free-ways/item/carbon-free-ways.html
Ahmedzade, P., and Sengoz, B. (2009). Evaluation of steel slag coarse aggregate in hot mix asphalt concrete. J. Hazard Mater.,165, 1–3, 300–305. doi:10.1016/j.jhazmat.2008.09.105
Al-Qadi, I. L., Elseifi, M., and Carpenter, S. H. (2007). Report No. FHWA-ICT-07-001. Reclaimed asphalt pavement–a literature review. Rantoul, IL: Illinois Center for Transportation. http://hdl.handle.net/2142/46007
ANAS (2019). I quaderni tecnici per la salvaguardia delle infrastrutture, Vol. V. https://www.stradeanas.it/sites/default/files/pdf/1.3.3/Quaderni_tecnici_volume_5.PDF.
BIOIS and EC (2011). Service contract on management of construction and demolition waste – SR1. Final report task 2. http://ec.europa.eu/environment/waste/pdf/2011_CDW_Report.pdf.
Blankendaal, T., Schuur, P., and Voordijk, H. (2014). Reducing the environmental impact of concrete and asphalt: a scenario approach. J. Clean. Prod. 66, 27–36. doi:10.1016/j.jclepro.2013.10.012
Carpenter, A. C., Gardner, K. H., Fopiano, J., Benson, C. H., and Edil, T. B. (2007). Life cycle based risk assessment of recycled materials in roadway construction. Waste Manag. 27 (10), 1458–1464. doi:10.1016/j.wasman.2007.03.007
Cass, D., and Mukherjee, A. (2011). Calculation of greenhouse gas emissions for highway construction operations using a hybrid life cycle assessment approach: a case study for pavement operations. J. Construct. Eng. Manag. 137 (11), 1015–1025. doi:10.1061/(ASCE)CO.1943-7862.0000349
Chester, M. V., and Horvath, A., (2009). Environmental assessment of passenger transportation should include infrastructure and supply chains. Environ. Res. Lett. 4 (2). 024008. doi:10.1088/1748-9326/4/2/024008
Chowdhury, R. D. A., and Fry, T. (2010). A life cycle based environmental impacts assessment of construction materials used in road construction. Resour. Conserv. Recycl. 54. 250–255. doi:10.1016/j.resconrec.2009.08.007
Degli Esposti, A., Magrini, C., and Bonoli, A. (2020). Industrial symbiosis and strategies to enhance pavement sustainability, in Proceedings of the third SUN Conference of the Best practices on industrial symbiosis in Italy and the contribution of regional policies, Rimini, Italy. November 7, 2019. https://www.enea.it/en/publications/abstract/industrial-symbiosis-in-italy.
Del Fabbro, M., Stefanutti, M., and Ceschia, C. (2001). Impiego di derivati delle scorie di forno ad arco elettrico come materiale eco-compatibile nella sovrastruttura stradale, Quarry & Construction, Prince Edward Island C1N 4K5: PEI.
EAPA (2018). Asphalt in figures 2018. Available at: https://eapa.org/asphalt-in-figures/.
Espinoza, M., Campos, N., Yang, R., Ozer, H., Aguiar-Moya, J. P., Baldi Sevilla, A., et al. (2019). Carbon footprint estimation in road construction: La Abundancia–Florencia case study. Sustainability 11, 2276. doi:10.3390/su11082276
European Asphalt Pavement Association (EAPA) (2011). The asphalt pavement industry. A global perspective. 2nd Edn. Global series 101.
European Commission - Joint Research Centre - Institute for Environment and Sustainability (2010). International reference life cycle data system (ILCD) handbook - general guide for life cycle assessment - detailed guidance. 1st Edn (2010). EUR 24708 EN. Luxembourg: Publications Office of the European Union.
European Commission (2011). COM (2011) 21, Communication from the commission to the European parliament, the council, the European economic and social committee and the committee of the regions, a resource-efficient Europe–flagship initiative under the Europe 2020 strategy. Available at: https://eur-lex.europa.eu/LexUriServ/LexUriServ.do?uri=COM:2011:0021:FIN:EN:PDF
European Commission (2020). COM (2020) 98, Final communication from the commission to the European parliament, the council, the European economic and social committee and the committee of the regions. A new circular economy action plan- for a cleaner and more competitive Europe.
European Union Road Federation (2017). Road statistics. Available at: http://www.erf.be/wp-content/uploads/2018/01/Road_statistics_2017.pdf.
European Union Road Federation (2019). Road statistics. Available at: https://erf.be/statistics/road-network-2019/
Fernández-Sánchez, G., and Rodríguez-López, F. (2010). A methodology to identify sustainability indicators in construction project management- application to infrastructure projects in Spain. Ecol. Indicat., 10 (6), 1193–1201. doi:10.1016/j.ecolind.2010.04.009
Ferreira, V. J., Sáez-De-Guinoa Vilaplana, A., García-Armingol, T., Aranda-Usón, A., Lausín-González, C., López-Sabirón, A. M., et al. (2016). Evaluation of the steel slag incorporation as coarse aggregate for road construction: technical requirements and environmental impact assessment, J. Clean. Prod.,130, 175–186. doi:10.1016/j.jclepro.2015.08.094
Garbarino, E., Rodriguez Quintero, R., Donatello, S., Gama Caldas, M., and Wolf, O. (2016). EUR 28013 EN. Revision of green public procurement criteria for road design, construction and maintenance. Technical report and criteria proposal. doi:10.2791/683567
Gasparatos, A., El-Haram, M., and Horner, M. (2008). A critical review of reductionist approaches for assessing the progress towards sustainability Environ. Impact Assess. Rev. 28, 286–311. doi:10.1016/j.eiar.2007.09.002
Giani, M. I., Dotelli, G., Brandini, N., and Zampori, L. (2015). Comparative life cycle assessment of asphalt pavements using reclaimed asphalt, warm mix technology and cold in-place recycling. Resour. Conserv. Recycl. 104, 224–238. doi:10.1016/j.resconrec.2015.08.006
Gu, X., Yu, B., Dong, Q., and Deng, Y. (2018). Application of secondary steel slag in subgrade: performance evaluation and enhancement, J. Clean. Prod. 181, 102–108. doi:10.1016/j.jclepro.2018.01.172
Guo, S., Hu, J., and Dai, Q. (2018). A critical review on the performance of portland cement concrete with recycled organic components. J. Clean. Prod., 188, 92–112. doi:10.1016/j.jclepro.2018.03.244
Häkkinen, T., and Mäkelä, K. (1996). Environmental adaption of concrete: environmental impact of concrete and asphalt pavements. Espoo: VTT Technical Research Centre of Finland. VTT Tiedotteita - Meddelanden - Research Notes, No. 1752.
Hanson, C. S., and Noland, R. B. (2015). Greenhouse gas emissions from road construction: an assessment of alternative staging approaches. Transport. Res. Transport Environ. 40, 97–103. doi:10.1016/j.trd.2015.08.002
Hasan, U., Whyte, A., and Al Jassmi, H. (2020). Life cycle assessment of roadworks in United Arab Emirates: recycled construction waste, reclaimed asphalt pavement, warm-mix asphalt and blast furnace slag use against traditional approach, J. Clean. Prod. 257, doi:10.1016/j.jclepro.2020.120531
Hill, N., Brannigan, C., Wynn, D., Milnes, R., van Essen, H., den Boer, E., an Grinsven, A., Ligthart, T., and van Gijlswijk, R. (2012). The role of GHG emissions from infrastructure construction, vehicle manufacturing, and ELVs in overall transport sector emissions. Task 2 paper produced as part of a contract between European Commission Directorate-General Climate Action and AEA Technology plc. http://eutransportghg2050.eu/cms/assets/Uploads/Reports/EU-Transport-GHG-2050-II-Task-2-draftfinal1Mar12.pdf.
Huang, L., Bohne, R. A., Bruland, A., Jakobsen, P. D., and Lohne, J. (2015). Life cycle assessment of Norwegian road tunnel. Int. J. Life Cycle Assess. 20, 174–184. doi:10.1007/s11367-014-0823-1
Huang, Y., Bird, R. N., and Heidrich, O. (2009). Development of life cycle assessment tool for construction and maintenance of asphalt pavements. J. Clean. Prod. 17, 283–296. doi:10.1016/j.jclepro.2008.06.005
ISO (2006a). Environmental management–life cycle assessment–principles and framework (ISO 14040: 2006), European Standard EN ISO 14040, Geneva, Switzerland: The International Organization for Standardization.
ISO (2006b). Environmental management–life cycle assessment–requirements and guidelines (ISO 14044: 2006), European Standard EN ISO 14044, Geneva, Switzerland: The International Organization for Standardization.
Italian Ministerial Decree Number 69 (2018). Available at: https://www.gazzettaufficiale.it/eli/id/2018/06/18/18G00093/sg
Jamshidi, A., Kurumisawa, K., Nawa, T., Jize, M., and White, G. (2017). Performance of pavements incorporating industrial byproducts: a state-of-the-art study, J. Clean. Prod. 164, 367–388. doi:10.1016/j.jclepro.2017.06.223
Jiang, R., and Wu, P. (2019). Estimation of environmental impacts of roads through life cycle assessment: a critical review and future directions, Transport. Res. Transport Environ. 77, 148–163. doi:10.1016/j.trd.2019.10.010
JRC (2016). EUR 28013 EN. Revision of green public procurement criteria for road design, Construction and Maintenance. doi:10.2791/201271
Kalman, B., et al. (2013). Final report Re-road – summary report. Available online at http://re-road.fehrl.org/?m=48&id_directory=7325
Kandhal, P., and Mallick, R. (1997). Pavement recycling guidelines for state and local governments. https://rosap.ntl.bts.gov/view/dot/33835.
Martin-Portugues Montoliu, C., Casado Barrada, R., and Guedella Bustamante, E. (2019). Generation of circular economy models and use of renewable materials for a more sustainable pavement construction. Carreteras. 4 (223), 62–70.
Meng, H. D., and Liu, L. (2000). Stability processing technology and application prospect of steel slag. Steelmaking (in Chinese), 25 (6), 74–78.
Miliutenko, S., Björklund, A., and Carlsson, A. (2013). Opportunities from environmentally improved asphalt recycling: the example from Sweden. J. Clean. Prod. 43, 156–165. doi:10.1016/j.jclepro.2012.12.040
Mladenovic, A., Turk, J., Kovac, J., Mauko, A., and Cotic, Z. (2015). Environmental evaluation of two scenarios for the selection of materials for asphalt wearing courses. J. Clean. Prod. 87, 683–691. doi:10.1016/j.jclepro.2014.10.013
Morfeldt, J., Nijs, W., and Silveira, S. (2015). The impact of climate targets on future steel production–an analysis based on a global energy system model, J. Clean. Prod. 103, 469–482. doi:10.1016/j.jclepro.2014.04.045
Noferini, L., Simone, A., Sangiorgi, C., and Mazzotta, F. (2018). Investigation on performances of asphalt mixtures made with Reclaimed Asphalt Pavement: effects of interaction between virgin and RAP bitumen. International Journal of Pavement Research and Technology.10, 322–332. doi:10.1016/j.ijprt.2017.03.011
Noland, R. B., and Hanson, C. S., (2015). Life-cycle greenhouse gas emissions associated with a highway reconstruction: a New Jersey case study. J. Clean. Prod. 107, 731–740. doi:10.1016/j.jclepro.2015.05.064
Pantini, S., Borghi, G., and Rigamonti, L. (2018). Towards resource-efficient management of asphalt waste in Lombardy region (Italy): identification of effective strategies based on the LCA methodology. Waste Manag. 80, 423–434. doi:10.1016/j.wasman.2018.09.035
Pasetto, M., and Baldo, N., (2017). Dissipated energy analysis of four-point bending test on asphalt concretes made with steel slag and RAP. Int. J. Pavement Res. Technol. 10 (5), 446–453, doi:10.1016/j.ijprt.2017.07.004
Praticò, F. G., Vaiana, R., and Iuele, T., (2015). Permeable wearing courses from recycling reclaimed asphalt pavement for low-volume roads. Transportation research record. J. Transp. Res. Board, 2474 (1), 65–72. doi:10.3141/2474-08
Rashad, A. M. (2019). A synopsis manual about recycling steel slag as a cementitious material. J. Mater. Res. 8 (5), 4940–4955. doi:10.1016/j.jmrt.2019.06.038
Roberts, B. H. (2004). The application of industrial ecology principles and planning guidelines for the development of eco-industrial parks: an Australian case study. J. Clean. Prod. 12, 997–1010. doi:10.1016/j.jclepro.2012.12.040
Sangiorgi, C., Tataranni, P., Lantieri, C., and Mazzotta, F. (2019). Application of mining waste powder as filler in hot mix asphalt. MATEC Web of Conferences 274, 04002. doi:10.1051/matecconf/201927404002
Santero, N. J., and Horvath, A. (2009). Global warming potential of pavements. Environ. Res. Lett. 4, 3. doi:10.1088/1748-9326/4/3/034011
Santero, N. J., Masanet, E., and Horvath, A. (2011a). Life-cycle assessment of pavements. Part I: critical review. Resour. Conserv. Recycl. 55 (9-10), 801–809. doi:10.1016/j.resconrec.2011.03.010
Santero, N. J., Masanet, E., and Horvath, A. (2011b). Life-cycle assessment of pavements Part II: filling the research gaps. Resour. Conserv. Recycl. 55 (9-10), 810–818. doi:10.1016/j.resconrec.2011.03.009
Santos, J., Ferreira, A., and Flintsch, G. (2015). A life cycle assessment model for pavement management: road pavement construction and management in Portugal, Int. J. Pavement Eng. 16 (4), 315–336. doi:10.1080/10298436.2014.942862
SETAC, SETAC (Society of Environmental Toxicology and Chemistry) (1993). Definition, “code of practice”, Bruxelles.
Sherwood, P. (2001). Alternative materials in road construction- A guide to the use of recycled and secondary aggregates. 2nd Edn. London, United Kingdom: ICE Publishing.
Steger, S., and Bleischwitz, R. (2011). Drivers for the use of materials across countries, J. Clean. Prod. 19 (8), 816–826. doi:10.1016/j.jclepro.2010.08.016
Taddeo, R. (2016). Local industrial systems towards the eco-industrial parks: the model of the ecologically equipped industrial areas. J. Clean. Prod. 131, 189–197. doi:10.1016/j.jclepro.2016.05.051
UNI EN 12697-12 (2018). Bituminous mixtures - Test methods for hot mix asphalt – Part 12: Determination of the water sensitivity of bituminous specimen.
UNI EN 12697-23 (2003). Bituminous mixtures - test methods for hot mix asphalt - Part 23: determination of the indirect tensile strength of bituminous specimens.
UNI EN 12697-26 (2012). Bituminous mixtures - Test methods for hot mix asphalt - Part 26: Stiffness.
UNI EN 933-1 (2012). Tests for geometrical properties of aggregates - Part 1: determination of particle size distribution - sieving method.
Keywords: life cycle assessement, circular economy, industrial symbiosis, road construction, electric arc furnace steel slags, reclaimed asphalt pavement, recycled aggregates, standard characterization
Citation: Bonoli A, Degli Esposti A and Magrini C (2020) A Case Study of Industrial Symbiosis to Reduce GHG Emissions: Performance Analysis and LCA of Asphalt Concretes Made With RAP Aggregates and Steel Slags. Front. Mater. 7:572955. doi: 10.3389/fmats.2020.572955
Received: 09 July 2020; Accepted: 09 November 2020;
Published: 10 December 2020.
Edited by:
Emilia Morallon, University of Alicante, SpainCopyright © 2020 Bonoli, Degli Esposti and Magrini. This is an open-access article distributed under the terms of the Creative Commons Attribution License (CC BY). The use, distribution or reproduction in other forums is permitted, provided the original author(s) and the copyright owner(s) are credited and that the original publication in this journal is cited, in accordance with accepted academic practice. No use, distribution or reproduction is permitted which does not comply with these terms.
*Correspondence: Alessandra Bonoli, YWxlc3NhbmRyYS5ib25vbGlAdW5pYm8uaXQ=; Chiara Magrini, Y2hpYXJhLm1hZ3Jpbmk3QHVuaWJvLml0
Disclaimer: All claims expressed in this article are solely those of the authors and do not necessarily represent those of their affiliated organizations, or those of the publisher, the editors and the reviewers. Any product that may be evaluated in this article or claim that may be made by its manufacturer is not guaranteed or endorsed by the publisher.
Research integrity at Frontiers
Learn more about the work of our research integrity team to safeguard the quality of each article we publish.