- 1School of Materials Science and Engineering, University of Jinan, Jinan, China
- 2State Key Laboratory of Biobased Material and Green Papermaking, Qilu University of Technology (Shandong Academy of Sciences), Jinan, China
Ce2(Zr0.94Sn0.06)3(MoO4)9 (CZSM) ceramics were synthesized via a traditional solid-state method. The effects of Sn-substitution on phase composition, microstructure, and microwave dielectric properties as a function of sintering temperature were discussed. The XRD results indicated that all the samples exhibited a single phase. The chemical bonds of CZSM ceramics were calculated based on P-V-L theory, which could be used to evaluate the relationship between structure and microwave dielectric properties. The tendency of dielectric constant was depended on the theoretical dielectric polarizability and bond ionicity. Moreover, the improvement of Q·f value was ascribed to the increase of packing fraction. The reduced τf value could be explained by decrease of the bond energy, enhanced the co-efficient of thermal expansion and increase of bond valence (VZr(Sn)). The complex permittivity values were obtained from infrared reflectivity spectra. The dielectric permittivity and loss were 10.92 and 3.08 × 10−4, respectively, which agreed well with the measured value. Typically, the optimal microwave dielectric properties of εr = 10.35, Q·f = 59,660 GHz (at 9.70 GHz), and τf = −7.52 ppm/°C were achieved in CZSM ceramics sintered at 800°C for 6 h.
Introduction
Owing to the characteristics of light weight, low cost, and high performance, the microwave dielectric ceramics have been widely used as filters, waveguides, resonators, substrates, and waveguides (Cava, 2001; Reaney and Iddles, 2006; Li et al., 2018; Chen et al., 2019; Cheng et al., 2019; Wu et al., 2019). Currently, 5th generation mobile communication systems have received increasing attention (Zhou et al., 2017; Pang and Zhou, 2019; Bafrooei et al., 2020; Lin et al., 2020). To meet the microwave devices applied at millimeter wave, new materials and technologies are required. As a new ceramic fabrication technology, the low temperature co-firing ceramics (LTCC) technology have been intensively studied, it provide the platform for fabrication of three-dimensional ceramic modules (Yu et al., 2015; Sebastian et al., 2016; Guo et al., 2019; Hsiang et al., 2019; Song et al., 2020). Materials in this discipline require a low sintering temperature to co-fire with electrode material and suitable properties: a low dielectric constant (εr) to avoid the signal delay, a high quality factor (Q·f) for better selectivity at higher frequencies and a near-zero temperature coefficient of resonant frequency (τf) for the frequency stability (Song et al., 2018; Lin et al., 2020). Therefore, it is essential to develop the microwave materials with low permittivity and high Q·f value.
Recently, Mo-based dielectric ceramics have been widely reported owing to low sintering temperature (<960°C) and good microwave dielectric properties (Choi et al., 2007; Pang et al., 2011; Surjith and Ratheesh, 2013; Zhang et al., 2015, 2019; Liu and Zuo, 2017, 2018; Tao et al., 2019; Xing et al., 2019; Zhang and Wu, 2019; Zheng et al., 2020). Among these excellent materials, the Ce2Zr3(MoO4)9 ceramic was regarded as candidate for LTCC materials applications due to low sintering temperature and near-zero τf value (Tao et al., 2019). However, the Ce2Zr3(MoO4)9 ceramics exhibited a higher dielectric losses, some strategies should be adopted in this paper. A large of studies for improving microwave dielectric properties have been carried out via Sn4+ ions substitution (Yang et al., 2009; Ma et al., 2013; Li et al., 2019). For instance, when x value increased from 0.0 to 0.6, the quality factor of Ca(Sn1−xSix)O3 (x = 0.5–1.0) ceramics increased from 16,000 to 63,000 GHz (Ma et al., 2013). The optimal microwave dielectric properties of Mg2(Ti0.8Sn0.2)O4 ceramics with εr = 12.18, Q·f = 170,130 GHz, and τf = −53.1 ppm/°C (Li et al., 2019). Thus, Sn4+ ions substitution was taken to improve Q·f value of Ce2Zr3(MoO4)9 ceramics in this work. The Ce2(Zr0.94Sn0.06)3(MoO4)9 (CZSM) ceramics were successfully prepared. The phase composition, sintering characteristics, microstructure, and dielectric properties of CZSM ceramics were investigated scientifically. Infrared reflectivity spectra (IR) and chemical bonds theory of complex crystals were adopted as useful tools to analyze the effect of intrinsic factor on microwave dielectric properties of CZSM ceramics.
Experimental
The reagent grade CeO2 (99.9%, Macklin, China), ZrO2 (99.99%, Macklin, China), SnO2 (99.95%, Aladdin, China), and MoO3 (99.95%, Aladdin, China) powders were used to fabricate CZSM ceramics by the traditional solid-state method. Based on the chemical formula, the raw materials were mixed by zirconia balls for 24 h with absolute ethanol as media. After drying at a temperature 80°C, the slurries were calcined at 700°C for 2 h. Then, the pre-sintered powders were crushed and re-milled for 24 h. Subsequently, 12 wt.% PVA added to the mixture, and the powders were pressed into pellets (10 mm in diameter and 6 mm in height). Finally, all the samples were sintered at 650–850°C for 6 h in air.
XRD was applied to examine the phase compositions of the sintered samples with Cu Kα radiation (D8 Advance, Bruker Co., Germany). The structure parameters and cell volume were obtained from refinement of XRD data using FullProf program. The microstructures of sintered samples were observed using SEM (FEI Co., United States) coupled with EDS. The εr value at microwave frequencies (13–15 GHz) and the Q·f value at microwave frequencies (9–11 GHz) were measured using the network analyzer (N5234A, Agilent Co., USA) (Hakki and Coleman, 1960; Courtney, 1970). The τf value was calculated using Equation (1):
where f1 and f2 are the resonant frequencies at the measuring temperatures T1 (25°C) and T2 (85°C), respectively. The apparent density was measured via the Archimedes method, and the theoretical density can be obtained through Equation (2).
where A, Z, and NA are the atomic weight, number of atom in unit cell and volume of unit cell, respectively. The relative density was calculated by Equation (3):
Results and Discussion
Figure 1 presented the XRD patterns of CZSM ceramics sintered between 650–850°C. It was observed that all of diffraction patterns match well with the Pr2Zr3(MoO4)9 (PDF#51-1851) phase, with no secondary phase detected. It indicated that all of sintered samples formed single phase solid solution, which belongs to trigonal system with R-3c (167) space group. To determinate its structure, refinement of the XRD data were conducted using FullProf program with Rietveld's method. The Nd2Zr3(MoO4)9 (ICSD File No. 92600) was used as starting structural model. The refinement pattern of samples sintered at range of 650–850°C, as shown in Figure 2. The main crystallographic parameters and reliability factors of Rwp, Rp, and χ2 for samples are summarized in Table 1. The Rwp, Rp, and χ2 values were found to be in the range of 9.5–10.9, 7.2–8.6%, and 1.8–2.5, respectively. The value χ2 were smaller to ensure the reliability of refinement. With the increasing sintering temperature, the a and b gradually increased from 9.8251 to 9.8269 Å, and c increased from 58.8278 to 58.8434 Å, which resulted in Vm increased from 4918.02 Å3 at 650°C to 4921.14 Å3 at 800°C. Figure 3 presented crystal structure of CZSM ceramics. It can be seen that the Ce, Zr(1), Zr(2), Mo(1), and Mo(2) atoms are nearest neighbors to 9, 6, 6, 4, and 4 oxygen atoms, respectively. The [CeO9] occupying the large cavities of the structure, the [CeO9] and [ZrO6] were connected by [MoO4] at the structure.
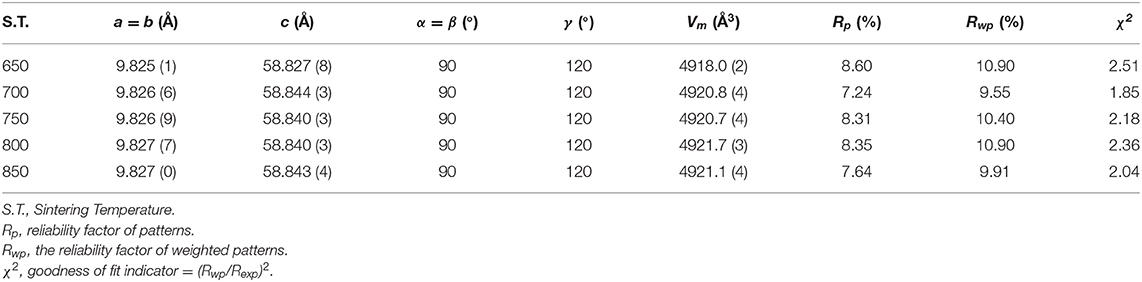
Table 1. The refinement parameters, theoretical densities and relative densities of Ce2(Zr0.94Sn0.06)3(MoO4)9 ceramics fired at 650–800°C.
Figure 4 illustrated the curves of the apparent and relative density of CZSM samples sintered at 650–850°C. The apparent densities increased from 3.50 g/cm3 to the maximum value of 3.90 g/cm3 with increasing sintering temperature. The relative density have same tendency as apparent density, a high relative density (>94%) was achieved at 750–850°C. Typical SEM images of CZSM ceramics sintered at different temperature were shown in Figures 5A–E. Grain boundaries were evident in all the samples, indicating that crystalline grains grew well in these samples. Moreover, the grain size increased from 1.93 to 3.19 μm and the pores gradually decreased with the increase of sintering temperature. The result of EDS about grain from samples sintered at 800°C was presented in Figure 5F. The ratio of Ce, Zr, Sn, Mo, and O atom were 3.93, 6.56, 0.34, 18.50, and 70.67%, respectively, which was corresponded to theoretical composition of CZSM ceramic.
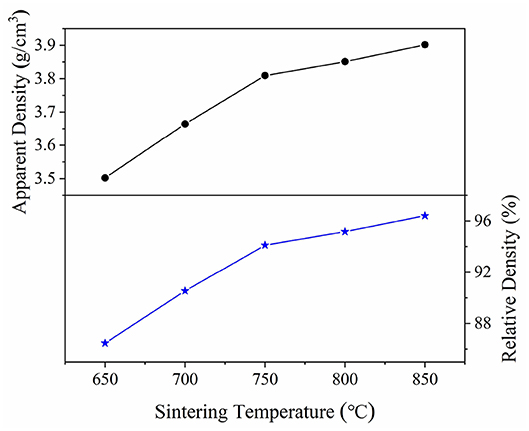
Figure 4. Apparent and relative density of Ce2(Zr0.94Sn0.06)3(MoO4)9 ceramics as a function of sintering temperature.
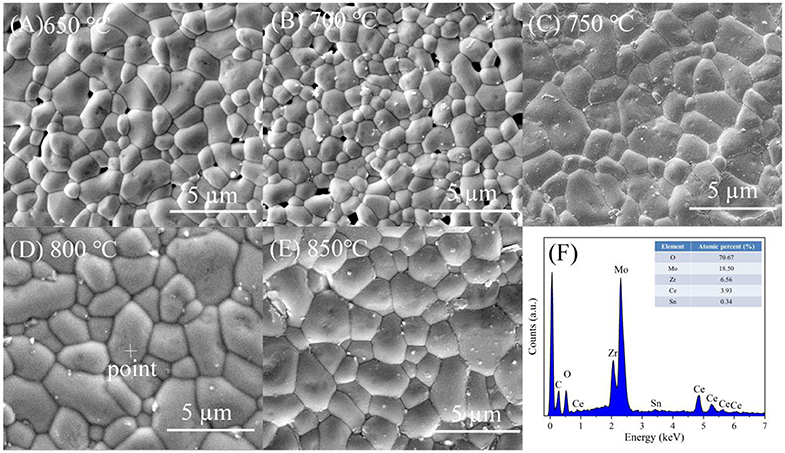
Figure 5. Typical SEM micrographs of Ce2(Zr0.94Sn0.06)3(MoO4)9 ceramics fired at (A) 650°C, (B) 700°C, (C) 750°C, (D) 800°C, (E) 850°C, and (F) EDS analysis about grain selected randomly from the sample sintered at 800°C.
The curves of microwave dielectric properties for CZSM samples were shown in Figure 6. The dielectric constant (εr) increased and reached maximum value of 10.47 as the temperature shifted from 650 to 750°C, and then kept stable with further increasing sintering temperature. In addition, the tendency of the εr value was corresponded to the density when sintering temperature at 650–800°C, suggesting that dielectric constant was closely related to the density in lower temperature. As we all know, apart from the extrinsic factors like density and phase composition, the εr also affected by the intrinsic factors such as polarizability and bond ionicity (Bi et al., 2018; Manan et al., 2018; Zhang et al., 2019). The XRD result suggesting the single phase ceramics was formed. Thus, the εr value of CZSM ceramics were mainly determined by polarizability and bond ionicity when the sample was compact. The theoretical dielectric polarizability (αtheo.) and observed dielectric polarizability (αobs.) were calculated through Equations (4) and (5), respectively.
where α(Ce3+) = 6.15 Å3, α(Zr4+) = 3.25 Å3, α(Sn4+) = 2.83 Å3, α(O2+) = 2.01 Å3 was reported by Shannon et al. and α(Mo6+) = 3.28 Å3 is calculated by Choi et al. (Shannon, 1993; Choi et al., 2007). Moreover, Vm is the molar volume and b is a constant value (4π/3), respectively. The results were listed in Table S1. The αtheo. value (123.85 Å3) lower than the Ce2Zr3(MoO4)9 ceramics (123.93 Å3), which could be attributed to the smaller polarizability of Sn4+:α(Sn4+) = 2.83 Å3 < α(Zr4+) = 3.25 Å3 (Tao et al., 2019). Besides, the tendency of εr value was similar to the αtheo. value and αobs. value, indicating that the reduced εr value was strongly dependent on the decrease of polarizability.
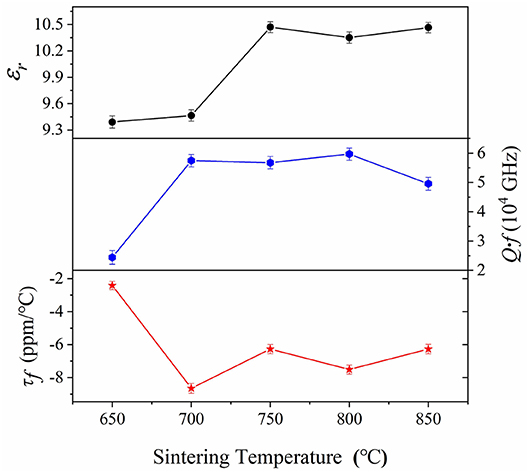
Figure 6. Curves of microwave dielectric properties as a function of sintering temperature for Ce2(Zr0.94Sn0.06)3(MoO4)9 ceramics in the temperature range from 650 to 850°C.
Moreover, the quality factor(Q·f) increased from 24,410 to 59,660 GHz, and then declined slightly with further increasing sintering temperature, the maximum of the Q·f was 59,660 GHz at 800°C. In general, the dielectric losses are composed of intrinsic losses (lattice vibration) and extrinsic losses (grain size and impure phase). The grain size showed upward tendency with increasing sintering temperature, it was similar to the variation of Q·f value. Therefore, the increased grain size was important for improvement of the Q·f value (Yang et al., 2002, 2019; Ichinose and Shimada, 2006). In order to investigate the effect of the intrinsic factors, the packing fraction has been calculated by Equation (6).
where Z is the number of molecules per cell. Kim et al. reported the effective ionic size (Kim et al., 2010). The results indicated that the smaller ions size of Sn4+ (0.69 Å) substitution to Zr4+ (0.72 Å) lead to the higher packing fraction (47.84 > 47.74%). Thus, the improvement of Q·f value was ascribed to the increase of packing fraction. Furthermore, it suggested that the Sn4+ ions substitution could improve the quality factor of Ce2Zr3(MoO4)9 ceramics.
The temperature co-efficient of resonant frequency (τf) of CZSM ceramics sintered at different temperatures from 650 to 850°C was shown in Figure 6. It can be seen that the saturation value of τf = −7.52 ppm/°C was obtained when sintered at 800°C and kept stable at 700–850°C. The temperature coefficient of resonant frequency was associated with bond valence (Yoon et al., 2003; Cai et al., 2015). Therefore, the bond valence of [Zr(Sn)O6] octahedra can be obtained by Equations (7) and (8).
where Vij represent the sum of all the bond valences, Rij is the bond valence parameter, dij is the length of the bond between atoms i and j, and b is an constant equal to 0.37. The results of bond valence were listed in Table S1. The bond valence (VZr(Sn)) increased from 8.92 to 9.17 with the Sn4+ substitution, and the τf value correlated to the change of bond valence (VZr(Sn)) (Tao et al., 2019). It indicated that the decline of τf value was related to the increase of bond valence (VZr(Sn)).
Figure 7 showed the microwave dielectric properties of Mo-based dielectric ceramics with low dielectric constant, and the parameters were summarized in Table S2 (Choi et al., 2007; Pang et al., 2011; Surjith and Ratheesh, 2013; Zhang et al., 2015, 2019; Liu and Zuo, 2017, 2018; Tao et al., 2019; Xing et al., 2019; Zhang and Wu, 2019; Zheng et al., 2020). The CZSM ceramics possessed excellent microwave dielectric properties and low sintering temperature in this system. It suggests that they have potential for exploitation in LTCC technology. Certainly, it is necessary to study the relationship between structure and microwave dielectric properties. Thus, the bond ionicity, lattice energy, bond energy, and coefficients of thermal expansion were calculated to investigate the relationships between chemical bond and dielectric properties of the CZSM ceramic. Based on P-V-L theory, the complex crystals CZSM were decomposed into the sum of binary crystals as Equation (9) (Xue and Zhang, 1996; Wu et al., 1998; Yang et al., 2019):
Equation (10) indicated that the dielectric constant can be predicted by bond ionicity (Batsanov, 1982).
where n is the refractive index. The bond ionicity were calculated using the Equations (11)–(13) (Xue and Zhang, 1996; Wu et al., 1998).
where is the average energy gap, and it can be separated into homopolar and heteropolar cμ parts. The exp(-) is Thomas-Fermi prescreening factor, and it was calculated through Equations (14) and (15) (Levine, 1973). The fi value of CZSM ceramics were shown in Figure 8, and the results were listed in Table 2. Decreasing sequence of fi was fi (Ce-O)> fi (Zr/Sn-O)> fi (Mo-O), the largest fi value of 85.64% was obtained in Ce-O bond. Besides, the average of fi (Ce-O) value decreased from 85.18 to 85.14% with the Sn4+ doped shifted from 0 to 0.06. The tendency of the εr value was consisted with the variation of fi (Ce-O) value. It indicated that the reduced εr value was mainly dominated by the degressive bond ioniticy of Ce-O bond.
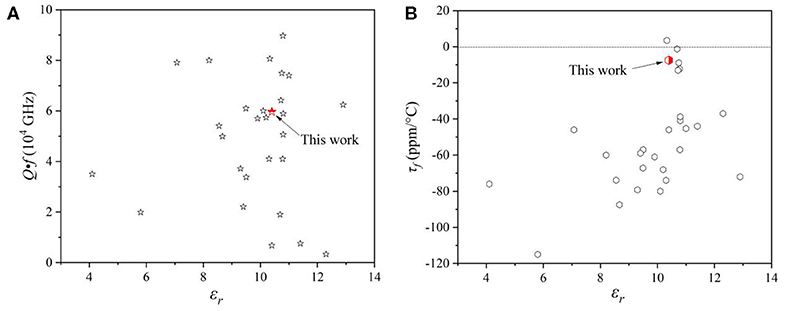
Figure 7. (A) Quality factors (Q·f) and (B) the temperature coefficient of resonant frequency (τf) of Mo-based microwave dielectric ceramics with a low dielectric constant.
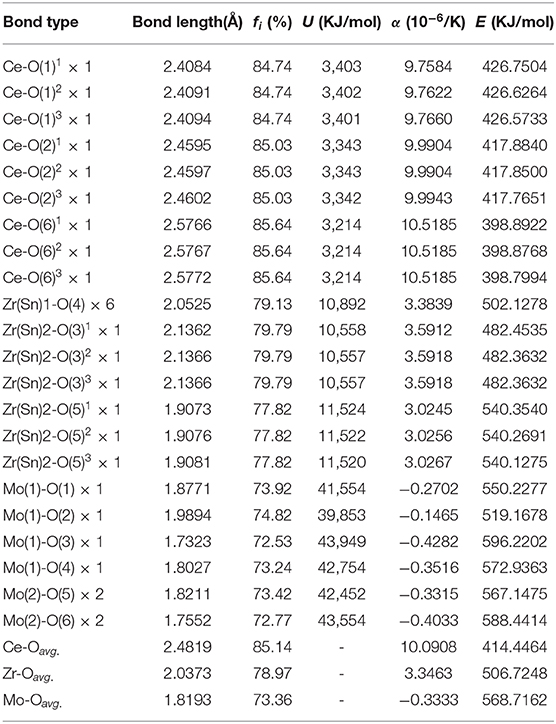
Table 2. Band ionicity fi, lattice energy U, bond energy E, and coefficient of thermal expansion α of each bond for Ce2(Zr0.94Sn0.06)3(MoO4)9 ceramic sintered at 800°C.
The dielectric loss can be control by the binding ability between cation and oxygen ion, high binding energy corresponds to low intrinsic loss. Thus, lattice energy of CZSM ceramics can be calculated by Equations (16)–(18) (Xue and Zhang, 1996; Wu et al., 1998).
where the lattice energy can be separated into covalent and ionic parts. According to Figure 9, the sequence of U (Mo-O)>U (Zr/Sn-O)>U (Ce-O) suggested that Mo-O bond play a vital role in enhancing the stability of ionic crystals.
What's more, the τf should be considered for realistic application of microwave dielectric ceramic. High bond energy could lead to a small absolute τf value. Hence, the bond energy can be obtained by Equations (19)–(21) (Sanderson, 1968, 1971, 1983):
where rcA and rcB are the covalent radii of atom A and atom B; ti and tc are ionic and covalent proportional coefficient of an individual bond μ. The bond energy EA−A and EB−B can be referred to handbook (Luo, 2007). What's more, SA and SB are the electronegativities of A and B atoms. Figure 10 illustrated bond energy of different bond type for Ce2(Zr0.94Sn0.06)3(MoO4)9 ceramics. The bond energy of Mo-O bond was larger than others, it suggested that Mo-O bond provided major contribution to the structural stability of CZSM ceramics. Compared with the Ce2Zr3(MoO4)9 ceramic, the bond energy of Mo-O bond declined from 574.8383 to 568.7162 KJ/mol. Meanwhile, the τf value was positively correlated with the change of bond energy (EMo−O). It suggested that the downward trend τf value could be explained by the decrease of bond energy (EMo−O).
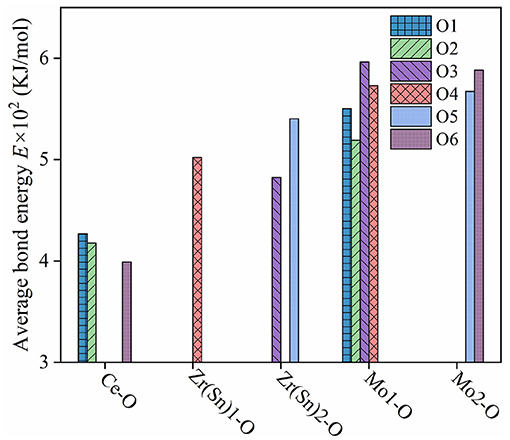
Figure 10. Bond energy of different bond type for Ce2(Zr0.94Sn0.06)3(MoO4)9 ceramics sintered at 800°C.
The τf value was negatively related to the coefficients of thermal expansion, which can be obtained via Equation (22). Thus, the coefficients of thermal expansion were calculated based on P-V-L theory (Hakki and Coleman, 1960; Courtney, 1970; Xing et al., 2019).
where τε is temperature coefficient of the dielectric constant. The average values of Ce-O, Mo-O, and Zr/Sn-O bond were 10.0908 × 10−6 K−1, −0.3333 × 10−6 K−1 and 3.3463 × 10−6 K−1, respectively. In addition, The Mo-O bonds had a positive effect on τf value due to αMo−O value was negative. The αMo−O value increased from −0.3575 × 10−6 K−1 to −0.3333 × 10−6 K−1 because of the Sn4+ substitution for Zr4+. The higher αMo−O value result in large absolute value. Hence, the reduced τf value was associated with enhanced the thermal expansion coefficient (αMo−O).
IR reflectivity spectra of CZSM ceramics was exhibited in Figure 11. Based on the classical harmonic oscillator model, the infrared spectra were fitted with 24 internal modes, which were listed in Table 3 (Petzelt and Kamba, 2003). ε* (ω) (the complex dielectric permittivity) and R (ω) (the complex reflectivity) were calculated by Equations (23) and (24) (Wakino et al., 1986; Li et al., 2016; Tang et al., 2019; Guo et al., 2020). Furthermore, the tan δ (dielectric loss tangent) can be obtained through Equation (25).
where ε∞ is dielectric constant by electronic polarization, n is the number of transverse phonon modes, ωpj, ωoj, and γj are plasma frequency, transverse frequency and damping factors of the j-th Lorentz oscillator, respectively.
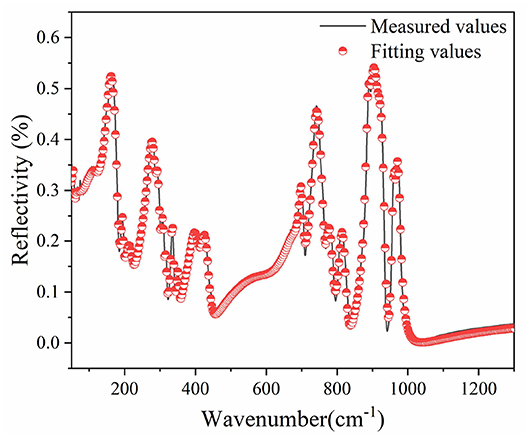
Figure 11. Measured (black line) and fitted (red circle) infrared reflectivity spectra of Ce2(Zr0.94Sn0.06)3(MoO4)9 ceramics sintered at 800°C.
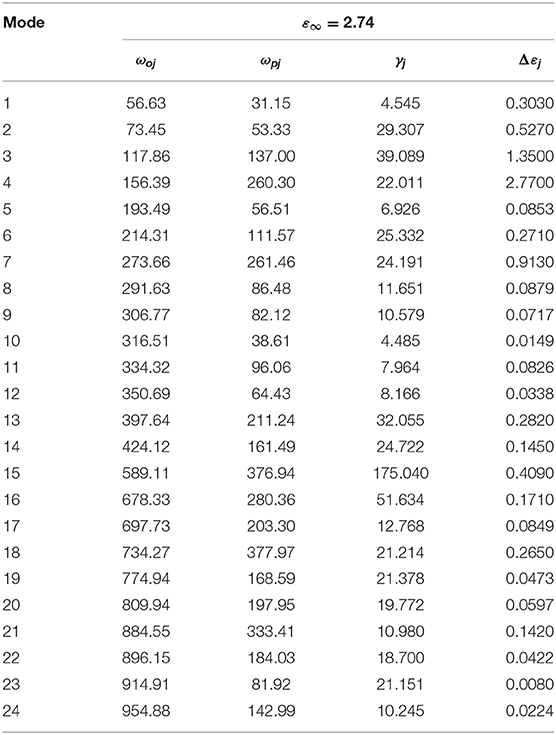
Table 3. Phonon parameters obtained from the fitting of the infrared spectra of Ce2(Zr0.94Sn0.06)3(MoO4)9 ceramic sintered at 800°C.
Figure 12 presented the complex permittivity of CZSM ceramics sintered at 800°C. The extrapolated dielectric permittivity and loss were 10.92 and 3.08 × 10−4, respectively. It can be seen that the calculated permittivity was slightly higher than the measured permittivity, the measured and calculated dielectric losses of CZSM ceramic were comparable with the same order of magnitudes. Therefore, the major dielectric contribution of the CZSM ceramics was in microwave region, and related to the absorptions of phonon oscillation.
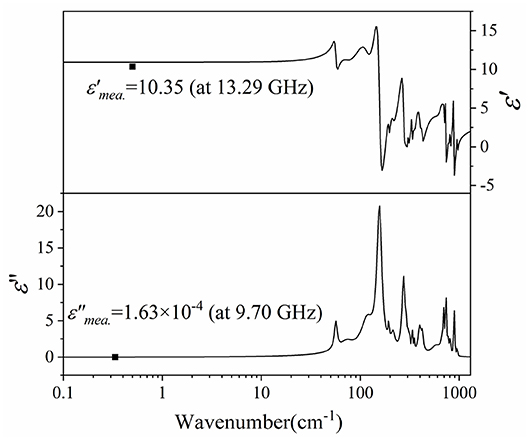
Figure 12. The real and imaginary parts of the complex permittivity for Ce2(Zr0.94Sn0.06)3(MoO4)9 ceramics sintered at 800°C (black points are measured value at microwave region).
Conclusion
Ce2(Zr0.94Sn0.06)3(MoO4)9 (CZSM) microwave dielectric ceramics were successfully prepared. The XRD patterns demonstrated that all samples displayed a single phase. The SEM results indicated that nearly compact structure can be obtained sintered at 750–850°C. Subsequently, the dependence of microwave dielectric properties on intrinsic factors was evaluated based on P-V-L theory. The decrease of dielectric constant was mainly dominated by the decline of polarizability and bond ioniticy of Ce-O bond. The improvement of quality factor was dependent on enhanced packing fraction. In addition, the reduced temperature coefficient of resonant frequency was related to the increase of thermal expansion coefficient and decrease of bond energy (EMo−O). The complex permittivity values were obtained by infrared spectra, which agreed well with the measured value. The CZSM ceramic sintered at 800°C exhibited an optimum microwave dielectric properties of εr = 10.35, Q·f = 59,660 GHz (at 9.70 GHz) and τf = −7.52 ppm/°C.
Data Availability Statement
The raw data supporting the conclusions of this article will be made available by the authors, without undue reservation, to any qualified researcher.
Author Contributions
HT: methodology, investigation, data curation, visualization, writing-original draft, and writing-review and editing. XL: investigation, validation, methodology, software and formal analysis. YY: software, validation, writing-review and editing. HW and ZZ: conceptualization, resources, writing-review and editing, project administration, supervision, and funding acquisition.
Funding
This work was supported by the National Natural Science Foundation of China (No. 51972143).
Conflict of Interest
The authors declare that the research was conducted in the absence of any commercial or financial relationships that could be construed as a potential conflict of interest.
Supplementary Material
The Supplementary Material for this article can be found online at: https://www.frontiersin.org/articles/10.3389/fmats.2020.00145/full#supplementary-material
References
Bafrooei, H. B., Liu, B., Su, W. T., and Song, K. X. (2020). Ca3MgSi2O8: novel low-permittivity microwave dielectric ceramics for 5G application. Mater. Lett. 263:127248. doi: 10.1016/j.matlet.2019.127248
Batsanov, S. S. (1982). Dielectric methods of studying the chemical bond and the concept of electronegativity. Russ. Chem. Rev. 51, 684–697. doi: 10.1070/RC1982v051n07ABEH002900
Bi, J. X., Xing, C. F., Yang, C. H., and Wu, H. T. (2018). Phase composition, microstructure and microwave dielectric properties of rock salt structured Li2ZrO3-MgO ceramics. J. Eur. Ceram. Soc. 38, 3840–3846. doi: 10.1016/j.jeurceramsoc.2018.04.034
Cai, H. C., Li, L. X., Gao, Z. D., and Lv, X. S. (2015). A temperature stable microwave dielectric material Ni0.35Zn0.65TiNb2O8. J. Mater. Sci. Mater. Electron. 26, 998–1003. doi: 10.1007/s10854-014-2495-9
Cava, R. J. (2001). Dielectric materials for applications in microwave communications. J. Mater. Chem. 11, 54–62. doi: 10.1039/b003681l
Chen, X. L., Li, X., Zhou, H. F., Sun, J., Li, X. X., Yan, X., et al. (2019). Phase evolution, microstructure, electric properties of (Ba1−xBi0.67xNa0.33x)(Ti1−xBi0.33xSn0.67x)O3 ceramics. J. Adv. Ceram. 8, 427–437. doi: 10.1007/s40145-019-0326-4
Cheng, K., Li, C. C., Yin, C. Z., Tang, Y., Sun, Y. H., and Fang, L. (2019). Effects of Sr2+ substitution on the crystal structure, Raman spectra, bond valence and microwave dielectric properties of Ba3−xSrx(VO4)2 solid solutions. J. Eur. Ceram. Soc. 39, 3738–3743. doi: 10.1016/j.jeurceramsoc.2019.05.030
Choi, G. K., Kim, J. R., Yoon, S. H., and Hong, K. S. (2007). Microwave dielectric properties of scheelite (A=Ca, Sr, Ba) and wolframite (A=Mg, Zn, Mn) AMoO4 compounds. J. Eur. Ceram. Soc. 27, 3063–3067. doi: 10.1016/j.jeurceramsoc.2006.11.037
Courtney, W. E. (1970). Analysis and evaluation of a method of measuring the complex permittivity and permeability microwave insulators. IEEE Trans. Microw. Theory Tech. 18, 476–485. doi: 10.1109/TMTT.1970.1127271
Guo, H. H., Zhou, D., Liu, W. F., Pang, L. X., Wang, D. W., Su, J. Z., et al. (2020). Microwave dielectric properties of temperature-stable zircon-type (Bi, Ce)VO4 solid solution ceramics. J. Am. Ceram. Soc. 103, 423–431. doi: 10.1111/jace.16759
Guo, H. H., Zhou, D., Pang, L. X., and Qi, Z. M. (2019). Microwave dielectric properties of low firing temperature stable scheelite structured (Ca, Bi)(Mo, V)O4 solid solution ceramics for LTCC applications. J. Eur. Ceram. Soc. 39, 2365–2373. doi: 10.1016/j.jeurceramsoc.2019.02.010
Hakki, B. W., and Coleman, P. D. (1960). A dielectric resonator method of measuring inductive capacities in the millimeter range. IRE Trans. Microw. Theory Tech. 8, 402–410. doi: 10.1109/TMTT.1960.1124749
Hsiang, H. I., Chen, C. C., and Yang, S. Y. (2019). Microwave dielectric properties of Ca0.7Nd0.2TiO3 ceramic-filled CaO-B2O3-SiO2 glass for LTCC applications. J. Adv. Ceram. 8, 345–351. doi: 10.1007/s40145-019-0316-6
Ichinose, N., and Shimada, T. (2006). Effect of grain size and secondary phase on microwave dielectric properties of Ba(Mg1/3Ta2/3)O3 and Ba([Mg,Zn]1/3Ta2/3)O3 systems. J. Eur. Ceram. Soc. 26, 1755–1759. doi: 10.1016/j.jeurceramsoc.2005.09.032
Kim, E. S., Chun, B. S., Freer, R., and Cernik, R. J. (2010). Effects of packing fraction and bond valence on microwave dielectric properties of A2+B6+O4 (A2+: Ca, Pb, Ba; B6+: Mo, W) ceramics. J. Eur. Ceram. Soc. 30, 1731–1736. doi: 10.1016/j.jeurceramsoc.2009.12.018
Levine, B. F. (1973). Bond susceptibilities and ionicities in complex crystal structures. J. Chem. Phys. 59, 1463–1486. doi: 10.1063/1.1680204
Li, C. C., Xiang, H. C., Xu, M. Y., Tang, Y., and Fang, L. (2018). Li2AGeO4 (A=Zn, Mg): two novel low-permittivity microwave dielectric ceramics with olivine structure. J. Eur. Ceram. Soc. 38, 1524–1528. doi: 10.1016/j.jeurceramsoc.2017.12.038
Li, H., Zhang, P. C., Yu, S. Q., Yang, H. Y., Tang, B., Li, F. H., et al. (2019). Structural dependence of microwave dielectric properties of spinel structured Mg2(Ti1−xSnx)O4 solid solutions: crystal structure refinement, Raman spectra study and complex chemical bond theory. Ceram. Int. 45, 11639–11647. doi: 10.1016/j.ceramint.2019.03.037
Li, W. B., Zhou, D., Xi, H. H., Pang, L. X., and Yao, X. (2016). Structure, infrared reflectivity and microwave dielectric properties of (Na0.5La0.5)MoO4-(Na0.5Bi0.5)MoO4 ceramics. J. Am. Ceram. Soc. 99, 2083–2088. doi: 10.1111/jace.14175
Lin, Q. B., Song, K. X., Lin, B., Bafrooei, H. B., Zhou, D., Su, W. T., et al. (2020). Vibrational spectroscopy and microwave dielectric properties of AY2Si3O10 (A=Sr, Ba) ceramics for 5G applications. Ceram. Int. 46, 1171–1177. doi: 10.1016/j.ceramint.2019.09.086
Liu, W. Q., and Zuo, R. Z. (2017). Low temperature fired Ln2Zr3(MoO4)9 (Ln=Sm, Nd) microwave dielectric ceramics. Ceram. Int. 43, 17229–17232. doi: 10.1016/j.ceramint.2017.09.083
Liu, W. Q., and Zuo, R. Z. (2018). A novel low-temperature firable La2Zr3(MoO4)9 microwave dielectric ceramic. J. Eur. Ceram. Soc. 38, 339–342. doi: 10.1016/j.jeurceramsoc.2017.08.023
Luo, Y. R. (2007). Comprehensive Handbook of Chemical Bond Energies. Boca Raton: CRC Press. doi: 10.1201/9781420007282
Ma, Q., Wu, S. P., Jiang, C., and Li, J. H. (2013). Microwave dielectric properties of SnO2-doped CaSiO3 ceramics. Ceram. Int. 39, 2223–2229. doi: 10.1016/j.ceramint.2012.08.066
Manan, A., Ullah, Z., Ahmad, A. S., Ullah, A., Khan, D. F., Hussain, A., et al. (2018). Phase microstructure evaluation and microwave dielectric properties of (1-x)Mg0.95Ni0.05Ti0.98Zr0.02O3-xCa0.6La0.8/3TiO3 ceramics. J. Adv. Ceram. 7, 72–78. doi: 10.1007/s40145-018-0258-4
Pang, L. X., Sun, G. B., and Zhou, D. (2011). Ln2Mo3O12 (Ln=La, Nd): a novel group of low loss microwave dielectric ceramics with low sintering temperature. Mater. Lett. 65, 164–166. doi: 10.1016/j.matlet.2010.09.064
Pang, L. X., and Zhou, D. (2019). Modification of NdNbO4 microwave dielectric ceramic by Bi substitutions. J. Am. Ceram. Soc. 102, 2278–2282. doi: 10.1111/jace.16290
Petzelt, J., and Kamba, S. (2003). Submillimetre and infrared response of microwave materials: extrapolation to microwave properties. Mater. Chem. Phys. 79, 175–180. doi: 10.1016/S0254-0584(02)00269-9
Reaney, I. M., and Iddles, D. (2006). Microwave dielectric ceramics for resonators and filters in mobile phone networks. J. Am. Ceram. Soc. 7, 2063–2072. doi: 10.1111/j.1551-2916.2006.01025.x
Sanderson, R. T. (1968). Multiple and single bond energies in inorganic molecules. Inorg. Nucl. Chem. Lett. 30, 375–393. doi: 10.1016/0022-1902(68)80464-6
Sanderson, R. T. (1983). Electronegativity and bond energy. J. Am. Chem. Soc. 105, 2259–2261. doi: 10.1021/ja00346a026
Sebastian, M. T., Wang, H., and Jantunen, H. (2016). Low temperature co-fired ceramics with ultra-low sintering temperature: a review. Curr. Opin. Solid. State. Mater. Sci. 3, 151–170. doi: 10.1016/j.cossms.2016.02.004
Shannon, R. D. (1993). Dielectric polarizabilities of ions in oxides and fluorides. J. Appl. Phys. 73, 348–366. doi: 10.1063/1.353856
Song, J. B., Song, K. X., Wei, J. S., Lin, H. X., Wu, J., Xu, J. M., et al. (2018). Ionic occupation, structures, and microwave dielectric properties of Y3MgAl3SiO12 garnet-type ceramics. J. Am. Ceram. Soc. 101, 244–251. doi: 10.1111/jace.15174
Song, X. Q., Lei, W., Zhou, Y. Y., Chen, T., Ta, S. W., and Fu, Z. X. (2020). Ultra-low fired fluoride composite microwave dielectric ceramics and their application for BaCuSi2O6-based LTCC. J. Am. Ceram. Soc. 103, 1140–1148. doi: 10.1111/jace.16795
Surjith, A., and Ratheesh, R. (2013). High Q ceramics in the ACe2(MoO4)4 (A=Ba, Sr and Ca) system for LTCC applications. J. Alloy. Compd. 550, 169–172. doi: 10.1016/j.jallcom.2012.09.055
Tang, Y., Xu, M. Y., Duan, L., Chen, J. Q., Li, C. C., Xiang, H. C., et al. (2019). Structure, microwave dielectric properties, and infrared reflectivity spectrum of olivine type Ca2GeO4 ceramic. J. Eur. Ceram. Soc. 39, 2354–2359. doi: 10.1016/j.jeurceramsoc.2019.02.039
Tao, B. J., Xing, C. F., Wang, W. F., Wu, H. T., and Zhou, Y. Y. (2019). A novel Ce2Zr3(MoO4)9 microwave dielectric ceramic with ultra-low firing temperature. Ceram. Int. 45, 24675–24683. doi: 10.1016/j.ceramint.2019.08.206
Wakino, K., Murata, M., and Tamura, H. (1986). Far infrared reflection spectra of Ba(Zn, Ta)O3-BaZrO3 dielectric resonator material, J. Am. Ceram. Soc. 69, 34–37. doi: 10.1111/j.1151-2916.1986.tb04689.x
Wu, M. J., Zhang, Y. C., and Xiang, M. Q. (2019). Synthesis, characterization and dielectric properties of a novel temperature stable (1-x)CoTiNb2O8-xZnNb2O6 ceramic. J. Adv. Ceram. 8, 228–237. doi: 10.1007/s40145-018-0308-y
Wu, Z. J., Meng, Q. B., and Zhang, S. Y. (1998). Semiempirical study on the valences of Cu and bond covalency in Y1−xCaxBa2Cu3O6+y. Phys. Rev. B 58, 958–962. doi: 10.1103/PhysRevB.58.958
Xing, C. F., Wu, B., Bao, J., Wu, H. T., and Zhou, Y. Y. (2019). Crystal structure, infrared spectra and microwave dielectric properties of a novel low-firing Gd2Zr3(MoO4)9 ceramic. Ceram. Int. 45, 22207–22214. doi: 10.1016/j.ceramint.2019.07.243
Xue, D. F., and Zhang, S. Y. (1996). Calculation of the non-linear optical coefficient of the NdAl3(BO3)4 crystal. J. Phys. Condens. Matter 8, 1949–1956. doi: 10.1088/0953-8984/8/12/009
Yang, C. F., Tzou, W. C., Chung, H. H., Diao, C. C., and Huang, C. J. (2009). The microwave dielectric characteristics of Ba(Ti4−xSnx)O9 ceramics. J. Alloy. Compd. 477, 673–676. doi: 10.1016/j.jallcom.2008.10.081
Yang, H. C., Zhang, S. R., Yang, H. Y., Yuan, Y., and Li, E. Z. (2019). Gd2Zr3(MoO4)9 microwave dielectric ceramics with trigonal structure for LTCC application. J. Am. Ceram. Soc. 103, 1131–1139. doi: 10.1111/jace.16744
Yang, J. I., Nahm, S., Choi, C. H., Lee, H. J., and Park, H. M. (2002). Microstructure and microwave dielectric properties of Ba(Zn1/3Ta2/3)O3 ceramics with ZrO2 addition. J. Am. Ceram. Soc. 85, 165–168. doi: 10.1111/j.1151-2916.2002.tb00060.x
Yoon, K. H., Kim, S. K., and Kim, E. S. (2003). Dependence of the octahedral bond valence on microwave dielectric properties of Ca1−xSm2x/3TiO3 ceramics. Mater. Sci. Eng. B Adv. 99, 112–115. doi: 10.1016/S0921-5107(02)00431-2
Yu, H. T., Liu, J. S., Zhang, W. L., and Zhang, S. R. (2015). Ultra-low sintering temperature ceramics for LTCC applications: a review. J. Mater. Sci. Mater. Electron. 12, 9414–9423. doi: 10.1007/s10854-015-3282-y
Zhang, G. Q., Wang, H., Guo, J., He, L., Wei, D. D., and Yuan, Q. B. (2015). Ultra-low sintering temperature microwave dielectric ceramics based on Na2O-MoO3 binary system. J. Am. Ceram. Soc. 98, 528–533. doi: 10.1111/jace.13297
Zhang, Y. H., Sun, J. J., Dai, N., Wu, Z. C., Wu, H. T., and Yang, C. H. (2019). Crystal structure, infrared spectra and microwave dielectric properties of novel extra low-temperature fired Eu2Zr3(MoO4)9 ceramics. J. Eur. Ceram. Soc. 39, 1127–1131. doi: 10.1016/j.jeurceramsoc.2018.12.042
Zhang, Y. H., and Wu, H. T. (2019). Crystal structure and microwave dielectric properties of La2(Zr1−xTix)3(MoO4)9 (0 ≤ x ≤ 0.1) ceramics. J. Am. Ceram. Soc. 102, 4092–4102. doi: 10.1111/jace.16268
Zheng, J. J., Xing, C. F., Yang, Y. K., Li, S. X., Wu, H. T., and Wang, Z. H. (2020). Structure, infrared reflectivity spectra and microwave dielectric properties of a low-firing microwave dielectric ceramic Pr2Zr3(MoO4)9. J. Alloy. Compd. 826:153893. doi: 10.1016/j.jallcom.2020.153893
Keywords: Ce2(Zr0.94Sn0.06)3(MoO4)9, low-temperature sintering, microwave dielectric properties, chemical bonds theory of complex crystals, infrared spectra
Citation: Tian H, Liu X, Yang Y, Wu H and Zhang Z (2020) Crystal Structure, Infrared Spectra, and Microwave Dielectric Properties of Ce2(Zr0.94Sn0.06)3(MoO4)9 Ceramics With Low Sintering Temperature. Front. Mater. 7:145. doi: 10.3389/fmats.2020.00145
Received: 16 February 2020; Accepted: 24 April 2020;
Published: 22 May 2020.
Edited by:
Antonio Feteira, Sheffield Hallam University, United KingdomReviewed by:
Kaixin Song, Hangzhou Dianzi University, ChinaRaz Muhammad, Abdul Wali Khan University Mardan, Pakistan
Hadi Barzegar Bafrooei, Hangzhou Dianzi University, China
Copyright © 2020 Tian, Liu, Yang, Wu and Zhang. This is an open-access article distributed under the terms of the Creative Commons Attribution License (CC BY). The use, distribution or reproduction in other forums is permitted, provided the original author(s) and the copyright owner(s) are credited and that the original publication in this journal is cited, in accordance with accepted academic practice. No use, distribution or reproduction is permitted which does not comply with these terms.
*Correspondence: Haitao Wu, mse_wuht@ujn.edu.cn; Zhiliang Zhang, zhzhl@iccas.ac.cn