- 1Institute of Metal Research, Chinese Academy of Sciences, Shenyang, China
- 2School of Materials Science and Engineering, University of Science and Technology of China, Shenyang, China
In this work, the microbiologically influenced corrosion (MIC) behaviors of welded 304L stainless steel (304L SS) and Cu-bearing 304L SS (304L-Cu SS) in a Pseudomonas aeruginosa culture medium were comparatively studied. Open circuit potential (OCP), linear polarization resistance (LPR), electrochemical impedance spectroscopy (EIS), and potentiodynamic polarization were employed to characterize the corrosion behavior of the (welded) steel substrates. The biofilm morphology and the live/dead staining condition of the sessile cells were observed by scanning electron microscopy (SEM) and confocal laser scanning microscopy (CLSM). And the pitting corrosion was investigated with a 3D mode of CLSM. The experimental results showed that the copper (Cu) addition had no obvious influence on the microstructure of 304L SS in welding zones (WZs) and base metal. The WZs of 304L SS and 304L-Cu SS in sodium chloride solution possessed a lower pitting potential and a higher corrosion current density. However, owing to the continuous release of Cu ions, inhibiting the excretion of corrosive extracellular polymeric substances (EPSs) and the extracellular electron transport (EET) process, both the localized and uniform corrosion attack induced by the P. aeruginosa biofilm was significantly reduced. In comparison with that of 304L SS, the corrosion resistance of 304L-Cu SS in the WZ against P. aeruginosa was obviously higher.
Introduction
The 304L stainless steel (SS) has been widely used in many fields such as food industry, medical device, transportation, and daily appliance, owing to its excellent combination of mechanical properties, corrosion resistance, and workability (Nie et al., 2011; Zhang et al., 2013). In some of those application conditions, the steel needs to be welded before use. Nevertheless, welding joints of steels are usually recognized as sensitive zones to corrosion. This is because the welding zone (WZ) of austenitic SSs has a microstructure of a dendritic austenite matrix with a small amount of δ-ferrite (Dadfar et al., 2007; Moon et al., 2011). And the formation of δ-ferrite under the pseudo-equilibrium condition in austenitic SSs may cause Cr-depleted zones and lead to localized corrosion (Lu et al., 2005). More seriously, the corrosion that occurred in the sensitive zones could be severely aggravated by corrosive microorganisms in service environments, which is named as microbiologically influenced corrosion (MIC; Neria-Gonzalez et al., 2006; Aktas et al., 2010; Huttunen-Saarivirta et al., 2012). According to the literatures, MIC accounts for 20% of all corrosion damages (Zhang et al., 2015; Xu et al., 2017a; Hashemi et al., 2018; Li et al., 2018). The formation of corrosive biofilm on metal surface changes the electrochemical conditions and thus accelerates both uniform and pitting corrosions (Vigneron et al., 2016; Skovhus et al., 2017).
As a new kind of structural and functional integrated metal material, the Cu-bearing SSs have been developed and attracted great attention in recent years (Jin et al., 2016; Jiang et al., 2016; Xi et al., 2016, 2017). By adding a proper amount of copper (Cu) into a steel matrix, trace amount of Cu ions could be continuously released in application environment, thus offering an antibacterial function for the SSs (Nan and Yang, 2010). Previous works have confirmed that the Cu-bearing SSs possessed excellent antibacterial property against Porphyromonas gingivalis, Staphylococcus aureus, and so forth (Zhang et al., 2013). It was reported that Cu-bearing SSs also showed a significant beneficial effect on MIC. Nan et al. (2015) confirmed that Cu-bearing 304L SS (304L-Cu SS) had higher resistance against MIC. Therefore, applying Cu-bearing SS to replace the commonly used SS could be a feasible solution to inhibit the MIC attack in some harsh corrosive environments (Li et al., 2015).
Pseudomonas aeruginosa, the aerobic Gram-negative bacterium, is a kind of typical corrosive bacteria, which are widely distributed in various types of water environments (Hamzah et al., 2013). In recent years, many researches proved that P. aeruginosa can induce the pitting corrosion of carbon steels and SSs (Hamzah et al., 2013; Wadood et al., 2017). Although there were some studies related to the influence of Cu-bearing SSs on MIC behaviors, a comparison between a steel matrix and WZ of Cu-bearing SS and the relevant effect on the MIC behavior are still lacking of enough understanding. Hence, the purpose of this work is to comparatively investigate the difference on corrosion behavior between the WZs and a matrix of 304L SS and 304L-Cu SS in a P. aeruginosa culture medium by means of linear polarization, electrochemical impedance spectroscopy (EIS), potentiodynamic polarization, and confocal laser scanning microscopy (CLSM).
Materials and Methods
Materials
In this work, 304L SS and 304L-Cu SS were melted in a 25-kg vacuum smelting furnace. The ingots were hot rolled into plate shape with a thickness of 4 mm and then finally cold rolled into 1-mm-thick sheets. Chemical compositions of the 304L SS and 304L-Cu SS are listed in Table 1. Both 304L SS and 304L-Cu SS were solution treated at 1,050°C for 0.5 h and followed by quenching in water. Afterward, a tungsten inert gas (TIG) welding was employed to weld the SS sheets together. Selected welding parameters were 93 A of welding current and 52 cm/min of welding speed. The WZs and base metal (BM) specimens of 304L SS and 304L-Cu SS were separately cut off and then machined into square coupons with dimensions of 10 mm × 10 mm × 1 mm. Among them, the coupons for making electrodes were sealed with epoxy resin and silica gel, leaving an exposed working area of 1 cm2. Each working surface for electrochemical measurements and immersion tests was polished to a 1,000-grit finish with metallographic sandpaper, then cleaned with absolute ethyl alcohol, and finally dried in hot air stream. The 75% (v/v) alcohol and ultraviolet (UV) light were used to sanitize the specimens and electrodes just before the experiment. The surfaces for microstructure analysis were polished to a mirror finish and electro-etched at a 2 V of direct current in 60% (v/v) nitric acid solution for 15 s and then observed by the metallurgical microscope (Z1 M, Zeiss, Germany).
Culture Medium and Inoculum
Pseudomonas aeruginosa (1A00099) was purchased from the Marine Culture Collection of China (MCCC) and cultured in the 2216E medium in this work. The 2216E culture medium was composed of following compounds: 3.24 g/L of Na2SO4, 1.8 g/L of CaCl2, 0.55 g/L of KCl, 0.16 g/L of Na2CO3, 0.08 g/L of SrBr2, 0.022 g/L of H3BO3, 0.004 g/L of NaSiO3, 0.0024 g/L of NaF, 0.0016 g/L of NH4NO3, 0.008 g/L of NaH2PO4, 5.0 g/L of peptone, 1.0 g/L of yeast extract, and 0.1 g/L of ferric citrate. The pH value of 2216E was adjusted to 7.2 ± 0.2. The cultural temperature was controlled at 37°C during the testing period, which was favorable to P. aeruginosa growth.
Electrochemical Measurements
To compare the corrosion resistance difference of 304L SS BM, 304L SS WZ, 304L-Cu SS BM, and 304L-Cu SS WZ, the polarization curves were measured with a three-electrode system in 3.5 wt.% of NaCl solution by using the electrochemical workstations (Reference 1000TM, Gamry Instruments, United States). The measured specimen was the working electrode in a three-electrode system; a saturated calomel electrode (SCE) and a platinum electrode were used as the reference electrode and the counter electrode, respectively. Prior to polarization curve measurements, the open circuit potential (OCP) was monitored at least 30 min until its value was relatively stable. In addition, linear polarization resistance (LPR) and EIS were chosen to monitor the change of corrosion resistance for different electrodes during the 14 days of testing period in the culture medium with presence of P. aeruginosa.
Linear polarization resistance measurements were conducted at a scan rate of 0.125 mV/s within the range of ± 5 mV versus EOCP. The EIS measurements were performed at a sinusoidal voltage signal of 10 mV in the frequency range from 105 to 10–2 Hz. And the impedance results were fitted by using ZSimpWin software with two reasonable equivalent circuit models. After 14 days, potentiodynamic polarization measurements were scanned from −250 to 600 mV versus EOCP (the maximum current limit was 1 mA/cm2) at a potential sweep rate of 0.5 mV/s (Li et al., 2016, 2017; Xu et al., 2017b; Liu et al., 2018a, b; Sun et al., 2019).
Surface and Biofilm Analysis
After the immersion tests, a field emission scanning electron microscopy (FE-SEM, Ultra-plus, Zeiss, Germany) was used to observe the P. aeruginosa biofilms formed on the surface of specimens. Those biofilms were fixed by using 2.5% (v/v) glutaraldehyde solution for 8 h and dehydrated by ethanol–water solutions successively with the concentrations of 30, 40, 50, 60, 70, 80, 90, 95, and 100 and then sputter-coated with gold to improve conductivity of the observing surfaces.
A CLSM (Model C2 Plus, Nikon, Japan) was used to further characterize the survival condition of P. aeruginosa cells in biofilm. Before the observation, all the surfaces were stained using following steps: firstly, the surfaces were cleaned with phosphate-buffered saline (PBS) to remove scum and planktonic cells and then stained using SYTO-9 and propidium iodide (PI; Invitrogen, Eugene, OR, United States). The live cells can be stained with SYTO-9, whereas the dead cells can be stained with PI. All the live cells can be observed as green dots under CLSM with the wavelength of 488 nm, and the red dots with the wavelength of 559 nm represent the dead cells.
After the biofilms and corrosion products were removed (Li et al., 2016), the corrosion pitting induced by P. aeruginosa was observed with CLSM (LSM 710, Zeiss, Germany). The depth of pits was measured with the 3D mode of CLSM.
Results
Microstructure Observation and Corrosion Resistance
Figure 1 shows the microstructures of the whole welding joint, BM, and WZ of 304L SS and 304L-Cu SS. As displayed in Figure 1, no significant difference was observed between 304L SS and 304L-Cu SS. The microstructures of BM for 304L SS and 304L-Cu SS consisted of fully austenite grains, with the grain size of about ∼35 μm, whereas the WZ microstructures were composed of a dendritic austenite matrix decorated with a small number of δ-ferrite (Pouranvari et al., 2015).
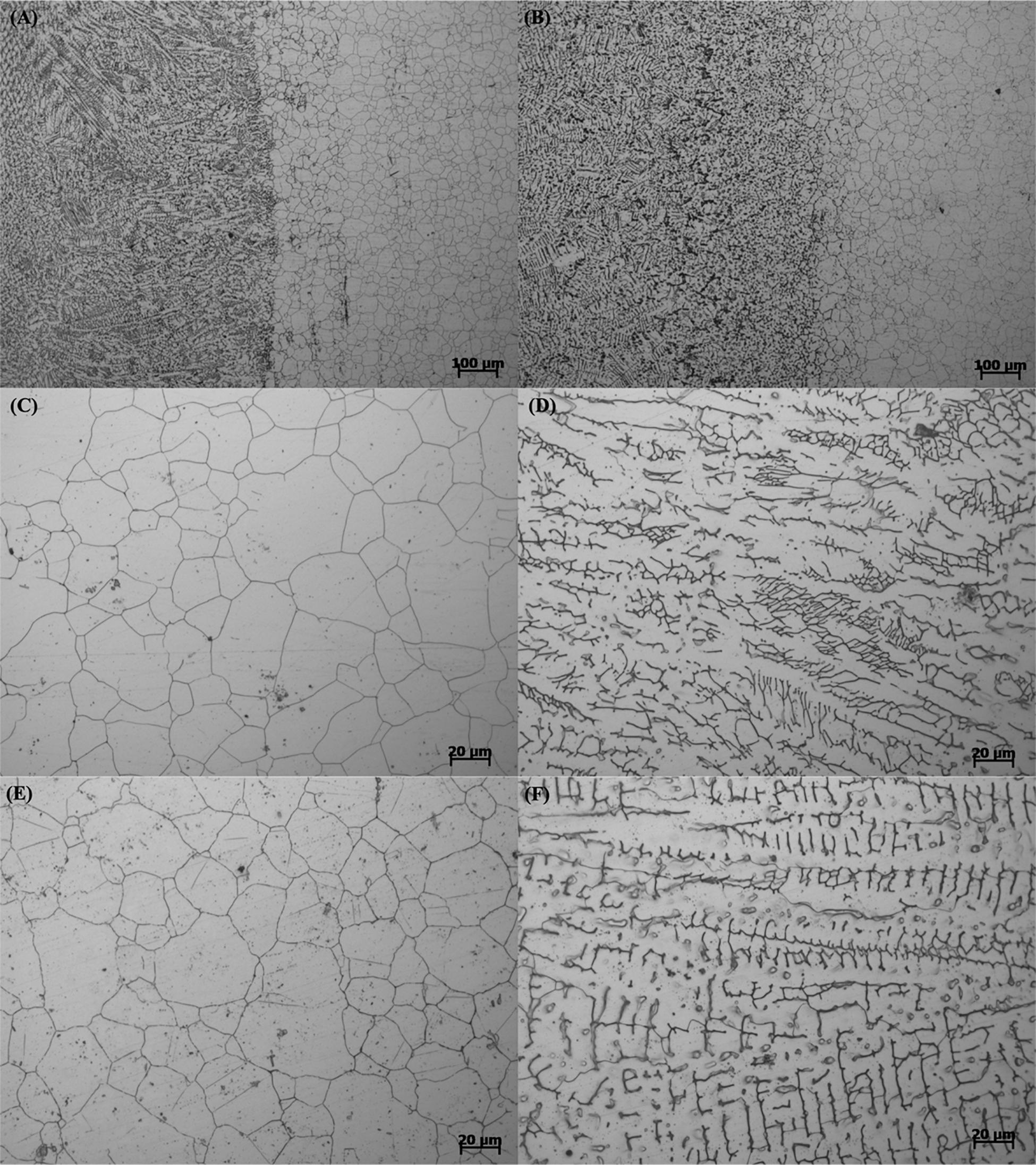
Figure 1. Metallographic microstructure images of (A) welding line of 304L, (B) welding line of 304L-Cu, (C) 304L BM, (D) 304L WZ, (E) 304L-Cu BM, and (F) 304L-Cu WZ. BM, base metal; WZ, welding zone.
Figure 2 shows the potentiodynamic polarization curves of BM and WZ from 304L SS and 304L-Cu SS in 3.5% NaCl solution. The values of corrosion potential (Ecorr), pitting potential (Epit), corrosion current density (Icorr), and passivation current density (Ipass) were derived and listed in Table 2. It is obvious that Cu addition led to a negative shift of Ecorr and a slightly decrease of Icorr from 55.2 nA/cm2 of 304L BM to 41.3 nA/cm2 of 304L-Cu BM. However, the Epit of 304L-Cu BM was much lower than that of 304L BM with values of 246.6 and 393.5 mV, respectively. Meanwhile the Ipass value was increased from 285.8 nA/cm2 of 304L BM to 338.1 nA/cm2 of 304L-Cu BM. 304L-Cu WZ had the lowest Epit value and the highest Ipass value among all electrodes, which were 210.3 mV/SCE and 358.9 nA/cm2, respectively. Of note, the increased Ipass and Icorr and reduced Epit values of 304L WZ and 304L-Cu WZ indicated the lower corrosion resistance than that of BM specimens.
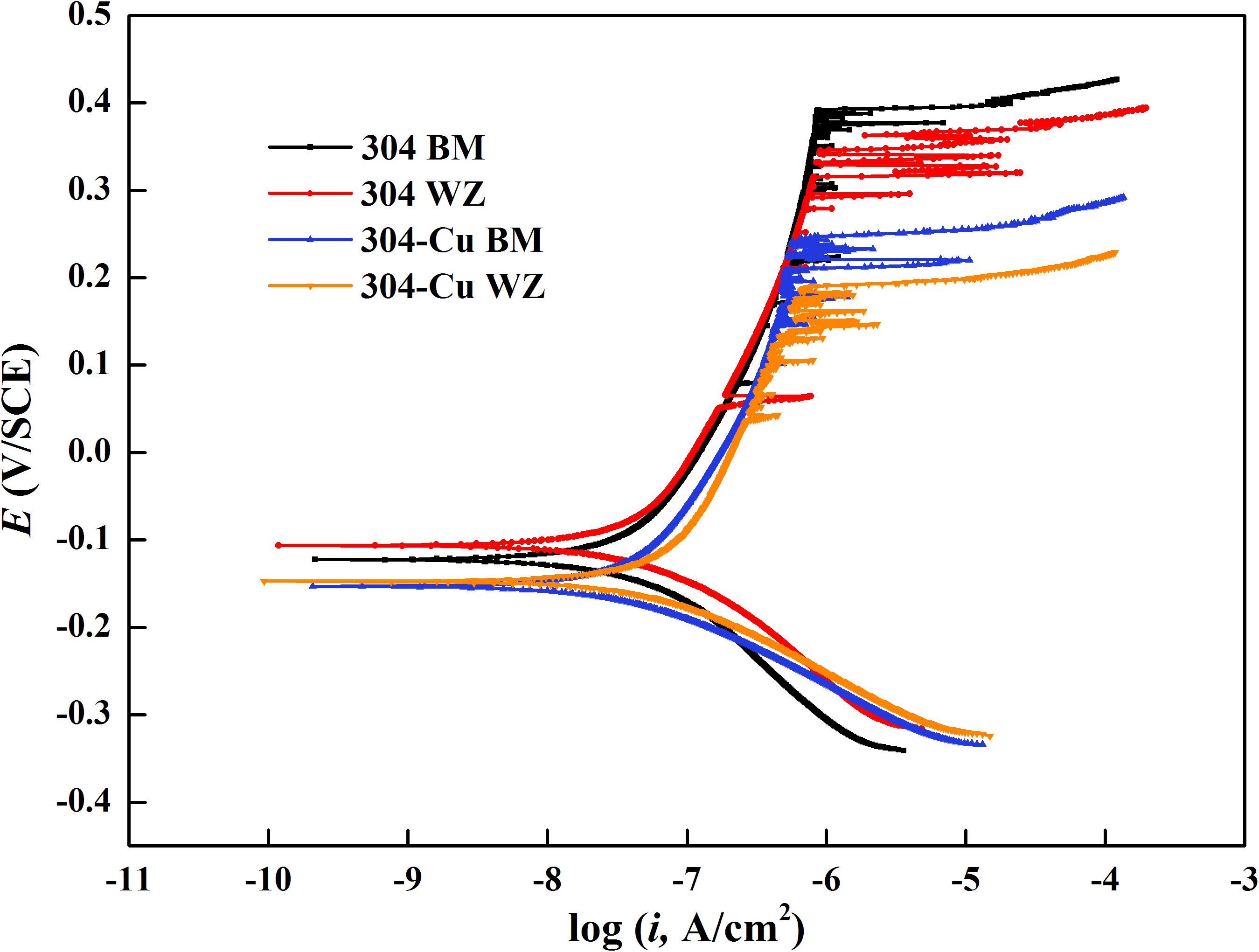
Figure 2. Potentiodynamic polarization curves of base metals and welding zones of 304L and 304L-Cu SS in 3.5% NaCl solution. SS, stainless steel.
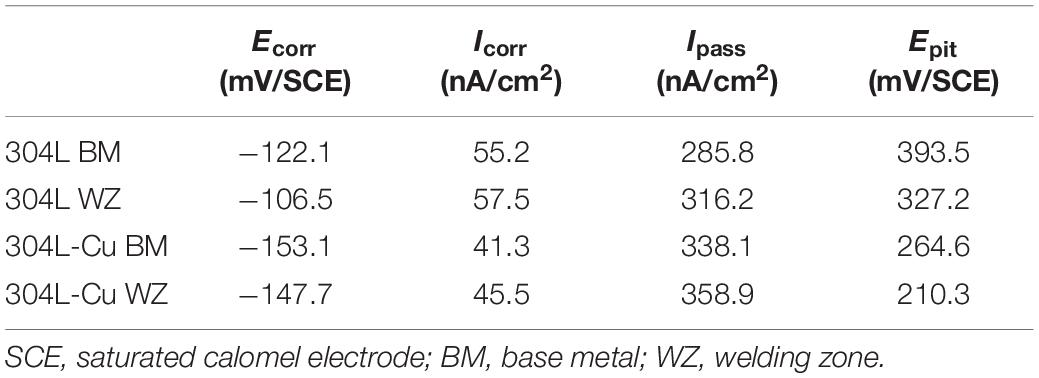
Table 2. Fitting results derived from the potentiodynamic polarization curves in Figure 2.
Surface and Biofilm Analysis
SEM images shown in Figure 3 depict the morphology of the P. aeruginosa biofilms on different specimen surfaces after 14 days of immersion in culture medium. For both 304L SS BM and 304L SS WZ (shown in Figures 3A,B, respectively), the amounts of sessile P. aeruginosa cells on their surfaces were much larger than those on Cu-bearing specimen surfaces (shown in Figures 3C,D). And the P. aeruginosa biofilms formed on 304L SS BM and 304L SS WZ were obviously more compact and concentrated compared with those on Cu-bearing BM and WZ specimens.
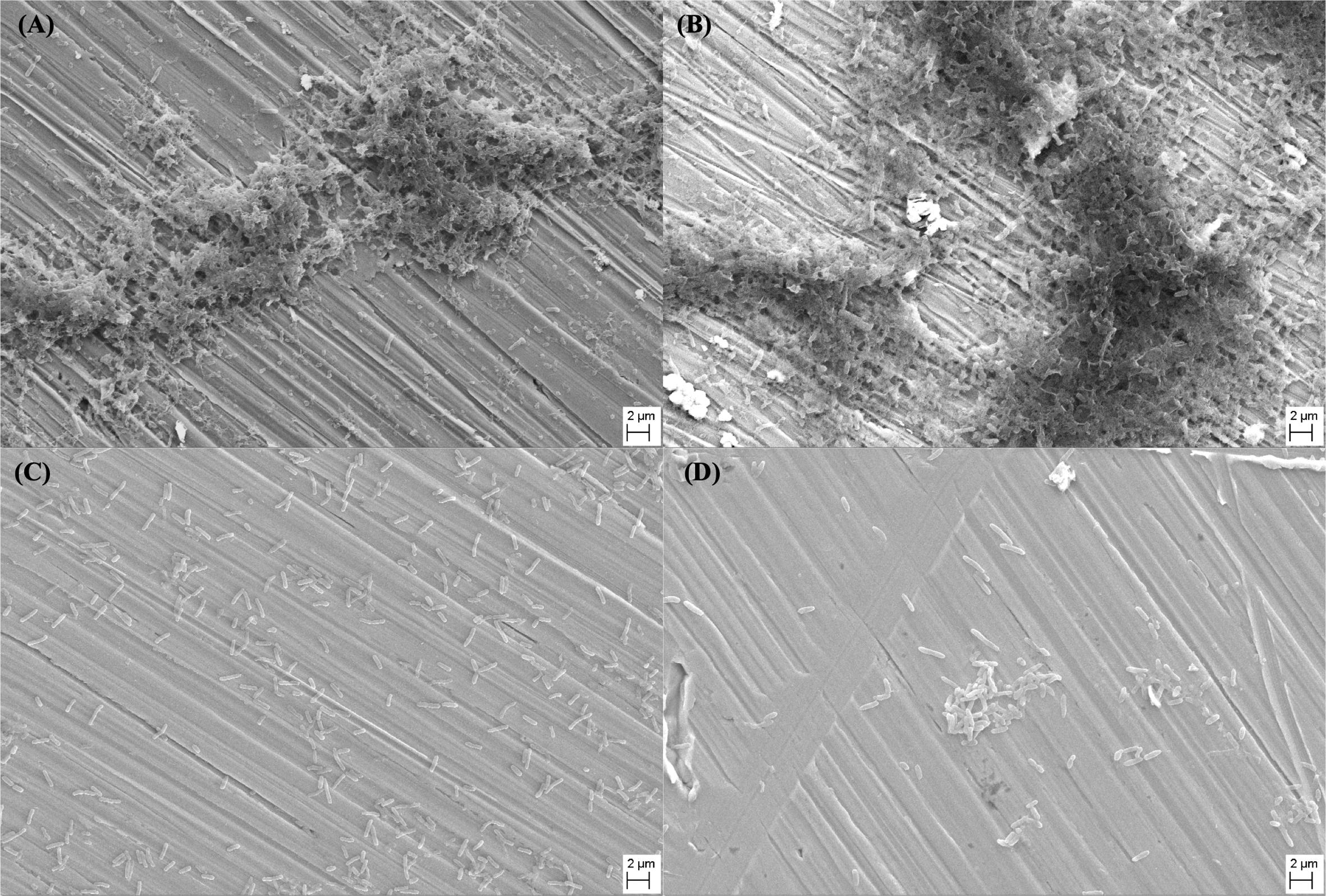
Figure 3. SEM images of Pseudomonas aeruginosa biofilms that formed on the surfaces of (A) 304L BM, (B) 304L WZ, (C) 304L-Cu BM, and (D) 304L-Cu WZ specimens after 14 days of immersion test. BM, base metal; WZ, welding zone.
To further detect the activation of the sessile P. aeruginosa cells, the biofilms were stained with SYTO-9 and PI and then observed under CLSM at wavelengths of 488 and 559 nm, respectively. The live/dead staining images are shown in Figure 4. It is obvious that almost all sessile P. aeruginosa cells on the surfaces of 304L BM and 304L WZ (shown in Figures 4A,B) were alive. However, plenty of dead cells appeared on the Cu-bearing specimen surfaces (shown in Figures 4C,D). In addition, the distribution of attached cells on each specimen imaged by CLSM consisted with that observed by SEM.
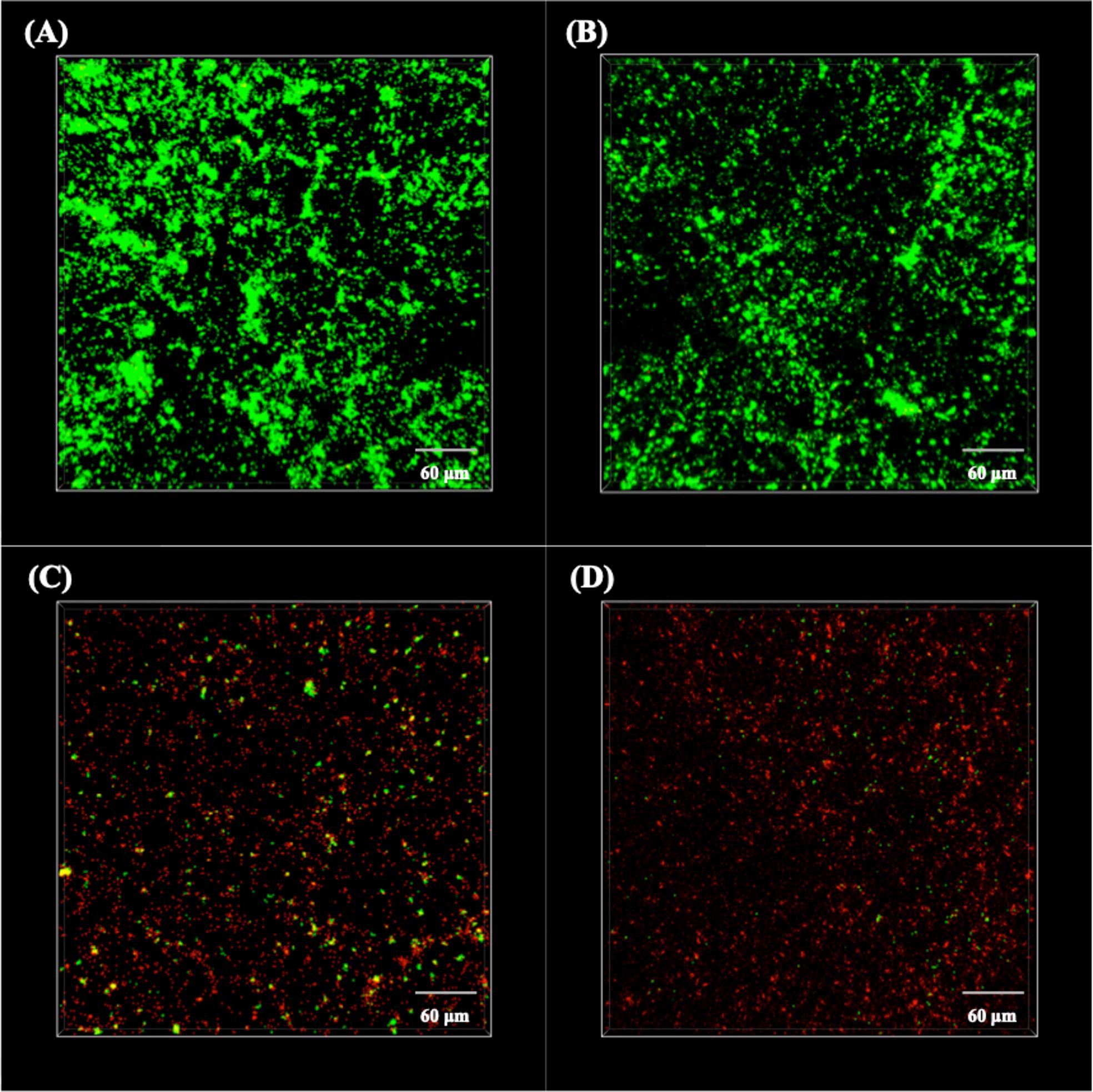
Figure 4. CLSM images of live/dead stained Pseudomonas aeruginosa biofilms on the surfaces of (A) 304L BM, (B) 304L WZ, (C) 304L-Cu BM, and (D) 304L-Cu WZ specimens after 14 days of immersion test. CLSM, confocal laser scanning microscopy; BM, base metal; WZ, welding zone.
Figure 5 shows the analysis results of the maximum pit depth with the CLSM in 3D mode. As shown in Figure 5B, the most severe pitting corrosion was observed on 304L WZ specimen, with maximum pit of 5.1 μm in depth and 12.3 μm in width. The maximum depth of pit found on the surface of 304L BM reached 4.9 μm, which was deeper than that of Cu-bearing specimens. The most serious pits found on 304L-Cu BM and 304L-Cu WZ were 2.7 and 2.9 μm in depth, respectively.
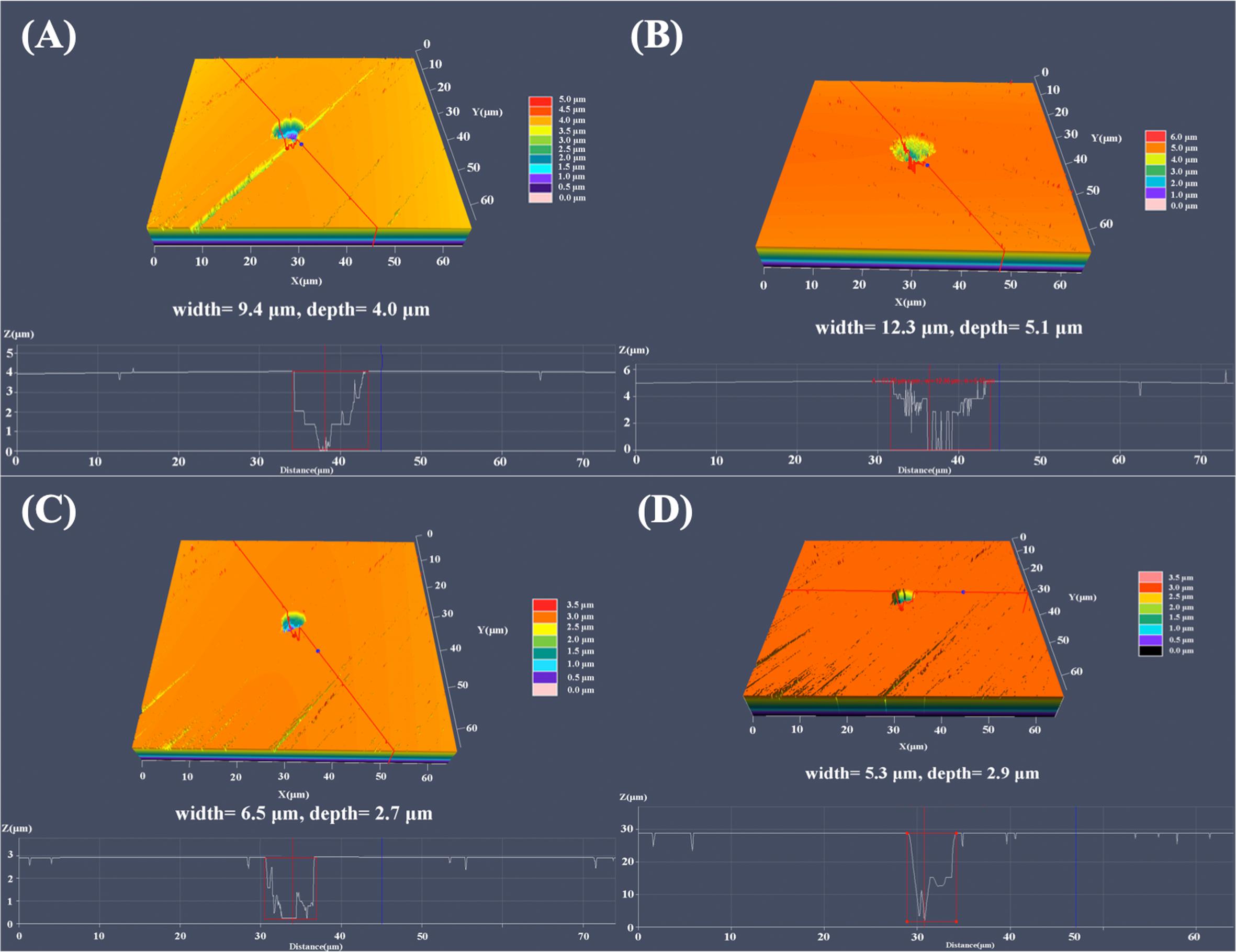
Figure 5. Largest pit depth measured by CLSM of (A) 304L BM, (B) 304L WZ, (C) 304L-Cu BM, and (D) 304L-Cu WZ in the Pseudomonas aeruginosa-inoculated culture medium after 14 days. CLSM, confocal laser scanning microscopy; BM, base metal; WZ, welding zone.
Electrochemical Measurements
Figure 6A shows the EOCP curves of four types of electrodes varying with immersion time in P. aeruginosa culture medium. The variation tendency of each curve reveals that the most significant changes in EOCP values occurred in the initial stage of the immersion test. The initial EOCP values of 304L BM and 304L WZ were higher than those of the Cu-bearing electrodes, which were −209.1 and −168.5 mV/SCE, respectively. However, after the sharp decline stage, the values of 304L BM and 304L WZ peaked around −470.1 and −474.8 mV/SCE at about 7 days and afterward maintained a relatively slow decline. After 14 days of immersion, the EOCP of 304L BM and 304L WZ was similar, which was −504.0 and −508.4 mV/SCE, respectively. Compared with those of 304L SS, the EOCP changes of Cu-bearing electrodes were smaller in the sharp decline stage. The initial values for EOCP of 304L-Cu BM and 304L-Cu WZ were −284.9 and −250.3 mV/SCE, respectively. But the final EOCP value of 304L-Cu BM at the 14 days was the highest among the four electrodes, which was −417.5 mV/SCE. Even the EOCP of 304L-Cu WZ was still higher than that of 304L-Cu BM and 304L-Cu WZ after 14 days of immersion.
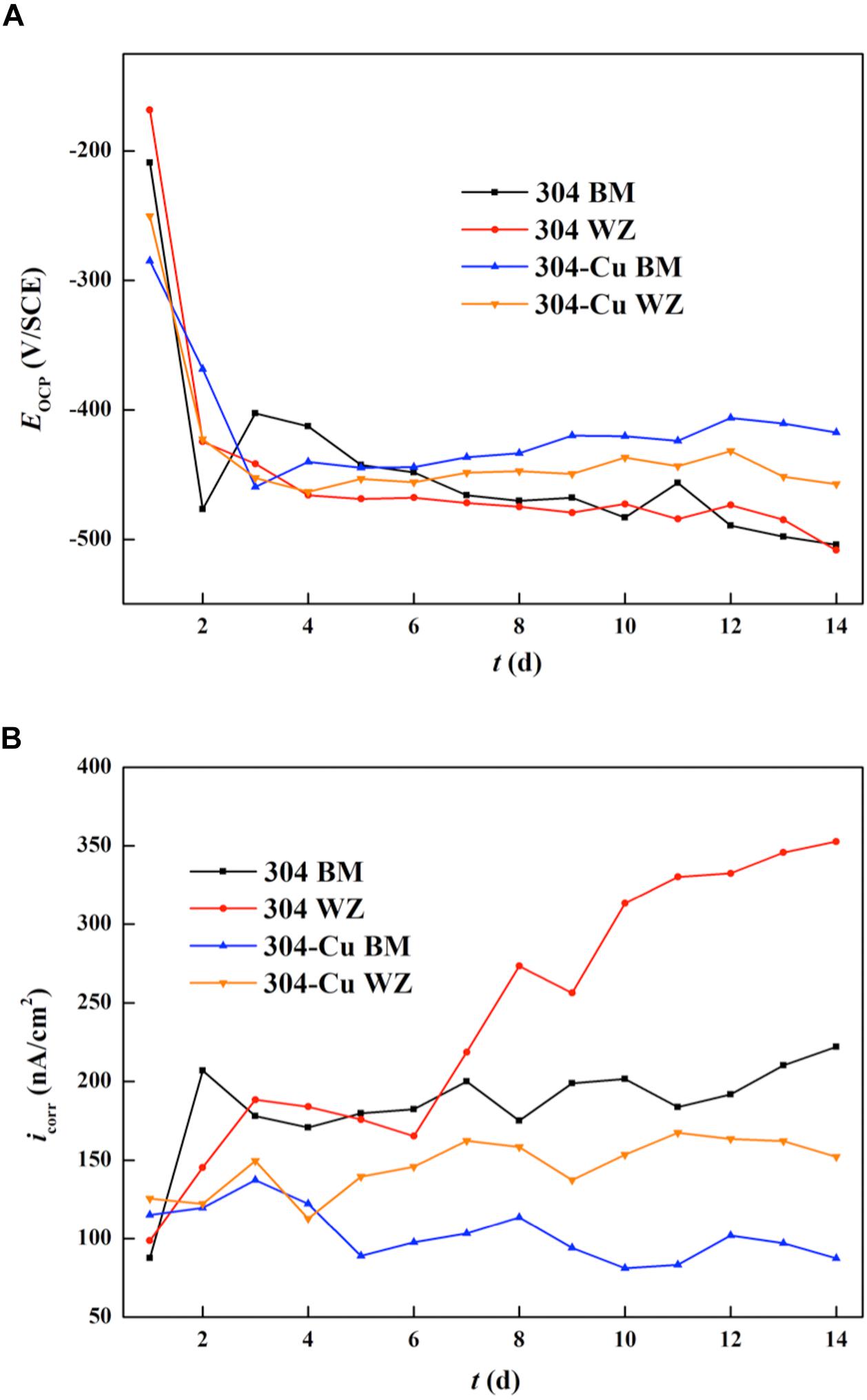
Figure 6. Changing curves of (A) OCP and (B) LPR values for various electrodes during 14 days. OCP, open circuit potential; LPR, linear polarization resistance.
Figure 6B shows the variation of Icorr values (measured by LPR tests) as a function of immersion time. The Icorr can directly reflect the uniform corrosion rate of the electrode. The initial Icorr values of 304L BM, 304L WZ, 304L-Cu BM, and 304L-Cu WZ were 87.6, 98.7, 115.2, and 125.5 nA/cm2, respectively. It indicated that the corrosion resistance at the incomplete formation stage of P. aeruginosa biofilm for each electrode was consistent with the measuring results in 3.5% NaCl solution. With the biofilm growth on the surface of electrodes, the Icorr values of 304L BM and 304L WZ electrodes experienced a continuous increase during the whole immersion period owing to the formation of P. aeruginosa. But for 304L-Cu BM and 304L-Cu WZ electrodes, the Icorr remained unchanged at later immersion time. At 14 days, the Icorr values of 304L BM, 304L WZ, 304L-Cu BM, and 304L-Cu WZ electrodes became 222.0, 352.7, 87.3, and 152.1 nA/cm2, respectively. 304L WZ showed the worst corrosion resistance among of all electrodes owing to the influence of P. aeruginosa biofilm. Obviously, 304L-Cu WZ and 304L-Cu BM possessed the better corrosion resistance than 304 WZ and 304 BM.
Figure 7 shows the Nyquist and Bode plots of four types of experimental electrodes in the P. aeruginosa culture medium. It can be found that the measured impedance plots were different, depending on the exposure time and the Cu addition. To compare the detailed impedance parameters, the EIS data were fitted with appropriate equivalent circuits as shown in Figure 8, where Rs represents the culture medium resistance; Rf and Qf account for the resistance and capacitance of the surface film, respectively; and Rct and Qdl denote the electron transfer resistance of passivation film and double layer capacitance, respectively. In this work, the capacitance C was replaced by the constant phase element (CPE, Q) because of the heterogeneity of the experimental electrode upon corrosion in the culture medium; thus, the ideal capacitance element was not good for fitting the corroded SS electrode (Liu et al., 2017). The expression for the CPE is
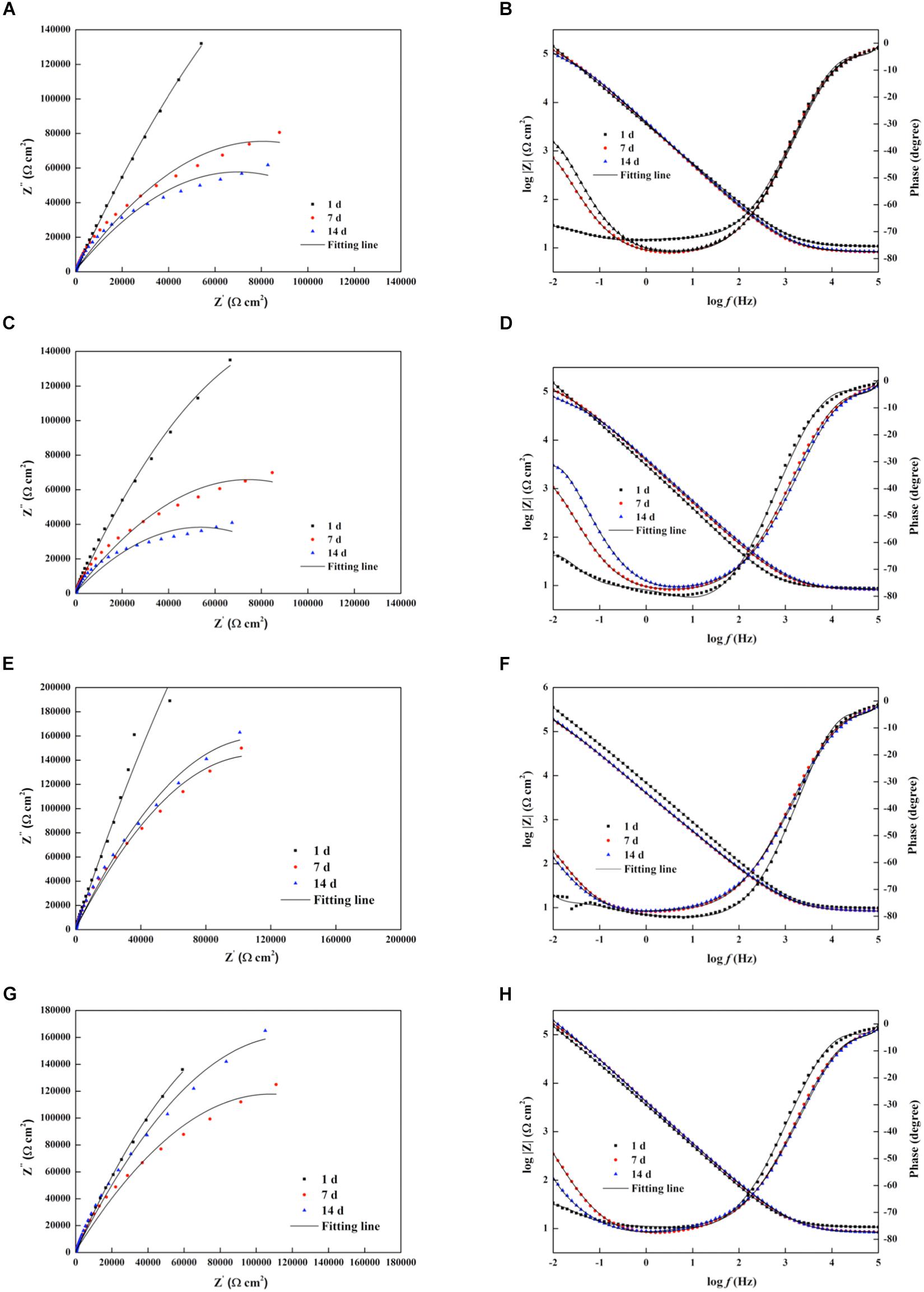
Figure 7. Nyquist and Bode diagrams of the impedance measurements of different electrodes in the Pseudomonas aeruginosa-inoculated culture medium as a function of time. (A,B) 304L BM, (C,D) 304L WZ, (E,F) 304L-Cu BM, and (G,H) 304L-Cu WZ. The black solid lines are fitting impedance curves with proper equivalent circuits in Figure 8. BM, base metal; WZ, welding zone.
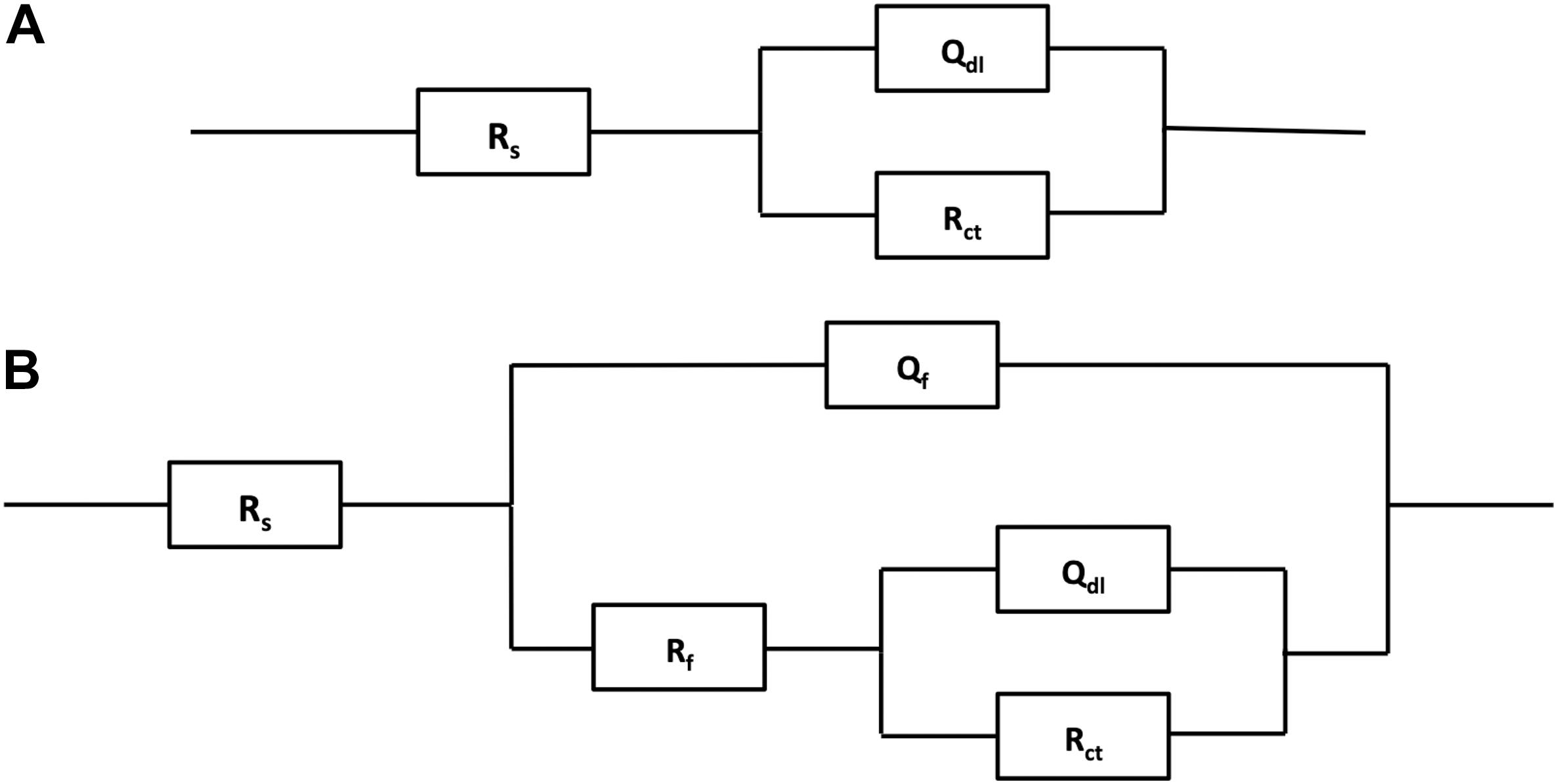
Figure 8. Electrochemical equivalent circuits for fitting the measured impedance data in Figure 7.
where ω is the angular frequency (rad/s) and Y0 and α are the coefficients reflecting the deviation of electrode from the ideal reaction model. Based on the shape of Bode plots and the images of SEM observation, the data of all electrodes at the first day were fitted with the equivalent circuit shown in Figure 8A, whereas other data were fitted with the equivalent circuit in Figure 8B. In the fitting process, all χ2 values were lower than 10–4, which means that fitting error was small. The black solid fitting lines in Nyquist and Bode also show the high coincidence degree. Through the diameter change of capacitance loops and the fitted values of Rf and Rct, the corrosion resistance of each electrode during testing period can be evaluated. The initial fitted Rct values of 304L BM, 304L WZ, 304L-Cu BM, and 304L-Cu WZ were 295.5, 262.6, 226.1, and 206.3 kΩ/cm2, respectively. At the 14 days, the sum of fitted Rf and Rct of 304L BM, 304L WZ, 304L-Cu BM, and 304L-Cu WZ changed to 117.2, 73.7, 218.6, and 176.4 kΩ/cm2, respectively. The tendency and conclusion were consistent with the LPR results.
Figure 9 shows the potentiodynamic polarization curves of the four types of electrodes after 14 days of immersion in the 2216E culture medium inoculated with P. aeruginosa. It can be seen that each electrode was still kept in the passivation state after immersion in this corrosive environment. 304L WZ had the largest corrosion Icorr value (877.0 nA/cm2) and the lowest Epit value (212.8 mV/SCE). As the Epit value reflects the resistance to pitting corrosion and the Icorr value indicates the stability of passive film on the surface of SS, the two Cu-bearing electrodes showed better pitting corrosion and uniform corrosion resistance against P. aeruginosa. 304L-Cu BM showed the highest Epit value (398.1 mV/SCE) among four electrodes. The Icorr values of 304L-Cu WZ and 304L BM were close, which were 305.9 and 399.0 nA/cm2, respectively. However, the Epit of 304L-Cu WZ (346.2 mV/SCE) was higher than that of 304L BM (302.0 mV/SCE). The Icorr and Epit values for 304L SS WZ were 877.0 and 212.8 mV/SCE, respectively, indicating the worst resistance to both pitting and uniform corrosion attack.
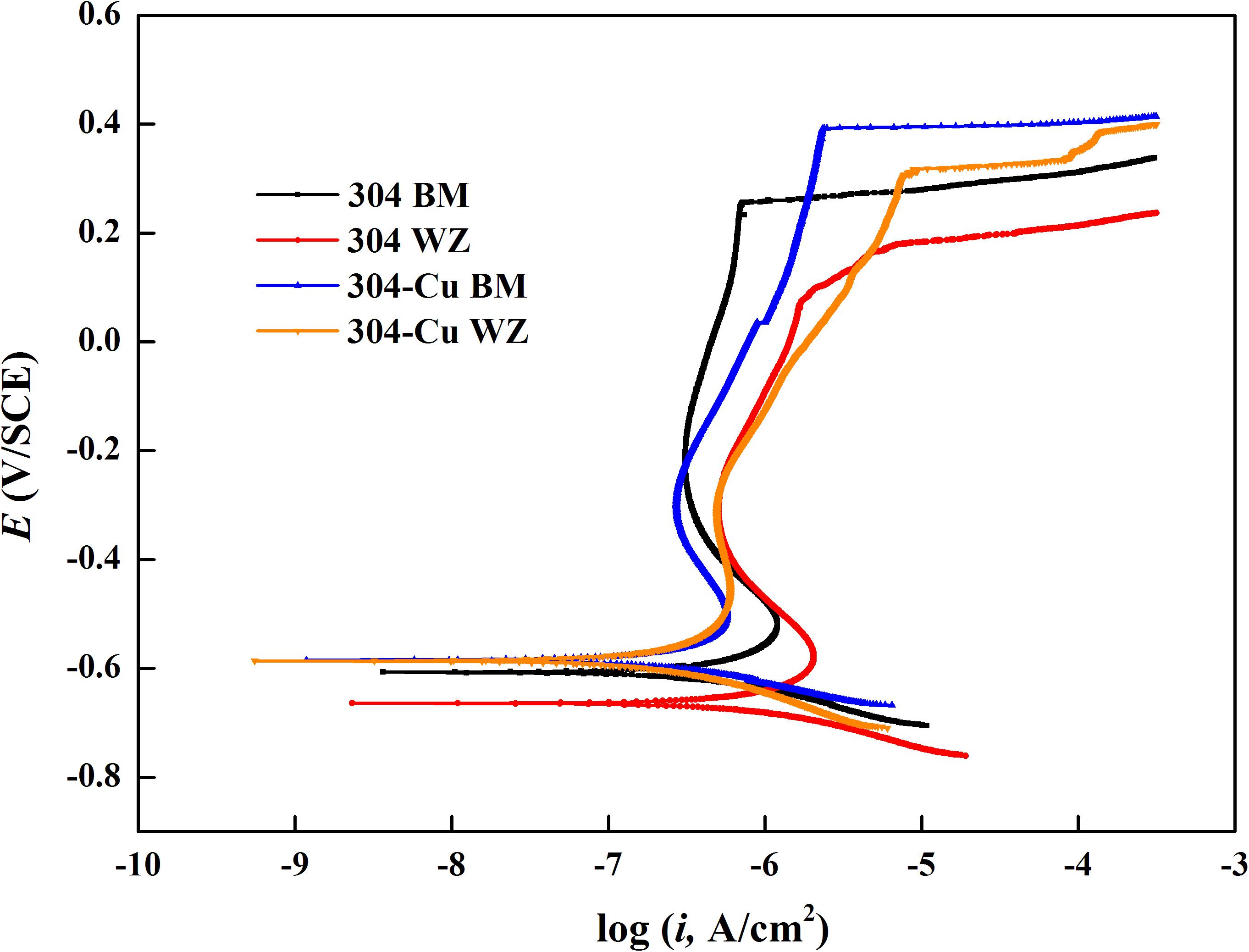
Figure 9. Potentiodynamic polarization curves of various 304L SS electrodes after 14 days of immersion in the 2216E culture medium with Pseudomonas aeruginosa. SS, stainless steel.
Discussion
Effects of Cu Addition and Tungsten Inert Gas Welding on Corrosion Resistance of 304L SS
The effects of microstructural changes in austenitic 304L SS by the processes of TIG welding and Cu addition on the corrosion behaviors are presented in Figure 2 and Table 2. Under the testing condition (3.5% NaCl), the high concentration of chloride ions was the main cause for the corrosion (Lu et al., 2005). According to the difference between 304L BM and 304L-Cu BM, the Cu addition slightly improved the uniform corrosion resistance of the austenitic SS, which was proved by decrease of the Icorr value. The previous works reported that the moderate amount of Cu addition in SSs could improve the corrosion resistance (Ishu et al., 2011). The dissolved copper ions could react with the chloride ions in solution on the material surface, and their reaction product CuCl2 could work as a protective film. However, at the high potential region, the Epit of 304L BM was appreciably reduced by Cu addition, and the Ipass was also increased, implying that Cu addition might weaken the compactness of passive film and then reduce the pitting resistance of 304L BM in the solution with a high concentration of chloride ions (Gui et al., 2016).
According to Figure 2 and Table 2, the 304L WZ possessed lower pitting resistance and uniform corrosion resistance with higher Ipass and lower Epit than did the 304L BM. Similarly, the corrosion resistance of 304L-Cu WZ was worse than that of 304L-Cu BM. That indicated that the TIG welding process decreases the anticorrosive property and that WZ is prone to the occurrence of the pitting corrosion. It is mainly due to the overquick cooling process after welding, the diffusion of the alloying elements was suppressed, and therefore those elements were nonuniformly distributed in WZs (Dadfar et al., 2007). Besides, in comparison with BM, WZ has more negative Ecorr value. Thus, when BM and WZ were adjacently placed together in a corrosive environment, the WZ (with the welding parameters as mentioned above) served as a corrosive weakness and decreases the service safety.
Resistance of Cu-Bearing Stainless Steel Against Pseudomonas aeruginosa-Induced Corrosion
As shown in Figure 6A, the sharp decline of each OCP curves in the initial stage may be induced by the formation of P. aeruginosa biofilm, which changed the interface state between the corrosive environment and the passive film of electrodes. It should be noted that the rising tendency of OCP curves was observed in all Icorr curves even in the initial stage. Those changes were attributed to the acceleration of anodic reaction induced by P. aeruginosa sessile cells. According to the researches on the corrosion mechanism induced by P. aeruginosa, the formation of biofilm on the metal surface is crucial to the biocorrosion. In the process of biofilm formation and growth, the extracellular polymeric substances (EPSs) secreted by sessile cells are essential (Pillay and Lin, 2013). Pyocyanin is one of the EPSs of P. aeruginosa, which can serve as the electron carrier and participate in the extracellular electron transport (EET) mechanism (Rabaey et al., 2004). In EET-MIC, the sessile cells in biofilms use the energetic metal (e.g., Fe) as the electron donor and the non-oxygen oxidant as the electron acceptor. However, the reduction of this oxidant has occurred inside the sessile cells’ cytoplasm because the reaction requires biocatalysts by intracellular enzymes (Jia et al., 2019). Owing to the iron oxidation that occurs extracellularly, the electron carrier (pyocyanin for P. aeruginosa) is needed to transport extracellular electrons to the cytoplasm for the reduction reaction. As a result, the whole process of EET leads to the depolarization and seriously accelerates the corrosion rate. The impedance plots in Figure 7 also confirm that the reaction on steel electrodes was accelerated owing to the existence of the P. aeruginosa biofilm.
However, the Cu-bearing specimens have strong antibacterial property. As shown in Figures 3, 4, the Cu-bearing specimens significantly inhibited the P. aeruginosa biofilm formation and killed most of sessile cells. The excellent antibacterial property of 304L-Cu BM and 304L-Cu WZ is due to the release of Cu+ and Cu2+ ions during corrosion process. Both Cu+ and Cu2+ ions are highly toxic to P. aeruginosa cells. The main bactericidal mechanism of Cu+ and Cu2+ is the adherence to the cytomembrane and cytoderm of bacteria and the damage of the intracellular protein of bacterial cells (Elguindi et al., 2011; Santo et al., 2011; Zhang et al., 2020).
Because of the protective effect of copper ions, both 304L-Cu BM and 304L-Cu WZ electrodes show a much better anticorrosive property than 304L BM and 304L WZ in the P. aeruginosa culture medium. This protection mechanism also works on the localized corrosion attack induced by P. aeruginosa biofilm. Previous research revealed that the non-uniform distributed P. aeruginosa biofilm can locally decrease the pH value at the interface, which is one of the main reasons to cause pitting corrosion (Hamzah et al., 2013; Li et al., 2016). As the biofilm cannot grow well on the surface of 304L-Cu BM and 304L-Cu WZ specimens, these Cu-bearing specimens had higher Epit values and smaller pit depths, as shown in Figures 5, 9. The results in Figure 2 also indicated that the Cu addition causes a remarkable inhibition effect on the MIC-induced pitting corrosion. Besides, the pits’ shape (shown in Figure 5) has little difference between 304L SS and 304L-Cu SS specimens. It can be inferred that the protective effect of Cu only works by killing the corrosive bacteria, which slows down the accelerated EET-MIC steps.
Conclusion
(1) After the welding treatment, the Cu addition had no obvious effect on the microstructure, which was composed of complete austenite grains in BM and the dendritic austenite matrix with small amount of δ-ferrite in WZs.
(2) The formation of biofilm was inhibited on the surface of 304L-Cu BM and 304L-Cu WZ.
(3) The MIC resistance of 304L SS was significantly improved by Cu addition. The resistance against uniform and pitting corrosion of 304L-Cu WZ was even much higher than that of 304L BM in P. aeruginosa culture medium.
Data Availability Statement
All datasets generated for this work are included in the article/supplementary material.
Author Contributions
CY and KY designed this work. LY, JZ, HZ, and YS prepared and characterized the samples. LY and TX cowrote the manuscript. CY revised the manuscript. All authors listed have made a substantial, direct and intellectual contribution to the work, and approved it for publication.
Funding
This work was financially supported by the National Key Research and Development Program of China (Grant No. 2016YFB0300205), the National Natural Science Foundation of China (Grant Nos. U1906226 and 51771199), the State Key Program of the National Natural Science of China (Grant No. 51631009), and Shenzhen Science and Technology Research Funding (JCYJ20160608153641020).
Conflict of Interest
The authors declare that the research was conducted in the absence of any commercial or financial relationships that could be construed as a potential conflict of interest.
References
Aktas, D. F., Lee, J. S., Little, B. J., Ray, R. I., Davidova, A. I., Lyles, C. N., et al. (2010). Anaerobic metabolism of biodiesel and its impact on metal corrosion. Energ. Fuel. 24, 2924–2928. doi: 10.1021/ef100084j
Dadfar, M., Fathi, M. H., Karimzadeh, F., Dadfar, M. R., and Saatchi, A. (2007). Effect of TIG welding on corrosion behavior of 316L stainless steel. Mater. Lett. 61, 2343–2346. doi: 10.1016/j.matlet.2006.09.008
Elguindi, J., Moffitt, S., Hasman, H., Andrade, C., Raghavan, S., and Rensing, C. (2011). Metallic copper corrosion rates, moisture content, and growth medium influence survival of copper ion-resistant bacteria. Appl. Microbiol. Biotechnol. 89, 1963–1970. doi: 10.1007/s00253-010-2980-x
Gui, Y., Zheng, Z. J., and Gao, Y. (2016). The bi-layer structure and the higher compactness of a passive film on nanocrystalline 304 stainless steel. Thin Solid Films 599, 64–71. doi: 10.1016/j.tsf.2015.12.039
Hamzah, E., Hussain, M. Z., Ibrahim, Z., and Abdolahi, A. (2013). Influence of Pseudomonas aeruginosa bacteria on corrosion resistance of 304 stainless steel. Corros. Eng. Sci. Technol. 48, 116–120. doi: 10.1179/1743278212Y.0000000052
Hashemi, S. J., Bak, N., Khan, F., Hawboldt, K., Lefsrud, L., and Wolodko, J. (2018). Bibliometric analysis of microbiologically influenced corrosion (MIC) of oil and gas engineering systems. Corrosion 74, 468–486. doi: 10.5006/2620
Huttunen-Saarivirta, E., Honkanen, M., Lepisto, T., Kuokkala, V. T., Koivisto, L., and Berg, C. G. (2012). Microbiologically influenced corrosion (MIC) in stainless steel heat exchanger. Appl. Surf. Sci. 258, 6512–6526. doi: 10.1016/j.apsusc.2012.03.068
Ishu, T., Ujiro, T., Hamada, E., Ishikawa, S., and Kato, Y. (2011). A mechanism of improvement in the corrosion resistance of ferritic stainless steels by Cu addition. Tetsu To Hagane 97, 441–449.
Jia, R., Unsal, T., Xu, D. K., Lekbach, Y., and Gu, T. Y. (2019). Microbiologically influenced corrosion and current mitigation strategies: a state of the art review. Int. Biodeterior. Biodegrad. 137, 42–58. doi: 10.1016/j.ibiod.2018.11.007
Jiang, J., Xu, D. K., Xi, T., Shahzad, M. B., Khan, M. S., Zhao, J. L., et al. (2016). Effects of aging time on intergranular and pitting corrosion behavior of Cu-bearing 304L stainless steel in comparison with 304L stainless steel. Corrosion Sci. 113, 46–56. doi: 10.1016/j.corsci.2016.10.003
Jin, S. J., Ren, L., and Yang, K. (2016). Bio-functional cu containing biomaterials: a new way to enhance bio-adaption of biomaterials. J. Mater. Sci. Technol. 32, 835–839. doi: 10.1016/j.jmst.2016.06.022
Li, H. B., Yang, C. T., Zhou, E. Z., Yang, C. G., Feng, H., Jiang, Z. H., et al. (2017). Microbiologically influenced corrosion behavior of S32654 super austenitic stainless steel in the presence of marine Pseudomonas aeruginosa biofilm. J. Mater. Sci. Technol. 33, 1596–1603. doi: 10.1016/j.jmst.2017.03.002
Li, H. B., Zhou, E. Z., Ren, Y. B., Zhang, D. W., Xu, D. K., Yang, C. G., et al. (2016). Investigation of microbiologically influenced corrosion of high nitrogen nickel-free stainless steel by Pseudomonas aeruginosa. Corrosion Sci. 111, 811–821. doi: 10.1016/j.corsci.2016.06.017
Li, M. J., Nan, L., Xu, D. K., Ren, G. G., and Yang, K. (2015). Antibacterial performance of a Cu-bearing stainless steel against microorganisms in tap water. J. Mater. Sci. Technol. 31, 243–251. doi: 10.1016/j.jmst.2014.11.016
Li, Y. C., Xu, D. K., Chen, C. F., Li, X. G., Jia, R., Zhang, D. W., et al. (2018). Anaerobic microbiologically influenced corrosion mechanisms interpreted using bioenergetics and bioelectrochemistry: a review. J. Mater. Sci. Technol. 34, 1713–1718. doi: 10.1016/j.jmst.2018.02.023
Liu, H. W., Gu, T. Y., Zhang, G. A., Liu, H. F., and Cheng, Y. F. (2018a). Corrosion of X80 pipeline steel under sulfate-reducing bacterium biofilms in simulated CO2-saturated oilfield produced water with carbon source starvation. Corrosion Sci. 136, 47–59. doi: 10.1016/j.corsci.2018.02.038
Liu, H. W., Sharma, M., Wang, J. L., Cheng, Y. F., and Liu, H. F. (2018b). Microbiologically influenced corrosion of 316L stainless steel in the presence of Chlorella vulgaris. Int. Biodeterior. Biodegrad. 129, 209–216. doi: 10.1016/j.ibiod.2018.03.001
Liu, H. W., Xu, D. K., Yang, K., Liu, H. F., and Cheng, Y. F. (2017). Corrosion of antibacterial Cu-bearing 316L stainless steels in the presence of sulfate reducing bacteria. Corrosion Sci. 132, 46–55. doi: 10.1016/j.corsci.2017.12.006
Lu, B. T., Chen, Z. K., Luo, J. L., Patchett, B. M., and Xu, Z. H. (2005). Pitting and stress corrosion cracking behavior in welded austenitic stainless steel. Electrochim. Acta 50, 1391–1403. doi: 10.1016/j.electacta.2004.08.036
Moon, K. M., Kim, Y. H., Kim, S. J., Yoon, J. H., Lee, Y. C., Lee, S. Y., et al. (2011). Electrochemical Evaluation on the corrosion properties of welding zones of 304 stainless steel. Adv. Mater. Res. 146-147, 1238–1242.
Nan, L., Xu, D. K., Gu, T. Y., Song, X., and Yang, K. (2015). Microbiological influenced corrosion resistance characteristics of a 304L-Cu stainless steel against Escherichia coli. Mater. Sci. Eng. C Mater. Biol. Appl. 48, 228–234. doi: 10.1016/j.msec.2014.12.004
Nan, L., and Yang, K. (2010). Cu ions dissolution from Cu-bearing antibacterial stainless steel. J. Mater. Sci. Technol. 26, 941–944. doi: 10.1016/S1005-0302(10)60152-1
Neria-Gonzalez, I., Wang, E. T., Ramirez, F., Romero, J. M., and Hernandez-Rodriguez, C. (2006). Characterization of bacterial community associated to biofilms of corroded oil pipelines from the southeast of Mexico. Anaerobe 12, 122–133. doi: 10.1016/j.anaerobe.2006.02.001
Nie, F. L., Wang, S. G., Wang, Y. B., Wei, S. C., and Zheng, Y. F. (2011). Comparative study on corrosion resistance and in vitro biocompatibility of bulk nanocrystalline and microcrystalline biomedical 304 stainless. Dent. Mater. 27, 677–683. doi: 10.1016/j.dental.2011.03.009
Pillay, C., and Lin, J. (2013). Metal corrosion by aerobic bacteria isolated from stimulated corrosion systems: effects of additional nitrate sources. Int. Biodeterior. Biodegrad. 83, 158–165. doi: 10.1016/j.ibiod.2013.05.013
Pouranvari, M., Alizadeh-Sh, M., and Marashi, S. P. H. (2015). Welding metallurgy of stainless steels during resistance spot welding part I: fusion zone. Sci. Technol. Weld. Join 28, 502–511. doi: 10.1179/1362171815Y.0000000015
Rabaey, K., Boon, N., Siciliano, S. D., Verhaege, M., and Verstraete, W. (2004). Biofuel cells select for microbial consortia that self-mediate electron transfer. Appl. Environ. Microbiol. 70, 5373–5382. doi: 10.1128/AEM.70.9.5373-5382.2004
Santo, C. E., Lam, E. W., Elowsky, C. G., Quaranta, D., Domaille, D. W., Chang, C. J., et al. (2011). Bacterial killing by dry metallic copper surfaces. Appl. Environ. Microbiol. 77, 794–802. doi: 10.1128/AEM.01599-10
Skovhus, T. L., Eckert, R. B., and Rodrigues, E. (2017). Management and control of microbiologically influenced corrosion (MIC) in the oil and gas industry-overview and a North Sea case study. J. Biotechnol. 256, 31–45. doi: 10.1016/j.jbiotec.2017.07.003
Sun, Y. P., Yang, C. T., Yang, C. G., Xu, D. K., Li, Q., Yin, L., et al. (2019). Stern–geary constant for X80 pipeline steel in the presence of different corrosive microorganisms. Acta Metall. Sin. (Engl. Lett.) 32, 1483–1489. doi: 10.1007/s40195-019-00902-6
Vigneron, A., Alsop, E. B., Chambers, B., Lomans, B. P. I, Head, M., and Tsesmetzis, N. (2016). Complementary microorganisms in highly corrosive biofilms from an offshore oil production facility. Appl. Environ. Microb. 82, 2545–2554. doi: 10.1128/AEM.03842-15
Wadood, H. Z., Rajasekar, A., Ting, Y. P., and Sabari, A. N. (2017). Role of Bacillus subtilis and Pseudomonas aeruginosa on corrosion behavior of stainless steel. Arab. J. Sci. Eng. 40, 1825–1836. doi: 10.1007/s13369-015-1590-4
Xi, T., Shahzad, M. B., Xu, D. K., Sun, Z. Q., Zhao, J. L., Yang, C. G., et al. (2017). Effect of copper addition on mechanical properties, and antibacterial property of 316L stainless steel corrosion resistance. Mater. Sci. Eng. C Mater. Biol. Appl. 71, 1079–1085. doi: 10.1016/j.msec.2016.11.022
Xi, T., Shahzad, M. B., Xu, D. K., Zhao, J. L., Yang, C. G., Qi, M., et al. (2016). Copper precipitation behavior and mechanical properties of Cu-bearing 316L austenitic stainless steel: a comprehensive cross-correlation study. Mater. Sci. Eng. A Struct. Mater. 675, 243–252. doi: 10.1016/j.msea.2016.08.058
Xu, D. K., Xia, J., Zhou, E. Z., Zhang, D. W., Li, H. B., Yang, C. G., et al. (2017a). Accelerated corrosion of 2205 duplex stainless steel caused by marine aerobic Pseudomonas aeruginosa biofilm. Bioelectrochemistry 113, 1–8. doi: 10.1016/j.bioelechem.2016.08.001
Xu, D. K., Zhou, E. Z., Zhao, Y., Li, H. B., Liu, Y. Z., Zhang, D. W., et al. (2017b). Enhanced resistance of 2205 Cu-bearing duplex stainless steel towards microbiologically influenced corrosion by marine aerobic Pseudomonas aeruginosa biofilms. J. Mater. Sci. Technol. 34, 1325–1336. doi: 10.1016/j.jmst.2017.11.025
Zhang, D., Ren, L., Zhang, Y., Xue, N., Yang, K., and Zhong, M. (2013). Antibacterial activity against Porphyromonas gingivalis and biological characteristics of antibacterial stainless steel. Colloid Surf. B Biointerfaces 105, 51–57. doi: 10.1016/j.colsurfb.2012.12.025
Zhang, P. Y., Xu, D. K., Li, Y. C., Yang, K., and Gu, T. Y. (2015). Electron mediators accelerate the microbiologically influenced corrosion of 304 stainless steel by the Desulfovibrio vulgaris biofilm. Bioelectrochemistry 101, 14–21. doi: 10.1016/j.bioelechem.2014.06.010
Keywords: Cu-bearing stainless steels, tungsten inert gas welding, microbiologically influenced corrosion, confocal laser scanning microscopy, pitting corrosion
Citation: Yin L, Xi T, Yang C, Zhao J, Sun Y, Zhao H and Yang K (2020) Investigation on Corrosion Resistance of Welded Cu-Bearing 304L Stainless Steel Against Pseudomonas aeruginosa. Front. Mater. 7:102. doi: 10.3389/fmats.2020.00102
Received: 14 October 2019; Accepted: 06 April 2020;
Published: 05 June 2020.
Edited by:
Daniel John Blackwood, National University of Singapore, SingaporeReviewed by:
Benjamin Salas Valdez, Autonomous University of Baja California, MexicoWolfram Fürbeth, DECHEMA-Forschungsinstitut (DFI), Germany
Copyright © 2020 Yin, Xi, Yang, Zhao, Sun, Zhao and Yang. This is an open-access article distributed under the terms of the Creative Commons Attribution License (CC BY). The use, distribution or reproduction in other forums is permitted, provided the original author(s) and the copyright owner(s) are credited and that the original publication in this journal is cited, in accordance with accepted academic practice. No use, distribution or reproduction is permitted which does not comply with these terms.
*Correspondence: Chunguang Yang, Y2d5YW5nQGltci5hYy5jbg==
†These authors have contributed equally to this work