- Wits Advanced Drug Delivery Platform Research Unit, Department of Pharmacy and Pharmacology, School of Therapeutic Sciences, Faculty of Health Sciences, University of the Witwatersrand, Johannesburg, South Africa
Targeted and controlled drug delivery employing “smart materials” is a widely investigated field, within which stimuli-responsive polymers, particularly those which are thermo-responsive, have received considerable attention. Thermo-responsive polymers have facilitated the formulation of in situ gel forming systems which undergo a sol-gel transition at physiological body temperature, and have revolutionized the fields of tissue engineering, cell encapsulation, and controlled, sustained delivery of both drugs and genes. However, the use of single thermo-responsive polymers in the creation of these systems has posed numerous problems in terms of physico-mechanical properties, such as poor mechanical strength, high critical gelation concentrations (CGC) resulting in increased production costs and solutions that are too viscous, toxicity, as well as gelation temperatures that are incompatible with physiological body temperatures. Hybridization of these thermo-responsive polymers with other polymers has therefore been employed, resulting in the creation of tailor-made drug delivery systems that have optimal gelation temperatures and concentrations, ideal viscosities and improved gel strengths. This article reviews various thermo-responsive polymers that have been employed in the formulation of thermo-gelling systems. Special attention has been given to the hybridization of each of these polymers, the resulting systems that have been created, and their biomedical applications.
Introduction
Stimuli responsive drug delivery systems have been widely investigated due to their role in facilitating targeted and controlled drug delivery (Thambi and Lee, 2019). Among the stimuli responsive systems, thermo-responsive drug delivery systems have received considerable attention, and will thus be the focal point of this article (Mura et al., 2013; Huang et al., 2019). Thermogels are gels that undergo an in situ sol-gel transition by virtue of the difference in their ambient storage temperature and the physiological temperature of the body (Kumar et al., 2018; Figure 1). Ideally, thermogels should be free flowing solutions at room temperature, and only form gels once administered into the body (Ruel-Gariépy and Leroux, 2004). This thermo-responsive behavior is attributed to a change in the hydrophilic and hydrophobic interactions among the polymer molecules and water molecules that make up the solution (Bajpai et al., 2008; Shah et al., 2018).
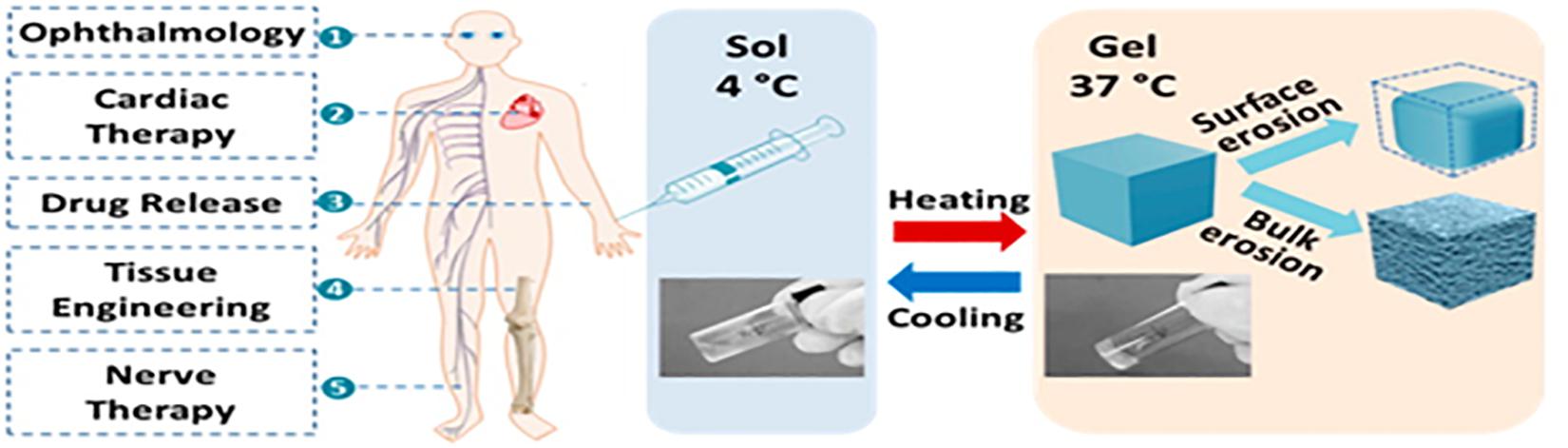
Figure 1. A graphical abstract of thermo-gelling systems and their biomedical applications. Reproduced with permission from Liow et al. (2016). Copyright 2016 American Chemical Society.
Among the decisive properties associated with thermo-responsive gels are the critical gelation concentration (CGC) and critical solution temperature (CST). The CGC is defined as the concentration of a material that results in thermo-gelation (Matanović et al., 2014). This is a crucial consideration in the formulation of thermogels (Bromberg and Ron, 1998), as it in turn affects the viscosity, gelation temperature and practicality of the formulation in terms of both usability and production costs. The CST is the temperature that is noted when phase separation of the solution occurs (Saeki et al., 1976). When polymers gel upon heating, they have a lower critical solution temperature, below which they are soluble and above which they become insoluble and hydrophobic (Matanović et al., 2014). Conversely, when polymers gel upon cooling, they display an upper critical solution temperature (Peppas et al., 2000). Hybridization of thermo-responsive polymers with other materials has allowed researchers to formulate ideal thermogels to suit their requirements, thereby mitigating issues that arise when the gelation concentrations of thermo-responsive polymers are too high, or gelation temperatures are not feasible for in situ gelation.
Properties of thermogels that make them advantageous for biomedical applications as opposed to existing, conventional drug delivery systems are as follows: (1) Thermogels mitigate the need for invasive surgical procedures and have a faster onset of action and better absorption as they by-pass first pass metabolism. Their sol-phase at ambient temperatures allows them to be injected at the site of action either intravenously or subcutaneously and take the shape of the area that they are injected into, where possible (Jeznach et al., 2018; Loh et al., 2018). (2) Thermo-gelling systems serve as a depot once injected into the body, mitigating the need for multiple daily dosing, thereby improving patient compliance and resulting in better therapeutic outcomes (Loh et al., 2007). Sustained release of a constant amount of drug is possible with thermogels, making it ideal in the treatment of chronic conditions (Liow et al., 2016). (3) Drug levels can be controlled, thus promoting safety of the patient. The need to administer a single dosage of drug at a high concentration is mitigated, thereby reducing the risk of systemic toxicity and unwanted adverse effects due to drug levels fluctuating or rising above safe concentrations. (4) The water content within the matrix of thermogels promotes their biocompatibility and enables them to serve as a system for drug delivery and lubrication in sensitive areas of the body (Caramella et al., 2015). (5) Most thermogels are easy to manufacture with the use of aqueous solvents, and are physically crosslinked in the presence of heat, thereby mitigating the need for organic solvents or crosslinking agents (Yan et al., 2015). This facilitates the delivery or proteins, peptides and genes via thermogels, by decreasing the risk of denaturation (Packhaeuser et al., 2004).
Thus, thermo-responsive systems have revolutionized the field of drug delivery. However, the use of single thermo-responsive polymers in drug delivery applications does have many setbacks which need to be overcome. These include: (1) high gelation concentrations which affects both manufacturing costs and convenience of application, (2) poor gel strengths resulting in very low viscosity gels which are unsuitable for certain applications, (3) high gelation temperatures that do not correlate with physiological body temperature, thereby not facilitating in situ gelation, and occasionally, (4) cytotoxicity. Hybridization of these single thermo-responsive polymers has allowed for the creation of tailor-made, targeted delivery systems with physico-mechanical properties precisely suited to the needs of the system (Mayet et al., 2014; Zarrintaj et al., 2019). These in situ gel forming systems have been shown to serve as excellent matrices for cell encapsulation, tissue engineering, and targeted, prolonged delivery of both drugs and genes. Their sol phase at ambient temperatures enables ease of application by injection at the desired site of action, and their ability to release drugs and genes in a sustained manner allows for less frequent dosing and administration, thereby contributing toward improved patient compliance and treatment efficacy.
This article is a review of key thermo-responsive polymers that have been used to form in situ gelling systems, namely: chitosan-β-glycerophosphate, poly (N-Isopropylacrylamide) (pNIPAAm), pluronic F127, methylcellulose and PEG-PCL. Special focus is given to the hybrid thermogels that have been formulated from each polymer, the advantages or reasons for hybridization and their applications in the biomedical field.
Hybrid Thermo-Responsive Polymer Systems
Chitosan Based Thermogels
Chitosan is a biodegradable, biocompatible polysaccharide that has been widely used in drug delivery applications. Consisting of N-acetyl-D-glucosamine and b-(1,4)-linked D-glucosamine that is randomly arranged, chitosan is a cationic polymer that is hydrophilic and has gel forming ability (Kristl et al., 1993). When the pH of a chitosan solution is increased to 7.2, chitosan can gel at body temperature. The first chitosan-based thermogel was created by Chenite et al. (2000). Chenite et al. (2000) developed a thermo-responsive gelling system by the addition of β-glycerophosphate, a polyol salt, to pH responsive chitosan solutions. The β-glycerophosphate serves to increase the pH of the acidic chitosan to 7–7.4 by acting as a weakly basic salt, allowing a gel to form in a controlled manner at 37°C, while maintaining its liquid state at 10°C (Chenite et al., 2000, 2001; Ruel-Gariépy et al., 2000). The resulting solution was a clear liquid at room temperature but gelled once at physiological body temperature. This thermo-gelling system was created without the use of any detergents, crosslinking agents or organic solvents. As the degree of deacetylation of chitosan increased, the sol stability at room temperature and the gelation time decreased (Ruel-Gariépy et al., 2000).
The rationale behind the thermo-responsive gelation of chitosan-β-glycerophosphate is as follows (Figure 2): (1) A reduction in the polarity of chitosan, resulting in an increase in the hydrophobicity of the matrix, (2) dehydration of the chitosan chain, (3) opportunities for hydrogen bonding together with hydrophobic interactions created by a shift in the charge density and attraction between chitosan and β-glycerophosphate due to the transfer of protons from chitosan to β-glycerophosphate (Ruel-Gariépy et al., 2000; Chenite et al., 2001). The above phenomena are achieved when an ideal balance between the following forces is achieved: (1) intramolecular electrostatic repulsive forces within chitosan, (2) attractive forces between the positively charged amine groups within chitosan and the negatively charged phosphate group within β-glycerophosphate, (3) hydrogen bonding and hydrophobic attractions that exist between the chitosan fragments, and (4) the ability of glycerol moieties to restructure water molecules (Chenite et al., 2001).
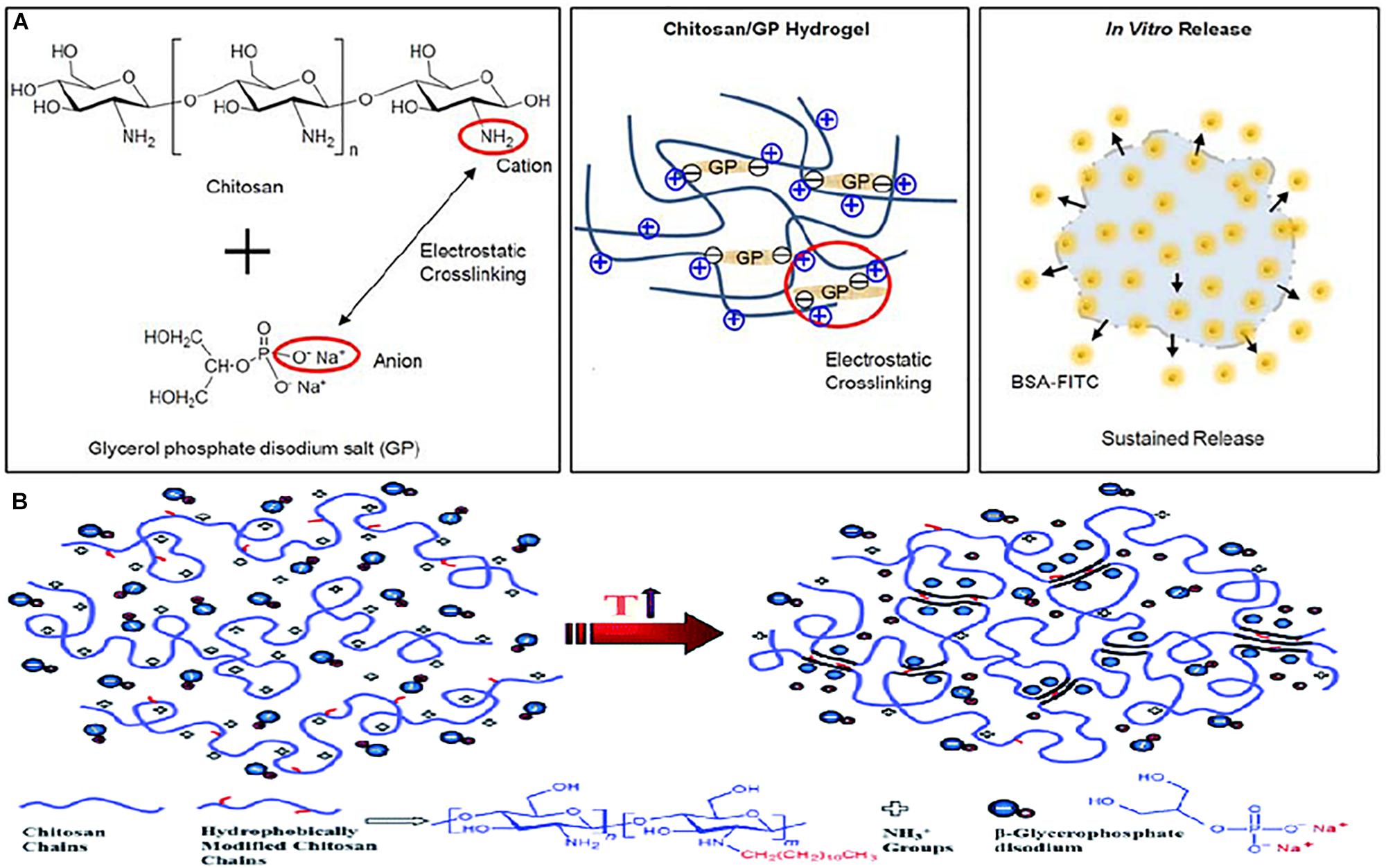
Figure 2. (A) Schematic image of chitosan/GP hydrogel formation via electrostatic crosslinking between amine cationic groups of the chitosan chains and the phosphate anionic groups of the GP, and in vitro release of BSA-FITC from BSA-FITC-loaded chitosan/GP hydrogels (Kim G. O. et al., 2012). Licensed under Creative Commons: Kim J. K. et al. (2012). (B) Illustration of the molecular interactions involve in the thermo-gelation process of chitosan-β-glycerophosphate. Licensed under Creative Commons: Dashtimoghadam et al. (2014).
Furthermore, Cho et al. (2005) attributed the thermo-gelation of chitosan-β-glycerophosphate to a decrease in hydrogen bonding within the chains, stating that as the temperature increases, vibrational and rotational energy transitions are experienced by the hydrated structures that surround the chitosan fragments, subsequently resulting in disorientation and dehydration. The now hydrophobic structures experience a change in conformation from a coiled, compact physical gel junction, with greater intramolecular hydrogen bonding, at lower temperatures, to an unfolded three-dimensional structure, with fewer intramolecular hydrogen bonds but more intermolecular hydrogen bonds, that is capable of forming contacts between zone junctions, thereby forming a gel at physiological body temperature (Kim et al., 2010).
Many attempts have been made in the hybridization of chitosan-β-glycerophosphate, particularly in the field of tissue engineering. It was found that hybridization of chitosan-glycerophosphate with collagen produced significantly better stem cell proliferation due to a reduction in toxicity of the thermogel as opposed to pure chitosan-glycerophosphate thermogels. This was depicted in a study conducted by Wang and co-workers, where chitosan-glycerophosphate was combined with type 1 collagen to form an in situ gel forming system capable of functioning as a matrix to encapsulate and deliver DNA and stem cells for tissue repair. Over a 12-day period, the content of DNA within the pure chitosan-glycerophosphate thermogel decreased by fifty percent, while a seventy percent increase in the hybridized thermogel was shown. The collagen containing composites promoted remodeling of the gel as well as survival of the cells when combined with the chitosan, which had an osteogenic effect (Wang and Stegemann, 2010). Furthermore, greater gel stiffness and gel compaction was observed. More recently, Song et al. (2017) depicted similar favorable results in terms of hybridization with collagen with an optical density that increased right up till day seven of their study, indicating cell growth, whereas the chitosan-glycerophosphate hydrogel devoid of collagen depicted an increase in optical density only until day three. The formulation gelled within 12 min at a temperature of 37°C, showed good mechanical strength and was able to maintain its integrity within the cell culture media for the entire duration of 4 weeks. In addition, in vivo studies depicted more favorable results for the hybridized thermogel than the pure chitosan-glycerophosphate thermogel, with lower immune resistance, better biocompatibility and approximately half of the gel biodegraded within 4 weeks post administration (Song et al., 2017). Furthermore, the current research shows that hybridization with NIPAAM also significantly reduces the toxicity of chitosan based thermogels, with an increase in cell proliferation correlating with an increase in the NIPAAM content within the hybridized thermogels. The addition of NIPAAm was also said to improve the solubility of the gels. This chitosan-NIPAAm thermogel formulated by Luo et al. (2020) was successful in the treatment of oral mucosal ulcers, with thermo-gelling temperatures of between 30.1 and 31.8°C thereby facilitating ease of application by injection at ulcer sites within the oral cavity. Chitosan was also shown to have antimicrobial activity in this study, and was able to inhibit both gram positive and gram negative bacterial growth, as well as promote gingival fibroblast proliferation and improve blood clotting, ultimately promoting the healing of ulcers and resulting in reduced ulcer healing times (Luo et al., 2020).
Interestingly, the release profile of chitosan based thermogels was optimized by first employing thiolation to form chitosan-4-thio-butylamide (CS-TBA), and then combining it with β-glycerophosphate and hydroxyapatite. The formulation gelled at a temperature of 37°C within 10 min and depicted good mucoadhesive properties, as well as good water solubility at a neutral pH. In vitro release studies showed an initial burst release of bovine serum albumin, followed by sustained release until day twenty of the study, with a total release of 98.8%. This release profile was attributed to the thiol groups and disulfide bonds which resulted in the formation of disulfide-linked covalent aggregates between bovine serum albumin and chitosan-4-thio-butylamide. The formulation showed potential in drug delivery and tissue engineering applications due to its low cytotoxicity, porous structure, favorable degradation rate, and uniform nano-hydroxyapatite distribution. Furthermore, the enhanced mucoadhesive properties of the system makes this system ideal for transmucosal drug delivery via oral, nasal, rectal, vaginal and ocular routes (Liu et al., 2014). In another study, hybridization with alginate was employed to improve the release profile of an mRNA loaded chitosan-glycerophosphate thermogel. The resulting system was able to gel at 37°C and showed a total of seventy percent cumulative release of the mRNA over a 3 week period. When chitosan was used alone, a slow release was depicted, and this was attributed to the positively charged chitosan interacting with the negatively charged mRNA, thereby retaining it. When alginate was used on its own, a fast release was shown, and this was attributed to electrostatic repulsive forces between the negatively charged alginate and the negatively charged synthetic mRNA. Thus, combining the negatively charged alginate with the positively charged chitosan-glycerophosphate resulted in a hybrid thermogel capable of sustained release of synthetic mRNA, at an optimal rate, faster than that of chitosan alone, but slower than that of alginate alone (Steinle et al., 2018).
Lastly, the gelation time as well as the gel strength of chitosan based thermogels was optimized in two ways. Shirosaki et al. (2015) developed a chitosan-glycerophosphate-γ glycidoxypropyltrimethoxysilane (GPTMS) thermogel for use as an injectable biomaterial in clinical applications. The addition of the γ-glycidoxypropyltrimethoxysilane decreased the gelation time of the chitosan-glycerophosphate hydrogel significantly, and increased the gel strength, in a proportionate manner. Results showed that the chitosan- γ-glycidoxypropyltrimethoxysilane gels did not degrade when exposed to phosphate buffer solution, and the rate of mass loss when the gel was exposed to lysozyme solution was inversely proportionate to the amount of γ-glycidoxypropyltrimethoxysilane added. The formulation formed an opaque gel at 36.5–37°C and showed excellent cytocompatibility with human osteoblasts and MG63 osteosarcoma cells. As the amount of γ-glycidoxypropyltrimethoxysilane was increased, the surface stiffness of the gel increased as well, resulting in improved cell attachment. The most favorable results in terms of cell proliferation was achieved when a moral ratio of 1:0.5 chitosan: γ-glycidoxypropyltrimethoxysilane was used (Shirosaki et al., 2015). Furthermore, manipulation of the gelation time and gel strength was also achieved when the effects of nanostructured chitosan/gelatin/bioactive glass thermogels in bone tissue engineering was investigated by Moreira et al. (2018). Increasing the content of gelatin and bioactive glass within the thermogel resulted in a decrease in the gelation time and temperature, as well as improved mechanical properties. Rheological studies depicted an increase in the elastic modulus (G’) of the gels from 5.4 Pa in pure chitosan gels to 12.4 Pa in the composite chitosan gels containing gelatin and bioactive glass. The live cell-viability of the osteosarcoma cell line was indicative of the cytocompatibility of this thermogel with human cells. The resulting formulation gelled at 37°C and was cationic, enabling it to interact with molecules that were negatively charged within the native extracellular matrix (Moreira et al., 2018).
Overall, hybrid chitosan based thermogels show promising results in terms of their potential in tissue engineering applications due to the reduction in toxicity and improvement in gel strength provided by hybridization (Zarrintaj et al., 2020). Hybridization of chitosan-β-glycerophosphate is possible with a range of different polymers to optimize the gel strength, gelation time, release profile and cytocompatibility of the gel.
Poly (N-Isopropylacrylamide) Based Thermogels
Poly (N-Isopropylacrylamide) (pNIPAAm) is synthetic thermo-responsive polymer derived from an acrylamide monomer, N-isopropylacrylamide (NIPAAM), consisting of amide and propyl moieties. pNIPAAm is an attractive prospect for thermo-responsive drug delivery applications due to its lower critical solution temperature (LCST) of approximately 32°C, close to the physiological human body temperature. Below the lower critical solution temperature, water molecules solvate the hydrophilic amide groups, pNIPAAm has good water solubility and exists in a flexible, coiled form (Doorty et al., 2003; Pei et al., 2004) with a hydration shell that is well structured. Above this temperature, pNIPAAm becomes insoluble in water and exists in globular form (Zhang et al., 2001) as interactions between hydrophobic propyl groups within the molecule become stronger and the hydrogen bonds weaken (Almeida et al., 2012). The chains within the polymer structure collapse and there is a loss of water from the structure, this volume phase transition (VPT) occurs at approximately 34°C (Figure 3) (Schild, 1992).
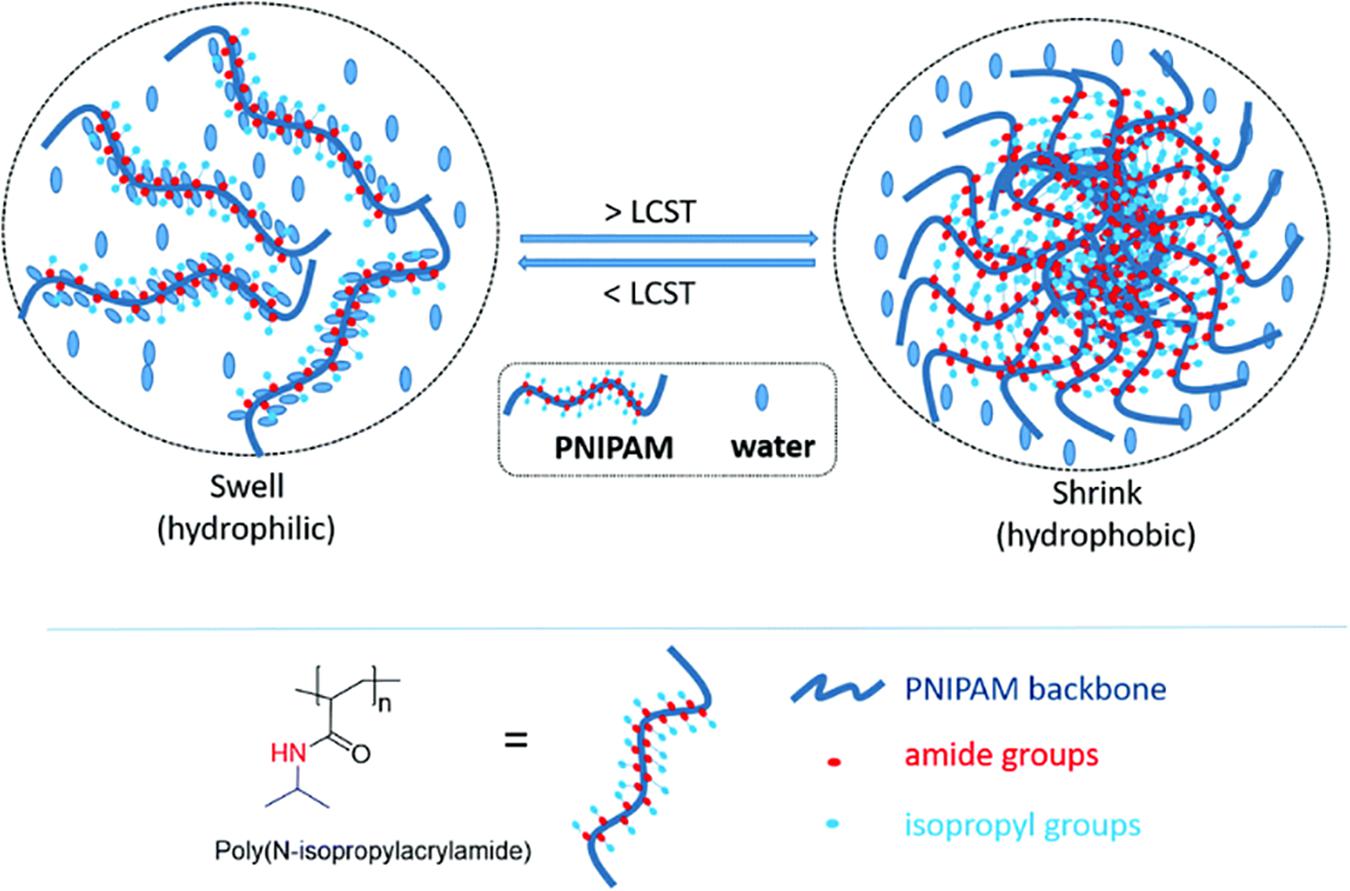
Figure 3. Schematic illustration of the chemical structure and thermo-gelling LCST behavior of PNIPAAm. Reproduced with permission from the Royal Society of Chemistry, Doberenz et al. (2020).
In the formulation of thermogels however, the use of pNIPAAm alone is limited, due to its poor mechanical strength (Zhang et al., 2016). This has resulted in copolymerization of NIPAAm with various other polymers such as poly (ethylene glycol) (PEG), acrylic acid (AA) and cellulose, to name a few. Copolymerization with polymers that are hydrophobic in nature results in a decrease in the lower critical solution temperature of pNIPAAm, while the opposite is true for copolymerization of NIPAAm with hydrophilic polymers (Kim et al., 2009). The increase in LCST when copolymerization with hydrophilic polymers occurs is attributed to an overall increase in hydrophilicity of the molecule, and hence an increase the hydrogen bonding interactions between the polymer and water molecules (Feil et al., 1993). When NIPAAm was copolymerized with cellulose, the LCST was increased when the ratio of methylcellulose within the copolymer was greater than that of NIPAAm, whereas when the percentage of methylcellulose was less than that of NIPAAm within the copolymer, the LCST was decreased. Furthermore, an improvement in mechanical strength of the gel was seen, with increasing storage moduli values at 36°C depicted as the cellulose content within the copolymer was increased. The resulting thermogel was shown to have promising application in blood vessel repair (Liu et al., 2004). Interestingly, unusual thermo-responsive behavior was seen when a poly(NIPAAm-co-AM)/PEG/PTA composite gel was developed by Guo et al. (2015). The formulation showed an upper critical solution temperature of 59.6°C, with no lower critical solution temperature seen within a 25–70°C temperature range. The upper critical solution temperature behavior was attributed to competitive behavior between PEG, p(NIPAAm-co-Acrylamide) and phosphoric tungstic acid (PTA). It was found that the upper critical solution temperature varied with the concentration of PEG. This thermo-responsive system has potential for application in catalysis and other separation systems (Guo et al., 2015).
Various studies have been conducted to improve the mechanical properties of NIPAAm by hybridization with other materials, as discussed below. Barnes et al. (2016) established that the addition of collagen to a poly(N-ispropylacrylamide) based thermogel resulted in a thermo-gelling system with improved mechanical strength, without compromising the cytocompatibility of the gel. It was found that with varying concentrations of the gels, the resulting formulations had shear moduli comparable with that of the soft tissues found within the human body, ranging from 105 Pa to less than 102 Pa. The pores and micro-structures within these gels also allowed for the encapsulated stromal cells to develop different morphologies, thus showing potential for directing the course of cell fate (Barnes et al., 2016). Hybridization with mesoporous silica (KIT-6) also proved to be an effective strategy in improving the mechanical strength of poly(N-ispropylacrylamide) based thermogels. The formulation, developed by Kamachi et al. (2016), had a volume phase transition at 34°C with the improved mechanical strength attributed to the formation of both topological and rigid crosslinks upon the addition of mesoporous silica. In addition, the employment of the mesoporous silica resulted in an increase in the adsorption capacity of the gel, with almost all the space offered by the mesopores available for drug or compound entrapment even after gelation, thereby offering a higher loading capacity for drugs or compounds (Kamachi et al., 2016). Zhang et al. (2016) successfully manipulated the mechanical properties of poly(N-ispropylacrylamide) via hybridization with acrylic acid, resulting in a dually crosslinked thermogel comprising of a two-level network structure. The first of which being the covalently crosslinked poly (N-ispropylacrylamide-co-acrylic acid) by the addition of a crosslinking agent, and the second being the multivalent ion pairing between acrylic acid groups in the poly (N-ispropylacrylamide-co-acrylic acid) and Fe (III). The resulting thermogel had a lower critical solution temperature of 32°C, with mechanical properties that varied per the concentration of acrylic acid within the hybrid gel. The mechanical strength of the gel was greatly improved, with results depicting strengths of up to 6 MPa tensile strength and 69.8 MPa compressive strength, with potential for application as tissues, soft robotics, sensors and manipulators (Zhang et al., 2016). Lastly, yet another strategy to improve the elasticity and strength of poly(N-isopropylacrylamide) based thermogels was developed by Yuan et al. (2018), by the reversable addition-fragmentation chain-transfer polymerization (RAFT synthesis) with ABA triblock copolymer flower-like nanogels. Apart from enhanced mechanical properties, the resulting formulation showed a phase transition temperature of 32°C with a rapid response and large swelling ratio. These properties were attributed to the small uniform pores within the gel structure, as well as the entanglement and crosslinking present between the gel matrix and the PDMA loops of the flower-like nanogel (Yuan et al., 2018).
Furthermore, the release profile of NIPAAm based thermogels can also be optimized by hybridization. A group of researchers formulated an injectable protein-reactive thermogel for the extended and sequential delivery of proteins, by first hybridizing NIPAAm with 2-Hydroxyethyl methacrylate (HEMA) and methacrylate polylactide (MAPLA) via free radical polymerization, and then incorporating protein loaded microspheres. The formulated copolymers were able to gel below 37°C and released protein for over 3 months. The injectability, biodegradability, and the extended and simultaneous sequential release characteristics of this system make it an attractive prospect in tissue engineering applications, particularly, intramyocardial injections following ischemic injury (Nelson et al., 2012). In another study by Lee et al. (2018), conjugation of NIPAAm with sulfonated poly(serinol hexamethylene urea) (SPSHU) was undertaken to formulate a thermogel for the controlled delivery of vascular endothelial growth factor (VEGF) in the promotion of therapeutic angiogenesis. The sulfonate groups within the gel created an electrostatic binding affinity to vascular endothelial growth factor (VEGF), thereby allowing the gel to bind to VEGF and localize it, facilitating its spatiotemporal control. Additionally, a decrease in the initial burst release was seen, together with a reduction in the sustained rate of release, showing the system’s potential for the delivery angiogenic factors (Lee et al., 2018).
NIPAAm has also shown promising results in the formulation of thermo-responsive, shape adaptive hydrogel actuators when hybridized with less- or non-responsive polymers, due to the differences in the thermo-responsive swelling and shrinking properties of the polymers (Zhang et al., 2019). Successful manipulation of both the lower critical solution temperature and shape adaptive behavior was achieved via copolymerization of NIPAAm with N-[3-(Dimethylamino)propyl]methacrylamide (DMAPMA) and acrylamide (AM) respectively. By combining pNIPAAm with polymers that are responsive to different stimuli, hydrogels with distinct swelling characteristics in each layer were formulated, such as a dually thermal and pH responsive pNIPAAm-PVA/PDMAEMA-PSS hydrogel, and the thermal and c(H’) responsive pNIPAAm-PDMAEMA. Further, to achieve faster, reversible, bidirectional bending in both hot and cold temperature conditions, NIPAAm, which possess a LCST, was copolymerized with poly(acrylic acid-co-acrylamide), which has an opposing UCST.
As it can be seen, hybridization of poly(N-isopropylacrylamide) in the formulation of thermogels not only results in an improvement in the mechanical strength and release profiles of the gel, but depending on the polymers used and the interactions between thermo-responsive NIPAAm and other less- or differently-responsive polymers, may display other favorable properties that may be exploited as well.
Pluronic Based Thermogels
Pluronic (commonly known as poloxamer) is a triblock co-polymer consisting of hydrophobic poly-propylene-oxide (PPO) at the center with hydrophilic poly-ethylene-oxide (PEO) on either side (Kabanov et al., 2002). The precise mechanism of gelation of pluronic has not been confirmed, but the following mechanisms have been proposed (Figure 4): (1) With an increase in temperature, there is a decrease in the micellar concentration due to changes of the properties of micelles with regards to aggregation and symmetry (Rassing and Attwood, 1982; Abou-Shamat et al., 2019); (2) The forces of friction between the copolymer micelles cease to exist with the dehydration of poly-propylene-oxide, consequently, there an increase in the viscosity of the system with gel formation (Rassing et al., 1984); (3) Water molecules around the core undergo re-organization, resulting in a change in entropy for (O–C–C–O) groups from a polar to a non-polar state, thereby propelling the sol-gel transition (Vadnere et al., 1984); (4) Hard-sphere crystallization occurs whereby above the critical micelle concentration, there is an equilibrium between the copolymer unimers and micelles at lower temperatures, with an increase in micelle formation and volume fraction in comparison to the unimers corresponding with an increase in temperature (Abdi et al., 2012).
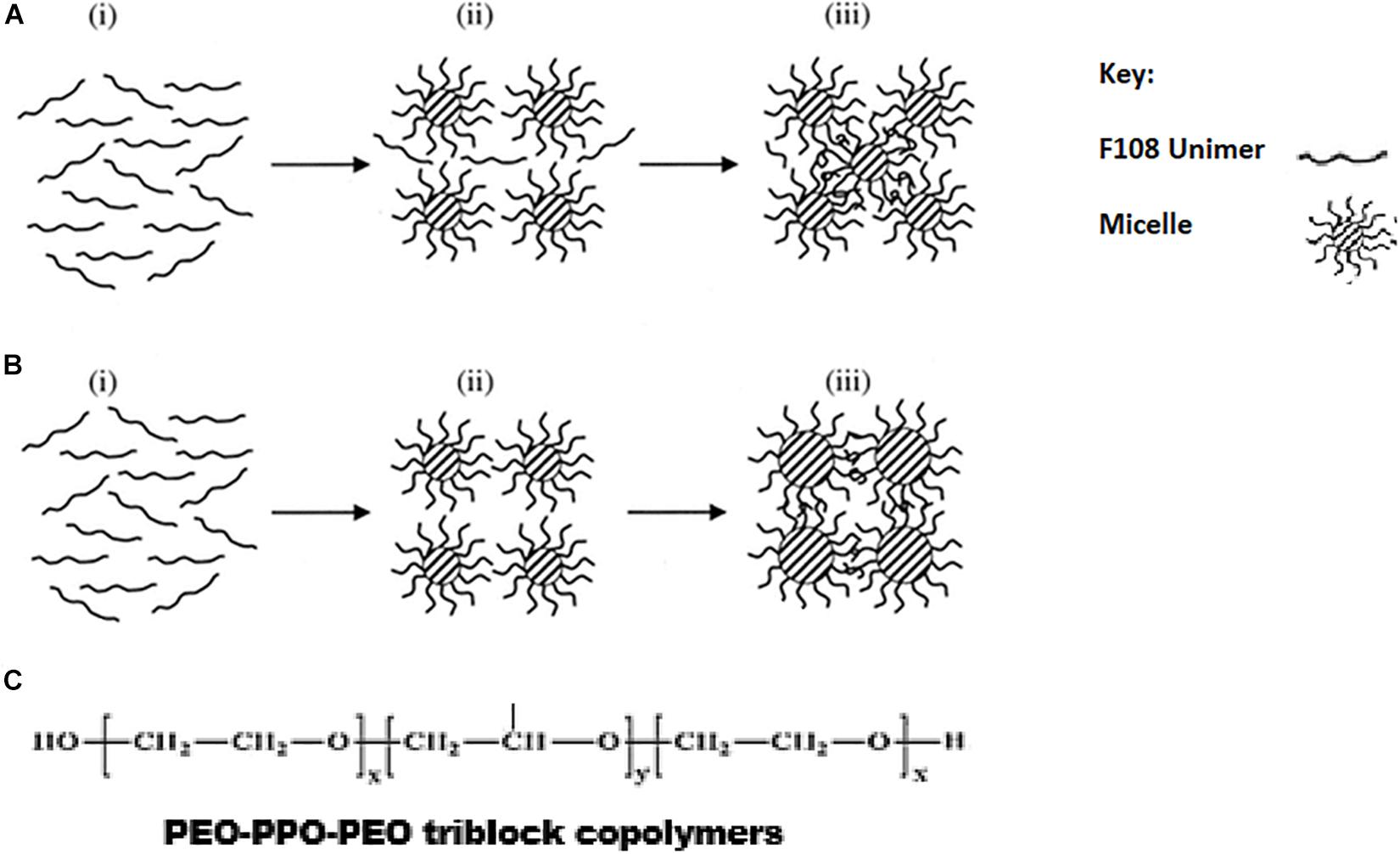
Figure 4. Schematic diagram for two mechanisms (A,B) for the gelation of F108 through micellization. From stage i to stage iii, the arrows indicate the direction of increasing temperature. F108 molecules exist as unimers at stage i, and the micelles are formed during stage ii. In mechanism A, the micellar sizes remain the same, but new micelles are formed at stage iii so that the intermicellar distance is shortened and gelation through entanglements is allowed. In mechanism B, the number of micelles remains unchanged during stage iii, but their size is increased with temperature so that the intermicellar distance is shortened and gelation through entanglements is allowed. Reproduced with permission from Lau et al. (2004), John Wiley and sons. (C) Chemical structure of PEO-PPO-PEO triblock co-polymers.
Pluronic F127 (also known as poloxamer 407) is a commonly used (PEO-PPO-PEO) triblock copolymer with a PEO: PPO ratio of 2:1 (Lin and Sung, 2000; Escobar-Chávez et al., 2006), and a gelation concentration greater than 20% at 25°C. At concentrations between 20 and 30%, the solution exists in the sol phase below 25°C and forms a thermo-reversible gel at body temperature (Miyazaki et al., 1984; Lenaerts et al., 1987). However, this high CGC is not ideal, resulting in the formation of gels with high viscosity (El-Kamel, 2002), gelation at ambient temperatures, and potential for cytotoxicity. In addition, the delicate structure of pluronic affects the stability of pluronic based thermogels in vitro in certain applications (Rangabhatla et al., 2016). To mitigate these problems, hybridization of pluronic has been used.
To combat the issue of high gelation concentrations, it was found that when hybridization with methylcellulose was employed, thermo-gelation was possible at concentrations of pluronic as low as 12% (Rangabhatla et al., 2016). In a study conducted by Rangabhatla et al. (2016), hybrid thermogels in ratios of 12 pluronic: methylcellulose and 14 pluronic: methylcellulose showed thermo-gelation at 37°C, while existing in the sol phase at room temperature. Furthermore, at room temperature, the gels exhibited low viscosities, thereby facilitating ease of injection into the body. Cell studies using these formulations depicted that the viability of the cells was increased upon the addition of methylcellulose to the pluronic based thermogel. The resulting formulation showed potential for application in bone remodeling as sustained release over a 28-day period was shown when etidronate disodium was incorporated (Rangabhatla et al., 2016).
To increase the strength of pluronic-based thermogels and improve the cell viability within pluronic-based thermogels, Abdi et al. (2012) proposed hybridization with alginate and hyaluronic acid. A blended hydrogel was formulated for cell injection applications. Due to the high concentrations of pluronic that is used in the formation of thermogels, toxicity is often problematic, resulting in poor cell viability. The formation of a pluronic-alginate blend increased the cell viability up to 80%. Furthermore, the incorporation of small amounts of hyaluronic acid (0.1%) to the blend showed even better cytocompatibility results, since hyaluronic acid is a component of the natural extracellular matrix, thereby facilitating the growth and survival of cells. The formulation also showed that as the concentration of alginate within the blend increased, the strength of the gel that was formed at physiological body temperature of 37°C was increased as well. When 3% of alginate was used, a strong gel capable of sustaining cells at physiological temperature and pH was achieved (Abdi et al., 2012). More recently, in a similar study, the efficacy of hybridization of pluronic with alginate in the formation of thermo-gelling systems was once again demonstrated by Quah et al. (2018). An increase of approximately 100-fold was also seen in the storage moduli of the formulated thermo-gelling system as the temperature increased from 15 to 40°C, indicating an increase in the stiffness of the gel, showing potential for application in soft tissue engineering, dental applications, wound healing and as an injectable drug delivery system. Interestingly, it was also found that while increasing the concentration of pluronic within the thermogels decreased the gelation temperature, increasing the concentration of alginate increased both the upper and lower transition temperatures (Quah et al., 2018).
To modify the gelation temperature of Pluronic F127 based thermogels such that they would only gel in situ, a group of researchers proposed hybridization with methacryloisobutyl- polyhedral oligosilsesquioxane (MA-POSS). The addition of MA-POSS to pluronic F127 via atom transfer radical polymerization resulted in the formation of a thermogel with a gelation temperature of 33.5°C, ten degrees higher than that of the pristine pluronic F127 gel, which displayed a gelation temperature of 23.5°C. This was attributed to the hinderance from the association of the hydrophobic poly-(propylene glycol) segments within pluronic F127 which induces gelation, by the rigid nano-block structure of POSS. Furthermore, the degradation temperature of the resulting hybrid thermogel was increased by 15°C as compared to that of the pristine pluronic F127 thermogel. In addition, rheological studies showed that the modulus of the gel was maintained upon the incorporation of MA-POSS, thus the strength of the gel was not compromised by the addition of POSS (Dou et al., 2016). Similarly, when pluronic F127 was hybridized with carboxymethyl chitosan (CMCS) for the localized and systemic delivery of chemotherapeutic agents, an increase both the gelation temperature and time was seen. This was attributed to the increase in hydrophilic groups upon the addition of the hydrophilic CMCS, and consequently a reduction in hydrophobic interactions. The incorporation of the chemotherapeutic 5-Flourouracil also resulted in an increase in the gelation time and temperature, by virtue of an increase in the hydrophilicity once again. Further, the increase in the hydrophilic carboxyl groups present when increasing the contents of CMCS within the formulation also resulted in an increase in drug release, particularly at pH7.4, due to a resulting increase in water uptake and subsequently drug diffusion (Khan et al., 2018).
Thus it can be seen that pluronic, while quite difficult to work within its pure form, can be copolymerized and hybridized with a range of different, easily available polymers to formulate ideal thermo-gelling systems that are suitable for use in a wide range of biomedical applications. Hybridization of pluronic in the formation of thermogels not only facilitates the use of lower concentrations of the polymer, but also results in improved strength and mechanical properties, and reduced toxicity.
Cellulose Based Thermogels
Methylcellulose and hydroxypropyl methylcellulose are hydrophobically substituted water soluble cellulose derivates that are thermo-responsive (Barros et al., 2014). At concentrations between one and ten percent, aqueous solutions of these polymers exist in solution form at lower temperatures, but form gels when the temperature is increased beyond the lower critical solution temperature. The sol-gel phase transition of methylcellulose occurs between 40 and 50°C, whereas that of hydroxypropyl methylcellulose occurs between 75 and 90°C (Sarkar, 1979; Kim J. K. et al., 2012).
Several mechanisms of thermo-reversible gelation of methylcellulose have been proposed (Figure 5), including: (1) Crosslinking loci formed by crystalline structures of trimethyl glucose create a gel network that has hydrogen bonds, hydrophobic interactions and dipolar interactions which support it (Kato et al., 1978); (2) At low temperatures, hydrophobic clustering occurs, resulting in unsubstituted chains aggregating and forming bundles, which then open at moderate temperatures, exposing the methyl groups to the aqueous solution thereby resulting in an increase in the volume of the solution. At high temperatures, hydrophobically crosslinked networks that are highly stable are formed as a result of loss of constituent water from the methyl side chains, depicting thermal hysteresis (Haque and Morris, 1993); (3) A cluster is formed due to the hydrophobic interactions between the methyl groups. Thereafter, at high temperatures, lattice restructuring occurs due to the imbalance between the polymer-polymer and polymer-water hydrogen bonding interactions, resulting in thermogelation induced by a phase-separation (Kobayashi et al., 1999; Bodvik et al., 2010); (4) Polymer chains aggregate and orient themselves parallel to each other, thereby reducing the interactions between water molecules and the hydrophobic groups, forming fibrillar structures (Jain et al., 2013).
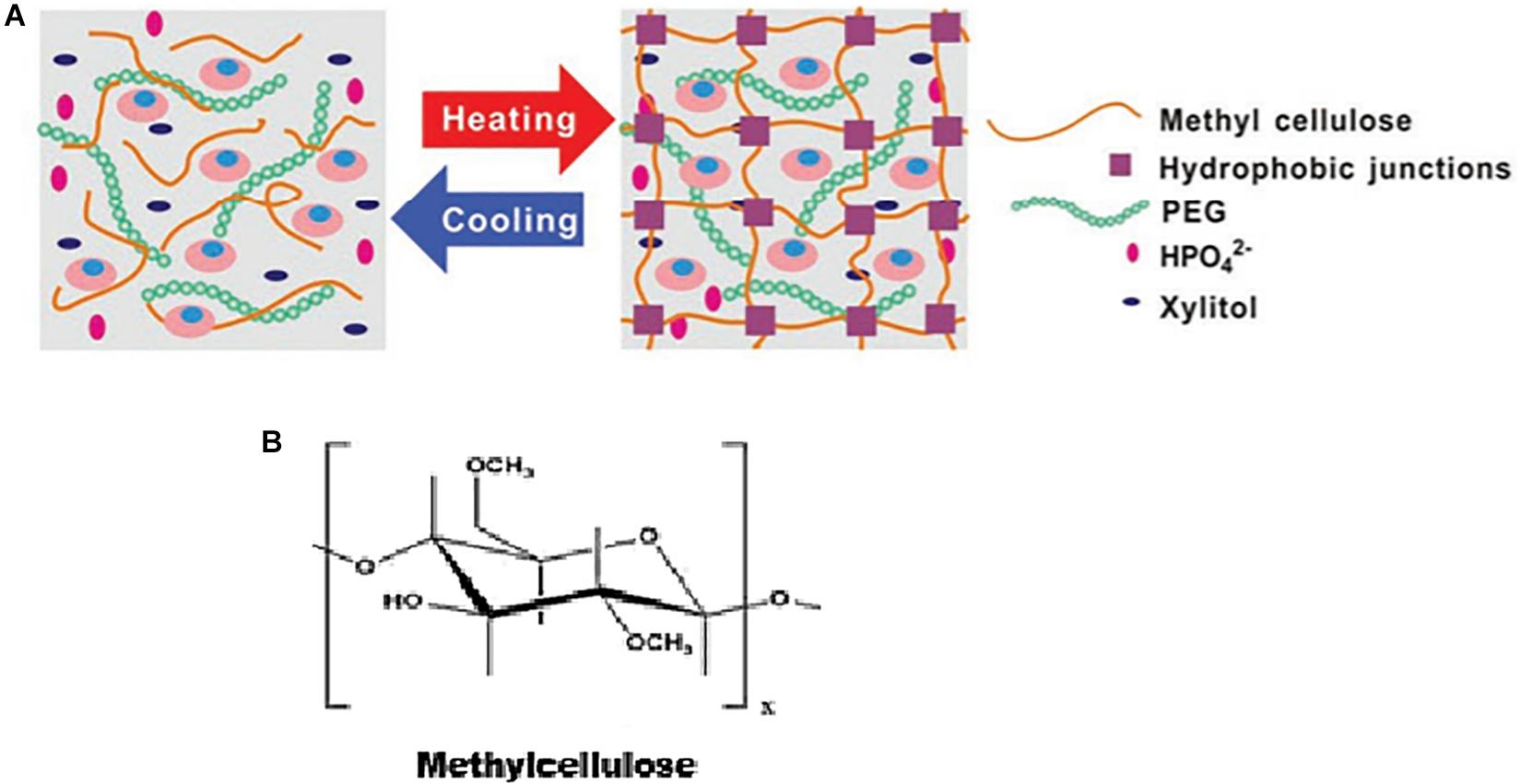
Figure 5. (A) Schematic illustrating the thermogelation of methylcellulose, as adapted from Wang et al. (2019). Reproduced with permission from John Wiley and sons. (B) Chemical structure of methylcellulose.
Recently, a group of researchers investigated the crosslinking kinetics of varying concentrations of methylcellulose in terms of heat exchange (Figure 6), onset temperatures (Figure 7) and reaction rates (Figure 8). It was found that methylcellulose displayed endothermic behavior during heating, with an exothermic effect preceding this in concentrations of methylcellulose below 2%. Furthermore, it was seen that exothermic heat was strongly dependent on the concentration of methylcellulose below 1%, and weakly dependent on the concentration methylcellulose above 1%. This phenomenon explained as follows: (1) At ambient temperature, hydrophilic interactions among the water molecules themselves and the between water molecules and methylcellulose are dominant. (2) There is a release of heat accompanied by the formation of methoxy groups and “water cages,” with water molecules surrounding the hydrophobic areas of methylcellulose in an orderly manner. (3) Intramolecular interactions occur between the polymer chains. (4) Upon heating the system, energy is consumed resulting in the release of methoxy groups and weakening of the “water cages.” This is known as the low temperature endothermic effect. (5) Intra- and intermolecular hydrophobic interactions result in the crosslinking of the methoxy group within the methylcellulose chains. This is known as the high temperature endothermic effect. In addition, at lower concentrations of methylcellulose, the destruction of the water cages (low temperature endothermic effect) occurred at a slower rate. In contrast, the rate of formation of the water cages increased as the concentration of methylcellulose increased. Lastly, the rate of crosslinking decreased with higher concentrations of methylcellulose (Niemczyk-Soczynska et al., 2019).
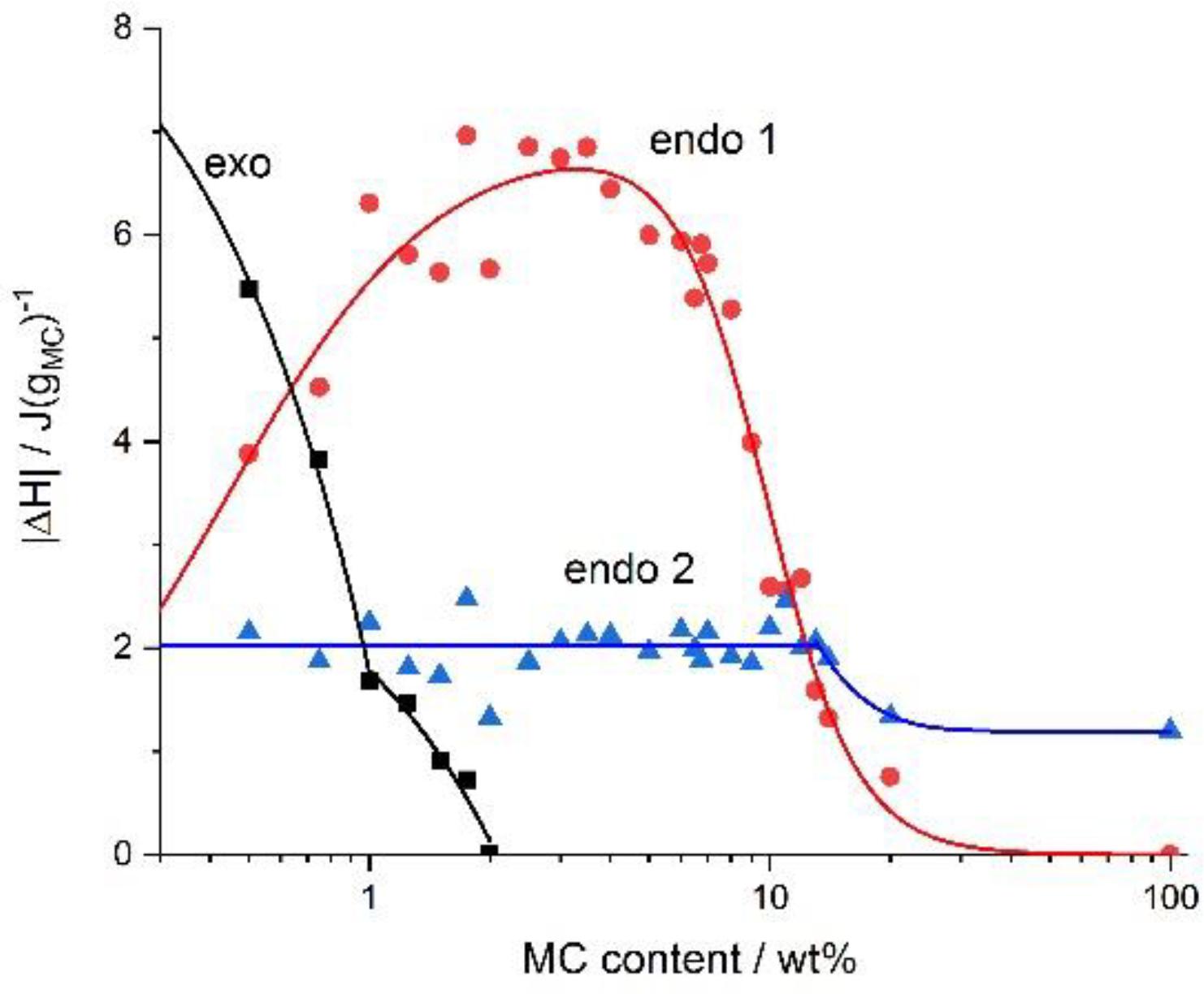
Figure 6. The absolute value of the heat exchanged upon heating during the exothermic, the low-temperature (endo 1) and the high-temperature (endo 2) endothermic effects versus the MC content. Reproduced with permission from Niemczyk-Soczynska et al. (2019). Copyright 2019 Polymers.
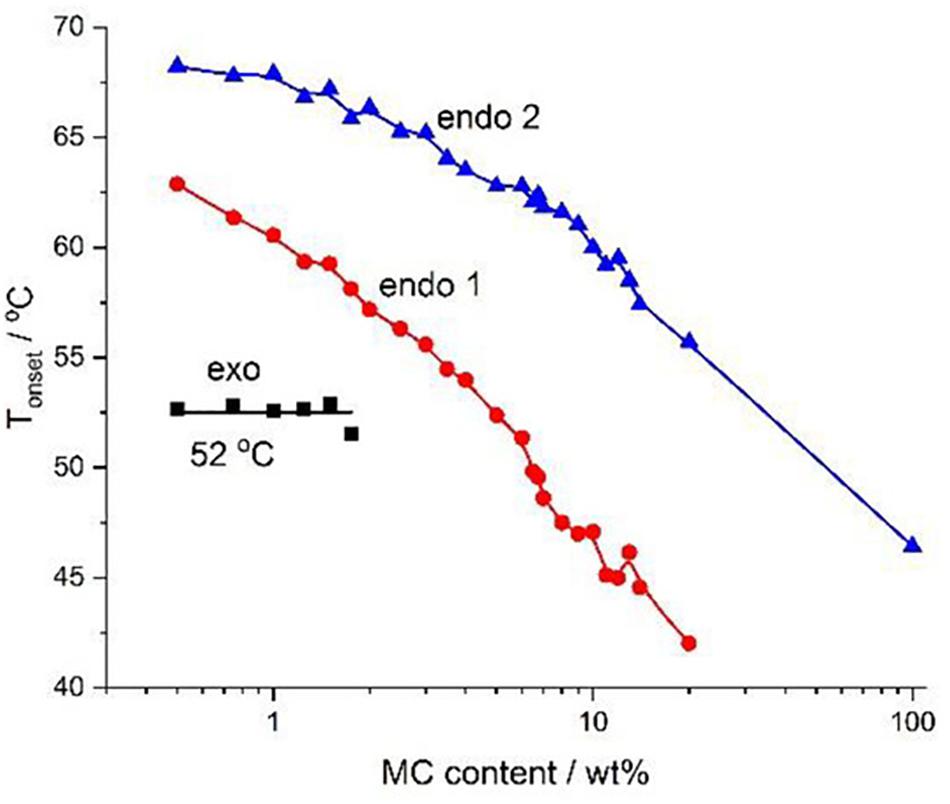
Figure 7. Phase diagram for various concentrations of MC constructed using the onset temperature. Reproduced with permission from Niemczyk-Soczynska et al. (2019). Copyright 2019 Polymers.
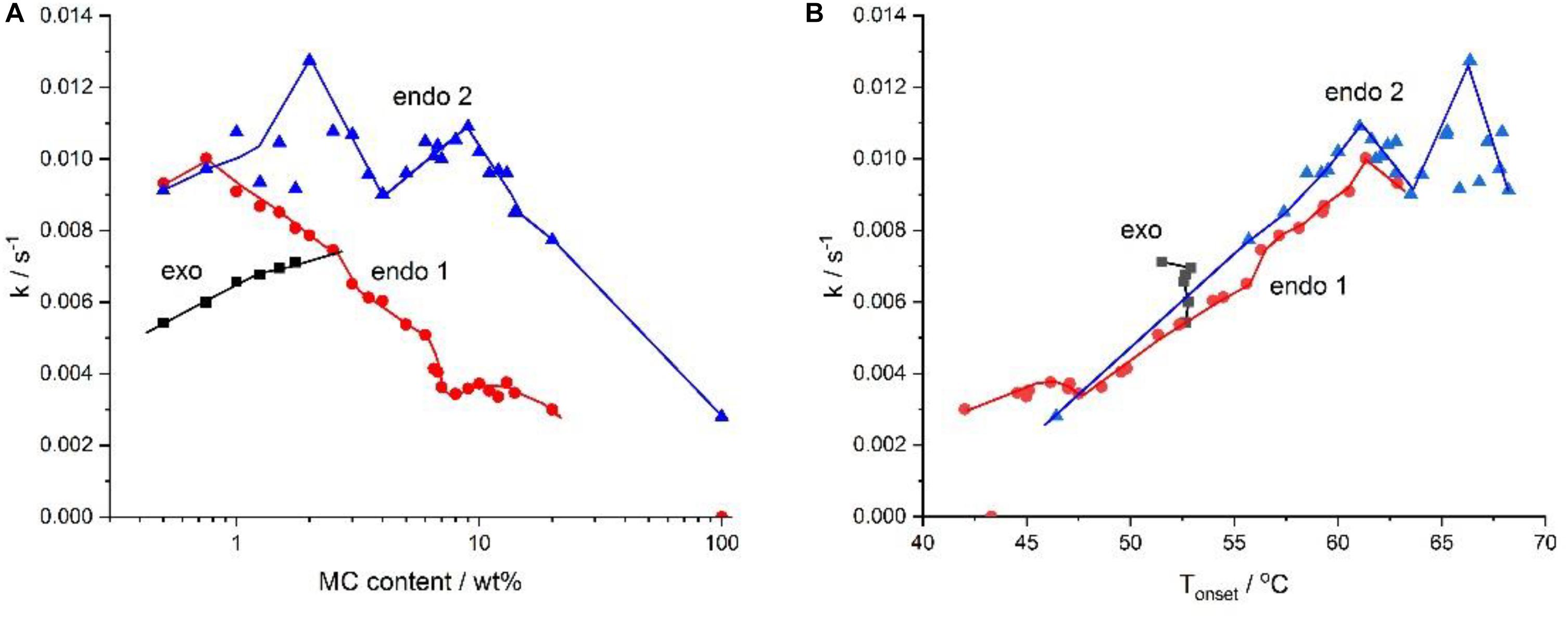
Figure 8. The rates, k, of water cages formation (exo), their destruction (endo 1), and of crosslinking (endo 2), as a function of – (A) the MC content and (B) the temperature, Tonset. Reproduced with permission from Niemczyk-Soczynska et al. (2019). Copyright 2019 Polymers.
Due to the high gelation temperature of cellulose based thermogels, hybridization of these polymers has been employed to formulate thermogels with gelation temperatures that are compatible with physiological body temperature. Nowald et al. (2016) developed a thermogel for wound healing applications by the addition of methylcellulose as a mechanical adjuvant to mucin. To combat this problem and induce gelation at a temperature of 37°C, glycerol was used. It was also shown that the higher the molecular weight of the methylcellulose used, the longer the gelation time, with a gelation time of approximately an hour for an 80 kDa methylcellulose gel compared to gelation within a mere few minutes when a 17 kDa methylcellulose was used. The only shortfall when using a 17 kDa methylcellulose, however, was the reduction in the stiffness and overall strength of the gel. To combat this problem, the concentration of methylcellulose that was used was increased simultaneously, yielding positive results. Furthermore, the substitution of NaCl with a kosmotropic ion KCl in an effort to speed up the gelation process in this study did not show any effect on the gelation kinetics of the gel (Nowald et al., 2016). Promising results in reducing the gelation temperature while simultaneously speeding up the gelation process were shown in another study, however, where xylitol was used in addition to sodium phosphate dibasic to reduce the gelation temperature of a methylcellulose based thermogel to 32°C, below the physiological body temperature, to facilitate the delivery of placental mesenchymal stem cells (PMSC) at lower body temperatures for the treatment of ischemia within limbs. The addition of polyethylene glycol to this system resulted in an increase the speed of gelation from 20 min, to a mere 150 s. It is worth noting that the sodium phosphate dibasic and xylitol worked synergistically to reduce the gelation temperature to 32°C, as when the sodium phosphate dibasic was used alone, the gelation temperature was only reduced to 36°C, and when the xylitol was used in isolation, a gelation temperature of 40°C was noted. The successful delivery of this thermogel together with the stem cells further showed potential in the promotion of vascular repair, by inhibiting fibrosis and muscular atrophy, promoting blood flow and stimulating angiogenesis. (Wang et al., 2019). Interestingly, synergistic behavior in the reduction of the thermo-gelling temperature of methylcellulose was also depicted with the incorporation of a biologically active molecule, gallic acid, together with a salt. Both gallic acid and sodium chloride were found to lower the gelation temperature of methylcellulose, however, when each was added to the methylcellulose in isolation, the gelation temperature was still above that of the physiological body temperature. When both gallic acid and sodium chloride were then combined simultaneously with methylcellulose in a ratio of 0.3:4:1, a gelation temperature of 36.7°C was achieved. Sustained release of drug together with the gallic acid was seen in the in vitro release studies (Sangfai et al., 2017).
The effects of the incorporation of different salts into methylcellulose-based thermogels on gel stability, transition temperature and the preparation process of thermogels was investigated in detail by Altomare et al. (2016). Gels containing higher concentrations of methylcellulose had a greater viscosity and a corresponding higher G’ value, and therefore a slower flow rate. An increase in the respective salt concentration within the thermogel for a constant concentration of methylcellulose resulted in an increase in G’, and a shift in the gelation temperature to lower temperatures, below 37°C. Different gelation temperatures were achieved depending on the salt added, for the same concentration of methylcellulose. When sodium sulfate and phosphate buffer saline were incorporated as salts respectively, a gelation temperature of 20°C was achieved. In contrast, when calcium chloride was added, a gelation temperature of 37°C was achieved. Furthermore, the critical salt concentration to achieve a gelation temperature of 37°C differed for each salt, due to the differences in the ionic interactions between the respective salts and methylcellulose. Overall, the thermogel containing 0.05M sodium sulfate and 8%w/v methylcellulose displayed the best results, with a transition temperature of 37°C, good stability and the best handling in terms of in vitro cell tests. This study showed potential for application of these gels in terms of cell sheet bio-fabrication and cell seeding (Altomare et al., 2016).
In theory, to decrease the gelation temperature of a methylcellulose-based thermogel, the concentration of methylcellulose can be increased, however, this results in an increase in viscosity such that the gel becomes difficult to handle, as well as a change in the kinetics of the formulation, as discussed above. Alternatively, a salt could be added to the gel to lower the gelation temperature, however, studies have shown a consequent decrease in the strength of such gels when in an aqueous solution or in a cell culture environment. To circumvent these issues and formulate a methylcellulose gel capable of thermogelation at physiological body temperature, Gaihre et al. (2019) recently blended calcium chloride and alginate with methylcellulose, resulting in the formation of a crosslinked, strong gel within 10 min at 37°C. The resulting formulation served as an injectable medium for the delivery of chitosan microparticles. Furthermore, faster gelation was observed upon the addition of the chitosan microparticles due to the absorption of water from the solution by the microparticles, thereby increasing the viscosity of the solution, as was evidenced by the swelling of the microparticles when added into the solution (Gaihre et al., 2019).
Many strategies have been proposed for the reduction of the gelation temperature of methylcellulose, each with their own setbacks, from the addition of salts, to merely increasing the concentration of methylcellulose within the gel. However, most recently, hybridization of methylcellulose to decrease the gelation temperature has shown promising results in terms of creating formulations that are capable of in situ gelation, making it an attractive prospect in biomedical applications. With further research, more successful hybrid combinations could be found.
PCL-PEG Based Thermogels
Poly(caprolactone) is a hydrophobic, biodegradable and biocompatible polymer that has been combined with the hydrophilic poly(ethylene glycol) in the formation of di- and triblock copolymers such as PEG-PCL-PEG and PCL-PEG-PCL(Bae et al., 2005). These crystalline polymers can be lyophilized and reconstituted into thermogels within minutes (Shah et al., 2018). The mechanism of PCL-PEG thermo-gelation has been described as follows: (1) at lower temperatures, a fully sol state is present, with the freely flowing micelles in the solution still small in size, (2) as the temperature is increased slightly, so too do the micelles increases in size, and this state is known as the favorably sol state, (3) as the temperature reaches the sol-gel transition temperature and the concentration is above CGC, we enter the favorably gel state, where micelles increases in size rapidly, (4) lastly, a dense gel is formed, as the micelles aggregate and pack closely together (Wei et al., 2009). The structure and mechanism of thermogelation of PEG-PCL based thermogels can be seen in Figure 9 in the case below. Hybridization of PEG-PCL based thermogels has been employed resulting in innovative in situ gel forming systems with improved mechanical strength, solubility, and release profiles, as discussed below.
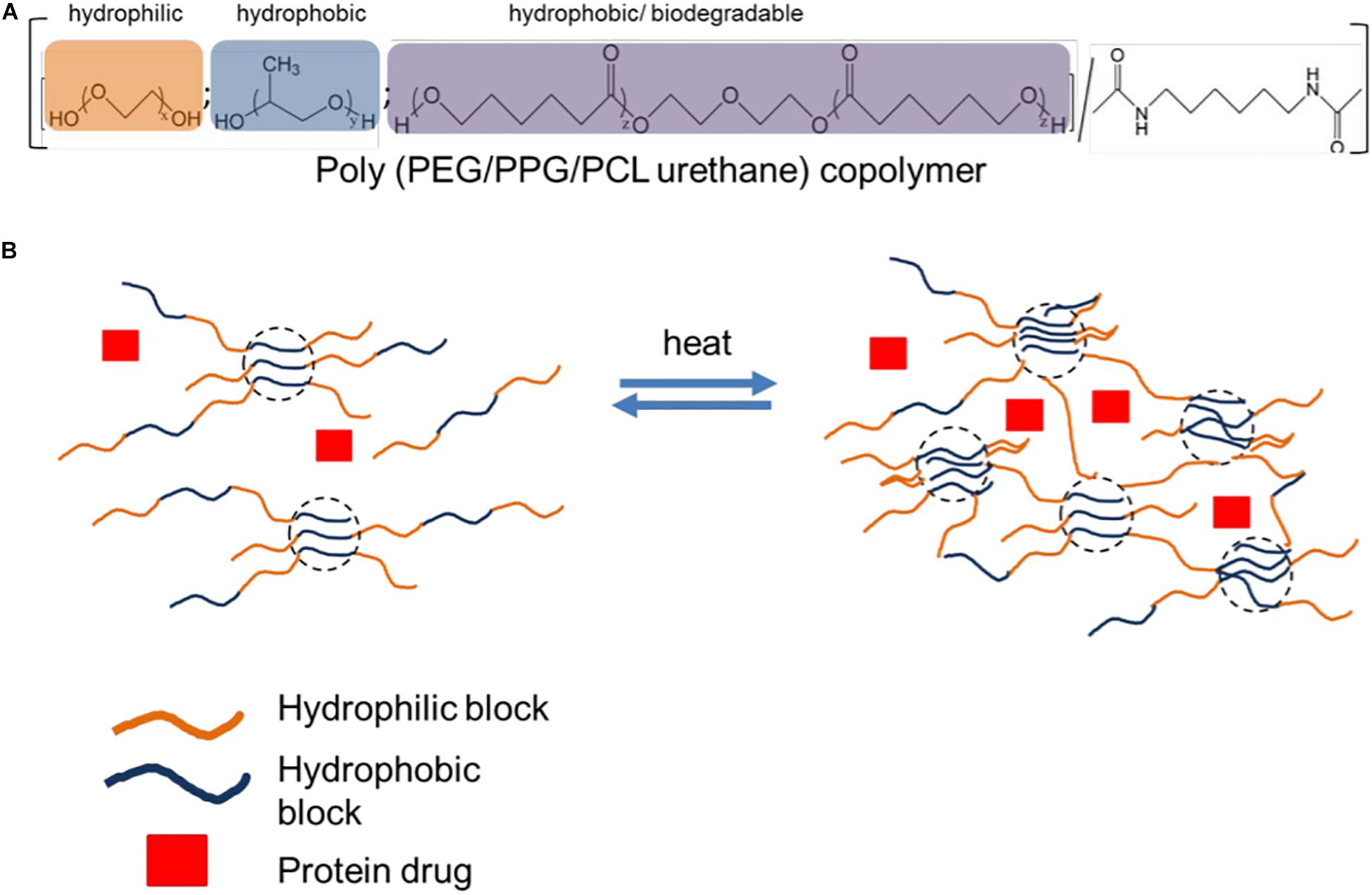
Figure 9. Thermogelling polyurethane copolymer for protein delivery. (A) Schematic of polyurethane copolymer composition based on function. (B) Protein drug is mixed with polyurethane copolymer. Upon heating, the multiblock copolymer is able to generate crosslinks and form a hydrogel under mild conditions to encapsulate protein drug. Reproduced with permission from Royal Society of Chemistry, Xue et al. (2019).
To improve the gel strength optimize the release profile of paclitaxel in the treatment of tumors, Loh et al. (2012) employed hybridization in the development of a poly(PEG-PPG-PCL-urethane) thermo-gelling system (Figure 9). The resulting solution formed a gel at physiological body temperature with an elastic modulus of 500 Pa and was able to withstand forces of up to 30 Pa. The CGC of the system was 3% wt. Drug release studies showed almost perfect zero order kinetics with the commonly seen “initial burst release” suppressed. Thus, this system proved to be ideal for the release of a consistent concentration of drug and it mitigated the danger of side effects that are often seen when chemotherapeutic drugs display an initial burst release, thereby resulting in concentrations of the chemotherapeutic agent rising above the maximum safe concentrations. In addition, when compared to a pluronic F127 gel in the study, the poly(PEG-PPG-PCL-urethane) thermogel showed more favorable results in terms of in vitro stability of the gel (Loh et al., 2012). This same group of researchers further employed their poly(PEG-PPG-PCL-urethane) thermogel in the delivery of anti-VEGFs to inhibit angiogenesis, mitigating the need for multiple injections. The release rate was now optimized by altering the hydrophile/lipophile balance, and a PEG:PPG ratio of 4:1 showed the best release profile for this application. The anti-VEGFs showed activity for up to 40 days, with anti-angiogenic effects depicted both ex vivo and in vivo. This study is a steppingstone for the development and use of thermogels in the delivery of bioactive protein molecules (Xue et al., 2019). In another study, gel strength drug release profiles were improved by combining PEG-PCL-PEG, pluronic F127, and poly propylene fumarate (PPF) in a ratio of 4:1:1 in the formulation of an innovative successful pseudo-bone thermogel. The resulting semi-elastic gel showed controlled, sustained release of the incorporated drug at physiological temperature over the entire 14-day period, compared with a shorter duration of drug release up till day 10 when at ambient room temperature. In addition, a viscous modulus of 0.1–0.3 Pa at temperatures between 10 and 20°C was noted, with an increase in gel strength by 45,000 times when physiological body temperature was reached (Kondiah et al., 2017).
The solubility and temperature responsiveness of PEG-PCL based thermogels was improved via the synthesis of a mPEG-b-[PCL-g-(MEO2MA-co-OEGMA)]-b-mPEG block-graft copolymer by Shang et al. (2019). Results showed that due to the hydrogen bonds formed between water molecules and the oligo (ethylene glycol) graft chain that is hydrophilic, the synthesized copolymer showed better solubility in water and formed a clear solution, compared to just PEG-PCL-PEG, which displayed a cloudy solution in water. In addition, the improvement in the thermo-responsiveness of the copolymer was attributed to the OEGMA and MEO2MA molecules, and the formulation showed a gelation temperature of 30°C (Shang et al., 2019).
Lastly, hybridization of PEG-PCL resulted in the formulation of an ideal penta-block PNIPAAm-PCL-PEG-PCL-PNIPAAm thermogel for application in wound healing. The formulation was able to gel at 37°C with a structure that was three dimensional and consisted of interconnected pores, similar to that of the extracellular matrix. Optimal results in terms of skin tissue engineering were achieved when a 20% formulation was used, with a thermogel pore size ideal for fibroblast attachment, and improved cell adhesion and cell proliferation indicating good biocompatibility. The formulation degraded gradually after 4 weeks and shows potential as an injectable dosage form for the sustained delivery of drugs, as well as in wound healing applications (Oroojalian et al., 2019). A comprehensive summary of the hybrid systems discussed within this article can be found in Table 1.
Future Prospects and Conclusion
In situ thermo-gelling systems may potentially replace conventional methods of treatment for diseases where sustained release of drugs, genes, proteins or other molecules are needed. These include chronic conditions which require constant amounts of drug over a long period of time, such as diabetes, cancer treatment and ocular conditions such as glaucoma, to name a few. Thermogels also show great potential for wound healing applications, due to their ability to adequately fill the irregularly shaped wound area, their ease of application and their biodegradability. There is great potential for the creation of tailor-made drug delivery systems by hybridization of thermo-responsive polymers with other compounds that either may or may not possess thermo-gelling ability but enhance the physico-mechanical properties and in vitro performance of thermo-gelling systems. Generally, natural polymers have the ability to mimic the human extra-cellular-matrix and provide good cell attachment but lack in mechanical strength, whereas synthetic polymers are often sought after due to their mechanical properties, but lack in their biocompatibility compared to natural polymers as well as in the ability to mimic the ECM. Therefore, hybridization of natural polymers with synthetic ones is a common trend that has been exploited by researchers. The creation of new thermo-gelling co-polymers via the hybridization process is an exciting prospect. However, further research in this field is needed to fully understand the thermo-gelling mechanisms and in vivo behavior of these systems to enable us to hybridize these polymers in a manner that will satisfy our dosage form and therapeutic requirements. Therefore, commercialization of thermo-gelling systems may still take a while. The inception of layer by layer dually or multi stimuli responsive polymer systems via the hybridization of polymers that are responsive to different stimuli will undoubtedly pave the way for the development of innovative hybrid polymer systems for a broad spectrum of applications, making it an intriguing prospect for future research. In addition, while the polymers reviewed within this article largely display LCST behavior, further research into polymers that display UCST and consequently the hybridization and exploitation of these polymers is needed. Lastly, while the scope of this article entailed thermo-responsive polymers and their applications in the biomedical field, many other stimuli responsive polymers exist, with a plethora of applications that have proven to be beneficial in a variety of other fields such as environmental remediation, oil adsorption, marine and textile applications to name a few (Li et al., 2018; Wang et al., 2018; Chatterjee and Chi-leung Hui, 2019; Tian et al., 2019; Gu et al., 2020).
Author Contributions
TS, PK, YC, and VP conceptualized the review. TS wrote the first draft. PK, YC, and VP reviewed, revised the manuscript, and supervised the study.
Funding
This work was funded by the University of the Witwatersrand, Johannesburg; the National Research Foundation of South Africa; and the South African Medical Research Council (SAMRC).
Conflict of Interest
The authors declare that the research was conducted in the absence of any commercial or financial relationships that could be construed as a potential conflict of interest.
References
Abdi, S. I. H., Choi, J. Y., Lee, J. S., Lim, H. J., Lee, C., Kim, J., et al. (2012). In vivo study of a blended hydrogel composed of pluronic F-127-alginate-hyaluronic acid for its cell injection application. Tissue Eng. Regen. Med. 9, 1–9. doi: 10.1007/s13770-012-0001-0
Abou-Shamat, M. A., Calvo-Castro, J., Stair, J. L., and Cook, M. T. (2019). Modifying the properties of thermogelling poloxamer 407 solutions through covalent modification and the use of polymer additives. Macromol. Chem. Phys. 220:1900173. doi: 10.1002/macp.201900173
Almeida, H., Amaral, M. H., and Lobão, P. (2012). Temperature and pH stimuli-responsive polymers and their applications in controlled and self-regulated drug delivery. J. Appl. Pharm. Sci. 2, 1–10. doi: 10.7324/JAPS.2012.2609
Altomare, L., Cochis, A., Carletta, A., Rimondini, L., and Farè, S. (2016). Thermo-responsive methylcellulose hydrogels as temporary substrate for cell sheet biofabrication. J. Mater. Sci. Mater. Med. 27:95. doi: 10.1007/s10856-016-5703-8
Bae, S. J., Suh, J. M., Sohn, Y. S., Bae, Y. H., Kim, S. W., and Jeong, B. (2005). Thermogelling poly(caprolactone-6-ethylene glycol-b-caprolactone) aqueous solutions. Macromolecules 38, 5260–5265. doi: 10.1021/ma050489m
Bajpai, A. K., Shukla, S. K., Bhanu, S., and Kankane, S. (2008). Responsive polymers in controlled drug delivery. Prog. Polym. Sci. 33, 1088–1118. doi: 10.1016/j.progpolymsci.2008.07.005
Barnes, A. L., Genever, P. G., Rimmer, S., and Coles, M. C. (2016). Collagen-Poly(N-isopropylacrylamide) hydrogels with tunable properties. Biomacromolecules 17, 723–734. doi: 10.1021/acs.biomac.5b01251
Barros, S. C., da Silva, A. A., Costa, D. B., Cesarino, I., Costa, C. M., Lanceros-Méndez, S., et al. (2014). Thermo-sensitive chitosan–cellulose derivative hydrogels: swelling behaviour and morphologic studies. Cellulose 21, 4531–4544. doi: 10.1007/s10570-014-0442-9
Bodvik, R., Dedinaite, A., Karlson, L., Bergström, M., Bäverbäck, P., Pedersen, J. S., et al. (2010). Aggregation and network formation of aqueous methylcellulose and hydroxypropylmethylcellulose solutions. Colloids Surf. A Physicochem. Eng. Asp. 354, 162–171. doi: 10.1016/j.colsurfa.2009.09.040
Bromberg, L. E., and Ron, E. S. (1998). Temperature-responsive gels and thermogelling polymer matrices for protein and peptide delivery. Adv. Drug Deliv. Rev. 31, 197–221. doi: 10.1016/S0169-409X(97)00121-X
Caramella, C. M., Rossi, S., Ferrari, F., Bonferoni, M. C., and Sandri, G. (2015). Mucoadhesive and thermogelling systems for vaginal drug delivery. Adv. Drug Deliv. Rev. 92, 39–52. doi: 10.1016/j.addr.2015.02.001
Chatterjee, S., and Chi-leung Hui, P. (2019). Review of stimuli-responsive polymers in drug delivery and textile application. Molecules 24:2547. doi: 10.3390/molecules24142547
Chenite, A., Buschmann, M., Wang, D., Chaput, C., and Kandani, N. (2001). Rheological characterisation of thermogelling chitosan/glycerol-phosphate solutions. Carbohydr. Polym. 46, 39–47. doi: 10.1016/S0144-8617(00)00281-2
Chenite, A., Chaput, C., Wang, D., Combes, C., Buschmann, M. D., Hoemann, C. D., et al. (2000). Novel injectable neutral solutions of chitosan form biodegradable gels in situ. Biomaterials 21, 2155–2161. doi: 10.1016/S0142-9612(00)00116-2
Cho, J., Heuzey, M. C., Bégin, A., and Carreau, P. J. (2005). Physical gelation of chitosan in the presence of β-glycerophosphate: the effect of temperature. Biomacromolecules 6, 3267–3275. doi: 10.1021/bm050313s
Dashtimoghadam, E., Mirzadeh, H., Taromi, F. A., and Nyström, B. (2014). Thermoresponsive biopolymer hydrogels with tunable gel characteristics. RSC Adv. 4, 39386–39393. doi: 10.1039/c4ra05246c
Doberenz, F., Zeng, K., Willems, C., Zhang, K., and Groth, T. (2020). Thermoresponsive polymers and their biomedical application in tissue engineering – a review. J. Mater. Chem. B 8, 607–628. doi: 10.1039/c9tb02052g
Doorty, K. B., Golubeva, T. A., Gorelov, A. V., Rochev, Y. A., Allen, L. T., Dawson, K. A., et al. (2003). Poly(N-isopropylacrylamide) co-polymer films as potential vehicles for delivery of an antimitotic agent to vascular smooth muscle cells. Cardiovasc. Pathol. 12, 105–110. doi: 10.1016/S1054-8807(02)00165-5
Dou, Q., Karim, A. A., and Loh, X. J. (2016). Modification of thermal and mechanical properties of PEG-PPG-PEG copolymer (F127) with MA-POSS. Polymers (Basel) 8:E341. doi: 10.3390/polym8090341
El-Kamel, A. H. (2002). In vitro and in vivo evaluation of Pluronic F127-based ocular delivery system for timolol maleate. Int. J. Pharm. 241, 47–55. doi: 10.1016/S0378-5173(02)00234-X
Escobar-Chávez, J. J., López-Cervantes, M., Naïk, A., Kalia, Y. N., Quintanar-Guerrero, D., and Ganem-Quintanar, A. (2006). Applications of thermo-reversible pluronic F-127 gels in pharmaceutical formulations. J. Pharm. Pharm. Sci. 9, 339–358.
Feil, H., Bae, Y. H., Feijen, J., and Kim, S. W. (1993). Effect of comonomer hydrophilicity and Ionization on the lower critical solution temperature of N-isopropylacrylamide copolymers. Macromolecules 26, 2496–2500. doi: 10.1021/ma00062a016
Gaihre, B., Unagolla, J. M., Liu, J., Ebraheim, N. A., and Jayasuriya, A. C. (2019). Thermoresponsive Injectable microparticle–gel composites with recombinant BMP-9 and VEGF enhance bone formation in rats. ACS Biomater. Sci. Eng. 5, 4587–4600. doi: 10.1021/acsbiomaterials.9b00082
Gu, H., Zhou, X., Lyu, S., Pan, D., Dong, M., Wu, S., et al. (2020). Magnetic nanocellulose-magnetite aerogel for easy oil adsorption. J. Colloid Interface Sci. 560, 849–856. doi: 10.1016/j.jcis.2019.10.084
Guo, P., Li, H., Ren, W., Zhu, J., Xiao, F., Xu, S., et al. (2015). Unusual thermo-responsive behaviors of poly(NIPAM-co-AM)/PEG/PTA composite hydrogels. Mater. Lett. 143, 24–26. doi: 10.1016/j.matlet.2014.12.074
Haque, A., and Morris, E. R. (1993). Thermogelation of methylcellulose. Part I: molecular structures and processes. Carbohydr. Polym. 22, 161–173. doi: 10.1016/0144-8617(93)90137-S
Huang, H., Qi, X., Chen, Y., and Wu, Z. (2019). Thermo-sensitive hydrogels for delivering biotherapeutic molecules: a review. Saudi Pharm. J. 27, 990–999. doi: 10.1016/j.jsps.2019.08.001
Jain, S., Sandhu, S., Malvi, R., and Gupta, B. (2013). Cellulose derivatives as thermoresponsive polymer: an Overview. J. Appl. Pharm. Sci. 3, 139–144. doi: 10.7324/JAPS.2013.31225
Jeznach, O., Kołbuk, D., and Sajkiewicz, P. (2018). Injectable hydrogels and nanocomposite hydrogels for cartilage regeneration. J. Biomed. Mater. Res. Part A 106, 2762–2776. doi: 10.1002/jbm.a.36449
Kabanov, A. V., Batrakova, E. V., and Alakhov, V. Y. (2002). Pluronic§block copolymers as novel polymer therapeutics for drug and gene delivery. J. Control. Release 82, 189–212. doi: 10.1016/S0168-3659(02)00009-3
Kamachi, Y., Bastakoti, B. P., Alshehri, S. M., Miyamoto, N., Nakato, T., and Yamauchi, Y. (2016). Thermo-responsive hydrogels containing mesoporous silica toward controlled and sustainable releases. Mater. Lett. 168, 176–179. doi: 10.1016/j.matlet.2015.12.132
Kato, T., Yokoyama, M., and Takahashi, A. (1978). Melting temperatures of thermally reversible gels IV. Methyl cellulose-water gels. Colloid Polym. Sci. 256, 15–21. doi: 10.1007/BF01746686
Khan, S., Akhtar, N., and Minhas, M. U. (2018). Fabrication, rheological analysis, and in vitro characterization of in situ chemically cross−linkable thermogels as controlled and prolonged drug depot for localized and systemic delivery. Polym. Adv. Technol. 30, 755–771. doi: 10.1002/pat.4514
Kim, G. O., Kim, N., Kim, D. Y., Kwon, J. S., and Min, B. H. (2012). An electrostatically crosslinked chitosan hydrogel as a drug carrier. Molecules 17, 13704–13711. doi: 10.3390/molecules171213704
Kim, J. K., Won, Y. W., Lim, K. S., and Kim, Y. H. (2012). Low-molecular-weight methylcellulose-based thermo-reversible gel/pluronic micelle combination system for local and sustained docetaxel delivery. Pharm. Res. 29, 525–534. doi: 10.1007/s11095-011-0581-8
Kim, S., Kim, J. H., Jeon, O., Kwon, I. C., and Park, K. (2009). Engineered polymers for advanced drug delivery. Eur. J. Pharm. Biopharm. 71, 420–430. doi: 10.1016/j.ejpb.2008.09.021
Kim, S., Nishimoto, S. K., Bumgardner, J. D., Haggard, W. O., Gaber, M. W., and Yang, Y. (2010). A chitosan/β-glycerophosphate thermo-sensitive gel for the delivery of ellagic acid for the treatment of brain cancer. Biomaterials 31, 4157–4166. doi: 10.1016/j.biomaterials.2010.01.139
Kobayashi, K., Huang, C. I., and Lodge, T. P. (1999). Thermoreversible gelation of aqueous methylcellulose solutions. Macromolecules 32, 7070–7077. doi: 10.1021/ma990242n
Kondiah, P. J., Choonara, Y. E., Kondiah, P. P. D., Kumar, P., Marimuthu, T., du Toit, L. C., et al. (2017). Development of an injectable pseudo-bone thermo-gel for application in small bone fractures. Int. J. Pharm. 520, 39–48. doi: 10.1016/j.ijpharm.2017.01.039
Kristl, J., Šmid-Korbar, J., Štruc, E., Schara, M., and Rupprecht, H. (1993). Hydrocolloids and gels of chitosan as drug carriers. Int. J. Pharm. 99, 13–19. doi: 10.1016/0378-5173(93)90317-9
Kumar, P., Choonara, Y. E., and Pillay, V. (2018). “Thermo-intelligent injectable implants: intricate mechanisms and therapeutic applications,” in Polymer Gels. Gels Horizons: From Science to Smart Materials, eds V. Thakur, M. Thakur, and S. Voicu (Singapore: Springer), 341–359. doi: 10.1007/978-981-10-6080-9_13
Lau, B. K., Wang, Q., Sun, W., and Li, L. (2004). Micellization to gelation of a triblock copolymer in water: thermoreversibility and scaling. J. Polym. Sci. Part B Polym. Phys. 42, 2014–2025. doi: 10.1002/polb.20105
Lee, D. J., Rocker, A. J., Bardill, J. R., Shandas, R., and Park, D. (2018). A sulfonated reversible thermal gel for the spatiotemporal control of VEGF delivery to promote therapeutic angiogenesis. J. Biomed. Mater. Res. Part A 106, 3053–3064. doi: 10.1002/jbm.a.36496
Lenaerts, V., Triqueneaux, C., Quartern, M., Rieg-Falson, F., and Couvreur, P. (1987). Temperature-dependent rheological behavior of Pluronic F-127 aqueous solutions. Int. J. Pharm. 39, 121–127. doi: 10.1016/0378-5173(87)90206-7
Li, S., Jasim, A., Zhao, W., Fu, L., Ullah, M. W., Shi, Z., et al. (2018). Fabrication of pH-electroactive bacterial cellulose/polyaniline hydrogel for the development of a controlled drug release system. ES Mater. Manuf. 1, 41–49. doi: 10.30919/esmm5f120
Lin, H. R., and Sung, K. C. (2000). Carbopol/pluronic phase change solutions for ophthalmic drug delivery. J. Control. Release 69, 379–388. doi: 10.1016/S0168-3659(00)00329-1
Liow, S. S., Dou, Q., Kai, D., Karim, A. A., Zhang, K., Xu, F., et al. (2016). Thermogels: in situ gelling biomaterial. ACS Biomater. Sci. Eng. 2, 295–316. doi: 10.1021/acsbiomaterials.5b00515
Liu, W., Zhang, B., Lu, W. W., Li, X., Zhu, D., De Yao, K., et al. (2004). A rapid temperature-responsive sol-gel reversible poly(N- isopropylacrylamide)-g-methylcellulose copolymer hydrogel. Biomaterials 25, 3005–3012. doi: 10.1016/j.biomaterials.2003.09.077
Liu, X., Chen, Y., Huang, Q., He, W., Feng, Q., and Yu, B. (2014). A novel thermo-sensitive hydrogel based on thiolated chitosan/hydroxyapatite/beta-glycerophosphate. Carbohydr. Polym. 110, 62–69. doi: 10.1016/j.carbpol.2014.03.065
Loh, X. J., Chee, P. L., and Owh, C. (2018). Biodegradable thermogelling polymers. Small Methods 3:1800313. doi: 10.1002/smtd.201800313
Loh, X. J., Goh, S. H., and Li, J. (2007). New biodegradable thermogelling copolymers having very low gelation concentrations. Biomacromolecules 8, 585–593. doi: 10.1021/bm0607933
Loh, X. J., Yee, B. J. H., and Chia, F. S. (2012). Sustained delivery of paclitaxel using thermogelling poly(PEG/PPG/PCL urethane)s for enhanced toxicity against cancer cells. J. Biomed. Mater. Res. Part A 100 A, 2686–2694. doi: 10.1002/jbm.a.34198
Luo, Z., Xue, K., Zhang, X., Lim, J. Y. C., Lai, X., Young, D. J., et al. (2020). Thermogelling chitosan-based polymers for the treatment of oral mucosa ulcers. Biomater. Sci. 8, 1364–1379. doi: 10.1039/c9bm01754b
Matanović, M. R., Kristl, J., and Grabnar, P. A. (2014). Thermoresponsive polymers: insights into decisive hydrogel characteristics, mechanisms of gelation, and promising biomedical applications. Int. J. Pharm. 472, 262–275. doi: 10.1016/j.ijpharm.2014.06.029
Mayet, N., Choonara, Y. E., Kumar, P., Tomar, L. K., Tyagi, C., Du Toit, L. C., et al. (2014). A comprehensive review of advanced biopolymeric wound healing systems. J. Pharm. Sci. 103, 2211–2230. doi: 10.1002/jps.24068
Miyazaki, S., Takeuchi, S., Yokouchi, C., and Takada, M. (1984). Pluronic F-127 gels as a vehicle for topical administration of anticancer agents1,2. Chem. Pharm. Bull. 32, 4205–4208. doi: 10.1248/cpb.32.4205
Moreira, C. D. F., Carvalho, S. M., Sousa, R. G., Mansur, H. S., and Pereira, M. M. (2018). Nanostructured chitosan/gelatin/bioactive glass in situ forming hydrogel composites as a potential injectable matrix for bone tissue engineering. Mater. Chem. Phys. 218, 304–316. doi: 10.1016/j.matchemphys.2018.07.039
Mura, S., Nicolas, J., and Couvreur, P. (2013). Stimuli-responsive nanocarriers for drug delivery. Nat. Mater. 12, 991–1003. doi: 10.1038/nmat3776
Nelson, D. M., Ma, Z., Leeson, C. E., and Wagner, W. R. (2012). Extended and sequential delivery of protein from injectable thermoresponsive hydrogels. J. Biomed. Mater. Res. Part A 100 A, 776–785. doi: 10.1002/jbm.a.34015
Niemczyk-Soczynska, B., Gradys, A., Kolbuk, D., Krzton-Maziopa, A., and Sajkiewicz, P. (2019). Crosslinking kinetics of methylcellulose aqueous solution and its potential as a scaffold for tissue engineering. Polymers (Basel) 11:1772. doi: 10.3390/polym11111772
Nowald, C., Penk, A., Chiu, H. Y., Bein, T., Huster, D., and Lieleg, O. (2016). A selective mucin/methylcellulose hybrid gel with tailored mechanical properties. Macromol. Biosci. 16, 567–579. doi: 10.1002/mabi.201500353
Oroojalian, F., Jahanafrooz, Z., Chogan, F., Rezayan, A. H., Malekzade, E., Rezaei, S. J. T., et al. (2019). Synthesis and evaluation of injectable thermosensitive penta−block copolymer hydrogel (PNIPAAm−PCL−PEG−PCL−PNIPAAm) and star−shaped poly(CL-CO-LA)−b−PEG for wound healing applications. J. Cell. Biochem. 120, 17194–17207. doi: 10.1002/jcb.28980
Packhaeuser, C. B., Schnieders, J., Oster, C. G., and Kissel, T. (2004). In situ forming parenteral drug delivery systems: an overview. Eur. J. Pharm. Biopharm. 58, 445–455. doi: 10.1016/j.ejpb.2004.03.003
Pei, Y., Chen, J., Yang, L., Shi, L., Tao, Q., Hui, B., et al. (2004). The effect of pH on the LCST of poly(N-isopropylacrylamide) and poly(N-isopropylacrylamide-co-acrylic acid). J. Biomater. Sci. Polym. Ed. 15, 585–594. doi: 10.1163/156856204323046852
Peppas, N. A., Bures, P., Leobandung, W., and Ichikawa, H. (2000). Hydrogels in pharmaceutical formulations. Eur. J. Pharm. Biopharm. 50, 27–46. doi: 10.1016/S0939-6411(00)00090-4
Quah, S. P., Smith, A. J., Preston, A. N., Laughlin, S. T., and Bhatia, S. R. (2018). Large-area alginate/PEO-PPO-PEO hydrogels with thermoreversible rheology at physiological temperatures. Polymer (Guildf.) 135, 171–177. doi: 10.1016/j.polymer.2017.12.003
Rangabhatla, A. S. L., Tantishaiyakul, V., Oungbho, K., and Boonrat, O. (2016). Fabrication of pluronic and methylcellulose for etidronate delivery and their application for osteogenesis. Int. J. Pharm. 499, 110–118. doi: 10.1016/j.ijpharm.2015.12.070
Rassing, J., and Attwood, D. (1982). Ultrasonic velocity and light-scattering studies on the polyoxyethylene-polyoxypropylene copolymer Pluronic F127 in aqueous solution. Int. J. Pharm. 13, 47–55. doi: 10.1016/0378-5173(82)90141-7
Rassing, J., McKenna, W. P., Bandyopadhyay, S., and Eyring, E. M. (1984). Ultrasonic and 13C-NMR studies on gel formation in aqueous solutions of the ABA block polymer Pluronic RF 127. J. Mol. Liq. 27, 165–178.
Ruel-Gariépy, E., Chenite, A., Chaput, C., Guirguis, S., and Leroux, J. C. (2000). Characterization of thermosensitive chitosan gels for the sustained delivery of drugs. Int. J. Pharm. 203, 89–98. doi: 10.1016/S0378-5173(00)00428-2
Ruel-Gariépy, E., and Leroux, J. C. (2004). In situ-forming hydrogels – review of temperature-sensitive systems. Eur. J. Pharm. Biopharm. 58, 409–426. doi: 10.1016/j.ejpb.2004.03.019
Saeki, S., Kuwahara, N., Nakata, M., and Kaneko, M. (1976). Upper and lower critical solution temperatures in poly (ethylene glycol) solutions. Polymer (Guildf.) 17, 685–689. doi: 10.1016/0032-3861(76)90208-1
Sangfai, T., Tantishaiyakul, V., Hirun, N., and Li, L. (2017). Microphase separation and gelation of methylcellulose in the presence of gallic acid and NaCl as an In Situ gel-forming drug delivery system. AAPS PharmSciTech 18, 605–616. doi: 10.1208/s12249-016-0546-7
Sarkar, N. (1979). Thermal gelation properties of methyl and hydroxypropyl methylcellulose. J. Appl. Polym. Sci. 24, 1073–1087. doi: 10.1002/app.1979.070240420
Schild, H. G. (1992). Poly(N-isopropylacrylamide): experiment, theory and application. Prog. Polym. Sci. 17, 163–249. doi: 10.1016/0079-6700(92)90023-R
Shah, V. M., Nguyen, D. X., Rao, D. A., Alany, R. G., and Alani, A. W. G. (2018). “Thermogel polymers for injectable drug delivery systems,” in Temperature-Responsive Polymers, eds V. V. Khutoryanskiy and T. K. Georgiou (Hoboken, NJ: John Wiley & Sons Ltd), 313–327. doi: 10.1002/9781119157830.ch13
Shang, P., Wu, J., Shi, X., Wang, Z., Song, F., and Liu, S. (2019). Synthesis of thermo-responsive block-graft copolymer based on pcl and peg analogs, and preparation of hydrogel via click chemistry. Polymers (Basel) 11:E765. doi: 10.3390/polym11050765
Shirosaki, Y., Hirai, M., Hayakawa, S., Fujii, E., Lopes, M. A., Santos, J. D., et al. (2015). Preparation and in vitro cytocompatibility of chitosan-siloxane hybrid hydrogels. J. Biomed. Mater. Res. Part A 103, 289–299. doi: 10.1002/jbm.a.35171
Song, K., Li, L., Yan, X., Zhang, W., Zhang, Y., Wang, Y., et al. (2017). Characterization of human adipose tissue-derived stem cells in vitro culture and in vivo differentiation in a temperature-sensitive chitosan/β- glycerophosphate/collagen hybrid hydrogel. Mater. Sci. Eng. C 70, 231–240. doi: 10.1016/j.msec.2016.08.085
Steinle, H., Ionescu, T. M., Schenk, S., Golombek, S., Kunnakattu, S. J., Özbek, M. T., et al. (2018). Incorporation of synthetic mRNA in injectable chitosan-alginate hybrid hydrogels for local and sustained expression of exogenous proteins in cells. Int. J. Mol. Sci. 19:E1313. doi: 10.3390/ijms19051313
Thambi, T., and Lee, D. S. (2019). “Stimuli-responsive polymersomes for cancer therapy,” in Stimuli Responsive Polymeric Nanocarriers for Drug Delivery Applications, eds A. S. Hamdy Makhlouf and N. Y. Abu-Thabit (Amsterdam: Elsevier), 413–438. doi: 10.1016/B978-0-08-101995-5.00016-7
Tian, J., Shao, Q., Zhao, J., Pan, D., Dong, M., Jia, C., et al. (2019). Microwave solvothermal carboxymethyl chitosan templated synthesis of TiO 2/ZrO 2 composites toward enhanced photocatalytic degradation of Rhodamine B. J. Colloid Interface Sci. 541, 18–29. doi: 10.1016/j.jcis.2019.01.069
Vadnere, M., Amidon, G., Lindenbaum, S., and Haslam, J. L. (1984). Thermodynamic studies on the gel-sol transition of some pluronic polyols. Int. J. Pharm. 22, 207–218. doi: 10.1016/0378-5173(84)90022-X
Wang, L., and Stegemann, J. P. (2010). Thermogelling chitosan and collagen composite hydrogels initiated with β-glycerophosphate for bone tissue engineering. Biomaterials 31, 3976–3985. doi: 10.1016/j.biomaterials.2010.01.131
Wang, R., Yao, X., Li, T., Li, X., Jin, M., Ni, Y., et al. (2019). Reversible thermoresponsive hydrogel fabricated from natural biopolymer for the improvement of critical limb ischemia by controlling release of stem cells. Adv. Healthc. Mater. 8:1900967. doi: 10.1002/adhm.201900967
Wang, W., Hao, X., Chen, S., Yang, Z., Wang, C., Yan, R., et al. (2018). pH-responsive Capsaicin@chitosan nanocapsules for antibiofouling in marine applications. Polymer (Guildf.) 158, 223–230. doi: 10.1016/j.polymer.2018.10.067
Wei, X. W., Gong, C. Y., Gou, M. L., Fu, S. Z., Guo, Q. F., Shi, S., et al. (2009). Biodegradable poly(ε-caprolactone)-poly(ethylene glycol) copolymers as drug delivery system. Int. J. Pharm. 381, 1–18. doi: 10.1016/j.ijpharm.2009.07.033
Xue, K., Zhao, X., Zhang, Z., Qiu, B., Tan, Q. S. W., Ong, K. H., et al. (2019). Sustained delivery of anti-VEGFs from thermogel depots inhibits angiogenesis without the need for multiple injections. Biomater. Sci. 7, 4603–4614. doi: 10.1039/c9bm01049a
Yan, Q., Xiao, L.-Q., Tan, L., Sun, W., Wu, T., Chen, L.-W., et al. (2015). Controlled release of simvastatin-loaded thermo-sensitive PLGA-PEG-PLGA hydrogel for bone tissue regeneration: in vitro and in vivo characteristics. J. Biomed. Mater. Res. Part A 103, 3580–3589. doi: 10.1002/jbm.a.35499
Yuan, B., Ding, M., Duan, W., Cao, M., Shi, K., and Zhang, W. (2018). Thermoresponsive hydrogels with high elasticity and rapid response synthesized by RAFT polymerization via special crosslinking. Polymer (Guildf.) 159, 1–5. doi: 10.1016/j.polymer.2018.11.017
Zarrintaj, P., Jouyandeh, M., Ganjali, M. R., Hadavand, B. S., Mozafari, M., Sheiko, S. S., et al. (2019). Thermo-sensitive polymers in medicine: a review. Eur. Polym. J. 117, 402–423. doi: 10.1016/j.eurpolymj.2019.05.024
Zarrintaj, P., Saeb, M. R., Jafari, S. H., and Mozafari, M. (2020). “Application of compatibilized polymer blends in biomedical fields,” in Compatibilization of Polymer Blends. (Amsterdam: Elsevier), 511–537. doi: 10.1016/b978-0-12-816006-0.00018-9
Zhang, F., Wu, W., Zhang, X., Meng, X., Tong, G., and Deng, Y. (2016). Temperature-sensitive poly-NIPAm modified cellulose nanofibril cryogel microspheres for controlled drug release. Cellulose 23, 415–425. doi: 10.1007/s10570-015-0799-4
Zhang, X. Z., Yang, Y. Y., Chung, T. S., and Ma, K. X. (2001). Preparation and characterization of fast response macroporous poly(N-isopropylacrylamide) hydrogels. Langmuir 17, 6094–6099. doi: 10.1021/la010105v
Keywords: thermo-responsive polymers, hybrid, chitosan-β-glycerophosphate, pNIPAAm, pluronic, cellulose, PEG-PCL
Citation: Sarwan T, Kumar P, Choonara YE and Pillay V (2020) Hybrid Thermo-Responsive Polymer Systems and Their Biomedical Applications. Front. Mater. 7:73. doi: 10.3389/fmats.2020.00073
Received: 25 November 2019; Accepted: 10 March 2020;
Published: 31 March 2020.
Edited by:
Roberto Brighenti, University of Parma, ItalyReviewed by:
Xufeng Dong, Dalian University of Technology, ChinaJohn Zhanhu Guo, University of Tennessee, Knoxville, United States
Copyright © 2020 Sarwan, Kumar, Choonara and Pillay. This is an open-access article distributed under the terms of the Creative Commons Attribution License (CC BY). The use, distribution or reproduction in other forums is permitted, provided the original author(s) and the copyright owner(s) are credited and that the original publication in this journal is cited, in accordance with accepted academic practice. No use, distribution or reproduction is permitted which does not comply with these terms.
*Correspondence: Viness Pillay, dmluZXNzLnBpbGxheUB3aXRzLmFjLnph
†These authors have contributed equally to this work