- 1Department of Chemistry, Bar-Ilan Institute for Nanotechnology and Advanced Materials, Bar-Ilan University, Ramat Gan, Israel
- 2Institute of Nano Science & Technology, Mohali, India
- 3Department of Materials Engineering, Ben-Gurion University of the Negev, Beer-Sheva, Israel
Transition metal phosphides already emerged with great interest due to their energy storage capacitance, superior metalloid characteristics, and decent electrical conductivity. To achieve a commercially viable outcome, these electrodes are fabricated with interconnected carbonaceous materials. Herein, we have synthesized hexagonal copper phosphide (Cu3P) platelets using chemical vapor deposition (CVD) and integrated it with highly conducting three-dimensional graphene (3DG), leading to a nanohybrid (Cu3P@3DG) as a coulombic efficient supercapacitor. This nanohybrid has exhibited a specific capacitance (Csp) of 1,095.85 F/g at 10 mV/s scan rate along with a cycling stability of 95% capacitive retention after 3,000 cycles at a current density of 8.97 A/g. The Csp is almost four times higher and the stability is 1.2 times higher compared to the bare Cu3P platelets. We have fabricated an asymmetric supercapacitor (ASC) using Cu3P@3DG on graphite as cathode and activated carbon (AC) on graphite as anode (Cu3P@3DG//AC) that has shown high specific capacity (108.78 F/g), energy density (8.23 Wh/kg), and power density (439.6 W/kg). Moreover, this ASC has exhibited an excellent life cycle (5,500 consecutive charge–discharge with 96% coulombic efficiency). Therefore, the proposed all-solid-state hybrid device can be considered as a route for next-generation high-performing energy storage devices.
Introduction
The relentless increase in energy demand and parallel steady reduction of fossil fuels drive the scientific and industrial need to develop renewable energy along with faster and compatible energy storage devices (Li et al., 2015, 2017; Zhang et al., 2019). Supercapacitors represent essential components that can bridge between stereotype capacitors and rechargeable batteries in terms of power and energy densities with a long cycle life (Wang et al., 2018; Cao et al., 2019; Chen et al., 2019). In recent times, the synthesis of materials having semiconducting, metalloid, and energy storage properties has emerged at the forefront of research activities (Augustyn et al., 2014; López-Ortega et al., 2015; Sivula and Van De Krol, 2016). The transition metal phosphides are a fascinating class of materials because of their wide range of properties depending on their size and shape (Barry et al., 2008; Shao et al., 2017; Aziz and Islam, 2018). Metal phosphides have been tested and found to be active catalysts with their potential applications in electro-catalysis and photo-catalysis. In the last few years, several phosphides of nickel, cobalt, iron, molybdenum, tungsten, copper, and many more with different morphologies and crystallinity have been synthesized using different methods including phosphorization, electrodeposition, and ball milling (Brock et al., 2004; Henkes et al., 2007; Barry et al., 2008; Brock and Senevirathne, 2008; Wang et al., 2010). Metal phosphides with controlled nanostructures and elemental compositions have been designed and reported to provide significant enhancement in electrochemical activity (Wang et al., 2015a; Chen et al., 2016; Seo et al., 2016; Wu H. et al., 2016). Also, metal phosphides have metalloid properties and exhibit high specific capacitances (Csp) and high volumetric and gravimetric capacities with lower intercalation potentials compared to commercial carbonaceous materials. Thus, they have attracted extensive interest to design novel electrode materials for supercapacitors rather than lithium-ion batteries (Li et al., 2017). Although transition metal hydroxides, metal oxides, and polymers (conducting) are commonly used electrode materials for lithium-ion batteries and pseudo-capacitors (Wang et al., 2015a, c; Zhu et al., 2015; Wu J. et al., 2016), however, these materials are kinetically slow for the fast electron transport and that invariably required for high-power density with moderate energy density (Li et al., 2017).
Copper phosphide (Cu3P) is an air-stable, low-cost, environment-friendly material that exhibits high volumetric as well as comparable gravimetric capacity to that of graphite (Pfeiffer et al., 2004). Cu3P have also been explored widely in the field of photo/electro-catalytic water splitting (Sun et al., 2015; Wei et al., 2016; Han et al., 2017; Hao et al., 2016). Recently, this material has been introduced in the energy storage sector to bridge the gap between electric double-layer capacitor (EDLC) and batteries (Chen et al., 2017; Zhang et al., 2019). It has been proved that the electrochemical activity of a material is strongly affected by its size, structure, and chemical composition. Cu3P with different morphology and crystallinity have been synthesized and investigated for energy storage devices showing an improvement in electrochemical activities (Liu et al., 2012, 2016; Fan et al., 2016).
Carbonaceous matrices [e.g., graphene, carbon natotube (CNT), graphite, etc.] are simultaneously proved to be a promising choice of electrode material due to its high conductivity, large surface area, flexibility, and transparency which drags its attention toward large-scale applications in energy storage devices. Among various carbonaceous materials, three-dimensional graphene (3DG) is the promising choice as a backbone for electroactive materials for supercapacitor due to the random orientation of graphene flakes in three dimensions leading to the formation of micro/mesoporous structures (Mukherjee and Austeria, 2016; Singh et al., 2017; Xue et al., 2018). The 3DG provides high conductive channels for ion and electron transfer in charging–discharging cycles leading to a prominent improvement in overall capacitive performance of electroactive materials. Also, 3DG effectively minimizes the unwanted agglomeration of metal nanoparticles, thereby enhancing the chemical stability of the electroactive materials. In compliance to develop a novel approach for the synthesis of new hybrid materials (Kumar et al., 2017; Itzhak et al., 2018) herein, we report a facile, industrially scalable and easy method to produce a high yield of hexagonal Cu3P by solid-state reaction at low temperature followed by the formation of nano-hybrid with 3DG matrix on graphite substrate. This nano-hybrid has demonstrated significantly high electrochemical performance at an optimized Cu3P:3DG ratio with a tremendous Csp of 1,095.85 F/g at a scan rate of 10 mV/s. The asymmetric device of Cu3P@3DG electrode (positive) and activated carbon (AC) (negative electrodes) on graphite substrate reveals worthy performance in power densities, cycling stability, and excellent capacitive retention.
Experimental Section
Materials
The copper foil was purchased from Alfa-Aesar. Red phosphorus was purchased from Sigma Aldrich and was used without any further purification. A compressed cylinder tank of argon gas was procured from Gas Technologies with a purity of 99.999%. An atmospheric-pressure three-zone chemical vapor deposition (CVD) furnace, fitted with a quartz tube with an internal diameter of 22 mm, was used for the synthesis. The built-in furnace thermocouples measured the furnace temperatures, and the flow of argon was maintained using electronic mass flow controllers (MKS P4B with digital mass flows control unit model 247D). Graphite powder, H2SO4, ortho-phosphoric acid, potassium permanganate, H2O2 (30%), HCl, polyvinyl alcohol, potassium hydroxide, graphite sheet, nafion, and AC were purchased from TCI Chemicals India. Glassy carbon electrode was purchased from Sinsil International Pvt. Ltd.
Synthesis of Hexagonal Copper Phosphide Platelets
Usual techniques to synthesize transition metal phosphide is via plasma-enhanced CVD (PECVD) using PH3 plasma (Liang et al., 2017) or multistep hydrothermal techniques (Zhou et al., 2015; Ding et al., 2019) via metal oxide/hydroxide intermediate followed by thermal annealing. Using the PECVD approach restricts the phosphorization on the material surface rather than bulk conversion. On the other hand, hydrothermal growth may lead to polycrystalline phosphides and oxide intermediates that are difficult to avoid, which may possibly be responsible for degrading the electrochemical performance.
CVD has been shown as a one-step process where hexagonal Cu3P can be synthesized at an optimized temperature (Wei et al., 2016; Riyajuddin et al., 2019). In a typical experimental procedure, 50 mg of red phosphorus is kept in a quartz boat and then covered with a 0.25-mm-thick copper foil (the native oxide layer on Cu-foil is removed by sonication with 4 M HCl for 20 min and washed with distilled water and isopropanol and then dried) as shown in Supplementary Figure S1. Initially, there is no direct physical contact between the red phosphorus and the copper foil, which is positioned above the red phosphorus-containing boat. This quartz boat is inserted in the quartz tube and positioned at the center of the furnace and heated at 450°C for 5 min under a flow of Ar (25 sccm) at an ambient pressure condition. In this process, phosphorus particles sublimate which are diffused into the copper and reacted with it to nucleate Cu3P crystallites and then grow into hexagonal platelets. A layer of dark shining gray color is observed on the surface of the copper foil exposed to the red phosphorus environment.
The synthesis of Cu3P experiments is performed by using the “fast-heat” technique in which the samples are initially positioned outside the heated zone of the furnace with a fan blowing on the exposed quartz tube surface to keep the sample at room temperature (Itzhak et al., 2018; Kumar et al., 2018). Once the set temperatures of furnaces have reached a stable value, then the quartz tube is shifted to position the sample in the growth zone to start the phosphorization process. Using this technique, the sample is placed immediately to the required growth temperature and avoided exposure during the ramping steps.
Synthesis of the Three-Dimensional Graphene Matrix
The modified hydrothermal reduction method has been used for the synthesis of 3DG on top of the graphite sheet as reported in our earlier work (Singh et al., 2017). Briefly, a piece of graphite sheet is dipped into 2 mg/ml aqueous dispersion of graphene oxide. This aqueous dispersed solution of the graphene along with graphite sheets is freeze-dried at −20°C for 12 h. After that, the solution temperature is allowed to reach room temperature followed by the addition of 0.6 ml of 2 M ascorbic acid to the graphene oxide aqueous dispersion, and the mixture is sealed in a Teflon-lined autoclave and heated in an oven at 120°C for 6 h. The as-grown material is thoroughly dried in a vacuum for the formation of a porous 3DG-coated graphite electrode.
Assembly of a Solid-State Asymmetric Supercapacitor Device
The ASC devices are assembled by utilizing the Cu3P@3DG on graphite electrode as positive electrodes and AC on graphite as negative electrodes with polyvinyl alcohol (PVA)/KOH as gel electrolyte. The KOH/PVA gel electrolyte is prepared by mixing 5 g KOH and 4 g PVA in 50 ml distilled water with vigorous stirring at 90°C until the mixture solution becomes clear to form a semi gel state. Then, the prepared homogeneous 1 ml of each Cu3P solution [∼0.3, 0.6, 0.91, 1.2, 1.5 mg/ml in Isopropyl alcohol (IPA)] is drop casted on top of 3DG-graphite electrodes (1 cm2 × 1 cm2) homogeneously and dried. Each electrode is covered with ∼1.1 mg of 3DG matrix. After that, the gel electrolyte is sandwiched between Cu3P@3DG and AC electrodes, and the solid-state device is assembled successfully by moderate pressing to remove excess gel electrolytes and dried overnight. The active device area is 1 cm2 × 1 cm2, where longitudinally extended graphites are being used for making the connection for electrochemical measurement.
Results and Discussion
In this work, hexagonal platelets were directly grown on copper foil by thermal annealing using a CVD system. A known amount of red phosphorus was kept in a quartz boat and covered with copper foil as shown in Supplementary Figure S1. Red phosphorus and copper foil were then heated at 450°C for 5 min under argon flow. A dark shiny gray color layer that formed on the red phosphorus-exposed side of the copper foil indicated the successful growth of Cu3P. To investigate the effect of temperature on the morphology of Cu3P on copper foil, the same experiment was repeated at 350, 450, 600, and 800°C. When the temperature was increased to 600°C, the material started losing its hexagonal morphology by condensing hexagonal platelets together. Upon further enhancement of the temperature up to 800°C, the entire hexagonal morphology was lost. On the other hand, lowering the temperature to 350°C, the hexagonal platelets did not nucleate, as shown in Supplementary Figure S2. These results confirmed that a specific temperature (450°C) was required for the growth of hexagonal platelets. HRSEM analysis of the synthesized Cu3P revealed stacked platelets of hexagonal morphology with a lateral dimension in the range of micrometers and a thickness of a few nanometers, as shown in Figure 1A. HRSEM images and the corresponding elemental mapping patterns of Cu and P had demonstrated that the Cu and P elements were uniformly distributed on the hexagonal platelets (Supplementary Figures S3c,d in ESI). Transmission electron microscopy (TEM) analysis was performed for in-depth characterization. A low-magnification image, shown in Figure 1B, illustrated the nature of the hexagonal platelets. It was found that they consist of domains, although TEM sample preparation might influence the agglomeration (Supplementary Figure S4). The electron diffraction pattern taken from the agglomerate portion (Supplementary Figure S4a) was successfully indexed in terms of the hexagonal structure of the Cu3P. It should be noted that the rings on the polycrystalline electron diffraction pattern indicated a large misorientation between the domains in the agglomerate. Using HRTEM, we studied small nano-sized domains constituting the agglomerate and did selected area electron diffraction (SAED), shown in Figures 1C–D. The SAED pattern was taken along [0001] orientation of one domain, and the corresponding HRTEM image is shown in Supplementary Figure S4b. In Supplementary Figures S4b,c, HRTEM image and SAED patterns were taken along [101 –0] orientation of the domain fitting the atomic structure of Cu3P, as reported by Olofsson (1972).
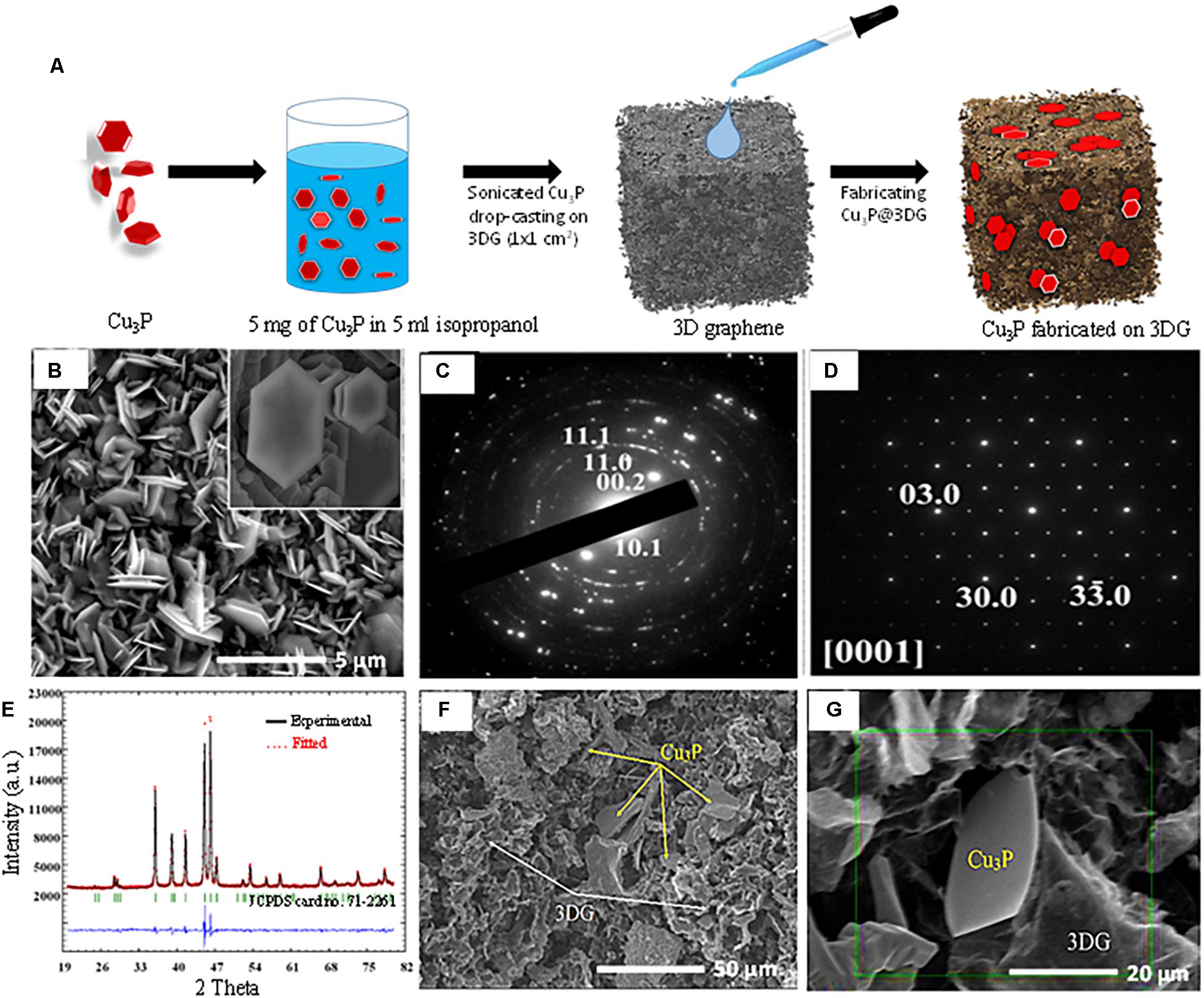
Figure 1. (A) Schematic representation of synthesis of three-dimensional graphene-decorated copper phosphide (Cu3P@3DG). (B) After synthesized Cu3P pallets, HR-SEM images of hexagonal Cu3P (hexagonal flake is shown inset). (C) Electron diffraction pattern taken from the agglomerate was indexed in terms of the hexagonal structure of the Cu3P. (D) Selected area electron diffraction (SAED) patterns taken along [0001] orientation of one domain. (E) Rietveld refinement of Cu3P: observed X-ray profile (open red circles), calculated profile (solid black line), and the difference between them (blue line on the bottom). Vertical bars refer to the calculated peak (JCPDS #71-2261). (F) Hybrid structure of hexagonal Cu3P platelets on the three-dimensional (3DG) template. (G) HR-SEM represents the hybrid Cu3P@3DG structure.
The phase content of the synthesized material was studied using X-ray diffraction (XRD) (Figure 1E). The XRD pattern exhibited strong, sharp peaks which were indexed in terms of hexagonal structure (P63cm) of the Cu3P (JCPDS card no. 71-2261) (Hou et al., 2016). Rietveld refinement resulted in reliability factors: Rp = 2.3%, Rwp = 3.27%, RBragg = 1.05%, and Rexp = 1.73%. These low-reliability values excluded the possibility of the presence of impurities. It should be noted that atomic positions, occupancies, and thermal displacement parameters of the Cu3P structure were not refined here, and the background was treated as linear. Lattice parameters were refined as a = 0.69673(1) nm and c = 0.71484(8) nm. The dependence of stoichiometry, temperature, and lattice parameters of the Cu3P structure was discussed in the earlier report (Olofsson, 1972). Since the refined lattice parameters (i.e., a = 0.697, c = 0.7145 nm) were fitted well with reported parameters (Olofsson, 1972), thus the synthesized material was stoichiometric (or closely stoichiometric) as Cu3P. To the best of our understanding, Rietveld refinement result of the as-synthesized sample was known to be the significant evidence for the formation of stoichiometric Cu3P rather than the energy-dispersive X-ray spectroscopy (EDS) shown in Supplementary Figure S3. The presence of elements could be found in the EDS analysis. However, it was hard to estimate the stoichiometry via EDS since a large difference in atomic numbers of the constituents did not allow accurate standard in the EDS analysis. Figure 1F showed the formation of a hybrid porous structure made of hexagonal Cu3P platelets along with 3DG. Careful observation indicated that the hexagonal Cu3P platelets (shown by yellow arrows) were randomly scattered on the porous foamy 3DG architecture (shown by white arrows). A close-up view of these hybrid structures, shown in Figure 1G, revealed a single flake of Cu3P trapped inside the 3DG matrix where a detailed EDS elemental mapping was performed to identify the distribution of Cu, P, and C elements, respectively, shown in Supplementary Figure S5.
The X-ray photoelectron spectroscopy (XPS) analysis of Cu3P elucidated the oxidation states and chemical interaction between Cu and red phosphorus (Supplementary Figure S6). The Cu, P, O, and C peaks appeared in the survey scan (shown in Supplementary Figure S6a). The presence of O1s peak indicated the superficial oxidation of Cu3P, while the carbon peak was due to the background. The high-resolution XPS spectrum of Cu 2p (Supplementary Figure S6b) exhibited two peaks at 932.4 and 934.8 eV, which were attributed to phosphorized copper (Cu–P) and oxidized copper (Cu–O), respectively (Lin et al., 2016; Jin et al., 2017). Supplementary Figure S6c showed double peaks in the phosphorus region. The peak at 133.6 eV was due to the oxidized phosphorus in the form of phosphate (Li et al., 2016), and 129.7 eV was the binding energy of P in Cu3P, which was lower than that of the binding energy of red phosphorus (130.2 eV), suggesting the conversion of copper to Cu3P (Pfeiffer et al., 2005). The presence of Cu–O and POx was probably due to oxide layer formation on the surface of Cu3P in ambient conditions.
To evaluate the as-grown nanomaterial as the promising electrode material, cyclic voltammetry (CV), galvanostatic charge–discharge (GCD), and electrochemical impendance spectroscopy (EIS) measurements were made to investigate the supercapacitive performance. The electrochemical window for CV and GCD measurements was kept in −0.5 to +0.5 V shown in Figures 2A,B. Figure 2A showed CV of the Cu3P nanoplatelets under a three-electrode system in 1 M Na2SO4 electrolyte at a different scan rate from 10 to 200 mV/s. The broad shape of the asymmetric CV curves observed for Cu3P nanoplatelets in the whole potential window indicated the presence of faradic behavior leading to pseudocapacitance along with electric double-layer capacitance (EDLC) existing simultaneously.
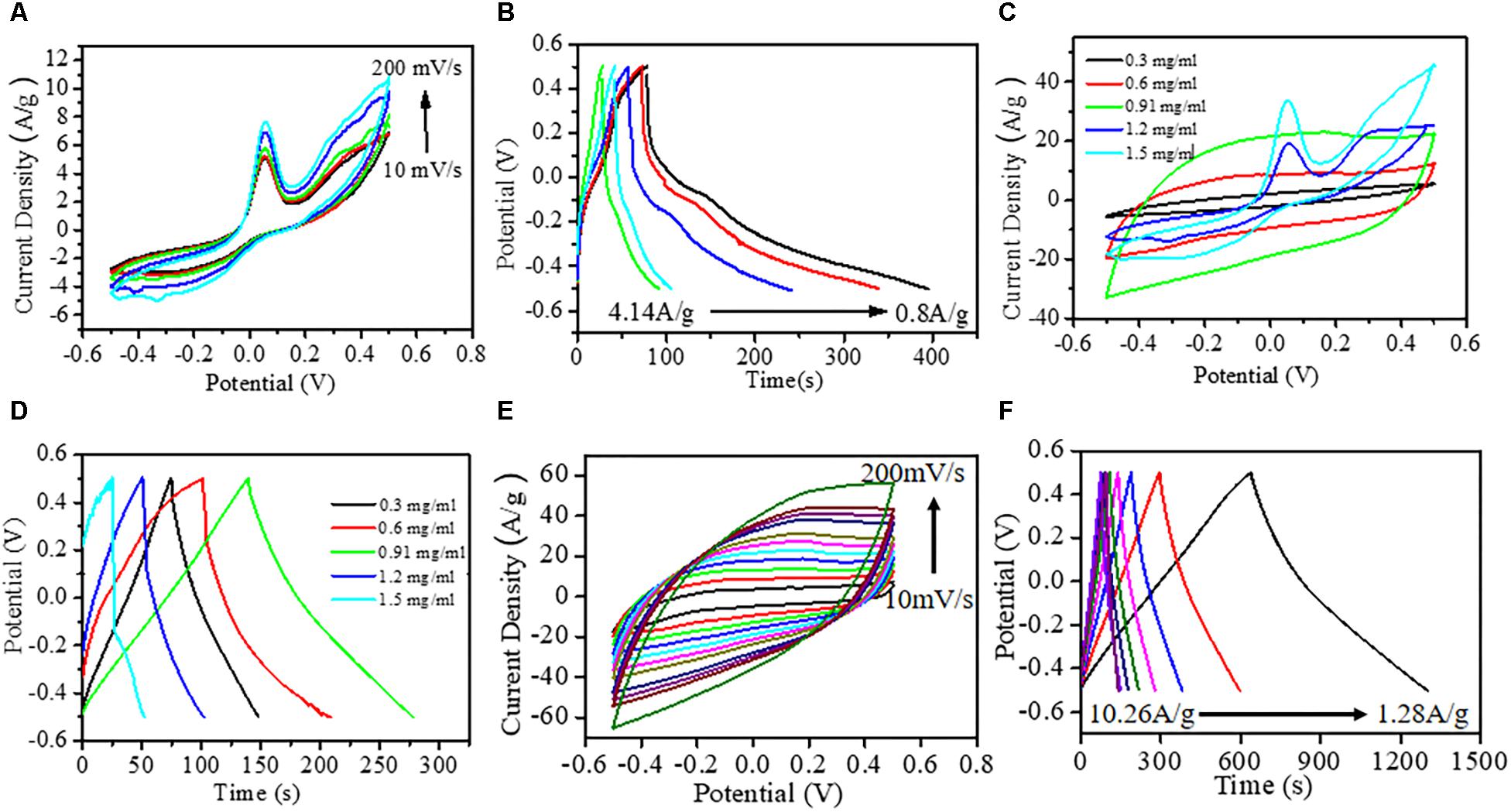
Figure 2. Electrochemical characterization. (A) Typical cyclic voltammetry (CV) curves of hexagonal copper phosphide (Cu3P) platelets on glassy carbon electrode at different scan rates (10, 50, 100, 150, and 200 mV/s). (B) Galvanostatic charge–discharge curves of Cu3P platelets at various current densities. (C) CV curves at 50 mV/s scan rates for different rational compositions (0.3, 0.6, 0.91, 1.2, and 1.5 mg/ml) of Cu3P on 3DG-graphite electrode. (D) Corresponding galvanostatic charge–discharge curves at 5.13 A/g current density. (E) Typical CV curves at different scan rates with the best weight ratio of 0.91 mg/ml of Cu3P on graphite electrode (10–200 mV/s). (F) Corresponding galvanostatic charge–discharge curves at various current densities.
The appearance of two humps in between −0.5 and +0.5 V corresponding to cathodic and anodic maxima indicated that the Cu3P platelets possess pseudocapacitive properties. These redox reactions attributed to the transition between metallic Cu(0) and Cu(I) species at an applied potential approximately −0.3 V vs. Ag/AgCl. While at the higher potential of 0.05 V vs. Ag/AgCl, the oxidation of Cu3P platelets to Cu(II) species was observed due to aqueous interaction (Figure 2A). Further, the broad anodic peak nearly at 0.3 V could be attributed to the formation of a hydrous oxide of copper which originated from the hydrated cupric oxide (Jayalakshmi and Balasubramanian, 2008), and the corresponding chemical reaction was shown in equation (3). Here, the potential window had been fixed to 0.5 V to avoid the formation of Cu(III) species. So far, the identification of intermediate species was hard to find due to the chemical transformation of Cu3P electrodes under electrochemical analysis, and the reaction mechanism was entirely unknown which required further detailed investigation. However, based on the available relevant literature, a possible reaction mechanism had been proposed in the following equations (Poli et al., 2016; Chen et al., 2017).
This mechanism could be attributed to redox reactions on the surface of Cu3P platelets. Pfeiffer et al. (2004) had explained that all the elements of the first transition metal series have partially filled 3d shells, except copper and zinc. Nonetheless, only the copper has a complete 3d shell and a single 4 s electron. Hence, copper was the only element in the series to have M+1 state with filled D-orbital in Cu3P platelets. The enhanced electrochemical performance might be attributed to the fact that the hexagonal Cu3P platelets were composed of many small particles, which had significantly increased the specific surface area and provided more reaction sites on the surface evident from the SEM image shown in Figures 1F,G.
To further improve the Cu3P electrode performance, we had designed a hybrid electrode with 3DG. The homogeneous distribution of Cu and P in hexagonal Cu3P flake and carbon in the foamy graphitic matrix had confirmed the formation of Cu3P@3DG hybrid network (shown in Supplementary Figure S5). For the development of effective electrode material, it was highly required to estimate the electrical conductivity of the proposed electrodes. The electrical conductivities of the Cu3P@3DG and 3DG electrodes via 4PP configuration with the electrode spacing of 2 mm were calculated to be 1,076 S m–1 and 978 S m–1, respectively. Here, the Cu3P@3DG electrode exhibited better conductivity as compared to the bare 3DG electrode on graphite substrate. This could be due to the metalloid property of Cu3P nanoparticles, shown in Supplementary Figure S7. To boost the kinetics of Cu3P, Cu3P@3DG had been elaborately designed, as schematically shown in Figure 1A. A systematic loading optimization of Cu3P on a 3DG matrix had been performed for extracting the best capacitive performance of Cu3P@3DG electrodes, as shown in Figures 2C,D. Here, the area under the CV curve was gradually increasing without any impressive redox peaks under the loading concentration of Cu3P that varied from 0.3 to 0.91 mg/ml. Optimizing the weight% of Cu3P in 3DG electrode, the redox probability of Cu3P on the 3DG matrix had been controlled until 0.91 mg/ml loading. However, increasing the loading concentration to 1.2 mg/ml and above, the redox peaks appeared more prominent at the same potential as bare Cu3P with increased current density due to the presence of the 3DG matrix. However, after a further increase in the concentration of Cu3P until 1.5 mg/ml, the prominent redox peaks appeared. This loading concentration of Cu3P on 3DG-graphite (0.91 mg/ml loading in 1 cm2 × 1 cm2) showed the best performance in galvanostatic charge–discharge curves at 5.13A/g current density (Figure 2D). For device fabrication as well as to evaluate the stability of the supercapacitor electrodes, the optimum loading concentration of Cu3P had been fixed to 0.91 mg/ml. The electrochemical CV curves and GCD performance (with different scan rates) of 3DG and bare Cu3P on graphite substrate are shown in Supplementary Figure S8. However, it had been observed that the Csp value of Cu3P on glassy carbon electrode (234.8 F/g) was slightly higher than that on graphite electrode (224 F/g) at a fixed current density of 1.28 A/g, which might be due to the interfacial interaction of electroactive material with the electrode substrate. The electrochemical analysis using this hybrid structure was carried out to evaluate its applicability toward energy storage devices. The Csp of Cu3P@3DG electrode was calculated to be 1,095.85, 901.50, 828.48, 778.70, 740.95, 706.66, 675.37, 590.95, 621.63, 597.45, 574.29, 495.97, and 421.65 F/g at the scan rate of 10, 20, 30, 40, 50, 60, 70, 80, 90, 100, 110, 150, and 200 mV/s, respectively from Figure 2E. Alternatively, the Csp extracted from the GCD measurements (Figure 2F) of the Cu3P@3DG electrode at various current density were calculated to be 849.81, 771.26, 734.35, 712.35, 692.39, 676.9, 659.98, and 644.75 F/g at 1.28, 2.56, 3.85, 5.13, 6.41, 7.69, 8.97, and 10.268 A/g, respectively (Supplementary Figure S9). The nearly isosceles triangular shape of the GCD (Figure 2F) implied excellent electron conductivity with tiny voltage drops at different current densities of Cu3P@3DG electrode. The energy and power density extracted from the charge and discharge analysis were about 118.1, 107.1, 102.0, 99.0, 96.2, 94.1, 91.7, and 89.5 Wh/kg and 641.0, 1,282.0, 1,923.0, 2,564.1, 3,205.2, 3,846.2, 4,487.2, and 7,132.2 W/kg at 1.28, 2.56, 3.85, 5.13, 6.41, 7.69, 8.97, and 10.268 A/g current density, respectively.
The comparative CV curves of the electrodes made of three different electroactive nanomaterials: Cu3P@3DG, 3DG, and bare Cu3P were carried at a scan rate of 20 mV/s (Figure 3A). The Csp of Cu3P@3DG, 3DG, and Cu3P electrodes were calculated to 901.5, 332.7, and 217.7 F/g (from CV curves), respectively, at a scan rate of 20 mV/s. Typically, the electrochemical properties like Csp of Cu3P@3DG were calculated to be nearly 2.5 times and four times enhanced as compared to bare 3DG and Cu3P-based electroactive materials, respectively. The results implied that Cu3P@3DG electrode referred to an excellent EDLC. The GCD measurements of the Cu3P@3DG, 3DG, and bare Cu3P on graphide electrodes at fixed current densities were performed with the potential window of 1.0 V (Figure 3B). The Cu3P@3DG exhibited a high Csp of 850 F/g (from GCD analysis) as compared to 3DG and bare Cu3P of 336 F/g and 224 F/g, respectively, at a current density of 1.28 A/g. Even at a very high current density of 10.27 A/g, the Csp reached up to 645 F/g which was about 76% of the capacitance retention compared to the current density of 1.28 A/g that invariably referred to the excellent stability of our electroactive material hetero-structure (shown in Figure 2F). The primary objective to incorporate 3DG along with Cu3P platelets was to provide high conductive channels for ion and electron transfer in the charging–discharging process leading to the improvement of the overall performance of hybrid electrodes (An et al., 2014). This hybrid structure could effectively minimize the unwanted agglomeration of Cu3P particles and simultaneously restricted the possibility of restacking of 3DG porous structure and thereby retaining the large surface area (Supplementary Figure S10), higher Csp, better rate capability, and overall device stability. However, the bare 3DG (made of three-dimensionally oriented rGO with very low oxygen content shown in Supplementary Figure S11) is known to be acting as promising anode materials due to high electronegativity rather than effective cathode material where graphene is difficult to get oxidized under the operating potential window (Hossain et al., 2012). CV analysis of bare glassy carbon and Cu3P decorated glassy carbon shown in Supplementary Figure S12.
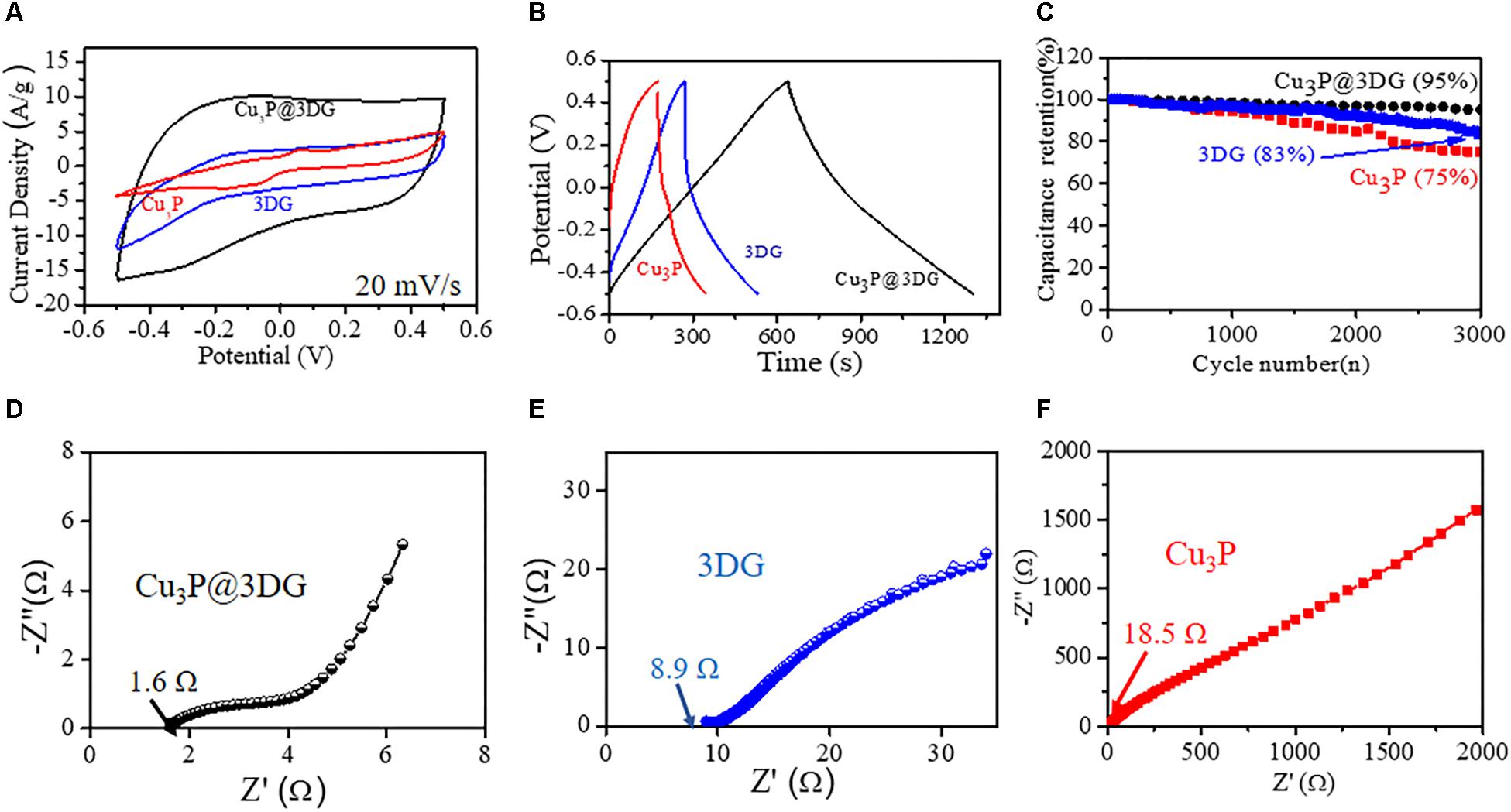
Figure 3. (A) Cyclic voltammetry (CV) curves of the three-dimensional graphene-decorated copper phosphide (Cu3P@3DG)-graphite (at 0.91 mg/ml), 3DG-graphite, and Cu3P-graphite at 20 mV/s. (B) Galvanostatic charge–discharge curves collected at a fixed current density of 1.28 A/g for Cu3P@3DG, 3DG, and Cu3P on graphite electrodes. (C) Cycling performance of Cu3P@3DG, 3DG, and Cu3P on graphite electrodes at 1.28 A/g current density. (D–F) Impedance Nyquist plots of the Cu3P@3DG, 3DG, and Cu3P on graphite electrodes, respectively.
The cycle stability had been evaluated for Cu3P@3DG, 3DG, and hexagonal Cu3P platelet-based electroactive materials on graphite substrate for its applicability in real phase energy storage devices. Figure 3C showed the variation of the Csp retention (%) as a function of the cycle number of the galvanostatic charge–discharge curves of the Cu3P@3DG, 3DG, and hexagonal Cu3P platelets at a fixed current density of 1.28 A/g. Surprisingly, after 3,000 cycles, the capacitive retention of Cu3P@3DG was found to be 95%, whereas that of 3DG was 83%. However, the stability degraded to 75% for bare Cu3P platelets. This gave us a clear understanding that the 3DG matrix was protecting Cu3P platelets from chemical degradation as well as agglomeration due to its high electrical and thermal conductivities. These excellent properties of 3DG in our nanohybrid materials had been further evaluated by characteristic electrochemical impedance study. Figures 3D–F showed the Nyquist plot of the Cu3P@3DG, bare 3DG, and Cu3P electrodes, respectively. In the spectrum, the equivalent series resistances of Cu3P@3DG, 3DG, and bare Cu3P on graphite electrodes were calculated to be 1.6, 8.9, and 18.5 Ω, respectively, which showed favorable conductivity (evident from the 4PP I-V characteristic, shown in Supplementary Figure S7) and very low internal resistance of Cu3P@3DG hybrid for high specific power density.
The asymmetric supercapacitor (ASC) device was fabricated to investigate the potential application of Cu3P@3DG nanohybrid as a positive electrode and AC as a negative electrode on a graphite substrate. The electrochemical performance of the ASC device was analyzed via CV at different scan rates, and GCD measurements were done at various current densities, as shown in Figures 4A,B. The potential window of the ASC Cu3P@3DG and AC electrodes was fixed to be 0–1 V. Figure 4A displayed the CV curves of the ASC device at different scan rates with a constant potential window, which showed rectangular like CV curves without redox peaks. It indicated the excellent reversibility and kinetics for electrochemical reactions over the ASC electrode. The Csp of the device was calculated to be 108.78, 61.65, 47.30, 39.86, and 35.09 F/g (217.56, 123.32, 94.61, 79.74, and 70.20 mF/cm2) at the scan rate of 10, 50, 100, 150, and 200 mV/s respectively. Alternatively, the Csp extracted from the GCD measurements of the device, as shown in Figure 4B, at various current densities were calculated to be 59.72, 51.82, 39.53, and 37.53 F/g (107.8, 94.33, 71.96, and 68.32 mF/cm2) at 0.5, 0.6, 0.7, and 0.8 mA/cm2 current densities, respectively. The energy and power densities were calculated to be 8.23, 7.2, 5.5, and 5.2 Wh/kg and 274.7, 329.7, 384.6, and 439.6 W/kg at 0.5, 0.6, 0.7, and 0.8 mA/cm2 current densities, respectively. To further elucidate the origin of high electrochemical performance, the electrochemical impedance spectrum (EIS) was carried out to examine the Cu3P@3DG//AC supercapacitor device. Figure 4C showed the Nyquist plot, the combined resistance Rs (the intrinsic, contact, and electrolyte resistance) of 4.24 Ω, the Cdl (the electric double layer capacitance) of 70.4 μF, and the Rct (the resistance of Faradic reaction) of 714 mΩ. The inclined line in the low-frequency region represented the Warburg impedance (W) of 19.2 mMho, and constant phase element (CF) 81.1 mMho. Simultaneously, the apparent straight-line nature of the plot in a low-frequency region described the ideal capacitive behavior of this prototype ASC device. The corresponding equivalent circuit was drawn based on the complex non-linear least-squares fitting method, shown as an inset of Figure 4C. Figure 4D displayed the Bode phase angle plot of Cu3P@3DG//AC. The absence of a horizontal segment in the Z-frequency plot at the low-frequency range revealed good charge-transfer behavior. Simultaneously, the high value of the phase angle curve manifested the great capacitive behavior of Cu3P@3DG//AC at the low-frequency range. Moreover, the long-term cycling stability performance was examined by GCD analysis at a current density of 0.7 mA/cm2. This supercapacitor device has demonstrated 82.5% capacitance retention after 5,500 cycles exhibiting outstanding cycling stability with 96% coulombic efficiency, as shown in Figure 4E. Figure 4F displayed the Ragone plot of ASC (Cu3P@3DG//AC) device. All these observations indicated that the Cu3P@3DG exhibited good electrochemical performance that could promote it to be an excellent material for its applicability in the next-generation energy storage device. In all the listed combination of metal phosphides, a direct comparison was not possible because of various factors like two-electrode experiment was performed in both solid and liquid state and the concentration and type of elecrolytes were different in all experimental conditions which had a prominent effect on the overall performance of the materials.
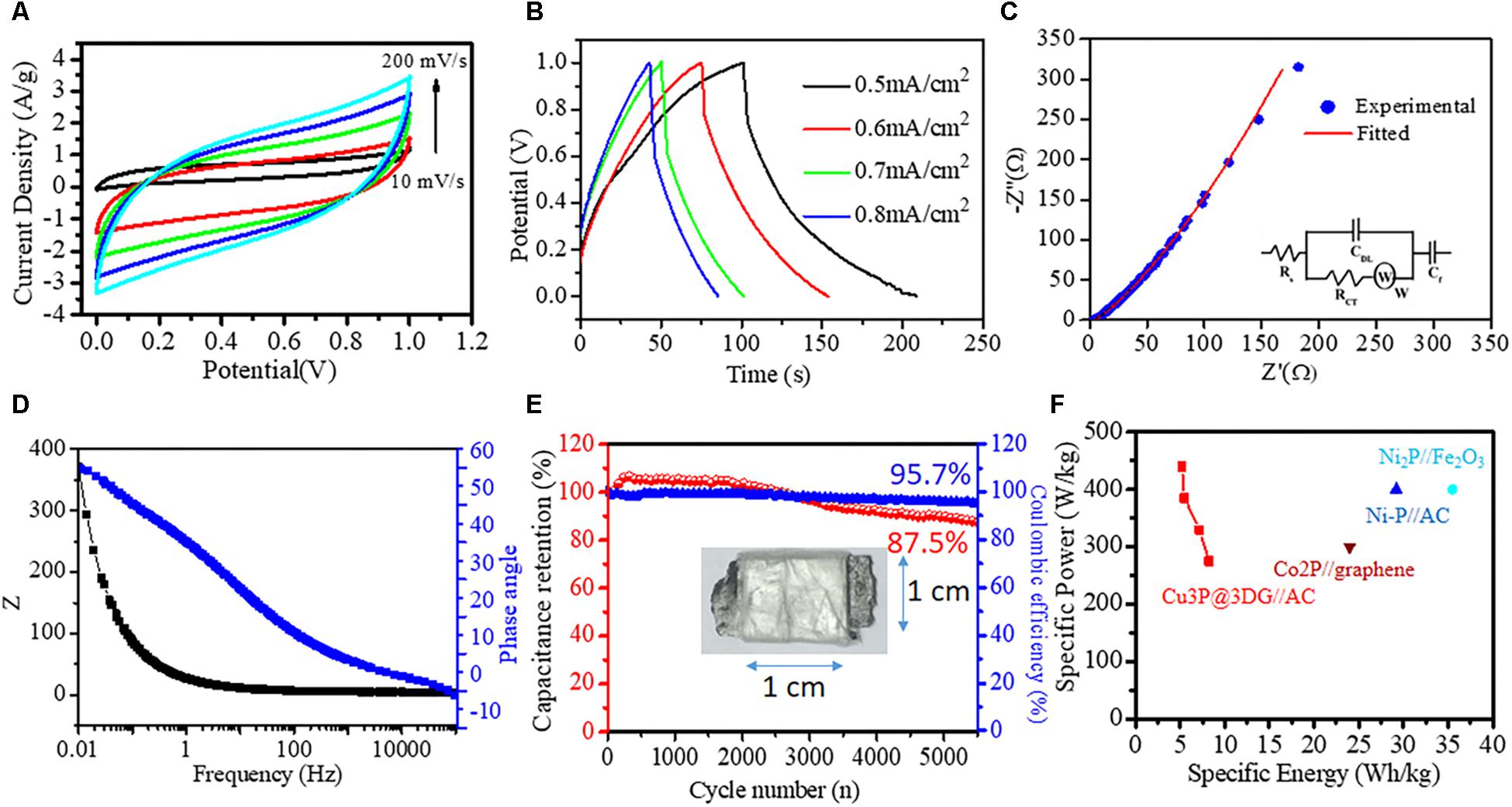
Figure 4. Solid-state asymmetric supercapacitor (ASC). (A) Cyclic voltammetry (CV) curves obtained at different scan rates (10, 50, 100, 150, and 200 mV/s). (B) Galvanostatic charge–discharge curves obtained at different current densities (0.5, 0.6, 0.7, and 0.8 mA/cm2). (C) Nyquist plot of the asymmetric device with the equivalent circuit as an inset. (D) Bode phase angle plot. (E) Capacitance retention (red ball-line) and coulombic efficiency (blue ball-line) of the assembled ASC device after 5,500 cycles; ASC device in the inset. (F) Ragone plots of ASC devices.
Table 1 depicts the transition metal phosphide-based active electrodes that so far had been tested as promising materials for supercapacitor devices. The synthesis of the metal phosphides with optimized nanostructures and composition was a real challenge. Although the reported specific capacitances of the active materials like Ni-P, Ni2P, CoP, Co2P, and Cu3P with different morphologies were quite high, but the working potential and capacitance retention were significantly low. However, it was found to be quite challenging to expand the working potential of the electrodes for practical applications. Here, we offered a strategy to synthesize hexagonal Cu3P platelets with 3DG as promising electrode materials for supercapacitors via controlling the directionality of the growth as well as engineering the facile interface between electrodes to electroactive materials which worked well in negative as well as positive working potentials.
Conclusion
In summary, the facile synthesis procedure of hexagonal Cu3P platelets and the fabrication of a heterostructure Cu3P with interwoven 3DG on graphite electrode (1 cm2 × 1 cm2) shows a simple way to design a supercapacitor device for practical applications. The Cu3P@3DG nanohybrid facilitates electron transfer and promotes kinetics with long cycling stability via lowering the internal resistance and providing electrochemical stability of Cu3P platelets. The high conductive channels for ions and electron transfer in the charging–discharging process leads to an enhancement of the overall device performance. The specific capacitance of the Cu3P@3DG is 849.81 F/g at a current density of 1.28 A/g and also exhibits superior cycling performance, with 95% retention of capacitance after 3,000 cycles at a current density of 1.28 A/g. In contrast, the solid-state asymmetric device shows the Csp of 109 F/g (from the CV) and 60 F/g (from GCD) with a capacitive retention of 82.5% and coulombic efficiency of 96% after 5,500 cycles, respectively. Due to the higher capacitance, lower cost, and excellent cycle stability of the Cu3P@3DG, it is anticipated that the material has potential for next-generation energy storage applications.
Data Availability Statement
All datasets generated for this study are included in the article/Supplementary Material.
Author Contributions
SBK designed the work. SSK and SA performed the experiments and characterizations. SSK and SR prepared the electrodes assembly of the samples and analyzed the electrochemical data. KG and GN participated in interpreting and analyzing all data and finalized the manuscript. GY and LM helped to characterize HRTEM and TEM analysis. All authors read and approved the final manuscript.
Funding
KG is thankful for the financial support from Nano Mission, Department of Science & Technology, India [Grant No: SR/NM/NS-91/2016 (G)]. GN would also like to thank the Israel Science Foundation, VATAT, and the Israel Prime Minister’s Office fuel alternative initiative for partial funding of this study under the Israel National Research Center for Electrochemical Propulsion (INREP).
Conflict of Interest
The authors declare that the research was conducted in the absence of any commercial or financial relationships that could be construed as a potential conflict of interest.
Acknowledgments
SBK would like to thank the Planning and Budgeting Committee of the Council for Higher Education for his PBC postdoctoral fellowship.
Supplementary Material
The Supplementary Material for this article can be found online at: https://www.frontiersin.org/articles/10.3389/fmats.2020.00030/full#supplementary-material
References
An, C., Wang, Y., Li, L., Qiu, F., Xu, Y., Xu, C., et al. (2014). Effects of highly crumpled graphene nanosheets on the electrochemical performances of pseudocapacitor electrode materials. Electrochim. Acta 133, 180–187. doi: 10.1016/j.electacta.2014.04.056
Augustyn, V., Simon, P., and Dunn, B. (2014). Pseudocapacitive oxide materials for high-rate electrochemical energy storage. Energy Environ. Sci. 7, 1597–1614. doi: 10.1039/c3ee44164d
Aziz, S. T., and Islam, R. U. (2018). Polymer-supported Cu–nanoparticle as an efficient and recyclable catalyst for oxidative homocoupling of terminal alkynes. Catal. Lett. 148, 205–213. doi: 10.1007/s10562-017-2237-8
Barry, B. M., Gillan, E. G., and Mater, R. E. C. (2008). Low-temperature solvothermal synthesis of phosphorus-rich transition-metal phosphides. Chem. Mater. 20, 2618–2620. doi: 10.1021/cm703095z
Brock, S. L., Perera, S. C., and Stamm, K. L. (2004). Chemical routes for production of transition-metal phosphides on the nanoscale: implications for advanced magnetic and catalytic materials. Chem. - A Eur. J. 10, 3364–3371. doi: 10.1002/chem.200305775
Brock, S. L., and Senevirathne, K. (2008). Recent developments in synthetic approaches to transition metal phosphide nanoparticles for magnetic and catalytic applications. J. Solid State Chem. 181, 1552–1559. doi: 10.1016/j.jssc.2008.03.012
Cao, J., Lei, C., Yang, B., Li, Z., Lei, L., Hou, Y., et al. (2019). Zeolitic imidazolate framework-derived core-shell-structured CoS 2/CoS2 -N-C supported on electrochemically exfoliated graphene foil for efficient oxygen evolution. Batter. Supercaps 2, 348–354. doi: 10.1002/batt.201800098
Chen, X., Cheng, M., Chen, D., and Wang, R. (2016). Shape-controlled synthesis of co2p nanostructures and their application in supercapacitors. ACS Appl. Mater. Interfaces 8, 3892–3900. doi: 10.1021/acsami.5b10785
Chen, Y., Zhang, W., Zhou, D., Tian, H., Su, D., Wang, C., et al. (2019). Co–Fe mixed metal phosphide nanocubes with highly interconnected-pore architecture as an efficient polysulfide mediator for lithium-sulfur batteries. ACS Nano 13, 4731–4741. doi: 10.1021/acsnano.9b01079
Chen, Y. C., Chen, Z. B., Lin, Y. G., and Hsu, Y. K. (2017). Synthesis of copper phosphide nanotube arrays as electrodes for asymmetric supercapacitors. ACS Sustain. Chem. Eng. 5, 3863–3870. doi: 10.1021/acssuschemeng.6b03006
Ding, L., Zhang, K., Chen, L., Yu, Z., Zhao, Y., Zhu, G., et al. (2019). Formation of three-dimensional hierarchical pompon-like cobalt phosphide hollow microspheres for asymmetric supercapacitor with improved energy density. Electrochim. Acta 299, 62–71. doi: 10.1016/j.electacta.2018.12.180
Fan, M., Chen, Y., Xie, Y., Yang, T., Shen, X., Xu, N., et al. (2016). Half-cell and full-cell applications of highly stable and binder-free sodium-ion batteries based on Cu3P nanowire anodes. Adv. Funct. Mater. 26, 5019–5027. doi: 10.1002/adfm.201601323
Han, A., Zhang, H., Yuan, R., Ji, H., and Du, P. (2017). Crystalline copper phosphide nanosheets as an efficient janus catalyst for overall water splitting. ACS Appl. Mater. Interfaces 9, 2240–2248. doi: 10.1021/acsami.6b10983
Hao, J., Yang, W., Huang, Z., and Zhang, C. (2016). Superhydrophilic and superaerophobic copper phosphide microsheets for efficient electrocatalytic hydrogen and oxygen evolution. Adv. Mater. Interfaces 3:1600236. doi: 10.1002/admi.201600236
Henkes, A. E., Vasquez, Y., and Schaak, R. E. (2007). Converting metals into phosphides: a general strategy for the synthesis of metal phosphide nanocrystals. J. Am. Chem. Soc. 129, 1896–1897. doi: 10.1021/ja068502l
Hossain, M. Z., Johns, J. E., Bevan, K. H., Karmel, H. J., Liang, Y. T., Yoshimoto, S., et al. (2012). Chemically homogeneous and thermally reversible oxidation of epitaxial graphene. Nat. Chem. 4, 305–309. doi: 10.1038/nchem.1269
Hou, C. C., Chen, Q. Q., Wang, C. J., Liang, F., Lin, Z., Fu, W. F., et al. (2016). Self-supported cedarlike semimetallic Cu3P nanoarrays as a 3D high-performance Janus electrode for both oxygen and hydrogen evolution under basic conditions. ACS Appl. Mater. Interfaces 8, 23037–23048. doi: 10.1021/acsami.6b06251
Itzhak, A., Teblum, E., Girshevitz, O., Okashy, S., Turkulets, Y., Burlaka, L., et al. (2018). Digenite (Cu9S5): layered p-type semiconductor grown by reactive annealing of copper. Chem. Mater. 30, 2379–2388. doi: 10.1021/acs.chemmater.8b00191
Jayalakshmi, M., and Balasubramanian, K. (2008). Cyclic voltammetric behavior of copper powder immobilized on paraffin impregnated graphite electrode in dilute alkali solution. Int. J. Electrochem. Sci. 3, 1277–1287.
Jin, Y., Zhao, C., Wang, Y., Jiang, Q., Ji, C., and Jia, M. (2017). Large-scale production of Cu3P nanocrystals for ultrahigh-rate supercapacitor. Ionics 23, 3249–3254. doi: 10.1007/s11581-017-2267-7
Kumar, S., Aziz, S. T., Girshevitz, O., and Nessim, G. D. (2018). One-step synthesis of N-Doped graphene quantum dots from chitosan as a sole precursor using chemical vapor deposition. J. Phys. Chem. C 122, 2343–2349. doi: 10.1021/acs.jpcc.7b05494
Kumar, S., Gonen, S., Friedman, A., Elbaz, L., and Nessim, G. D. (2017). Doping and reduction of graphene oxide using chitosan-derived volatile N-heterocyclic compounds for metal-free oxygen reduction reaction. Carbon N. Y. 120, 419–426. doi: 10.1016/j.carbon.2017.05.071
Li, D., Baydoun, H., Verani, C. N., and Brock, S. L. (2016). Efficient water oxidation using CoMnP nanoparticles. J. Am. Chem. Soc. 138, 4006–4009. doi: 10.1021/jacs.6b01543
Li, X., Elshahawy, A. M., Guan, C., and John, W. (2017). Metal phosphides and phosphates-based electrodes for electrochemical supercapacitors. Small 13:1701530. doi: 10.1002/smll.201701530
Li, Z., Ding, J., and Mitlin, D. (2015). Tin and tin compounds for sodium-ion battery anodes: phase transformations and performance. Acc. Chem. Res. 48, 1657–1665. doi: 10.1021/acs.accounts.5b00114
Liang, H., Xia, C., Jiang, Q., Gandi, A. N., Schwingenschlögl, U., and Alshareef, H. N. (2017). Low-temperature synthesis of ternary metal phosphides using plasma for asymmetric supercapacitors. Nano Energy 35, 331–340. doi: 10.1016/j.nanoen.2017.04.007
Lin, Y.-G., Hsu, Y.-K., Lin, Y.-C., Chang, Y.-H., Chen, S.-Y., and Chen, Y.-C. (2016). Synthesis of Cu2O nanoparticle films at room temperature for solar water splitting. J. Colloid Interface Sci. 471, 76–80. doi: 10.1111/poms.12938
Liu, S., He, X., Zhu, J., Xu, L., and Tong, J. (2016). Cu3P/RGO nanocomposite as a new anode for lithium-ion batteries. Sci. Rep. 6, 1–10. doi: 10.1038/srep35189
Liu, S., Li, S., Wang, J., Shi, Q., and Li, M. (2012). Surfactant-assisted synthesis and electrochemical performances of Cu 3 P dendrites. Mater. Res. Bull. 47, 3352–3356. doi: 10.1016/j.materresbull.2012.07.026
López-Ortega, A., Lottini, E., Fernández, C. D. J., and Sangregorio, C. (2015). Exploring the magnetic properties of cobalt-ferrite nanoparticles for the development of a rare-earth-free permanent magnet. Chem. Mater. 27, 4048–4056. doi: 10.1021/acs.chemmater.5b01034
Mukherjee, D., and Austeria, M. (2016). Two-dimensional, few-layer for electrochemical hydrogen evolution over phosphochalcogenide, FePS3: a new catalyst wide pH range. ACS Energy Lett. 1, 367–372. doi: 10.1021/acsenergylett.6b00184
Olofsson, O. (1972). The crystal structure of Cu3P. Acta Chem. Scand. 26, 2777–2787. doi: 10.3891/acta.chem.scand.26-2777
Pfeiffer, H., Tancret, F., Bichat, M. P., Monconduit, L., Favier, F., and Brousse, T. (2004). Air stable copper phosphide (Cu3P): a possible negative electrode material for lithium batteries. Electrochem. Commun. 6, 263–267. doi: 10.1016/j.elecom.2003.12.012
Pfeiffer, H., Tancret, F., and Brousse, T. (2005). Synthesis, characterization and electrochemical properties of copper phosphide (Cu3P) thick films prepared by solid-state reaction at low temperature: a probable anode for lithium-ion batteries. Electrochim. Acta 50, 4763–4770. doi: 10.1016/j.electacta.2005.02.024
Poli, F., Wong, A., Kshetrimayum, J. S., Monconduit, L., and Letellier, M. (2016). In situ NMR insights into the electrochemical reaction of Cu3P electrodes in lithium batteries. Chem. Mater. 28, 1787–1793. doi: 10.1021/acs.chemmater.5b04802
Riyajuddin, S. K., Aziz, S. K. T., Kumar, S., Nessim, G. D., and Ghosh, K. (2019). 3D-graphene decorated with g-C3N4/Cu3P composite: a noble metal-free bifunctional electrocatalyst for overall water splitting. ChemCatChem. doi: 10.1002/cctc.201902065
Seo, B., Baek, D. S., Sa, Y. J., and Joo, S. H. (2016). Shape effects of nickel phosphide nanocrystals on hydrogen evolution reaction. CrystEngComm 18, 6083–6089. doi: 10.1039/c6ce00985a
Shao, Y., Shi, X., and Pan, H. (2017). Electronic, magnetic, and catalytic properties of thermodynamically stable two-dimensional transition-metal phosphides. Chem. Mater. 29, 8892–8900. doi: 10.1021/acs.chemmater.7b03832
Singh, K., Kumar, S., Agarwal, K., Soni, K., Ramana Gedela, V., and Ghosh, K. (2017). Three-dimensional graphene with MoS 2 nanohybrid as potential energy storage/transfer device. Sci. Rep. 7:9458. doi: 10.1038/s41598-017-09266-2
Sivula, K., and Van De Krol, R. (2016). Semiconducting materials for photoelectrochemical energy conversion. Nat. Rev. Mater 1:15010. doi: 10.1038/natrevmats.2015.10
Sun, Z., Yue, Q., Li, J., Xu, J., Zheng, H., and Du, P. (2015). Copper phosphide modified cadmium sulfide nanorods as a novel p-n heterojunction for highly efficient visible-light-driven hydrogen production in water. J. Mater. Chem. A 3, 10243–10247. doi: 10.1039/c5ta02105g
Wang, D., Kong, L., Liu, M. C., Luo, Y. C., and Kang, L. (2015a). An approach to preparing Ni-P with different phases for use as supercapacitor electrode materials. Chem. A Eur. J. 21, 17897–17903. doi: 10.1002/chem.201502269
Wang, D., Kong, L., Liu, M., Zhang, W., and Luo, Y. (2015b). Amorphous Ni-P materials for high-performance pseudocapacitors. J. Power Sources 274, 1107–1113. doi: 10.1016/j.jpowsour.2014.10.179
Wang, X., Li, M., Chang, Z., Wang, Y., Chen, B., Zhang, L., et al. (2015c). Orientated Co 3 O 4 nanocrystals on MWCNTs as superior battery-type positive electrode material for a hybrid capacitor. J. Electrochem. Soc. 162, A1966–A1971. doi: 10.1149/2.0041511jes
Wang, J., Yang, Q., Zhang, Z., and Sun, S. (2010). Phase-controlled synthesis of transition-metal phosphide nanowires by ullmann-type reactions. Chem. A Eur. J. 16, 7916–7924. doi: 10.1002/chem.200902151
Wang, W., Zhang, L., Xu, G., Song, H., Yang, L., Zhang, C., et al. (2018). Structure-designed synthesis of CoP Microcubes from metal-organic frameworks with enhanced supercapacitor properties. Inorg. Chem. 57, 10287–10294. doi: 10.1021/acs.inorgchem.8b01524
Wei, S., Qi, K., Jin, Z., Cao, J., Zheng, W., Chen, H., et al. (2016). One-step synthesis of a self-supported copper phosphide nanobush for overall water splitting. ACS Omega 1, 1367–1373. doi: 10.1021/acsomega.6b00366
Wu, H., Ni, Y., Wang, M., and Lu, D. (2016). Shape-controlled synthesis and performance comparison of Ni2P nanostructures. CrystEngComm 18, 5155–5163. doi: 10.1039/c6ce00386a
Wu, J., Zhang, Q., Zhou, A., Huang, Z., Bai, H., and Li, L. (2016). Phase-separated polyaniline/graphene composite electrodes for high-rate electrochemical supercapacitors. Adv. Mater. 28, 10211–10216. doi: 10.1002/adma.201601153
Xue, S., Chen, L., Liu, Z., Cheng, H., and Ren, W. (2018). NiPS 3 nanosheet - graphene composites as highly efficient electrocatalysts for oxygen evolution reaction. ACS Nano 2018, 5297–5305. doi: 10.1021/acsnano.7b09146
Zhang, Y., Wang, G., Wang, L., Tang, L., Zhu, M., Wu, C., et al. (2019). Graphene-encapsulated CuP 2 : a promising anode material with high reversible capacity and superior rate-performance for sodium-ion batteries. Nano Lett 196, 2575–2582. doi: 10.1021/acs.nanolett.9b00342
Zhou, K., Zhou, W., Yang, L., Lu, J., Cheng, S., Mai, W., et al. (2015). Ultrahigh-performance pseudocapacitor electrodes based on transition metal phosphide nanosheets array via phosphorization: a general and effective approach. Adv. Funct. Mater. 25, 7530–7538. doi: 10.1002/adfm.201503662
Keywords: hexagonal copper phosphide platelets, chemical vapor deposition, red phosphorus, three-dimensional graphene (3DG), energy storage devices
Citation: Kumar S, Aziz SKT, Kumar S, Riyajuddin S, Yaniv G, Meshi L, Nessim GD and Ghosh K (2020) Three-Dimensional Graphene-Decorated Copper-Phosphide (Cu3P@3DG) Heterostructure as an Effective Electrode for a Supercapacitor. Front. Mater. 7:30. doi: 10.3389/fmats.2020.00030
Received: 28 October 2019; Accepted: 28 January 2020;
Published: 18 March 2020.
Edited by:
Federico Cesano, University of Turin, ItalyReviewed by:
David Aradilla, Commissariat à l’Energie Atomique et aux Energies Alternatives (CEA), FranceShuling Liu, Shaanxi University of Science and Technology, China
Copyright © 2020 Kumar, Aziz, Kumar, Riyajuddin, Yaniv, Meshi, Nessim and Ghosh. This is an open-access article distributed under the terms of the Creative Commons Attribution License (CC BY). The use, distribution or reproduction in other forums is permitted, provided the original author(s) and the copyright owner(s) are credited and that the original publication in this journal is cited, in accordance with accepted academic practice. No use, distribution or reproduction is permitted which does not comply with these terms.
*Correspondence: Louisa Meshi, bG1lc2hpLmxtQGdtYWlsLmNvbQ==; Gilbert D. Nessim, R2lsYmVydC5OZXNzaW1AYml1LmFjLmls; Kaushik Ghosh, a2F1c2hpa0BpbnN0LmFjLmlu
†These authors have contributed equally to this work