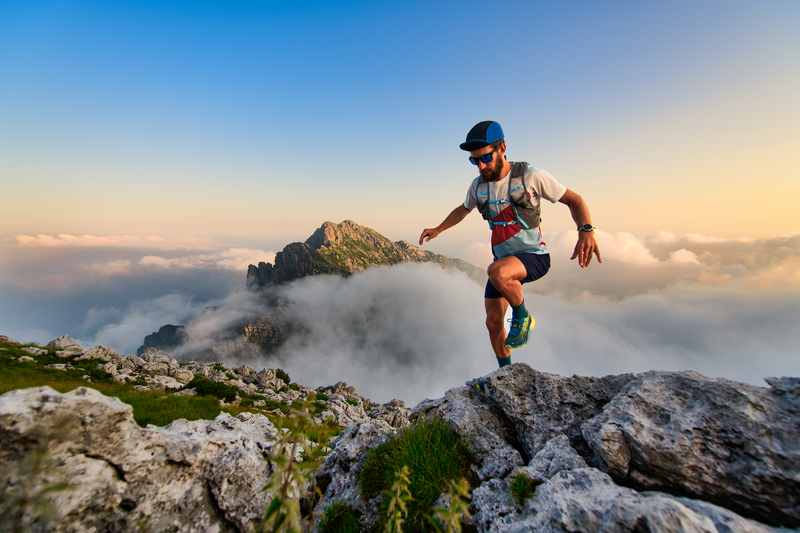
95% of researchers rate our articles as excellent or good
Learn more about the work of our research integrity team to safeguard the quality of each article we publish.
Find out more
PERSPECTIVE article
Front. Mater. , 07 February 2020
Sec. Polymeric and Composite Materials
Volume 7 - 2020 | https://doi.org/10.3389/fmats.2020.00025
This article is part of the Research Topic Biodegradable Matrices and Composites View all 25 articles
Since the introduction of tissue engineering as an encouraging method for the repair and regeneration of injured tissue, there have been many attempts by researchers to construct bio-mimetic scaffolds which mimic the native extracellular matrix, with the aim of promoting cell growth, cell proliferation, and restoration of the tissue's native functionality. Among the different materials and methods of scaffold fabrication, one particularly promising class of materials, hydrogels, has been extensively studied, with the inclusion of nano-scaled materials into hydrogels leading to the creation of an exciting new generation of nanocomposites, known as nanocomposite hydrogels. To closely mimic the native tissue behavior, scientists have recently focused on the functionalization of incorporated nanomaterials via chiral biomolecules, with reported results showing great potential. The current article aims to introduce a perspective of nano-scaled cellulose as a promising nanomaterial which can be multi-functionalized for the fabrication of nanocomposite hydrogels with applications in tissue engineering and drug delivery systems. This article also briefly reviews the recently reported literature on nanocomposite hydrogels incorporated with chiral functionalized nanomaterials. Such knowledge paves the path for the development of tailored hydrogels toward practical applications.
Tissue engineering using polymeric biomaterials has emerged as a promising and novel medical alternative to current transplantation therapies in the treatment of various diseases (Nezhad-Mokhtari et al., 2019). Transplantation poses several shortcomings such as the risk of immune rejection, infection, stress shielding, limitation in availability of proper tissues, long-lasting, and high-cost surgeries, disease-transmitting possibility, contaminations by bacteria, and incompatibility. The aforementioned limitations can potentially be overcome with tissue engineering techniques, with recent advances showing its great potential for repairing and regeneration of injured tissues (Xu et al., 2015).
At its core, tissue engineering is an approach involving the design of tissue constructs with the capability of mimicking native tissue in vitro. These constructs are subsequently implanted in vivo to regenerate damaged tissue functionality and to help millions of people who suffer from diseases, or impaired organs (Kock et al., 2012). This method combines scaffolds, cells, and growth factors in which the cells are cultured on the scaffold and grown. Subsequently, this tissue construct is implanted at the site of injury without the need for multiple surgeries, thereby reducing the costs, risks, and recovery time associated with conventional treatments (Mohammadzadehmoghadam and Dong, 2019). In general, extracellular matrix (ECM) as a hierarchical hybrid nanostructure, plays a pivotal role in cellular activities such as cell promotion and regulating the conditions under which cells can grow, migrate, proliferate, differentiate, and communicate with each other (Zagris, 2001). The adhesion of cells to the ECM is controlled by specific sites on glycoproteins including fibronectin, laminin, and collagen (Gullberg and Ekblom, 1995). Nanocomposite (NC) hydrogels incorporating functionalized nanomaterials (NMs) are therefore of tremendous research interest precisely because they can mimic and simulate the organization and characteristics of native ECM, such as having a nanoporous morphology and functionality for cellular activities (Schexnailder and Schmidt, 2009).
Understanding tissue organization from the molecular to the macroscopic level helps in the process of designing scaffolds (Zhang et al., 2005). Among the diverse techniques for producing tissue engineering scaffolds, the design and fabrication of hydrogels have shown attractive features and gained popularity among researchers. Hydrogels, with a three-dimensional (3D) structure, present the capability to absorb and retain large amounts of water molecules (Bakaic et al., 2015). Hydrogels can also be injected directly into the site of injury, circumventing the need for complex surgeries. There is also a further benefit of seamlessly integrating the engineered implant into the existing tissue, thereby preventing stress shielding and damaging the healthy surrounding tissue (Hu et al., 2019).
The essential requirements for tissue engineering scaffolds are biocompatibility, biodegradability, having an interconnected porous structure, adequate mechanical properties, and appropriate surface chemistry. Since hydrogels have the potential to maintain a large water content, they are highly biocompatible (Thürmer et al., 2014). In addition, due to the hydrogel's inherent flexible and soft structure, they cause minimal harm when exposed to body tissue. Furthermore, their highly permeable nature makes hydrogels an ideal scaffold to provide the required nutrient permeability to sustain cell growth. Such features make hydrogels an exceptional choice for bio-therapeutic applications.
The hydrogel degradation within the body can proceed via hydrolytical or enzymatical mechanisms in which the erosion of the scaffold occurs in bulk or at the surface of the hydrogel (Curvello et al., 2019). Bulk erosion is the predominant mechanism of hydrogel biodegradation which is attributable to the permeability and large water content present in hydrogels. The erosion type and degradation rate depend on the in vivo hydrogel characteristics and can be tuned for different applications. In general, the best in vivo performance of hydrogels can be achieved through optimizing the degradation rate via control over the polymer characteristics such as surface properties and degree of cross-linking (Tan and Marra, 2010; Bae et al., 2013; Tanan et al., 2019).
Although the biodegradability of the employed hydrogel for tissue engineering is a significant parameter, the scaffold's mechanical durability is considered the critical characteristic of scaffold materials with the target of long-term applications. The integrity of the implanted hydrogels may deteriorate when they are subjected to constant load due to wearing effects from repetitive body movements, leading to a reduction in their performance and a higher risk of infection. The created cavities or cracks provide locations for the intrusion of the bacteria and microorganisms, leading to acceleration in the scaffold failure It should be noted that a wide range of mechanical properties could be tailored through the changes in the concentration of the constituents, composition, and cross-linking density of the 3D network; however, manipulation of these could compromise the particular composition or chemistry of the hydrogels and reduce its targeted therapeutic effect. It follows then that these approaches have also shown some pitfalls, especially considering the difficulty in manipulating the mechanical properties in vivo, which also does not guarantee stable mechanical properties during the implant's lifetime. The missing link that could allow NC hydrogels approach to reach functional integrity could, therefore, lie in the preparation of the hydrogels with self-healing ability (Wang et al., 2018). Similar to other self-healing polymers, the self-healing hydrogels were developed based on two strategies—intrinsic and extrinsic, where the former has garnered more attention. The extrinsic strategy is applied by incorporation of the modified nanoparticles (Rao et al., 2019) and nanocellulose (Bai et al., 2019) or via the release of healing agents from ruptured micro/nanoscale capsules and fibers (Fang et al., 2013). The intrinsic self-healing ability of hydrogels is mostly induced through the physical non-covalent interactions and reversible chemical covalent bonds. The physical non-covalent interactions could include hydrogen bonding, metal-ligand coordination, hydrophobic interaction, and π-π stacking. This is in contrast with reversible chemical covalent bonds which provide hydrogels with the self-healing properties via Diel-Alder (DA) “click chemistry,” imine bonds, boronate-catechol complexation, and other dynamic reshuffling radical reactions (Hao et al., 2019; Jing et al., 2019; Tu et al., 2019). Figure 1A schematically illustrates the strategies for the preparation of self-healing hydrogels using intrinsic approaches (Tu et al., 2019).
Figure 1. (A) Intrinsic strategies for the preparation of self-healing hydrogels (Tu et al., 2019), adapted with permission from Elsevier. (B) Regulation of stem cell function and fate in a 3D hydrogel network with chiral motifs (Zheng et al., 2018), reprinted with permission from American Chemical Society (ACS). (C) Effect of l- and d-cysteine on single neuronal growth (Baranes et al., 2014), adapted with permission from American Chemical Society (ACS). (D) Nanocomposite alginate hydrogels incorporated with Dye-loaded periodic mesoporous organosilica particles functionalized with bioactive molecules (Kehr et al., 2013), adapted with permission from John Wiley & Sons. (E) Chemically functionalized periodic mesoporous organosilica particles/alginate 3D hydrogels as simultaneous ECM-model and drug delivery system (Kehr, 2016), adapted with permission from American Chemical Society (ACS).
Some advantageous features such as 3D porous structure, biocompatibility, and hydrophilicity, make hydrogels a suitable analog of natural tissue. However, due to their mechanical weakness upon dehydration, they possess only limited potential in bioapplications (Billiet et al., 2012). This shortcoming has been successfully addressed by developing NC hydrogels (Sanka et al., 2019) where certain organic/inorganic NMs are used to physically/chemically cross-link a water-soluble polymer and serve to enhance the mechanical strength of the polymeric matrix. In addition, inorganic NMs can boost the optical, magnetic, or biological properties of the 3D hybrid hydrogel (Gaharwar et al., 2014). The right selection, proper functionalization, and careful design of polymer/nanomaterial play crucial roles in developing a novel and advanced NC hydrogel for tissue engineering applications. From another perspective, due to the large surface area, NMs can effectively increase biological functionality, hence NC hydrogels have been shown to more closely mimic native tissues compared with free-NM hydrogels (Kehr et al., 2015). In the case of some specific applications, e.g., regeneration of the central nervous system, the addition of nanofibers to a hydrogel matrix can be morphologically beneficial because the elongated shape of the scaffold is favored by neural cells (Niemczyk et al., 2018).
NC hydrogels have been categorized based on their stimulus-sensitivity which includes thermo-responsive, light-responsive, electro-responsive, and magnetic-responsive hydrogels. In addition, they have been classified based on their applications, such as in sensors, catalysts, drug delivery systems, wound dressings, and tissue engineering (Song et al., 2015; Vashist et al., 2018). They are also classified according to the type of incorporated nanomaterials, which is the more common type of classification (Sharma et al., 2018; Vashist et al., 2018; Rafieian et al., 2019). Frequently reported nanofillers in NC hydrogels are silica such as nano-clay (Jin et al., 2018) and fumed silica (Kehr et al., 2013), carbon in the form of carbon nanotubes, graphene (Servant et al., 2014) and graphene oxide (GO) (Rasoulzadeh and Namazi, 2017; Tarashi et al., 2019), metal and metal oxide nanoparticles (Tan et al., 2019) such as gold, silver, iron oxide, titanium oxide (TiO2), nanohydroxyapatite, alumina and zirconia, and polymeric nanoparticles such as nano-scaled cellulose (NSC) (Dutta et al., 2019), including cellulose nanofibers, nanocrystals, and nanowhiskers. In general, depending on the surface chemistry of the nanomaterials, they can act as physical or chemical crosslinkers in NC hydrogels. Nanoparticles such as carbon, silica, and NSC, owing to the presence of hydroxyl groups on their surfaces, possess active surfaces for physical crosslinking such as hydrogen bonding. They could also serve as chemical crosslinkers in polymeric hydrogels containing reactive functional groups on their backbone, which would either require reaction initiators or coupling agents such as silanes. Some NMs without active surface chemistry, such as metals and metal oxides, need surface treatment or modification to act as a crosslinker within the NC hydrogel. In the following section, some recent advances on NC hydrogels are reviewed.
As a bone tissue engineering platform, injectable NC hydrogels from methacrylated glycol chitosan-montmorillonite were studied by Cui et al. (2019). In this work, the direct stem cell differentiation in a 3D micro-porous structure was addressed. The photocrosslinked chitosan/silicates NC hydrogels showed a six-fold Young's modulus increase. In addition, in vitro mesenchymal stem cell studies proved the NC hydrogel's efficacy in cell proliferation, promotion, and differentiation induction (Cui et al., 2019). In one contribution, Rasoulzadeh and Namazi developed bio-NC hydrogels based on carboxymethyl cellulose and GO with anticancer drug delivery potential (Rasoulzadeh and Namazi, 2017). The physically crosslinked (with FeCl3.6H2O) NC hydrogels were loaded with Doxorubicin as the anticancer drug to be delivered. In comparison with the neat carboxymethyl cellulose hydrogel, the incorporation of GO led to NC hydrogels with enhanced loading capacity and a lasting release of the loaded therapeutic drug. Most recently, the stimuli-responsive load-bearing double network NC hydrogels, based on κ-carrageenan (κ-Car)/polyacrylamide (PAm) crosslinked and reinforced with GO nanosheets, were fabricated (Tarashi et al., 2019). Compared to the non-loaded GO hydrogel, at 3 wt. % of GO nanosheets, optimum mechanical properties i.e., 21.7 MPa compression strength, 0.64 MPa ultimate tensile strength, 2,398% elongation at break, and 5.7 MJ/m3 fracture energy were obtained. These improvements, as well as the self-healing performance of NC hydrogels, were attributed to the synergistic influence of the reversible interactions due to bonding of the GO nanosheets between the κ-Car and PAm networks. In a novel and interesting piece of research, Toledo et al. explored the additional effect of low contents (<1.0 wt.%) of both non-functionalized, and functionalized TiO2 nanoparticles into poly (2-hydroxyethylmethacrylate) based physical NC hydrogels (Toledo et al., 2018). While pristine TiO2 resulted in higher swelling capacity and greater solvent diffusion coefficient, functionalized TiO2-filled NC hydrogels behaved contrarily. In addition, functionalized NC hydrogels exhibited improved ability in retaining aggregates of skeletal muscle cells (C2C12). Hydroxyapatite nanorods were also employed in the fabrication of chitosan NC hydrogels as potential scaffolds in cartilage regeneration (Kumar et al., 2019). In this contribution, Kumar et al. reported essential improvement in the mechanical properties of developed NC hydrogels at the optimum level (1.5 wt.%) of nanohydroxyapatite. Antimicrobial tests against three different microbes (Escherichia coli, Staphylococcus aureus bacteria, and Candida albicans fungi) demonstrated significant enhancement in the antimicrobial activity of the NC hydrogel. L929 fibroblast cell cultures (after 72 h) also confirmed 90% cell viability. In another novel work, Lee et al. (2018) investigated the application of functionalized gold nanoparticles (GNPs) in developing NC hydrogels for bone tissue engineering. The GNPs were modified with N-acetyl cysteine (G-NAC) and then incorporated into tryamine-gelatin (Gel-Ty) hydrogel. The fabricated NC hydrogel (Gel-Ty/G-NAC) supported human adipose-derived stem cell growth. Moreover, G-NAC had a crucial role in promoting osteo-differentiation and stimulating bone regeneration. This group suggested Gel-Ty/G-NAC hydrogels as a biodegradable platform in bone-related applications such as bone treatment, drug delivery, and cell delivery. In another effort, methoxy (polyethylene glycol)-polyalanine (mPA), as a di-block copolymer based thermo-responsive hydrogel, was incorporated by superparamagnetic Fe3O4 (osteoinductive) and hydroxyapatite (osteoconductive) nanoparticles in various contents (Huang and Chu, 2019). Modulation of bone differentiation bio-markers, as well as bone mineralization enhancement, was the result of the inclusion of such nanoparticle combinations into the polypeptide hydrogel. Recently, a fully physically crosslinked NC hydrogel based on polyacrylamide/cellulose nanofibers (PAM/CNF) was introduced (Niu et al., 2018). Initially, NC hydrogels were produced via radical polymerization and then further strengthened by ferric ions (from FeCl3) via ionic interactions between the ferric ions and carboxyl groups on CNF. Hydrogen bonding and ionic interactions induced dual physical crosslinks in NC hydrogels, which in turn led to structures with enhanced stiffness and toughness, rapid recovery, and self-healing abilities.
In recent years, the fabrication of NC hydrogels, including functionalized NMs has been a hot button research topic (Kehr, 2016; Zhao et al., 2017; Motealleh et al., 2018; Zheng et al., 2018). The aim of NM functionalization can be, for example, the improvement of cell-scaffold interactions, the creation of crosslinking between organic polymer and NM, drug delivery, or the development of the self-healing ability. Meanwhile, using chiral biomolecules to functionalize NMs has gained significant attention due to the various impacts on cell behaviors. Table 1 summarizes the research activities carried out on chirality functionalized systems i.e., NC hydrogels, hydrogels, and nanoparticles. In biological systems, the majority of the biologically significant molecules are chiral. For instance, natural proteins composed of laevorotatory (l-) amino acids. It is not yet known the reason why naturally-occurring proteins prefer only one of the chiral positions and under what criteria hemochorial molecules prefer selective interactions (Zheng et al., 2018).
In an attempt by Wang et al. to explore chiral effects on cell behavior, three chiral species including, l(d)-alanine, l(d)-valine, and l(d)-leucine were grafted onto silicon substrates (Wang et al., 2012). Fabricated chiral polymer brush films proved that chirality is effective for triggering cell differentiation and regulating multifold cell behaviors. In addition, l-films exhibited higher cytocompatibility. Zheng et al. reported the impact of chirality on function and fate of stem cells in fibrous hydrogels including self-assembled l-and d-form Fmoc-Phe-Phe-Cys networks which were photocrosslinked with poly (ethylene glycol) (l- and d-gel), respectively. Encapsulating human bone marrow-derived mesenchymal stem cells in hydrogels, it was found that cells tended to spread and grow in the l-gel (Figure 1B; Zheng et al., 2018). Using simultaneous opposite enantiomers in so-called Janus NC hydrogels was explored by Motealleh et al. (2018) to observe the reaction of healthy and cancer cells to a single biomaterial under the same condition. They used enantiomers of biodegradable poly-d (l)-lysine (PDL and PLL) to the functionalized external surface of zeolites and periodic mesoporous organosilicas (PMOs). Their findings showed higher affinity and migration of cells to the portion of the Janus NC hydrogel including the biopolymer enantiomer that the cells opt. In another contribution, Shefi et al. coated chiral d (l)-cysteine onto gold self-assembled monolayers and explored chirality influence on neuronal structure and adhesion (Figure 1C) (Baranes et al., 2014). Kehr et al. (2013) prepared PMOs/ biodegradable alginate-based NC hydrogels in which POMs were functionalized with cell adhesive bioactive tripeptide Arg-Gly-Asp (RGD) molecules (Kehr et al., 2013). The incorporation of PMOs only improved the mechanical properties of the alginate-based NC hydrogel marginally, however, the inclusion of biomolecule functionalized PMOs significantly increased the quantity of live cells which adhered to the NC hydrogel scaffold (Figure 1D). In another study, Kehr reported the development of multifunctional 3D NC hydrogels composed of enantiomerically functionalized PMOs [with amino acid d(l)-penicillamine (PEN) and carbohydrate mannose derivative d(l)-MAN)] and biodegradable alginate as ECM-model (for cell adhesion and cell enrichment) and drug delivery system (Figure 1E; Kehr, 2016). In another contribution, Au l(d)-penicillamine (Pen) was employed to modify plasmonic chiral nanoparticle films (PEN-NP films) by Zhao et al. (2017). It was found that l-PEN-NP films accelerated cell proliferation, while d-PEN-NP films behaved in the opposite manner. The preparation of functionalized zeolites and its effect on cell adhesion was also studied by Kehr et al. (2014).
Till date, a variety of NMs based on silica, carbon, gold, iron oxide, calcium phosphate, cellulose nanocrystals, and chitin whiskers have been used to develop mechanically strong biodegradable NC hydrogels with enhanced cell activities, and/or for drug release applications (Motealleh and Kehr, 2017). Among the various NMs, NSC has attracted great attention within recent years (Enayati et al., 2019). Since 2000 to the present, publications show significant growth in developing cellulose-based hydrogels for tissue engineering (Trache et al., 2017; Dutta et al., 2019). Besides the good biocompatibility and biodegradability, NSCs, derived from abundant and renewable resources, have high elastic moduli (110–220 GPa) and tensile strength (7.5–7.7 GPa), low density, high aspect ratio and surface area. These characteristics dramatically enhance their possible applications in the biomedical and tissue engineering fields. Usov et al. (2015) also studied structurally the rod-like cellulose nanoparticles and proved their right-handed chirality. Furthermore, due to the high concentration of hydroxyl groups on cellulose chains, the NSC's surface can be modified in myriad ways, i.e., oxidation, esterification, etherification, silylation, or polymer grafting—consequently, it can play multifunctional roles such as bioactive molecule container, reinforcing agent, and cross-linker. From another point of view, chemical isolation via sulfuric acid hydrolysis inserts negatively charged sulfate ester groups on the NSC's surface. This is a positive characteristic for NSC-functionalized NC hydrogels as they resemble native ECM sulfated glycosaminoglycans which stimulate and tune microscale cell functionalities (Domingues et al., 2015). In recent years, some notable research has been carried out on cellulose-based composite hydrogels. In the following paragraph, the most recent literature on research conducted on NSC-based NC hydrogels is highlighted.
Pereira et al. (2018) studied gellan-gum hydrogels reinforced by nanocellulose for the regeneration of annulus fibrous (AF) tissue. Upon nanocellulose incorporation, the compressive modulus of NC hydrogels approximated natural AF tissue. In vitro cell studies also demonstrated cell viability promotion. In another contribution, Phogat and Bandyopadhyay-Ghosh developed injectable bio-NC hydrogels of ultrafine fluorcanasite glass-ceramic particles incorporated into nanocellulose with enhanced stiffness, injectability, swelling behavior, and structural integrity (Phogat and Bandyopadhyay-Ghosh, 2018). Recently, Cheng et al. exploited chitosan-cellulose nanofiber self-healing hydrogels for neural regeneration. Improved neural differentiation and oxygen metabolism were observed upon embedding neural stem cells (Cheng et al., 2019). In other study by Li et al. (2018) polyacrylamide grafted cellulose nanocrystals (CNC-g-PAM), as physical crosslinkers and interfacial compatible nanofillers, were incorporated into poly(acrylic acid) to develop NC hydrogels. NC hydrogels showed significant improvement in tensile properties and self-recovery ability compared to the pure poly(acrylic acid) hydrogel. In a new effort to develop self-healing cellulose NC hydrogels, Xiao et al. (2019) exploited acylhydrazine-terminated polyethylene glycol to crosslink dialdehyde cellulose nanocrystals. Apart from an increase in the tensile and compressive strength of the hydrogel, self-healing efficiency, and biocompatibility of the hydrogel improved by 90 and 100%, respectively. Three-dimensional bioprinted constructs composed of either alginate/cellulose nanocrystals or alginate/cellulose nanocrystals containing fibroblast and hepatoma cells, namely bioinks, were introduced by Wu et al. as liver-mimetic platforms (Wu et al., 2018). The bioprinted constructs were crosslinked by CaCl2. It was reported that prepared hydrogels had shear storage moduli of 8–300 Pa and cell viability higher than 67%. The NC hydrogels of polyurethane/cellulose nanofibers were also fabricated by Chen et al., through the bioprinting process (Chen et al., 2019). The 3D-printed NC hydrogels exhibited a compression storage modulus of ~1.57 MPa and demonstrated excellent mouse and human fibroblast cell proliferation. In addition, taking into account the noteworthy features of NSCs, recently, self-healing hydrogels have been developed by the incorporation of cellulose nanocrystals within the poly(acrylic acid) mediated hydrogels. It was shown that incorporation of surface-modified CNC resulted in self-healing properties with up to ~90% self-healing efficiency (Bai et al., 2019).
This article reviews the progress in the development of biodegradable nanocomposite (NC) hydrogels with an emphasis on the chiral functionalized nanomaterials to provide a perspective for future research in the field. Due to the outstanding features of nano-scaled cellulose (NSC), one promising future direction of chiral functionalized NC hydrogels can be the application of this renewable, cheap, biocompatible, and biodegradable nanomaterial in developing the next generation of biotherapeutic materials. There is also much potential in the development of multi-functionalized NSC. On the one hand, it can function as a physical and chemical crosslinker in the fabrication of NC hydrogels and act as a reinforcing agent due to its significant mechanical strength. On the other hand, its surface modification with chiral biomolecules will allow the fabrication of novel biodegradable NC hydrogels as an advanced model implant for both tissue engineering and drug delivery systems. Furthermore, with some chemical modifications on the NSC, it would be possible to induce self-healing ability in the prepared hydrogels for autonomous self-healing and minimum invasive implantation.
RE, ME, and ZP summarized literature and wrote a major part of the manuscript. PS and SR conducted deep review, editing, guidance, and supervision. All authors have read and approved the article for publication.
The authors declare that the research was conducted in the absence of any commercial or financial relationships that could be construed as a potential conflict of interest.
The authors would like to appreciate Jeremy Kong Yoong Lee for his helpful advice and comments.
Bae, K. H., Wang, L.-S., and Kurisawa, M. (2013). Injectable biodegradable hydrogels: progress and challenges. J. Mater. Chem. 1, 5371–5388. doi: 10.1039/c3tb20940g
Bai, L., Jiang, X., Sun, Z., Pei, Z., Ma, A., Wang, W., et al. (2019). Self-healing nanocomposite hydrogels based on modified cellulose nanocrystals by surface-initiated photoinduced electron transfer ATRP. Cellulose 26, 5305–5319. doi: 10.1007/s10570-019-02449-2
Bakaic, E., Smeets, N. M. B., and Hoare, T. (2015). Injectable hydrogels based on poly(ethylene glycol) and derivatives as functional biomaterials. RSC Adv. 5, 35469–35486. doi: 10.1039/C4RA13581D
Baranes, K., Moshe, H., Alon, N., Schwartz, S., and Shefi, O. (2014). Neuronal growth on l- and d-cysteine self-assembled monolayers reveals neuronal chiral sensitivity. ACS Chem. Neurosci. 5, 370–376. doi: 10.1021/cn500015s
Benson, K., Galla, H. J., and Kehr, N. S. (2014). Cell adhesion behavior in 3D hydrogel scaffolds functionalized with D-or L-aminoacids. Macromol. Biosci. 14, 793–798. doi: 10.1002/mabi.201300485
Billiet, T., Vandenhaute, M., Schelfhout, J., Van Vlierberghe, S., and Dubruel, P. (2012). A review of trends and limitations in hydrogel-rapid prototyping for tissue engineering. Biomaterials 33, 6020–6041. doi: 10.1016/j.biomaterials.2012.04.050
Chen, R.-D., Huang, C.-F., and Hsu, S.-H. (2019). Composites of waterborne polyurethane and cellulose nanofibers for 3D printing and bioapplications. Carbohydr. Polym. 212, 75–88. doi: 10.1016/j.carbpol.2019.02.025
Cheng, K.-C., Huang, C.-F., Wei, Y., and Hsu, S.-H. (2019). Novel chitosan–cellulose nanofiber self-healing hydrogels to correlate self-healing properties of hydrogels with neural regeneration effects. NPG Asia Mater. 11:25. doi: 10.1038/s41427-019-0124-z
Cui, Z.-K., Kim, S., Baljon, J. J., Wu, B. M., Aghaloo, T., and Lee, M. (2019). Microporous methacrylated glycol chitosan-montmorillonite nanocomposite hydrogel for bone tissue engineering. Nat. Commun. 10:3523. doi: 10.1038/s41467-019-11511-3
Curvello, R., Raghuwanshi, V. S., and Garnier, G. (2019). Engineering nanocellulose hydrogels for biomedical applications. Adv. Colloid Interface Sci. 267, 47–61. doi: 10.1016/j.cis.2019.03.002
Domingues, R. M., Silva, M., Gershovich, P., Betta, S., Babo, P., Caridade, S. G., et al. (2015). Development of injectable hyaluronic acid/cellulose nanocrystals bionanocomposite hydrogels for tissue engineering applications. Bioconjug. Chem. 26, 1571–1581. doi: 10.1021/acs.bioconjchem.5b00209
Dutta, S. D., Patel, D. K., and Lim, K.-T. (2019). Functional cellulose-based hydrogels as extracellular matrices for tissue engineering. J. Biol. Eng. 13:55. doi: 10.1186/s13036-019-0177-0
Enayati, M. S., Neisiany, R. E., Sajkiewicz, P., Behzad, T., Denis, P., and Pierini, F. (2019). Effect of nanofiller incorporation on thermomechanical and toughness of poly (vinyl alcohol)-based electrospun nanofibrous bionanocomposites. Theor. Appl. Fract. Mech. 99, 44–50. doi: 10.1016/j.tafmec.2018.11.006
Fang, Y., Wang, C.-F., Zhang, Z.-H., Shao, H., and Chen, S. (2013). Robust self-healing hydrogels assisted by cross-linked nanofiber networks. Sci. Rep. 3:2811. doi: 10.1038/srep02811
Gaharwar, A. K., Peppas, N. A., and Khademhosseini, A. (2014). Nanocomposite hydrogels for biomedical applications. Biotechnol. Bioeng. 111, 441–453. doi: 10.1002/bit.25160
Gullberg, D., and Ekblom, P. (1995). Extracellular matrix and its receptors during development. Int. J. Dev. Biol. 39, 845–854.
Hao, Y., Zhou, X., Shao, J., and Zhu, Y. (2019). The influence of multiple fillers on friction and wear behavior of epoxy composite coatings. Surf. Coat. Tech. 362, 213–219. doi: 10.1016/j.surfcoat.2019.01.110
Hu, X., Gao, Z., Tan, H., Wang, H., Mao, X., and Pang, J. (2019). An injectable hyaluronic acid-based composite hydrogel by DA click chemistry with pH sensitive nanoparticle for biomedical application. Front. Chem. 7:477. doi: 10.3389/fchem.2019.00477
Huang, W.-S., and Chu, I.-M. (2019). Injectable polypeptide hydrogel/inorganic nanoparticle composites for bone tissue engineering. PLoS ONE 14:e0210285. doi: 10.1371/journal.pone.0210285
Jin, Y., Shen, Y., Yin, J., Qian, J., and Huang, Y. (2018). Nanoclay-based self-supporting responsive nanocomposite hydrogels for printing applications. ACS Appl. Mater. Interfaces 10, 10461–10470. doi: 10.1021/acsami.8b00806
Jing, Z., Xu, A., Liang, Y.-Q., Zhang, Z., Yu, C., Hong, P., et al. (2019). Biodegradable Poly(acrylic acid-co-acrylamide)/Poly(vinyl alcohol) double network hydrogels with tunable mechanics and high self-healing performance. Polymers 11:952. doi: 10.3390/polym11060952
Kehr, N. S. (2016). Enantiomorphous periodic mesoporous organosilica-based nanocomposite hydrogel scaffolds for cell adhesion and cell enrichment. Biomacromolecules 17, 1117–1122. doi: 10.1021/acs.biomac.5b01739
Kehr, N. S., Atay, S., and Ergün, B. (2015). Self-assembled monolayers and nanocomposite hydrogels of functional nanomaterials for tissue engineering applications. Macromol. Biosci. 15, 445–463. doi: 10.1002/mabi.201400363
Kehr, N. S., Ergün, B., Lülf, H., and De Cola, L. (2014). Spatially controlled channel entrances functionalization of zeolites L. Adv. Mater. 26, 3248–3252. doi: 10.1002/adma.201305745
Kehr, N. S., Prasetyanto, E. A., Benson, K., Ergün, B., Galstyan, A., and Galla, H. J. (2013). Periodic mesoporous organosilica-based nanocomposite hydrogels as three-dimensional scaffolds. Angew. Chem. Int. Ed. 52, 1156–1160. doi: 10.1002/anie.201206951
Kock, L., Van Donkelaar, C. C., and Ito, K. (2012). Tissue engineering of functional articular cartilage: the current status. Cell Tissue Res. 347, 613–627. doi: 10.1007/s00441-011-1243-1
Kumar, B. Y. S., Isloor, A. M., Kumar, G. C. M., Inamuddin and Asiri, A. M. (2019). Nanohydroxyapatite reinforced chitosan composite hydrogel with tunable mechanical and biological properties for cartilage regeneration. Sci. Rep. 9:15957. doi: 10.1038/s41598-019-52042-7
Lee, D., Heo, D. N., Nah, H. R., Lee, S. J., Ko, W.-K., Lee, J. S., et al. (2018). Injectable hydrogel composite containing modified gold nanoparticles: implication in bone tissue regeneration. Int. J. Nanomed. 13:7019–7031. doi: 10.2147/IJN.S185715
Li, B., Zhang, Y., Wu, C., Guo, B., and Luo, Z. (2018). Fabrication of mechanically tough and self-recoverable nanocomposite hydrogels from polyacrylamide grafted cellulose nanocrystal and poly(acrylic acid). Carbohydr. Polym. 198, 1–8. doi: 10.1016/j.carbpol.2018.06.047
Mohammadzadehmoghadam, S., and Dong, Y. (2019). Fabrication and characterization of electrospun silk fibroin/Gelatin Scaffolds crosslinked with glutaraldehyde vapor. Front. Mater. 6:91. doi: 10.3389/fmats.2019.00091
Motealleh, A., Hermes, H., Jose, J., and Kehr, N. S. (2018). Chirality-dependent cell adhesion and enrichment in Janus nanocomposite hydrogels. Nanomedicine 14, 247–256. doi: 10.1016/j.nano.2017.10.014
Motealleh, A., and Kehr, N. S. (2017). Nanocomposite hydrogels and their applications in tissue engineering. Adv. Healthc. Mater. 6:1600938. doi: 10.1002/adhm.201600938
Nezhad-Mokhtari, P., Ghorbani, M., Roshangar, L., and Soleimani Rad, J. (2019). A review on the construction of hydrogel scaffolds by various chemically techniques for tissue engineering. Eur. Polym. J. 117, 64–76. doi: 10.1016/j.eurpolymj.2019.05.004
Niemczyk, B., Sajkiewicz, P., and Kolbuk, D. (2018). Injectable hydrogels as novel materials for central nervous system regeneration. J. Neural Eng. 15:051002. doi: 10.1088/1741-2552/aacbab
Niu, J., Wang, J., Dai, X., Shao, Z., and Huang, X. (2018). Dual physically crosslinked healable polyacrylamide/cellulose nanofibers nanocomposite hydrogels with excellent mechanical properties. Carbohydr. Polym. 193, 73–81. doi: 10.1016/j.carbpol.2018.03.086
Pereira, D. R., Silva-Correia, J., Oliveira, J. M., Reis, R. L., Pandit, A., and Biggs, M. J. (2018). Nanocellulose reinforced gellan-gum hydrogels as potential biological substitutes for annulus fibrosus tissue regeneration. Nanomedicine 14, 897–908. doi: 10.1016/j.nano.2017.11.011
Phogat, K., and Bandyopadhyay-Ghosh, S. (2018). Nanocellulose mediated injectable bio-nanocomposite hydrogel scaffold-microstructure and rheological properties. Cellulose 25, 5821–5830. doi: 10.1007/s10570-018-2001-2
Rafieian, S., Mirzadeh, H., Mahdavi, H., and Masoumi, M. E. (2019). A review on nanocomposite hydrogels and their biomedical applications. Sci. Eng. Compos. Mater. 26, 154–174. doi: 10.1515/secm-2017-0161
Rao, Z., Liu, S., Wu, R., Wang, G., Sun, Z., Bai, L., et al. (2019). Fabrication of dual network self-healing alginate/guar gum hydrogels based on polydopamine-type microcapsules from mesoporous silica nanoparticles. Int. J. Biol. Macromol. 129, 916–926. doi: 10.1016/j.ijbiomac.2019.02.089
Rasoulzadeh, M., and Namazi, H. (2017). Carboxymethyl cellulose/graphene oxide bio-nanocomposite hydrogel beads as anticancer drug carrier agent. Carbohydr. Polym. 168, 320–326. doi: 10.1016/j.carbpol.2017.03.014
Sanka, R. V. S. P., Krishnakumar, B., Leterrier, Y., Pandey, S., Rana, S., and Michaud, V. (2019). Soft self-healing nanocomposites. Front. Mater. 6:137. doi: 10.3389/fmats.2019.00137
Schexnailder, P., and Schmidt, G. (2009). Nanocomposite polymer hydrogels. Colloid Polym. Sci. 287, 1–11. doi: 10.1007/s00396-008-1949-0
Servant, A., Leon, V., Jasim, D., Methven, L., Limousin, P., Fernandez-Pacheco, E. V., et al. (2014). Graphene-based electroresponsive scaffolds as polymeric implants for on-demand drug delivery. Adv. Healthc. Mater. 3, 1334–1343. doi: 10.1002/adhm.201400016
Sharma, G., Thakur, B., Naushad, M., Kumar, A., Stadler, F. J., Alfadul, S. M., et al. (2018). Applications of nanocomposite hydrogels for biomedical engineering and environmental protection. Environ. Chem. Lett. 16, 113–146. doi: 10.1007/s10311-017-0671-x
Song, F., Li, X., Wang, Q., Liao, L., and Zhang, C. (2015). Nanocomposite hydrogels and their applications in drug delivery and tissue engineering. J. Biomed. Nanotechnol. 11, 40–52. doi: 10.1166/jbn.2015.1962
Tan, H., and Marra, K. G. (2010). Injectable, biodegradable hydrogels for tissue engineering applications. Materials 3, 1746–1767. doi: 10.3390/ma3031746
Tan, H.-L., Teow, S.-Y., and Pushpamalar, J. (2019). Application of metal nanoparticle-hydrogel ydrogel composites in tissue regeneration. Bioengineering 6:E17. doi: 10.3390/bioengineering6010017
Tanan, W., Panichpakdee, J., and Saengsuwan, S. (2019). Novel biodegradable hydrogel based on natural polymers: Synthesis, characterization, swelling/reswelling and biodegradability. Eur. Polym. J. 112, 678–687. doi: 10.1016/j.eurpolymj.2018.10.033
Tarashi, S., Nazockdast, H., and Sodeifian, G. (2019). Reinforcing effect of graphene oxide on mechanical properties, self-healing performance and recoverability of double network hydrogel based on κ-carrageenan and polyacrylamide. Polymer 183:121837. doi: 10.1016/j.polymer.2019.121837
Thürmer, M. B., Diehl, C. E., Brum, F. J. B., and Santos, L. A. D. (2014). Preparation and characterization of hydrogels with potential for use as biomaterials. Mater. Res. 17, 109–113. doi: 10.1590/1516-1439.223613
Toledo, L., Racine, L., Pérez, V., Henríquez, J. P., Auzely-Velty, R., and Urbano, B. F. (2018). Physical nanocomposite hydrogels filled with low concentrations of TiO2 nanoparticles: swelling, networks parameters and cell retention studies. Mater. Sci. Eng. C Mater. Biol. Appl. 92, 769–778. doi: 10.1016/j.msec.2018.07.024
Trache, D., Hussin, M. H., Haafiz, M. M., and Thakur, V. K. (2017). Recent progress in cellulose nanocrystals: sources and production. Nanoscale 9, 1763–1786. doi: 10.1039/C6NR09494E
Tu, Y., Chen, N., Li, C., Liu, H., Zhu, R., Chen, S., et al. (2019). Advances in injectable self-healing biomedical hydrogels. Acta Biomater. 90, 1–20. doi: 10.1016/j.actbio.2019.03.057
Usov, I., Nyström, G., Adamcik, J., Handschin, S., Schütz, C., Fall, A., et al. (2015). Understanding nanocellulose chirality and structure–properties relationship at the single fibril level. Nat. Commun. 6:7564. doi: 10.1038/ncomms8564
Vashist, A., Kaushik, A., Ghosal, A., Bala, J., Nikkhah-Moshaie, R., A Wani, W., et al. (2018). Nanocomposite hydrogels: advances in nanofillers used for nanomedicine. Gels 4:E75. doi: 10.3390/gels4030075
Wang, S., Zhang, Z., Chen, B., Shao, J., and Guo, Z. (2018). Self-healing hydrogel of poly(vinyl alcohol)/graphite oxide with pH-sensitive and enhanced thermal properties. J. Appl. Polym. Sci. 135:46143. doi: 10.1002/app.46143
Wang, X., Gan, H., Zhang, M., and Sun, T. (2012). Modulating cell behaviors on chiral polymer brush films with different hydrophobic side groups. Langmuir 28, 2791–2798. doi: 10.1021/la204143g
Wu, Y., Lin, Z. Y., Wenger, A. C., Tam, K. C., and Tang, X. (2018). 3D bioprinting of liver-mimetic construct with alginate/cellulose nanocrystal hybrid bioink. Bioprinting 9, 1–6. doi: 10.1016/j.bprint.2017.12.001
Xiao, G., Wang, Y., Zhang, H., Chen, L., and Fu, S. (2019). Facile strategy to construct a self-healing and biocompatible cellulose nanocomposite hydrogel via reversible acylhydrazone. Carbohydr. Polym. 218, 68–77. doi: 10.1016/j.carbpol.2019.04.080
Xu, T., Miszuk, J. M., Zhao, Y., Sun, H., and Fong, H. (2015). Electrospun polycaprolactone 3D nanofibrous scaffold with interconnected and hierarchically structured pores for bone tissue engineering. Adv. Healthc. Mater. 4, 2238–2246. doi: 10.1002/adhm.201500345
Zagris, N. (2001). Extracellular matrix in development of the early embryo. Micron 32, 427–438. doi: 10.1016/S0968-4328(00)00011-1
Zhang, Y., Venugopal, J., Huang, Z.-M., Lim, C., and Ramakrishna, S. (2005). Characterization of the surface biocompatibility of the electrospun PCL-collagen nanofibers using fibroblasts. Biomacromolecules 6, 2583–2589. doi: 10.1021/bm050314k
Zhao, X., Xu, L., Sun, M., Ma, W., Wu, X., Xu, C., et al. (2017). Tuning the interactions between chiral plasmonic films and living cells. Nat. Commun. 8:2007. doi: 10.1038/s41467-017-02268-8
Keywords: scaffold, nanocomposite hydrogels, biodegradable hydrogels, chiral biomolecules, self-healing
Citation: Esmaeely Neisiany R, Enayati MS, Sajkiewicz P, Pahlevanneshan Z and Ramakrishna S (2020) Insight Into the Current Directions in Functionalized Nanocomposite Hydrogels. Front. Mater. 7:25. doi: 10.3389/fmats.2020.00025
Received: 01 August 2019; Accepted: 21 January 2020;
Published: 07 February 2020.
Edited by:
Miroslav Slouf, Institute of Macromolecular Chemistry (ASCR), CzechiaReviewed by:
Dong-Wook Han, Pusan National University, South KoreaCopyright © 2020 Esmaeely Neisiany, Enayati, Sajkiewicz, Pahlevanneshan and Ramakrishna. This is an open-access article distributed under the terms of the Creative Commons Attribution License (CC BY). The use, distribution or reproduction in other forums is permitted, provided the original author(s) and the copyright owner(s) are credited and that the original publication in this journal is cited, in accordance with accepted academic practice. No use, distribution or reproduction is permitted which does not comply with these terms.
*Correspondence: Rasoul Esmaeely Neisiany, ci5lc21hZWVseUBoc3UuYWMuaXI=; Mohammad Saeid Enayati, bS5zLmVuYXlhdGlAZ21haWwuY29t
Disclaimer: All claims expressed in this article are solely those of the authors and do not necessarily represent those of their affiliated organizations, or those of the publisher, the editors and the reviewers. Any product that may be evaluated in this article or claim that may be made by its manufacturer is not guaranteed or endorsed by the publisher.
Research integrity at Frontiers
Learn more about the work of our research integrity team to safeguard the quality of each article we publish.