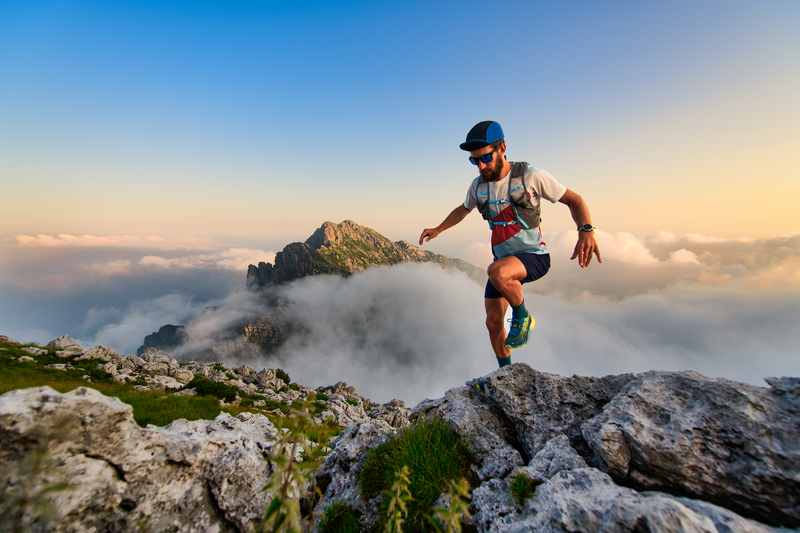
95% of researchers rate our articles as excellent or good
Learn more about the work of our research integrity team to safeguard the quality of each article we publish.
Find out more
ORIGINAL RESEARCH article
Front. Mater. , 18 December 2019
Sec. Biomaterials
Volume 6 - 2019 | https://doi.org/10.3389/fmats.2019.00331
This article is part of the Research Topic Frontiers in Silk Science and Technology View all 18 articles
The development of sustainable and degradable biosensors and bioelectronics has implications for implantable systems, as well in addressing issues of electronic waste. Mechanically flexible and bioresorbable sensors can find applications at soft biological interfaces. While devices typically use metallic and synthetic components and interconnects that are non-degradable or have the potential to cause adverse tissue reactions, the use of nature-derived materials and conducting polymers can provide distinct advantages. In particular, silk fibroin and sericin can provide a unique palette of properties, providing both structural and functional elements. Here, a fully organic, mechanically flexible biosensor in an integrated 3-electrode configuration is demonstrated. Silk sericin conducting ink is micropatterned on a silk fibroin substrate using a facile photolithographic process. Next, using a conducting polymer wire sheathed in silk fibroin, organic interconnects are used to form the electrical connections. This fully organic electrochemical system has competitive performance metrics for sensing in comparison to conventional systems, as verified by detection of a model analyte—ascorbic acid. The stability of the silk biosensor through biodegradation was observed, showing that the sensors can function for several days prior to failure. Such protein-based systems can provide a useful tool for biomonitoring of analytes in the body or environment for controlled periods of time, followed by complete degradation, as transient systems for various applications.
Devices with properties of biocompatibility, flexibility, and degradability can function as on-body (e.g., wearables) or in vivo (e.g., implantables) monitoring systems for human health, soft robotics, or human-machine interfaces (Wang et al., 2017a,b, 2018; Feiner and Dvir, 2018; Xu et al., 2018). The use of mechanically compliant substrates allows function in contact with delicate biological interfaces and physical deformation. Components that can break down functionally and/or physically at the end of their operational period with programmable lifetime and functionality, can potentially resolve risks associated with chronic implants, or the need for additional extractive surgery (Tan et al., 2016; Lei et al., 2017). Concurrently, there is an increasing desire for “green electronics” with sustainable fabrication and lifecycle, with the objective of reducing e-waste (Irimia-Vladu, 2014). Qualities of sustainability, miniaturization, flexibility, biodegradability, and biocompatibility can therefore provide great utility. This class of devices is being enabled by an evolution in organic electronic materials (Balint et al., 2014; Liao et al., 2015), as well as advanced synthetic or bio-derived/inspired substrates (Zhu et al., 2016; Liu et al., 2017). Natural, intrinsically flexible and mechanically robust biomaterials have been proposed as structural components for a wide variety of devices (Suginta et al., 2013; Zhu et al., 2016; Liu et al., 2017). In particular, silk proteins (fibroin and sericin) provide a unique ensemble of properties that make them ideal for these applications. In comparison to synthetic polymers, they can provide controllable biodegradability, and mild immune and inflammatory responses (Enomoto et al., 2010; Thurber et al., 2015). We posit the ability of silk proteins to provide not just the structural components of biodegradable devices, but also the functional constituents therein (Kim et al., 2010; Hwang et al., 2013).
In this work, we show the use of silk proteins to fabricate electrochemical biosensors as well as their interconnects, demonstrating how fibroin and sericin can provide viable and competitive alternatives. Specifically the goal is to outline the substitution of inorganic/metallic materials at sensor-biological interfaces (Rivnay et al., 2014). In conventional electrochemical systems, a 3-electrode configuration with metal electrodes (e.g., Pt, Ag/AgCl) is used. Conducting or semiconducting fibers/wires are needed to connect various components and external power sources. While metals present high conductivity and low cost, they tend to be oxidized under ambient and especially, physiological conditions, and can possess a mechanical mismatch in terms of weight and modulus at soft tissue interfaces (Geddes and Roeder, 2003). Often, they have low biocompatibility, cytotoxicity, and foreign body reaction (Voskerician et al., 2005; Gong et al., 2016). With a few exceptions, they are not biodegradable (Yun et al., 2009). In some cases, electrodes have to be coated with soft materials to minimize inflammatory responses or blocking due to protein adsorption (Hanssen et al., 2016). However, there have been relatively few studies in the development of fully organic, flexible functional devices, typically owing to lower performance metrics, leading to the need to incorporate metallic elements. Here we show how silk proteins in conjunction with conducting polymers can be used for the fabrication of functional fully organic, flexible, degradable biosensors.
In this system, conductive electrodes are micropatterned on a silk fibroin support substrate to form biosensing elements. The electrodes are formed using an ink comprising the conducting polymer poly (3,4-ethylenedioxythiophene):poly(styrene sulfonate) (PEDOT:PSS) dispersed within a silk sericin protein matrix. We utilize photocrosslinkable variants of both silk fibroin and sericin, that permits the use of a facile photolithographic technique to fabricate a flexible device (Kurland et al., 2013, 2014). Using these biomaterials, our group has earlier shown the fabrication of flexible biosensors for electroactive targets (e.g., ascorbic acid, dopamine, glucose), biomacromolecules such as vascular endothelial growth factor (VEGF), and microsupercapacitors for energy storage (Pal et al., 2016b, 2018; Xu and Yadavalli, 2019). Here, for the first time, we show a 3-electrode electrochemical cell using only the silk conductive ink + flexible silk substrate, without metal components. This implies that the working (WE), reference (RE), and counter electrodes (CE) are formed using the organic ink. We further show the use of core-sheath PEDOT:PSS-silk fibroin conductive wires to connect the devices. Conductive polymers constituting “organic wiring,” offer advantages in terms of biocompatibility, low cytotoxicity, and ease of biochemical modification (Liao et al., 2015; Aydemir et al., 2016; Pappa et al., 2018). Polymer sheathing can protect their connections with external power sources or readouts. Protective sheaths around the wires can improve flexibility and operational life by providing shielding from the external environment, as well as protecting the surrounding tissue from damage (Green et al., 2008). In this work, ascorbic acid is used as a model small molecule analyte to demonstrate the function of the sensor. Fully organic silk biosensors with organic wiring can potentially permit real-time, continuous monitoring of health parameters in a minimally- or non-invasive manner, while providing mechanical conformability to the intrinsically curvy body or tissue interface. The system is stable in a liquid environment, but can be proteolytically degraded. These studies lay the groundwork for the use of silk biomaterials toward mechanically flexible, functionally transient devices that can provide highly biocompatible, and degradable systems for healthcare or environmental applications.
All analytical grade reagents were used unless stated otherwise. Silk fibroin was extracted and purified from Bombyx mori silkworms following a well-established protocol (Rockwood et al., 2011). Silk sericin was used as received from Wako Chemicals (Richmond, VA). PEDOT:PSS (dry re-dispersible pellets) was purchased from Sigma-Aldrich (St. Louis, MO) and made into 1% solution in DI-water. The PEDOT:PSS solution was homogenized by ultrasonication (20 kHz, Cole-Parmer, Vernon Hills, IL) for 30 min, and filtered through a 0.45 μm-pore-size filter. A conductive ink composite consisting of PEDOT:PSS suspension in photosericin was stored at room temperature for later use. For the chemical modification of silk proteins, 2-isocyanatoethyl methacrylate (IEM, 98% with ≤0.1% BHT as inhibitor) and anhydrous lithium chloride (LiCl) (Sigma-Aldrich) were used. Anhydrous dimethyl sulfoxide (DMSO) and formic acid (FA) were from Thermo Fisher Scientific (Waltham, MA). L-ascorbic acid powder was purchased from Duda Energy LLC (Decatur, AL).
Photoreactive fibroin and sericin were synthesized according previously reported protocols (Kurland et al., 2013; Pal et al., 2016a). Briefly, the silk protein (fibroin or sericin) was dissolved in 1 M LiCl in DMSO to form 1% (w/v) solution. The proteins were conjugated with photoreactive methacrylate moieties by reaction with IEM at 60°C for 5 h with continuous N2 purge. The modified proteins were precipitated in excess cold ethanol overnight. The precipitate was washed with cold ethanol/acetone (1:1) solution and centrifuged (4,000 rpm, 20 min, 4°C), and this process was repeated 3 times. Finally, the product was lyophilized to obtain photocrosslinkable fibroin and sericin powder (referred to herewith as photofibroin and photosericin).
PEDOT:PSS pellets were dispersed in water followed by ultrasonication to form a homogeneous stock solution of 10% (w/w). Fiber spinning was carried out using a wet-spinning method (Zhang et al., 2019). PEDOT:PSS solution was extruded via a syringe pump (Harvard Apparatus PHD 2000) into a 98% H2SO4 coagulation bath. Extrusion was performed from the bottom of the bath. Optimizing for the processing conditions, the fiber diameter (strength), and conductivity, fibers with 10 wt.% PEDOT:PSS were used, which were extruded using a blunt 27G needle at 0.25 μl/min. This composition resulted in highly stable fibers ~50 μm diameter that can be easily handled, with a conductivity of ~140 S/cm. The spun fibers were directly washed using an ethanol/water mixture (3:1 v/v) to remove any residual H2SO4. PEDOT:PSS fibers were collected on a reel then dried at room temperature.
Silk-sheathed PEDOT:PSS wires were formed by coating the conductive fibers in photofibroin via photocrosslinking at an ambient environment. Formic acid was used as the solvent with 13.2% (w/v) of photofibroin and 4.4% of Irgacure 2959 photoinitiator (BASF, Germany). The photoreactive, optically transparent coating solution was applied on a glass microchannel support to form a bottom layer. PEDOT:PSS fibers were then straightened and anchored on the bottom. A top layer was then formed by drop-casting. Following evaporation of the excess solvent, the sheathed fiber was crosslinked using UV light for 5 s (OmniCure S1000 UV Spot Curing lamp (Lumen Dynamics, Ontario, Canada) at 365 nm (2 mW cm−2). Free-standing, sheathed, conducting wires were obtained by separating from the glass support using water immersion.
Flexible silk fibroin substrates and conductive microelectrodes were formed using contact photolithography with designed features (Figure 1) (Xu et al., 2019). The substrate was formed from a solution composed of 7.5% (w/v) of photofibroin and 1.5% of Irgacure 2959 photoinitiator in formic acid (FA). The solution was drop-cast on a clean glass substrate. To avoid adhesion between the photoresist and the photomask, the excess solvent was allowed to completely evaporate in a fume hood. The cast fibroin thin films were polymerized by UV exposure using an OmniCure S1000 UV Spot Curing lamp (Lumen Dynamics, Ontario, Canada). The entire film was exposed at 365 nm UV (2 mW cm−2) for 2 s to form the flexible substrates. The conductive ink is a water-based biocomposite with a formulation of 2.5% (w/v) of photosericin, 1% (w/v) PEDOT:PSS, and 0.5% (v/v) of Darocur 1173 photoinitiator. The ink was drop-cast on the fibroin substrates. After the conductive ink was completely dried under dark in the fume hood, microelectrodes were patterned under UV light for 1.5 s through a chrome photomask. The microelectrodes were developed in DI-water under sonication, followed by rinsing with excess water to remove any residual ink.
Figure 1. (A) Fabrication of the fully-organic, flexible 3-electrode silk biosensor using photolithography. (B) Fabrication of the fibroin sheathed conducting wires. The images in the center show the biosensor and the connected device.
Silk fibroin sheathed PEDOT: PSS wires were then connected to the electrodes using a conductive ink composite of 6.25% (w/w) PEDOT:PSS in photosericin. The conductive ink was cast at the junction between the wires and the electrodes and dried slowly under ambient conditions. This was followed by photocrosslinking of the junction using UV. A 7.25% (w/v) solution of photofibroin in HFIP was used to further seal the junction. The solution was cast on the junction, dried and crosslinked under UV light. The presence of residual acrylate groups on the surface of the electrodes in the conductive ink and in the photofibroin solution allows the covalent attachment of the entire system on the substrate. Therefore, the connection of the wire with the electrode do not easily get damaged under mechanical deformation. Finally, the system was connected to a potentiostat for electrochemical measurements.
Electrochemical measurements were conducted using a Gamry Interface 1010E Potentiostat (Gamry Instruments, Warminster, PA). The electrical conductivity of uncoated PEDOT:PSS fibers and sheathed fibers were characterized by cyclic voltammetry (CV) using a two-electrode configuration. In order to test if the coating was successful, Cu tape was attached to the exposed portion of the fiber and different positions on the sheath. The ability of the silk fibroin to form an electrically insulating sheath on the conductive fibers was also tested. The experimental set up and the conductivity of the bare and sheathed wires are shown in Figure S1. In order to compare the performance of the designed all-organic electrodes, a standard three-electrode cell configuration was used (organic electrode as the working electrode, Ag/AgCl as the standard reference electrode and Pt as the counter electrode). Cyclic voltammetry (CV) and the chronoamperometry were carried out to characterize the biosensor and to conduct the electrochemical detection. The scanning range was −1 to 1 V at the rate of 50 mV s−1. In amperometric measurements, the potential was set to 0.5 V.
To determine the response of the biosensor as they degrade, the organic microelectrodes were immersed in 3 ml of PBS solution containing 1 mg ml−1 protease (Protease XIV from Streptomyces griseus, ≥3.5 U mg−1, Sigma Aldrich) and stored at 37°C. As a control, PBS buffer without protease was used. Samples were extracted at regular intervals and investigated under an optical microscope for degradation. In addition, the function of the biosensors was studied over this period. The sensors were taken out at Day 1, 4, and 7, cleaned with DI-water, and dried using N2. The sensors were then used for sensing of ascorbic acid. The degradation evaluation was tested by cyclic voltammetry (CV) using a standard three-electrode cell configuration as noted above.
In comparison to their rigid counterparts, mechanically flexible sensors are designed to function at non-planar, soft, and even non-stationary bio-interfaces. They can be useful for the accurate detection and quantification of specific biomarkers in clinical diagnosis toward evaluating biological and pathological activities, or response to therapeutic interventions (Xu et al., 2018). Biosensors and bioelectronics characterized by the ability to fully or partially dissolve, disintegrate, or physically or chemically decompose in a controlled fashion in a working environment, can further enhance the applications of flexible devices. These biodegradable or bioresorbable systems would provide a “transient” function useful in a number of spheres of operation e.g., implantable devices, devices that minimize waste, and platforms for data security (Kang et al., 2018; Li et al., 2018; Chatterjee et al., 2019). Our objective in this work is to show that silk proteins can enable such flexible (bio)sensors without the need for either metallic electrodes or interconnects. We discuss the fabrication and characterization of a fully-organic biosensor that is also mechanically flexible and compliant, and can be completely degraded under the action of proteolytic enzymes. Further we show that connections to external devices can be facilitated by long, highly conductive PEDOT:PSS fibers. The fibers are coated with optically transparent silk fibroin to form sheathed, insulated, fully organic conducting wires. Dopamine, a neurotransmitter, ascorbic acid (vitamin C), and glucose are three model analytes monitored using the developed organic bioelectrodes to demonstrate direct electrochemical biosensing. While the results for ascorbate is shown in the manuscript, the data for dopamine and glucose are shown in the Supplementary Information in the interest of brevity.
The proposed system is based on photocrosslinkable silk proteins which are compatible with, and can be functionalized by the commercially available conducting polymer PEDOT:PSS to form an integrated functional and structural device. The underlying substrate is formed from silk fibroin that can provide strong support to the functional sensing patterns as well as be mechanically compliant to a curvy biological surface. The electrochemical cell is fabricated using a sericin/PEDOT:PSS biocomposite. As a photocrosslinkable conducting ink, this can be micropatterned to form a 3-electrode design on the flexible support (Figure 1A). The sensor design is shown in detail in Figure 2a. The sensors are around 1 cm2 in area with electrode patterns 1,000 μm wide. In contrast to earlier reported silk-based biosensors in which only the working electrode was fabricated from the organic ink (necessitating Pt, Ag/AgCl counter and reference electrodes), here the working, reference, and counter electrodes are all fabricated from the same material (silk biocomposite). Photolithography remains a desirable technique to fabricate structures of high complexity over large areas in a fast and reproducible fashion. As a high-resolution technique, it is possible to fabricate multiplexed microsensors with patterns on the order of 10 s of micrometers.
Figure 2. (a) Sensor design showing the position of the working, reference, and counter electrodes. The biosensors with the core-sheath PEDOT:PSS wires/fibroin sheaths are conformable, flexible and can be subjected to mechanical deformation. (b) SEM images of the cross section of the organic electrodes, showing the underlying fibroin substrate (1) and sericin-PEDOT:PSS conductive microelectrodes (2). (c) SEM images of the sheathed wire showing the cross-section and the PEDOT:PSS wire (red circle), a closeup of which can be seen in the bottom right panel.
In order to connect the devices, core-sheath PEDOT:PSS-silk fibroin conductive wires are used to form a fully organic design (Figure 1B). Conductive polymers represent “organic wiring” with biocompatibility and low cytotoxicity (Liao et al., 2015; Aydemir et al., 2016; Pappa et al., 2018). Sheathed multifunctional fibers have been previously reported in tissue engineering (e.g., PCL/PPy and PLA/PPy core-sheath nanofibers) to improve flexibility and operational life (Wei et al., 2005; Xie et al., 2009). Silk-PEDOT-PSS composites have also been previously reported (Müller et al., 2011; Tsukada et al., 2012). However, PEDOT:PSS/fibroin core-sheath configurations and/or connection to a biosensor have not been shown. In this work, fibers optimized for diameter and conductivity were used. The use of silk fibroin to form a protective sheath around the fiber not only improves the operation of the wires, but also protect the connections from the external environment, while minimizing any auxiliary damage to surrounding tissue (Green et al., 2008). These integrated organic sensors are mechanically flexible and can be wrapped, twisted, or otherwise conform to soft tissue interfaces (Figure 2a). As a thin sheet (~20–40 μm thick), they can easily conform to an underlying curvy and soft surface (including soft tissue—Figure S2). The protein micro-matrix can entrap the conductive polymers and prevent them leaching out, while allowing small metabolites such as glucose or ascorbic acid to diffuse into the film and trigger the electrochemical reaction. SEM imaging of the electrode surface on the flexible substrate shows the porous electrode surface (Figure 2b, Figure S3).
Electrochemical characterization was conducted using cyclic voltammetry to clarify the difference in performance between the developed fully-organic biosensor and a conventional organic electrochemical system. Conventional electrochemical biosensors for the monitoring of metabolites usually utilize Ag/AgCl as the standard reference electrode (RE) and Pt as the counter electrode (CE). These are well-investigated systems known to provide steady current flow and stable signal output. In earlier reports, we demonstrated the use of this organic electrode for the detection of various analytes including glucose, ascorbic acid, and vascular endothelial growth factor (Pal et al., 2016b; Xu and Yadavalli, 2019). Ag/AgCl and Pt were employed as independent reference and counter electrodes, respectively. In considering bioresorbable biosensors or electronics, the goal is to provide diagnostic or monitoring function while avoiding extended device load on the body, or requiring secondary surgical procedures for removal. Therefore, using separate standard and counter electrodes is not optimal. However, in comparison to their metallic counterparts, organic electrodes face problems of current stability due to the fact that the continuous electrochemical reaction of PEDOT:PSS affects performance [i.e., oxidized state (conductive) vs. neutral state (non-conductive)].
The electrochemical behavior of organic electrodes used as RE and CE (free of metallic components) was studied to clarify feasibility for sensing. We will refer to the system with all organic electrodes (WE, CE, and RE) as O3E. In comparison, the system with only one organic electrode but with any conventional RE and CE will be referred as OE. For this work, the same material (sericin/PEDOT:PSS conducting ink) was used to fabricate the working electrodes (WE). For the OE, a standard Ag/AgCl reference electrode (RE), and the Pt counter electrode (CE) was used. Cyclic voltammetry (CV) was conducted on both systems. Over a scan range of −1 to 1 V, the O3E system yielded −83.8 and 122 μA at −1 and 1 V, respectively, whereas the OE system exhibited −22.8 and 83.2 μA at the same potentials. CVs are shown in the Figure S4. Interestingly, the O3E sensor had a higher current response than the OE sensor, presumably due to the electrochemical redox reaction occurring in the O3E that can be summarized as the oxidation/reduction of PEDOT:PSS at WE, RE, and CE (Marzocchi et al., 2015).
where C+ and e− represent cations and electrons, respectively. On the other hand, the electrochemical redox reaction in the OE is the Equation (1) at the WE, the reaction:
Occurs at the reference electrode (RE). When the PEDOT:PSS is polarized anodically/cathodically from the neutral state by the applied potential, it is more conductive at both the WE and RE. Therefore, with all three electrodes formed using the PEDOT:PSS ink (O3E), the developed biosensor would be more sensitive to the changes of electrochemical potential than the OE system that only uses PEDOT:PSS as WE. In order to present a miniaturized biosensor, we used the design shown in Figure 2 which allows connections of all electrodes to an external potentiostat.
Following electrochemical analysis, the O3E system was tested as a biosensor. The electroactive species ascorbic acid (AA) was used as a model metabolite in order to demonstrate electrochemical performance and compare to OE configurations. AA, widely known as vitamin C, plays an important role in the formation of collagen in the development of bones, muscles and blood vessels (Rawat et al., 2015). The performance metrics of the O3E are competitive as seen in Figure 3. In all figures, the sensor response is presented as a normalized current (normalized to the baseline signal in pure PBS –Inor = IAA/IPBS × 100%). This allows comparison across different sensors (at least three sensors were tested in each case). The inset shows a rapid response time of the sensor on the order of a few seconds. It can be seen that a higher sensitivity to the addition of AA was observed with a correspondingly higher slope in the calibration curve in comparison to the OE configuration. This is because the redox reactions of AA occur not only at the WE, but also at the RE and CE, implying that the O3E biosensor displays an amplified current response toward the addition of the analyte. The detection limits (LODs) of the developed O3E biosensor and the OE are 49.2 and 50.2 μM, respectively. The close LODs for the two configurations are expected given the identical compositions of the working electrodes for both systems. The OE value is consistent with our earlier report (Pal et al., 2016b). While these values are higher than previously reported LODs for ascorbate biosensors which have been reported to be ~5–10 μM (Pakapongpan et al., 2012; Csiffáry et al., 2016), the ability to be within the same order of magnitude despite the lack of metals is very promising for use as organic, degradable, and flexible biosensors.
Figure 3. The detection of ascorbic acid using different electrode combinations. The blue represents the electrochemical biosensor using sericin/PEDOT:PSS organic electrodes as Working Electrode (WE), Reference Electrode (RE), and Counter Electrode (CE) (O3E). The gray represents the electrochemical cell setup using sericin/PEDOT:PSS organic electrode as WE, Ag/AgCl electrode as RE, Pt as CE (OE). The inset figure is the calibration curve (normalized current vs. concentration of ascorbic acid) for the detection of ascorbic acid using the two electrical setups. The control sample was PBS (Inor = 1). The addition of AA occurred at 100 s intervals. In all experiments, at least three different sensors were studied.
Despite the higher current response, the OE configuration (organic electrodes as WE, the standard Ag/AgCl reference electrode and the Pt counter electrode) yields a higher sensor signal. Therefore, the conductivity of the O3E configuration is not as good as metallic electrodes or even OE. This is likely because the direct electron transfer from the analyte through the PEDOT:PSS is much slower and limited in comparison to Ag or Pt electrodes. This further indicates that these kinds of fully organic (O3E) biosensors would have a narrower detection range in comparison. Since the microfabrication process is conducted at room temperature using only water as the solvent, it is possible to immobilize enzymes and antibodies in the conducting ink, thereby forming biosensors for a wide variety of target metabolites. Detection of glucose (via the enzyme glucose oxidase) and the neurotransmitter, dopamine was demonstrated (Figures S6, S7). In both cases, detection of these targets was achievable over physiologically relevant concentrations, showing the versatility of this approach. The overall area of the electrodes also plays a role in determining the dynamic range with smaller surface areas having similar sensitivities and limits of detection but lower range. Thus, the design of the sensors would have to be optimized depending on the application.
The primary advantage of using a fully-organic biosensor without metallic components is the potential to be controllably degradable (e.g., in vivo or in nature). In vitro, this process may be replicated by the use of proteolytic enzymes. Silk-based materials have demonstrated their utility in this regard and have been extensively studied for their controllable biodegradation (Lu et al., 2011), an important property in their applications in tissue engineering. While resorbable and degradable sensors have been proposed, their performance over time in their working environment has not been fully characterized. Here, the organic devices were examined for sensing of AA under proteolytic biodegradation.
The O3E biosensors were immersed in a solution containing 1 unit ml−1 protease at 37°C over a 1 week period to provide an in vitro simulation of an in vivo environment. The electrochemical sensing for performance is shown in Figure 4. The sensors maintain a linear response Inor to ascorbic acid during the entire process (1 week). Over a period of 24 h, there is no change in the sensor function. Interestingly, from Day 1 to Day 4, the slopes of the calibration curves increased slightly, indicating the sensitivity of the developed biosensor to the addition of AA was improved. While this appears counterintuitive, this is likely due to the biodegradation of the silk matrix causing an exposure of the entrapped PEDOT:PSS, which in turn improves the direct electron transfer from the electrolyte to the conductive polymers. From Day 4 to Day 7, the slope of the calibration curve remains the same. Following around 10 days, the sensor connections degrade, making it impossible to get an accurate reading. Therefore, we can specify a working life-time for the devices as studied in this work to be ~1 week. Given that the linear response of the sensors is unaffected by the degradation, it is therefore possible to envision a simple mathematical relationship to provide the accurate concentrations on a given day from the calibration curves shown.
Figure 4. The electrochemical performance of the O3E flexible biosensor when stored in 1 unit ml−1 of protease solution at 37°C. The sensors were monitored over a period of 1 week. The control sample was PBS (Inor = 1). It may be noted that after 10 days, the sensors begin to lose mechanical integrity at the macroscale and are not easily connected for reliable measurements. The relatively high error bars can be explained by sensor-to-sensor variability obtained during averaging of the signals from three separate sensors.
At the macroscale, the biosensors lose structural integrity over ~1 month (Images showing the electrode surface degradation are shown in Figure S5). It may be noted that the PEDOT:PSS itself is not biodegradable. However, due to the degradation of the matrix, causes the entire sensor to break down. This was earlier shown by our group (Pal et al., 2016a,b). In these experiments, the sensors tested are formed on fibroin films ~20 μm thick with the electrodes themselves being ~20 μm thick. By carefully controlling the material thickness and crosslinking, it is possible to form devices that can function for longer periods of time (e.g., months), which provides interesting possibilities. However, as designed, these sensors can be used for “transient” devices to monitor wound healing for instance, wherein a short timeframe of operation is required.
One of the possible applications of using such flexible, fully organic biosensors is in vivo monitoring of metabolites. However, sensor fouling continues to be one of the major challenges facing biosensors. Non-specific absorption of proteins or cells results in sensor occlusion and therefore a loss in performance (Wang et al., 2015). It has been suggested that a layer of membrane from naturally derived materials (e.g., silk fibroin, cellulose, chitosan) could help reduce biofouling and prolong the lifetime of implanted biosensors (Wisniewski and Reichert, 2000). We observed that interestingly, the developed silk biosensor possesses a natural resistance to biofouling owing to its protein-based structural composition. Given the reference range for albumin concentrations in serum is ~35–50 mg/ml, the O3E biosensor was testing by immersing in a 0.1 M PBS buffer containing 40 mg/ml BSA. The control group was immersed in 0.1 M PBS buffer. Both groups were examined for their performance in detecting ascorbic acid following 2 days of immersion (Figure 5). The linear response of the biosensors is maintained in both PBS even in the presence of the strong challenge of BSA blocking. An O3E biosensor was also tested against glucose in similar conditions with PBS control and 40 mg/ml BSA. A slight reduction in sensitivity was observed, but the sensor again maintained a linear response (Figure S7). It is therefore hypothesized that the BSA can absorb to the electrode surface, but is isolated by the silk protein matrix from insulating the conductive materials (PEDOT:PSS) in the matrix and therefore does not affect the diffusion to the electrodes. However, it could potentially affect the encapsulated enzymes, thereby reducing performance. It may be noted that typically much lower concentrations of BSA are studied (2–5 mg/ml) for such sensors, which reflects that these sensors display good promise for the next series of experiments (i.e., translation to animal models and longer-term investigations). Even though the tested solution containing BSA is a simplified system and does not fully represent a complex biofluid system, the performance of the developed organic flexible biosensor was able to exhibit good stability against protein absorption. Future studies are focused on studying the function of biosensors in a working environment of such complex fluids that can present biodegradation and biofouling challenges along with the presence of other chemical interferents.
Figure 5. The O3E biosensor was evaluated in the presence of a blocking agent (BSA). The biosensors were immersed in 40 mg/ml BSA solution (in 0.1 M PBS, pH 7.4) (blue line), or PBS buffer (as control) (gray line) over 2 days. A linear response is maintained over 2 days, with very small change in comparison to the starting biosensors (Day 0–orange).
In summary, here we demonstrate the feasibility of using silk proteins to construct a fully-organic, flexible biosensor for the monitoring of metabolites. The entire fabrication process is based on contact photolithography which is simple, rapid, and can be performed under ambient conditions. Using a combined 3-electrode configuration, the working, reference, and counter electrodes are fabricated from an organic conducting ink. The developed biosensors display excellent performance in terms of sensitivity and detection range for the sensing of analytes. Thus, these biosensors can be considered for in vivo implantation whereby they can function in challenging biological environments. Most interestingly, these biosensors display a transient function whereby they can function for a period of 1 week after which they begin to degrade. They display a stable linear performance even during proteolytic biodegradation. The target repertoire of the biosensors can be expanded by adding different enzymes in the basic formulation of sericin/PEDOT:PSS conductive ink. This concept of fully-organic devices can therefore provide a pathway for the development of next-generation, ease-to-use, and degradable flexible biosensors for health monitoring.
The datasets generated for this study are available on request to the corresponding author.
MX, YJ, and VY conceptualized and designed the study. MX and SP performed the integrated device fabrication and biosensing. YJ performed the experiments of PEDOT:PSS fiber sheathing. All authors contributed to data analysis and writing the manuscript.
This research was partly funded by the National Science Foundation (CBET-1704435), the State Scholarship Fund (No. 201808505099), and National Science Foundation of China (No. 31402144).
The authors declare that the research was conducted in the absence of any commercial or financial relationships that could be construed as a potential conflict of interest.
The Supplementary Material for this article can be found online at: https://www.frontiersin.org/articles/10.3389/fmats.2019.00331/full#supplementary-material
Aydemir, N., Malmstrom, J., and Travas-Sejdic, J. (2016). Conducting polymer based electrochemical biosensors. Phys. Chem. Chem. Phys. 18, 8264–8277. doi: 10.1039/C5CP06830D
Balint, R., Cassidy, N. J., and Cartmell, S. H. (2014). Conductive polymers: towards a smart biomaterial for tissue engineering. Acta Biomater. 10, 2341–2353. doi: 10.1016/j.actbio.2014.02.015
Chatterjee, S., Saxena, M., Padmanabhan, D., Jayachandra, M., and Pandya, H. J. (2019). Futuristic medical implants using bioresorbable materials and devices. Biosens. Bioelectron. 142:111489. doi: 10.1016/j.bios.2019.111489
Csiffáry, G., Futo, P., Adányi, N., and Kiss, A. (2016). Ascorbate oxidase-based amperometric biosensor for L-ascorbic acid determination in beverages. Food Technol. Biotechnol. 54, 31–35. doi: 10.17113/ftb.54.01.16.4135
Enomoto, S., Sumi, M., Kajimoto, K., Nakazawa, Y., Takahashi, R., Takabayashi, C., et al. (2010). Long-term patency of small-diameter vascular graft made from fibroin, a silk-based biodegradable material. J. Vasc. Surg. 51, 155–164. doi: 10.1016/j.jvs.2009.09.005
Feiner, R., and Dvir, T. (2018). Tissue-electronics interfaces: from implantable devices to engineered tissues. Nat. Rev. Mater. 3:17076. doi: 10.1038/natrevmats.2017.76
Geddes, L., and Roeder, R. (2003). Criteria for the selection of materials for implanted electrodes. Ann. Biomed. Eng. 31, 879–890. doi: 10.1114/1.1581292
Gong, C.-S. A., Syu, W.-J., Lei, K. F., and Hwang, Y.- S. (2016). Development of a flexible non-metal electrode for cell stimulation and recording. Sensors 16:1613. doi: 10.3390/s16101613
Green, R. A., Lovell, N. H., Wallace, G. G., and Poole-Warren, L. A. (2008). Conducting polymers for neural interfaces: challenges in developing an effective long-term implant. Biomaterials 29, 3393–3399. doi: 10.1016/j.biomaterials.2008.04.047
Hanssen, B. L., Siraj, S., and Wong, D. K. (2016). Recent strategies to minimise fouling in electrochemical detection systems. Rev. Anal. Chem. 35, 1–28. doi: 10.1515/revac-2015-0008
Hwang, S. W., Kim, D. H., Tao, H., Kim, T. I., Kim, S., Yu, K. J., et al. (2013). Materials and fabrication processes for transient and bioresorbable high-performance electronics. Adv. Funct. Mater. 23, 4087–4093. doi: 10.1002/adfm.201300127
Irimia-Vladu, M. (2014). “Green” electronics: biodegradable and biocompatible materials and devices for sustainable future. Chem. Soc. Rev. 43, 588–610. doi: 10.1039/C3CS60235D
Kang, S.-K., Koo, J., Lee, Y. K., and Rogers, J. A. (2018). Advanced materials and devices for bioresorbable electronics. Acc. Chem Res. 51, 988–998. doi: 10.1021/acs.accounts.7b00548
Kim, D. H., Viventi, J., Amsden, J. J., Xiao, J. L., Vigeland, L., Kim, Y. S., et al. (2010). Dissolvable films of silk fibroin for ultrathin conformal bio-integrated electronics. Nat. Mater. 9, 511–517. doi: 10.1038/nmat2745
Kurland, N. E., Dey, T., Kundu, S. C., and Yadavalli, V. K. (2013). Precise patterning of silk microstructures using photolithography. Adv. Mater. 25, 6207–6212. doi: 10.1002/adma.201302823
Kurland, N. E., Dey, T., Wang, C., Kundu, S. C., and Yadavalli, V. K. (2014). Silk protein lithography as a route to fabricate sericin microarchitectures. Adv. Mater. 26, 4431–4437. doi: 10.1002/adma.201400777
Lei, T., Guan, M., Liu, J., Lin, H. C., Pfattner, R., Shaw, L., et al. (2017). Biocompatible and totally disintegrable semiconducting polymer for ultrathin and ultralightweight transient electronics. Proc. Natl. Acad. Sci. U.S.A. 114, 5107–5112. doi: 10.1073/pnas.1701478114
Li, R. F., Wang, L., Kong, D. Y., and Yin, L. (2018). Recent progress on biodegradable materials and transient electronics. Bioact. Mater. 3, 322–333. doi: 10.1016/j.bioactmat.2017.12.001
Liao, C., Zhang, M., Yao, M. Y., Hua, T., Li, L., and Yan, F. (2015). Flexible organic electronics in biology: materials and devices. Adv. Mater. 27, 7493–7527. doi: 10.1002/adma.201402625
Liu, Y., He, K., Chen, G., Leow, W. R., and Chen, X. (2017). Nature-inspired structural materials for flexible electronic devices. Chem. Rev. 117, 12893–12941. doi: 10.1021/acs.chemrev.7b00291
Lu, Q., Zhang, B., Li, M., Zuo, B., Kaplan, D. L., Huang, Y., et al. (2011). Degradation mechanism and control of silk fibroin. Biomacromolecules 12, 1080–1086. doi: 10.1021/bm101422j
Marzocchi, M., Gualandi, I., Calienni, M., Zironi, I., Scavetta, E., Castellani, G., et al. (2015). Physical and electrochemical properties of PEDOT:PSS as a tool for controlling cell growth. ACS Appl. Mater. Interfaces 7, 17993–18003. doi: 10.1021/acsami.5b04768
Müller, C., Hamedi, M., Karlsson, R., Jansson, R., Marcilla, R., Hedhammar, M., et al. (2011). Woven electrochemical transistors on silk fibers. Adv. Mater. 23, 898–901. doi: 10.1002/adma.201003601
Pakapongpan, S., Mensing, J. P., Lomas, T., and Tuantranont, A. (2012). “Electrochemical sensor for ascorbic acid based on graphene/CuPc/PANI nanocomposites,” in 2012 IEEE International Conference on Electron Devices and Solid State Circuit (EDSSC) (Bangkok: IEEE).
Pal, R. K., Farghaly, A. A., Collinson, M. M., Kundu, S. C., and Yadavalli, V. K. (2016a). Photolithographic micropatterning of conducting polymers on flexible silk matrices. Adv. Mater. 28, 1406–1412. doi: 10.1002/adma.201504736
Pal, R. K., Farghaly, A. A., Wang, C., Collinson, M. M., Kundu, S. C., and Yadavalli, V. K. (2016b). Conducting polymer-silk biocomposites for flexible and biodegradable electrochemical sensors. Biosens. Bioelectron. 81, 294–302. doi: 10.1016/j.bios.2016.03.010
Pal, R. K., Kundu, S. C., and Yadavalli, V. K. (2018). Fabrication of flexible, fully organic, degradable energy storage devices using silk proteins. Acs Appl. Mater. Interfaces 10, 9620–9628. doi: 10.1021/acsami.7b19309
Pappa, A.-M., Parlak, O., Scheiblin, G., Mailley, P., Salleo, A., and Owens, R. M. (2018). Organic electronics for point-of-care metabolite monitoring. Trends Biotechnol. 36, 45–59. doi: 10.1016/j.tibtech.2017.10.022
Rawat, K., Sharma, A., Solanki, P. R., and Bohidar, H. B. (2015). Potential of gelatin-zinc oxide nanocomposite as ascorbic acid sensor. Electroanalysis. 27, 2251–2491. doi: 10.1002/elan.201500090
Rivnay, J., Owens, R. M., and Malliaras, G. G. (2014). The rise of organic bioelectronics. Chem. Mater. 26, 679–685. doi: 10.1021/cm4022003
Rockwood, D. N., Preda, R. C., Yücel, T., Wang, X., Lovett, M. L., and Kaplan, D. L. (2011). Materials fabrication from Bombyx mori silk fibroin. Nat. Protoc. 6, 1612–1631. doi: 10.1038/nprot.2011.379
Suginta, W., Khunkaewla, P., and Schulte, A. (2013). Electrochemical biosensor applications of polysaccharides chitin and chitosan. Chem. Rev. 113, 5458–5479. doi: 10.1021/cr300325r
Tan, M. J., Owh, C., Chee, P. L., Kyaw, A. K., Kai, D., and Jun Loh, X. (2016). Biodegradable electronics: cornerstone for sustainable electronics and transient applications. J. Mater. Chem. C 4, 5531–5558. doi: 10.1039/C6TC00678G
Thurber, A. E., Omenetto, F. G., and Kaplan, D. L. (2015). In vivo bioresponses to silk proteins. Biomaterials 71, 145–157. doi: 10.1016/j.biomaterials.2015.08.039
Tsukada, S., Nakashima, H., and Torimitsu, K. (2012). Conductive polymer combined silk fiber bundle for bioelectrical signal recording. PLoS ONE 7:e33689. doi: 10.1371/journal.pone.0033689
Voskerician, G., Chung-Chiun, L., and Anderson, J. M. (2005). Electrochemical characterization and in vivo biocompatibility of a thick-film printed sensor for continuous in vivo monitoring. IEEE Sens. J. 5, 1147–1158. doi: 10.1109/JSEN.2005.857877
Wang, C., Wang, C., Huang, Z., and Xu, S. (2018). Materials and structures toward soft electronics. Adv. Mater. 30:1801368. doi: 10.1002/adma.201801368
Wang, L., Chen, D., Jiang, K., and Shen, G. (2017a). New insights and perspectives into biological materials for flexible electronics. Chem. Soc. Rev. 46, 6764–6815. doi: 10.1039/C7CS00278E
Wang, X. W., Liu, Z., and Zhang, T. (2017b). Flexible sensing electronics for wearable/attachable health monitoring. Small 13:1602790. doi: 10.1002/smll.201602790
Wang, Y., Vaddiraju, S., Gu, B., Papadimitrakopoulos, F., and Burgess, D. J. (2015). Foreign body reaction to implantable biosensors: effects of tissue trauma and implant size. J. Diabetes Sci. Technol. 9, 966–977. doi: 10.1177/1932296815601869
Wei, M., Lee, J., Kang, B., and Mead, J. (2005). Preparation of core-sheath nanofibers from conducting polymer blends. Macromol. Rapid Comm. 26, 1127–1132. doi: 10.1002/marc.200500212
Wisniewski, N., and Reichert, M. (2000). Methods for reducing biosensor membrane biofouling. Colloids Surf. B Biointerfaces 18, 197–219. doi: 10.1016/S0927-7765(99)00148-4
Xie, J., MacEwan, M. R., Willerth, S. M., Li, X., Moran, D. W., Sakiyama-Elbert, S. E., et al. (2009). Conductive core–sheath nanofibers and their potential application in neural tissue engineering. Adv. Funct. Mater. 19, 2312–2318. doi: 10.1002/adfm.200801904
Xu, M., Obodo, D., and Yadavalli, V. K. (2018). The design, fabrication, and applications of flexible biosensing devices. Biosens. Bioelectron. 124–125, 96–114. doi: 10.1016/j.bios.2018.10.019
Xu, M., Pradhan, S., Agostinacchio, F., Pal, R. K., Greco, G., Mazzolai, B., et al. (2019). Easy, scalable, robust, micropatterned silk fibroin cell substrates. Adv. Mater. Interfaces 6:801822. doi: 10.1002/admi.201801822
Xu, M., and Yadavalli, V. K. (2019). Flexible biosensors for the impedimetric detection of protein targets using silk-conductive polymer biocomposites. ACS Sensors 4, 1040–1047. doi: 10.1021/acssensors.9b00230
Yun, Y., Dong, Z., Lee, N., Liu, Y., Xue, D., Guo, X., et al. (2009). Revolutionizing biodegradable metals. Mater. Today 12, 22–32. doi: 10.1016/S1369-7021(09)70273-1
Zhang, J., Seyedin, S., Qin, S., Lynch, P. A., Wang, Z., Yang, W., et al. (2019). Fast and scalable wet-spinning of highly conductive PEDOT: PSS fibers enables versatile applications. J. Mater. Chem. A 7, 6401–6410. doi: 10.1039/C9TA00022D
Keywords: organic electronics, flexible biosensor, silk fibroin, silk sericin, conductive polymers, biodegradable, metabolite monitoring
Citation: Xu M, Jiang Y, Pradhan S and Yadavalli VK (2019) Use of Silk Proteins to Form Organic, Flexible, Degradable Biosensors for Metabolite Monitoring. Front. Mater. 6:331. doi: 10.3389/fmats.2019.00331
Received: 15 September 2019; Accepted: 03 December 2019;
Published: 18 December 2019.
Edited by:
Antonella Motta, University of Trento, ItalyReviewed by:
PaYaM ZarrinTaj, Oklahoma State University, United StatesCopyright © 2019 Xu, Jiang, Pradhan and Yadavalli. This is an open-access article distributed under the terms of the Creative Commons Attribution License (CC BY). The use, distribution or reproduction in other forums is permitted, provided the original author(s) and the copyright owner(s) are credited and that the original publication in this journal is cited, in accordance with accepted academic practice. No use, distribution or reproduction is permitted which does not comply with these terms.
*Correspondence: Vamsi K. Yadavalli, dnlhZGF2YWxsaUB2Y3UuZWR1
Disclaimer: All claims expressed in this article are solely those of the authors and do not necessarily represent those of their affiliated organizations, or those of the publisher, the editors and the reviewers. Any product that may be evaluated in this article or claim that may be made by its manufacturer is not guaranteed or endorsed by the publisher.
Research integrity at Frontiers
Learn more about the work of our research integrity team to safeguard the quality of each article we publish.