- 1Institute of Research and Development, Duy Tan University, Da Nang, Vietnam
- 2Laboratory of Applied Physics, Advanced Institute of Materials Science, Ton Duc Thang University, Ho Chi Minh City, Vietnam
- 3Faculty of Applied Sciences, Ton Duc Thang University, Ho Chi Minh City, Vietnam
In this work, the Cd1−xMnxSe alloy was successfully prepared using a successive ionic layer adsorption and reaction method to investigate the layers' effect on the properties of devices while concentration dopant was optimized at 20% (molar concentrations between Mn2+ and Cd2+ ions in the Cd1−xMnxSe material). The layers of the Cd1−xMnxSe alloy play a role in improving the optical, photovoltaic, and electrochemical properties of the solar cells. Hence, the efficiency performance of devices based on the Cd1−xMnxSe alloy reached ~3.8%. Besides, in order to explain this result, the experimental I–V curve was also used to determine the resistances at the interfaces and the resistance diffusion of the devices. This dynamic resistance can be compared with that of electrochemical impedance spectra.
Introduction
Semiconductor quantum dots (QDs) have drawn great attention for application in a number of fields due to the optical properties of these materials (Chen et al., 2016; Liu et al., 2016, 2019a,b; Li et al., 2019). Now, nanoparticles prominently become a dye sensitized for the third-generation solar cells because of low-cost fabrication technology, high photostability, the controlled sizes (Peng and Peng, 2001), higher absorption coefficient (Beard Matthew, 2011), and the multiple exciton generation (Sargent, 2005). However, QDSSCs (quantum dot sensitized solar cells) have reached ~13% performance, which is lower than the theory limits (Jiao et al., 2017). Recently, plenty of QDs [CdS, CdSe, CdTe (Shen et al., 2015), and PbS (Jumabekov et al., 2014)] are widely applied in the QDSSCs because of their unique properties (Duan et al., 2014). It is noticeable that the CdS and CdSe QDs have attracted considerable interest due to their optical property stability (Lin et al., 2014), a higher conduction band than TiO2 (Lee and Lo, 2009), low resistivity (Mendoza-Perez et al., 2009), and wide absorbed spectrum (Liji Sobhana et al., 2011). However, this result was still low compared with that of dye-sensitized solar cells (DSSCs). So, a CdS/CdSe system was widely investigated due to its wide absorption spectrum, the shift of the absorption peak toward in the visible region, and rising of the conduction band (CB) as the combined CdS and CdSe QDs compared with TiO2 CB. However, the performance based on this system achieved 4% efficiency (Lee and Lo, 2009), and its performance was still lower than that of DSSCs due to much trapping and recombination at the TiO2/QDs/electrolyte triple interfaces (Abdellah et al., 2014).
In recent times, metal ions doped into the QDs can be replaced by the single QDs and co-sensitized system to reduce achievable recombination (Hodes et al., 1987; Fang et al., 1997, 2011; Gratzel, 2001, 2003; William Yu et al., 2003; Shen and Lee, 2008; Fan et al., 2009; Gimenez et al., 2009; Zhuge et al., 2009; Gonzalez-Pedro et al., 2010; Schmid, 2014; Tan Phat et al., 2018) because it can improve the charge collection and transfer process. In addition, metal ions are famous for their lowest resistance and large mobility. For example, Tan Phat et al. recorded a performance of 4.22% as Cu2+ ion doped into CdSe QDs because its attractive optical and magnetic properties were more interesting than that of CdSe and PbS QDs (Tan Phat et al., 2018). Their improving properties can be archived by doping metal in QDs like those in Refs. (Hodes et al., 1987; Gratzel, 2001, 2003; Fan et al., 2009; Gimenez et al., 2009; Zhuge et al., 2009; Gonzalez-Pedro et al., 2010) to make contributions to the more absorption photons of photoelectrodes.
Herein, Mn2+ ions were doped on CdSe nanoparticles to study the optical and photovoltaic properties of the QDSSCs. We investigate how changing the thickness of Cd1−xMnxSe films affects efficiency performance. Besides, in order to explain this result, the experimental I–V curve was also used to determine the resistances at the interfaces and the resistances diffusion of the devices. This dynamic resistance can be compared with that of electrochemical impedance spectra.
Experiment
Materials
Na2SO3, NaOH, Cd(CH3COO)2.2H2O, Zn(NO3)2, Na2S.9H2O, methanol, TiCl4, and Mn(CH3COO)2.2H2O were purchased from Merck and the fluorine-doped tin oxide was from Dyesol.
Preparation
TiO2 Films
The TiO2 paste was deposited onto transparent conducting substrates F-doped SnO2 (FTO) with 7 Ω cm−2 of the sheet resistance. The FTO/TiO2 film was sintered in air at 500°C for 30 min.
TiO2/CdS Films
The FTO/TiO2 film immersed in 0.1 M Cd2+ solution [2.66 g Cd(CH3COO)2.2H2O was mixed with 100 ml of de-ionized water] followed by 0.1 M S2− solution (2.4 g Na2S.9H2O was dissolved in 100 ml of methanol). All processes were repeated from one to three times (denoted FTO/TiO2/CdS photoelectrode).
TiO2/CdS/Cd1−xMnxSe Photoelectrode
The Se powder was mixed with Na2SO3 (0.6 M) and 100 ml of pure water at 70°C for about 7 h. To accommodate the doping of Mn metal ion, relevant molar concentrations of 0.3 mM of Mn(CH3COO)2.2H2O were mixed with Cd(CH3COO)2.2H2O anion source. The SILAR process of CdSe and Mn-doped CdSe QDs was similar to that of CdS except that 15 min and 50°C were required for dipping the TiO2/CdS film in the Se aqueous solution. Then, the FTO/TiO2/CdS film was dipped in the above solution for 1 min before dipping in Se2− solution for 1 min at 80°C (called 1 layer).
Polysulfide solution was made by dissolving 0.5 M Na2S.9H2O, 0.2 M S, and 0.2 M KCl in DI water/methanol (7:3 by volume). The Cu2S counter electrode was synthesized through chemical bath deposition according to a previous publication (Fan et al., 2009). Briefly, 0.24 g CuSO4 was dissolved in 60 ml of DI in a glass bottle. N2 was bubbled through the water for 10 min to remove the dissolved oxygen from the system. Then 0.37 g of Na2S2O3.5H2O was mixed in the solution, and the color turned to light green. Afterwards, a clean FTO glass was immersed in the solution, with its conductive surface facing down and had an angle against the wall. The system was then settled in the water bath of 90°C and kept for 1 h. The Cu2S crystal would directly grow onto the conductive surface of FTO glass. Finally, the as-prepared Cu2S-coated FTO glass sample was rinsed with deionized water and dried in air. The post-heat treatment was carried out in an N2 atmosphere at 200°C for 30 min and a structure of device was shown in Figure 1.
Characterization
The scanning electron microscopy (SEM) with a JEOL 7500 F high-resolution scanning electron microscope was used to determine the morphology of films. The structure of materials were recorded by an X-ray diffraction pattern, Philips model, and the absorption spectrum was investigated by a JASCO V-670. The I–V curve was recorded using simulated AM 1.5 sunlight with an output power of 100 mW cm−2. The resistances of QDSSCs were studied by electrochemical impedance spectroscopy (EIS) Series G750.
Results and Discussion
Figures 2A–D are the FE-SEM and cross-section of TiO2/CdS(3), TiO2/CdS(3)/Cd0.8Mn0.2Se(3), and TiO2/CdS(3)/Cd0.8Mn0.2Se(3) photoanodes with a Mn2+ concentration of 0.2 and a thickness of three layers, respectively. The porous TiO2 nanoparticles look like a sphere, which can be seen obviously in the inset image with 65 nm of an average size. Every layer of TiO2/CdS(3), TiO2/CdS(3)/Cd0.8Mn0.2Se(3), and TiO2/CdS(3)/Cd0.8Mn0.2Se(3) photoanodes was determined to be ~11.606, 11.750, and 12.056 μm from Figures 2B–D, respectively. Moreover, 0.5 μm in Figure 2C (0.563 μm in Figure 2D) and 11.006 μm are the thickness of FTO and the TiO2/CdS(3) film without FTO. The energy peaks related to Ti and O elements in the TiO2 film and Cd, Se, and S elements of CdS and CdSe nanocrystal were clearly found in the EDX spectra of TiO2/Cd1−xMnxSe/CdSe photoanode. Si and C energy peaks had been originated from FTO and excessive organic solution remaining in the layer (since the electrodes were sintered in vacuum), respectively. Mn energy peaks came from the anion precursor solution. The EDX spectra confirmed that QDs had been assembled and crystallized on the TiO2 layer (Figure 2E).
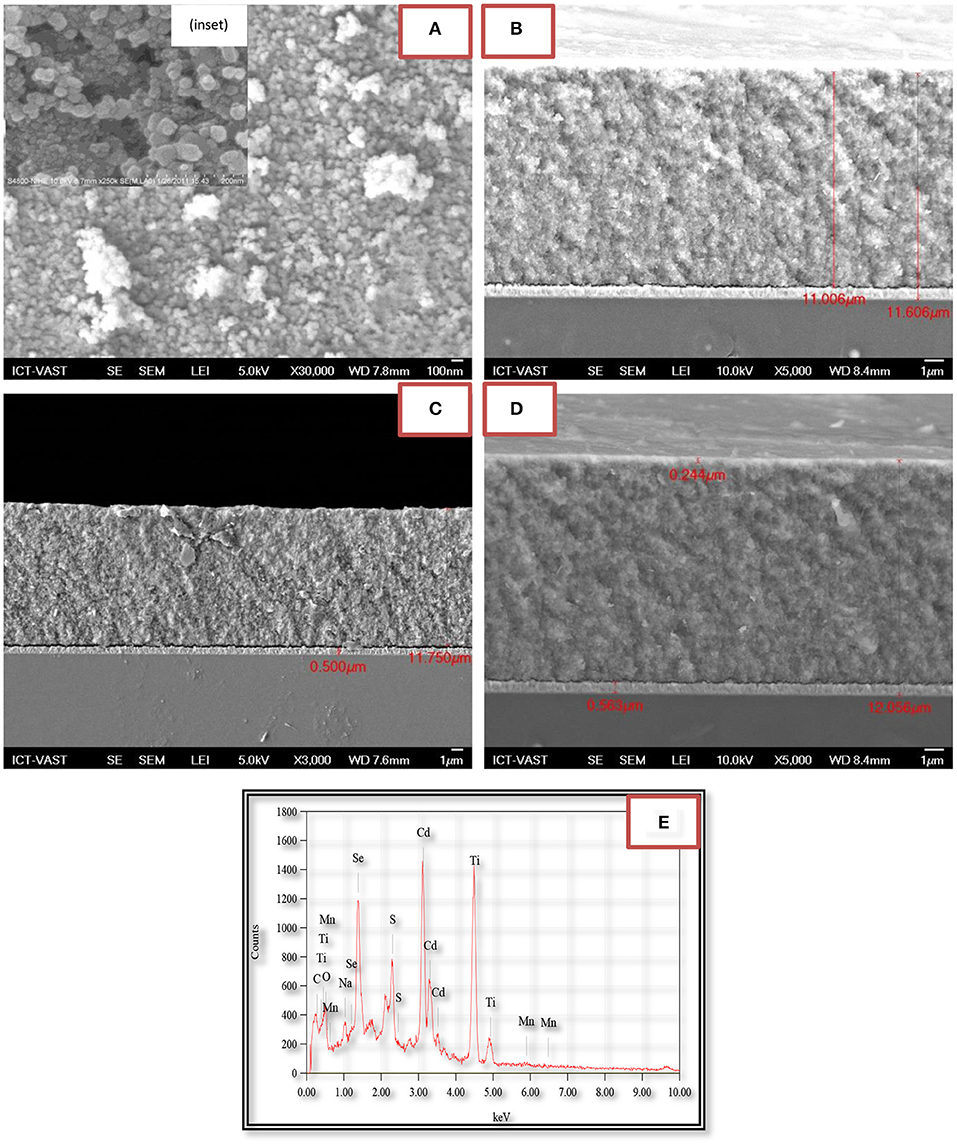
Figure 2. FE-SEM of the TiO2 film (inset) and (A) TiO2/CdS/Cd0.8Mn0.2Se. Cross-sectional FE-SEM of (B) TiO2/CdS, (C) TiO2/CdS/Cd0.8Mn0.2Se, (D) TiO2/CdS/Cd0.8Mn0.2Se photoanodes, and (E) energy dispersive X-ray (EDX) of TiO2/CdS/Cd0.8Mn0.2Se photoanode.
The optical properties of TiO2/CdS/Cd1−xMnxSe photoelectrodes were investigated by UV-Vis spectra with different thicknesses (Supplementary Table 1). The red shift is more pronounced with the increase of SILAR cycles due to the growth and thickness of film and attributed to the size quantization effect. This indicates that the high absorption coefficient of CdSe:Mn2+ QDs is attributed to TiO2 nanoparticles, which are extended to almost the whole visible region as corresponding to SILAR cycles from 1 to 3 (Figure 3A). However, a decline in overall absorption was observed when SILAR cycle is higher than 3. This can be attributed to the aggregation of CdSe:Mn2+ nanocrystal due to decreasing photocurrent and increase in dynamic resistances (Singh et al., 2008; Bhupendra et al., 2011; Cao et al., 2015; Muthalif et al., 2016). Furthermore, the Tauc plot and additional information on it are shown in Table 1, Supplementary Table 2 and Figure 3B. The bandgap of QDs decreased from 2.04 eV for Cd0.8Mn0.2Se (1) to 1.7 eV for Cd0.8Mn0.2Se (3) QDs. This result shows that there is a strong influence of the doped concentration and thickness on the energy band structure of the CdSe host material (Gopi et al., 2015).
Figure 4A exhibits an alignment energy of photoanode, which includes a dopant energy in the bandgap of CdSe QDs caused by the shift peak, an increasing absorption intensity (shown in Figure 3A), and the (αhν)2 vs. (hν) curves (shown in Figure 3B). The results are also confirmed by the time-resolved photoluminescence spectrum in Figure 4B and the data in Supplementary Table 3 and Table 1. In a similar manner, the lifetimes of charges in the CB of CdSe nanoparticles were shorter than those of Cd0.8Mn0.2Se QDs. In particular, the lifetimes of charges increase from 198.1 to 206.5 ns when SILAR cycles changed from 1 to 3. The probability of charge transfer from Cd0.8Mn0.2Se to CdS and TiO2 was facilitated as large lifetimes. However, a decline in the lifetimes was recorded with loading higher than three layers due to the aggregation of Cd0.8Mn0.2Se nanoparticles.
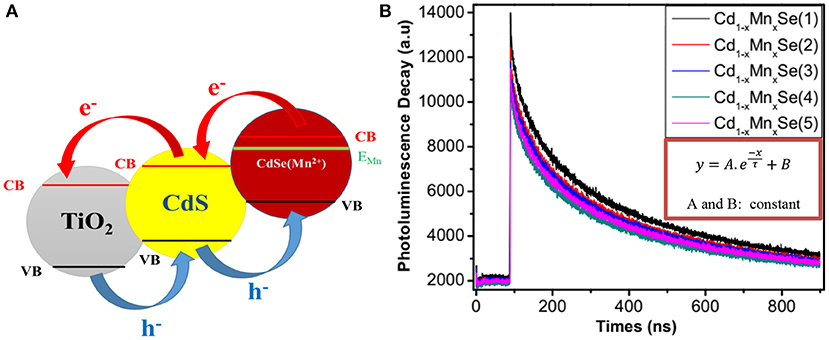
Figure 4. (A) Alignment energy and (B) time - resolved Photoluminescence of TiO2/CdS(3)/Cd0.8Mn0.2Se photoanodes.
Herein, both the I–V model from Refs. (Thongpron and Kirtikara, 2006; Thanh et al., 2015) and our experimental I–V curves were used to calculate the external dynamic resistance (RD) and the internal dynamic resistance (Rd), the series resistance (Rs), and the shunt resistance (RSH) of cells. It is necessary and more important to obtain the reliable characterization in the QDSSCs when the dynamic parameters were determined. We can control and determine the amount of loss mechanism as accurately as possible to improve the efficiency performance in the next work (Sze and Ng, 1981).
The photo current density (Iph) and open voltage circuit (VOC) of a solar cell is given by
with .
RD and Rd are the external dynamic resistance and internal dynamic resistance of the equivalent circuit of solar cells.
The shunt resistance (RSH) was obtained:
where Vo is the initial voltage.
In order to determine the performance, we recorded the I–V curves of QDSSCs with the different layers of Cd1−xMnxSe QDs, which is shown in Figure 5. In comparison, It is obvious that the optimized thickness of Cd1−xMnxSe (3 layers) QDs made contributions to boost the efficiency of QDSSCs (~3.8%) (Supplementary Table 4). This result is suitable to that of UV-Vis, lifetime, and IES.
On the whole, our view is that resistances showed up as the increasing SILAR cycles of Cd1−xMnxSe films (Sze and Ng, 1981). The result agrees well with that of the I–V curve (3.8% of efficiency). Furthermore, RSH was calculated from Equation 5, and it depended on the technology process. The values of RSH are large, corresponding to a good QDSSC. Looking at Table 1, it reveals that the RSH of CdS/Cd1−xMnxSe co-sensitized TiO2 is the largest. This is also confirmed by the long lifetimes of charges with loading SILAR cycles more than 3. In brief, the dynamic resistances, saturated current intensity, lifetimes of charges, and bandgap depend on the thickness of TiO2/CdS(3)/Cd0.8Mn0.2Se(3) with the highest efficiency of 3.8%.
Figure 6A gives information about the circuit, which corresponds to the QDSSCs. Figure 6B shows the experimental Nyquist plots of devices corresponding to the resistance at the surface of the polyelectrolyte/counter electrode (denoted as Rct1) and the diffuse resistance in the TiO2 film and TiO2/QDs surface (denoted as Rct2) (Veerathangam et al., 2017). The lifetime of excited electron (τn) is determined from Figure 4B, and the capacitance (cμ) can be determined by and listed in Supplementary Table 5 and Table 2. As a rule, the Cd0.8Mn0.2Se (1), Cd0.8Mn0.2Se (2), Cd0.8Mn0.2Se (3), Cd0.8Mn0.2Se (4), and Cd0.8Mn0.2Se (5) photoelectrodes have significantly changed in the photovoltaic because layers played a role in the recombination process. It is obvious that the thicker the film is, the larger resistance becomes. From Table 2, the resistances of four to five layers are larger than the resistance of three layers, while the excited electrons' lifetime and capacitances are much lower (Omid et al., 2015). Above all, the performance increased because of a rise in CB of the Cd1−xMnxSe QDs and a shift of the absorption peak after doping (shown in Figure 4A).
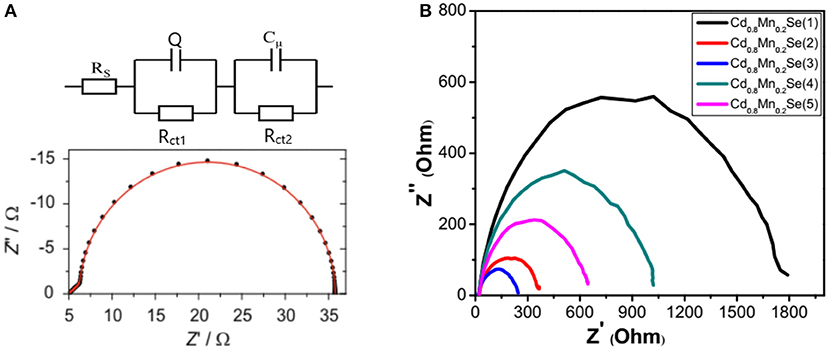
Figure 6. (A) The theoretical circuit and theoretical Nyquist plot and (B) the experimental Nyquist plot of solar cells.
Table 1 illustrates the value of dynamic resistances from one illuminated I–V curve and EIS with the same conditions. Looking at the graph, it is immediately obvious that they depend on the SILAR cycles of deposition of Cd0.8Mn0.2Se with the same rules. In this case, the results show that the RD, Rd, Rct1, and Rct2 are the smallest with loading at three SILAR cycles of deposition, but the value of RSH is the largest. We noted larger RSH indicates a better quality of QDSSCs. The trend of the recombination resistance (Rct2) of all devices can clearly be analyzed when the SILAR cycles of deposition are changed. The RD, Rd, and Rct2 are characterized by the dynamic processes, dynamic resistances, and resistance transfer at surfaces of TiO2/QDs. With the smallest RD, Rd, and Rct2, the optimum energy conversion efficiency was obtained ~3.8% at three cycles of deposition (Supplementary Table 6). This is completely suitable with the results of UV-Vis and lifetimes.
Conclusions
To summarize, photoelectrodes such as TiO2/CdS/Cd1−xMnxSe have successfully been prepared using SILAR. The thickness of the Cd1−xMnxSe film affected the optical and photovoltaic properties of QDSSCs. The J–V curves show that the conversion efficiency is improved due to the optimized thickness at three cycles and Cd1−xMnxSe QDs. In addition, this result is also confirmed by the shift of absorption toward to the visible region, increasing lifetimes, and reducing charge recombination at the polyelectrolyte/counter electrode, TiO2/Cd0.8Mn0.2Se/polyelectrolyte interfaces, and diffusion resistance in TiO2 films. As a result, QDSSCs exhibited a high conversion efficiency of 3.8%.
Data Availability Statement
All datasets generated for this study are included in the article/Supplementary Material.
Author Contributions
HT and DP conceived and planned the experiments and carried out the experiments, contributed to sample preparation, took the lead in writing the manuscript. They also performed the experiments about the structural materials and contributed to the analysis of the new results of the manuscript.
Conflict of Interest
The authors declare that the research was conducted in the absence of any commercial or financial relationships that could be construed as a potential conflict of interest.
Acknowledgments
The authors would like to thank the University of Science, VNU-HCM, Vietnam.
Supplementary Material
The Supplementary Material for this article can be found online at: https://www.frontiersin.org/articles/10.3389/fmats.2019.00304/full#supplementary-material
References
Abdellah, M., Marschan, R., Zıdek, K., Messing, M. E., Abdelwahab, A., Chábera, P., et al. (2014). Hole trapping: the critical factor for quantum dot sensitized solar cell performance. J. Phys. Chem. 118, 25802–25808. doi: 10.1021/jp5086284
Beard Matthew, C. (2011). Multiple exciton generation in semiconductor quantum dots. J. Phys. Chem. Lett. 2, 1282–1288. doi: 10.1021/jz200166y
Bhupendra, B. S., Jana, S., and Pradhan, N. (2011). Doping cu in semiconductor nanocrystals: some old and some new physical insights. J. Am. Chem. Soc. 133:1007–15. doi: 10.1021/ja1089809
Cao, S., Jialong, Z., Weiyou, Y., Chengming, L., and Jinju, Z. (2015). Mn2+-doped Zn-in-S quantum dots with tunable bandgaps and high photoluminescence properties. J. Mater. Chem. 3, 8844–8851. doi: 10.1039/C5TC01370D
Chen, K., Zhou, J., Chen, W., Chen, Q., Zhou, P., and Liu, Y. (2016). A green synthesis route for the phase and size tunability of copper antimony sulfide nanocrystals with high yield. Nanoscale 8, 5146–5152. doi: 10.1039/C5NR09097K
Duan, J., Tang, Q., He, B., and Yu, L. (2014). Electrochim. Acta 139 381–385. doi: 10.1016/j.electacta.2014.06.165
Fan, S. Q., Cao, R. J., Xi, Y. X., Gao, M., Wang, M. D., and Kima, D. H. (2009). CdSe quantum dots as co-sensitizers of organic dyes in solar cells for red-shifted light harvesting. J. Optoelectr. Adv. Mater. Rap Commun. 10, 1027–1033.
Fang, B., Kim, M., Fan, S. Q., Kim, J. H., Wilkinson, D., Ko, J., et al. (2011). Facile synthesis of open mesoporous carbon nanofibers with tailored nanostructure as a highly efficient counter electrode in CdSe quantum-dot-sensitized solar cells. J. Mater. Chem. 21, 8742–8748. doi: 10.1039/c1jm10113g
Fang, J., Wu, J., Lu, X., Shen, Y., and Lu, Z. (1997). Sensitization of nanocrystalline TiO2 electrode with quantum sized CdSe and ZnTcPc molecules. Chem. Phys. Lett. 270, 145–51. doi: 10.1016/S0009-2614(97)00333-3
Gimenez, S., Mora-Sero, I., Macor, L., Guijarro, N., Lana-Villarreal, T., and Gomez, R. (2009). Improving the performance of colloidal quantum-dot-sensitized solar cells. Nanotechnology 20:295204. doi: 10.1088/0957-4484/20/29/295204
Gonzalez-Pedro, V., Xu, X., Mora-Sero, I., and Bisquert, J. (2010). Modeling high-efficiency quantum dot sensitized solar cells. ACS Nano 4, 5783–90. doi: 10.1021/nn101534y
Gopi, C. V. V. M., Bae, J.-H., Venkata-Haritha, M., Kim, S.-K., Lee, Y.-S., Sarat, G., et al. (2015). One-step synthesis of solution processed time-dependent highly efficient and stable PbS counter electrodes for quantum dot-sensitized solar cells. RSC Adv. 5, 107522–107532. doi: 10.1039/C5RA22715A
Gratzel, M. (2003). Dye-sensitized solar cells. J. Photochem. Photobiol. 4, 145–53. doi: 10.1016/S1389-5567(03)00026-1
Hodes, G., Albu-Yaron, A., Decker, F., and Motisuke, P. (1987). Three-dimensional quantum-size effect in chemically deposited cadmium selenide films, Phys. Rev. 36, 4215–4221. doi: 10.1103/PhysRevB.36.4215
Jiao, S., Du, J., Du, Z., Long, D., Jiang, W., Pan, Z., et al. (2017). Nitrogen-doped mesoporous carbons as counter electrodes in quantum dot sensitized solar cells with a conversion efficiency exceeding 12%. J. Phys. Chem. Lett. 8, 559–564. doi: 10.1021/acs.jpclett.6b02864
Jumabekov, A. N., Siegler, T. D., and Cordes, N. (2014). Comparison of solid-state quantum-dot-sensitized solar cells with exsitu and in situ grown PbS quantum dots. J. Phys. Chem. 118, 25 853–856. doi: 10.1021/jp5051904
Lee, Y. L., and Lo, Y. S. (2009). Highly efficient quantum-dot-sensitized solar cell based on co-sensitization of CdS/CdSe. Adv. Funct. Mater. 19, 604–609 doi: 10.1002/adfm.200800940
Li, Y., Wang, L., Li, Z., Liu, Y., Peng, Z., Zhou, M., et al. (2019). Synthesis and photocatalytic property of V2O5@ TiO2 core-shell microspheres towards gaseous benzene. Catal. Today 321, 164–171. doi: 10.1016/j.cattod.2018.02.029
Liji Sobhana, S. S., Vimala Devi, M., and Sastry, T. P. (2011). CdS quantum dots for measurement of the size-dependent optical properties of thiol capping. J. Nanopart. Res. 13:1747. doi: 10.1007/s11051-010-9934-1
Lin, L., Zou, X., Zhou, H., and Teng, G. (2014). Cu-doped-CdS/In-Doped-CdS cosensitized quantum dot solar cells. J. Nanomat. 2014:314386. doi: 10.1155/2014/314386
Liu, Y., Wang, H., Chen, K., Yang, T., Yang, S., and Chen, W. (2019b). Acidic site-assisted ammonia sensing of Novel CuSbS2 quantum dots/reduced graphene oxide composites with an ultralow detection limit at room temperature. ACS Appl. Mater. Interfaces 11, 9573–9582. doi: 10.1021/acsami.8b20830
Liu, Y., Wang, L., Wang, H., Xiong, M., Yang, T., and Zakharova, G. S. (2016). Highly sensitive and selective ammonia gas sensors based on PbS quantum dots/TiO2 nanotube arrays at room temperature. Sens. Actuat. 236, 529–536. doi: 10.1016/j.snb.2016.06.037
Liu, Y., Zhou, M., Zhang, W., Chen, K., Mei, A., Zhang, Y., et al. (2019a). Enhanced photocatalytic properties of TiO2 nanosheets@ 2D layered black phosphorus composite with high stability under hydro-oxygen environment. Nanoscale 12, 5674–5683. doi: 10.1039/C8NR10476J
Mendoza-Perez, R., Sastre-Hernandez, J., Puente, G., and Vigil-Galan, O. (2009). Solar energymater. Solar Cells 8:79. doi: 10.1016/j.solmat.2008.09.016
Muthalif, M. P. A., Lee, Y. S., Sunesh, C. D., Kim, H. J., and Choe, Y. (2016). Enhanced photovoltaic performance of quantum dot-sensitized solar cells with a progressive reduction of recombination using Cu-doped CdS quantum dots. Appl. Surf. Sci. 396, 582–589. doi: 10.1016/j.apsusc.2016.10.200
Omid, A., Salavati-Niasari, M., and Farangi, M. (2015). Enhancement of dye-sensitized solar cells performance by core shell Ag@ organic (organic = 2-nitroaniline, PVA, 4-choloroaniline and PVP): effects of shell type on photocurrent. Electrochim. Acta 153, 90–96. doi: 10.1016/j.electacta.2014.11.195
Peng, Z. A., and Peng, X. (2001). Formation of high-quality CdTe, CdSe, and CdS nanocrystals using CdO as precursor. J. Am. Chem. Soc. 123, 183–184. doi: 10.1021/ja003633m
Shen, X., Jia, J., and Lin, Y. (2015). Enhanced performance of CdTe quantum dot sensitized solar cell via anion exchanges. J. Power Sour. 277, 215–218. doi: 10.1016/j.jpowsour.2014.12.022
Shen, Y.-J., and Lee, Y.-L. (2008). Assembly of CdS quantum dots onto mesoscopic TiO2 films for quantum dot-sensitized solar cell application, Nanotechnology 19:045602. doi: 10.1088/0957-4484/19/04/045602
Singh, S. B., Limaye, M. V., Lalla, N. P., and Kulkarni, S. K. (2008). Copper-ion-induced photoluminescence tuning in CdSe nanoparticles. J. Lumin. 128, 1909–1912. doi: 10.1016/j.jlumin.2008.05.022
Sze, S. M., and Ng, K. K. (1981). Physics of Semiconductor Devices. New York, NY: John Willey & Sons.
Tan Phat, N., Tung Ha, T., Thao Nguyen, T., Phuong Ho, N., Dat Huynh, T., Vinh Lam, Q., et al. (2018). Effect of Cu2+ ions doped on the photovoltaic features of CdSe quantum dot sensitized solar cells. Electrochim. Acta 20, 16–23. doi: 10.1016/j.electacta.2018.06.046
Thanh, T. H., Quang, V. L., and Huynh Thanh, D. (2015). Determination of the dynamic resistance of the quantum dots solar cells by one I–V curve and electrochemical impedance spectra. Solar Energy Mater. Solar Cells 143, 269–274. doi: 10.1016/j.solmat.2015.07.007
Thongpron, J., and Kirtikara, K. (2006). “Voltage and frequency dependent impedances of monocrystalline, polycrystalline and amorphous silicon solar cells,” 2006 IEEE 4th World Conference on Photovoltaic Energy Conference (Waikoloa, HI: IEEE), May 7–12. doi: 10.1109/WCPEC.2006.279922
Veerathangam, K., Pandian, M. S., and Ramasamy, P. (2017). Photovoltaic performance of Ag-doped CdS quantum dots for solar cell application. Mater. Res. Bull. 94, 371–377. doi: 10.1016/j.materresbull.2017.06.024
William Yu, W., Qu, L., Guo, W., and Peng, X. (2003). Experimental determination of the extinction coefficient of CdTe, CdSe, and CdS nanocrystals. Chem. Mater. 15, 2854–2860. doi: 10.1021/cm034081k
Keywords: nanomaterials, solar cell, photovoltaic, metal dopant, efficiency
Citation: Tung HT and Phuc DH (2019) Effect of Cd1-xMnxSe Alloy Thickness on the Optical and Photovoltaic Properties of Quantum Dot-Sensitized Solar Cells. Front. Mater. 6:304. doi: 10.3389/fmats.2019.00304
Received: 27 July 2019; Accepted: 14 November 2019;
Published: 11 December 2019.
Edited by:
Joe Shapter, University of Queensland, AustraliaCopyright © 2019 Tung and Phuc. This is an open-access article distributed under the terms of the Creative Commons Attribution License (CC BY). The use, distribution or reproduction in other forums is permitted, provided the original author(s) and the copyright owner(s) are credited and that the original publication in this journal is cited, in accordance with accepted academic practice. No use, distribution or reproduction is permitted which does not comply with these terms.
*Correspondence: Dang Huu Phuc, ZGFuZ2h1dXBodWNAdGR0dS5lZHUudm4=