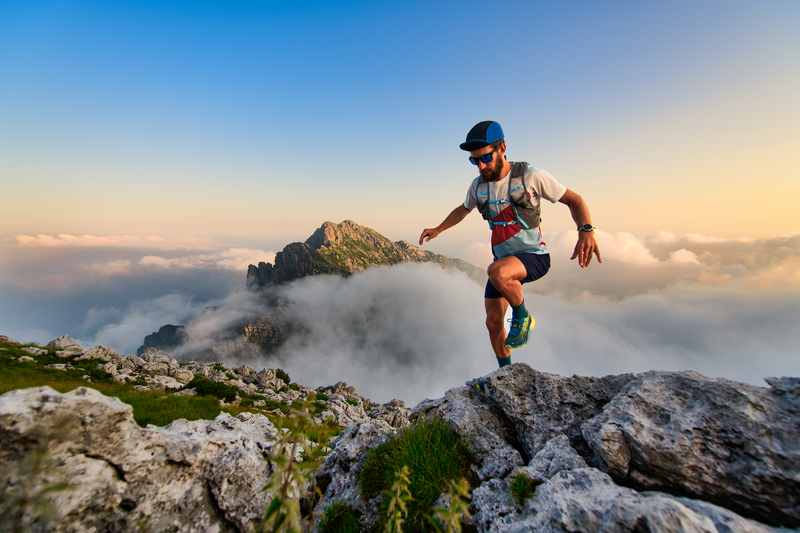
95% of researchers rate our articles as excellent or good
Learn more about the work of our research integrity team to safeguard the quality of each article we publish.
Find out more
ORIGINAL RESEARCH article
Front. Mater. , 10 December 2019
Sec. Biomaterials
Volume 6 - 2019 | https://doi.org/10.3389/fmats.2019.00274
This article is part of the Research Topic Catechol and Polyphenol Chemistry for Smart Polymers View all 7 articles
Indwelling urethral catheters are widely used in hospitalized patients. However, they are associated with bacterial infection and biofilm formation due to the suboptimal surface properties of the elastic materials used for the catheters. Although there are several antibacterial coating technologies to modify the surface properties of the catheter including hydrophilic polymeric coating, the risk of infection is still high given the absence of reactive functional groups on the surface of elastomers. In this study, we describe the use of catechol-functionalized hydrophilic polymers and explore strategies to create antibacterial hydrogel coatings. Three different types of catechol-functionalized polymers, chitosan, hyaluronic acid, and human serum albumin were synthesized and deposited using simple dip-coating method. All of the tested polymers could coat different types of elastomers widely used for urethral catheters independent of the surface properties while the thickness of the coating could be controlled by the number of depositions. The coating formed stable water-containing lubricant surface beneficial as a physical repellant of microbial attachment. In addition, the coating could be combined with additional antibacterial agents such as silver nanoparticles to maximize the antibacterial effect on the surface of urethral catheter materials.
Urethral catheters are inserted through urinary tract to drain urine from urethra and it is estimated to be used by 15–25% of hospitalized patients and 75% of patients at critical care (Shackley et al., 2017; Singha et al., 2017), including patients with urethral incontinence who require short- and long-term usage of urethral catheters. Each year, around 100 million urethral catheters are sold worldwide, 25 millions of which are in the United States (Saint et al., 2000). Elastomers are commonly used materials for urethral catheters, such as latex rubber, silicone, polyvinyl chloride (PVC), and polyurethane (PU), given their excellent conformability and mechanical strength to form a robust channel for drainage (Dellimore et al., 2013). However, in general, the surface of elastomers has high lateral friction coefficient, causing the adhesion of urine components and external bacteria such as Escherichia coli (E. coli) and Staphylococcus aureus (S. aureus), which is a pivotal step in their colonization and further biofilm formation (Swartjes et al., 2014; An et al., 2017). Furthermore, in the presence of urease-producing bacteria forming salt crystals on the surface, the catheter can be easily roughened to further increase friction, if not treated timely, causing massive obstruction (i.e., encrustation), antibiotic resistance of bacteria, and severe bacterial infection (Wang et al., 2015). To solve this problem, several antibacterial coating strategies have been developed including passive anti-adhesion to repel bacterial adhesion [e.g., poly(N-hydroxyethylacrylamide), and zwitterionic polymers], and active biocidal coating that can directly kill the bacteria on the surface (e.g., chitosan, N-halamine polymer, and other polymers with strong positive charges) combined with antibiotic agents to maximize the efficacy (e.g., silver; Dallas et al., 2011; Zhao et al., 2013; Ng et al., 2014; GhavamiNejad et al., 2016; Li et al., 2017; Yong et al., 2019). Along with the absence of surface functional groups in the elastomers used for urethral catheters, surface modification requires material-specific complexes and toxic chemical reactions producing coatings that lack appropriate mechanical stability for long-term use in a dynamic environment in vivo, hampering the translation into clinic (Voccia et al., 2006; Such et al., 2010). As a result, catheterization still causes patient discomfort and distress; and is a leading cause for urinary tract infection in hospitalized patients (i.e., catheter-associated urinary tract infection, CAUTI), with 26% incidence rate after only 2–10 days of catheterization (Saint, 2000; Gerard et al., 2003; Siderias et al., 2004). Therefore, there is a critical unmet need for a method producing robust and long-lasting low-friction antibacterial coating on elastomeric materials used in urethral catheters potentially capable of antibacterial drug loading and sustained release.
Here, we explore the use of catechol-functionalized polymers for a simple and robust antibacterial coating on elastomeric urethral catheter materials without the need of an additional surface functionalization process. Under physiological or alkaline conditions, catechol groups can be readily transformed into more reactive o-quinones, which subsequently can react with many other functional groups such as primary and secondary amines, carboxyls, and other adjacent catechol groups. Especially, when two of these functional groups are present in a single molecule (e.g., dopamine), this oxidative reaction results in polymerization of the molecules forming a robust coating on surfaces of multiple different types of materials without the need for surface functionalization. Due to the high affinity to multiple different surfaces and extremely simple procedure, the catechol moiety and its in-situ polymerization (also called polydopamine) have been extensively studied to prime the biomedical materials including elastomers with functional groups and normalize the surface properties of different materials (Lee et al., 2008; Yang et al., 2012; Hong et al., 2013). In this paper, we evaluated the use of polydopamine and catechol-functionalized polymers, using chitosan as a primary example that has excellent antibacterial properties, to coat elastomeric urethral catheter materials to prime the surfaces and coat an antibacterial hydrogel film with low friction. Stable chitosan hydrogel coatings with micrometer-scale thickness were formed on the candidate substrates including PU and PVC using simple dip coating methods with catechol-conjugated chitosan solutions in slightly alkaline buffer (pH 8.5 Tris buffer solution). The hydrogel coating based on the catechol-conjugated chitosan could reduce the surface friction and this coating strategy could be used to coat other polymers with different chemical properties (e.g., hyaluronic acid, proteins). Antibacterial silver nanoparticles could be incorporated into the hydrogel by simply adding silver nitrate solutions using the conjugated catechol groups as reducing agents. The hydrogel coating on the urethral catheter materials could minimize the binding of bacteria on catheter surfaces in combination with antibacterial agents such as silver nanoparticles, demonstrating its potential for clinical applications.
Hyaluronic acid (HA) (17 kDa) was purchased from Lifecore biomedical (Chaska, MN, USA). Human serum albumin (HSA) (66~67 kDa), Chitosan (50~190 kDa, 75–85% deacetylated), dopamine hydrochloride, 3,4-dihydroxy-hydrocinnamic acid, 1-Ethyl-3-(3-dimethylaminopropyl) carbodiimide hydrochloride (EDC), and N-hydroxysuccinimide (NHS), 2-(N-morpholino) ethanesulfonic acid (MES) buffer, Tris buffer, Silver Nitrate (AgNO3), and RBS-35 detergent were purchased from Sigma (St. Louis, MO, USA). Escherichia Coli (E. coli) (ATTC# 29906) and Staphylococcus aureus (S. aureus) (ATTC# 6341) were purchased from ATCC (Manassas, VA, USA). The E. coli medium used in the study was composed of 5 g of NaCl (Sigma), 2.5 g of Yeast Extract (Sigma), and 5 g of Tryptone (Sigma) whereas the S. Aureus medium was composed of 15 g/L Tryptic Soy Broth (Sigma).
Each macromolecule (HA, chitosan, and HSA) was modified with unoxidized catechol groups using EDC chemistry. For catechol-conjugation to HA (HA-C), HA (1.2 mmol) was dissolved in 50 mL of 2-(N-morpholino) ethanesulfonic acid (MES) buffer (50 mM, pH 4.7) at 2% concentration to which dopamine hydrochloride (2.4 mmol) and EDC (2.4 mmol) solutions were added dropwise. The pH was adjusted to 4.7 by adding 1N HCl solution to water and the reaction mixture was stirred for 4 h at room temperature. Then, 4 mL of saturated NaCl solution (30%) were added to the reaction solution and dialyzed using a dialysis membrane with molecular weight cut-off (MWCO) of 1,000 Da against pH adjusted distilled deionized water (DDW) (pH 4.5) for 3 days and was lyophilized. For catechol-conjugation to chitosan (CHI-C), chitosan (3.1 mmol) was dissolved in 50 mL of pH adjusted MES buffer (50 mM, pH 4.7) to which 3,4-dihydroxyhydrocinnamic acid (3.1 mmol) and EDC (3.1 mmol) solutions were added to the reaction solution dropwise. After 4 h, saturated NaCl solution (30%) was added to the reaction solution, dialyzed (MWCO 1,000 Da), and lyophilized. To synthesize catechol-conjugated HSA (HSA-C), HSA (66 kDa, 15 μmol, 70 lysine groups per protein) was dissolved in 50 mL of pH adjusted MES buffer (50 mM, pH 4.7) and reacted with 3,4-dihydroxy-hydrocinnamic acid (750 μmol) and EDC (75 μmol). After 4 h, saturated NaCl solution (30%) was added to the reaction solution, dialyzed (MWCO 1,000 Da), and lyophilized. Degrees of catechol substitution for the functionalized polymers were measured by UV-Vis spectroscopy, especially using the intensity of the peak at 280 nm corresponding to the catechol groups. Dopamine hydrochloride (for HA) or 3,4-dihydroxy-hydrocinnamic acid (for chitosan and HSA) solutions in pH adjusted MES buffer (50 mM, pH 4.7) were used to plot standard curves. For HSA, the percentage degree of catechol substitution was calculated considering the total number of epsilon-amines (i.e., lysine residues) in a single protein as 100%.
Polyurethane (PU), polyvinyl chloride (PVC), and polydimethylsiloxane (PDMS) substrates were cut into 1 × 1 cm2 size substrates and sequentially sonicated in DDW, 5% RBS-35 detergent solution, and DDW for 15 min each. The substrates were immersed in dopamine hydrochloride solution (2 mg/mL Tris buffer, pH 8.5) and then placed on a 3D shaker (TW3, FINEPCR, Seoul, Korea) for 3 h. All the substrates were then sonicated in DDW for 15 min and dried with nitrogen gas. Unoxidized HSA-C or CHI-C was dissolved in Tris buffer (10 mM, pH 8.5) at 5 mg/mL and HA-C was dissolved at 3 mg/ml. Polydopamine coated substrates were immersed in appropriate solutions for 4 h. Subsequently, the substrates were withdrawn, carefully washed with DDW and dried with nitrogen gas. This process was repeated for several cycles as mentioned in the Results and Discussion section. All the samples were then washed using DDW and incubated at 50°C for 15 min to maximize crosslinking between unreacted catechols and fully dried for storage.
CHI-C and HSA-C coatings were prepared on each substrate using the described protocols. To observe the surface morphology of modified PU substrate, the coated film was imaged with Scanning Electron Microscope (SEM) (S3400N, Hitachi High-Tech, Tokyo, Japan). To measure the water contact angle, PDMS, PVC, and PU substrates were prepared and coated with six layers of hydrogels, 6 deposition cycles of CHI-C, HA-C, and HSA-C. The hydrophilic property was characterized by contact angle measuring apparatus (SEO300A, Surface Electro Optics co., Suwon, Korea). Subsequently, the substrates were withdrawn, carefully washed with DDW and dried with nitrogen gas. An 18G syringe was used to make droplets and each photograph was taken 30 s after DDW dropped from the syringe. The image-processing software provided by the contact angle apparatus was used to calculate the angle at the junction of water droplet, the surface, and air.
CHI-C, HA-C, and HSA-C (6 deposition cycles) were coated on polydopamine coated PDMS, PVC, and PU as described in the previous section. The surface friction was characterized by scanning probe microscope (SPM) (XE-100 SPM, Park Systems, Suwon, Korea). A silicon dioxide cantilever was used to measure lateral force in contact mode. All samples were immersed in phosphate buffered saline (PBS) (pH 7.4, Gibco BRL, Gaithersburg, MD, USA) for 10 min and immediately placed on the working table. A size of 5 × 5 μm was imaged at three different forces, 1, 2, 5 nN and the corresponding values in voltage (VLFM) were measured. Two lateral forces were measured in terms of forward and backward lateral voltages. The lateral voltage (Va−b) was taken as the half of the value between the forward and backward scan in the friction loop and relative friction force was calculated from the slope of VLFM/Va−b.
PU and PVC substrates were coated with CHI-C using 6 deposition cycles of CHI-C as described in the previous section. The composition of both modified and unmodified PU and PVC was examined using X-ray photoelectron spectroscope (XPS) (Sigma Probe, Thermo Scientific, Waltham, MA, USA). The modified PU and PVC were each placed in 2 mL of 1x PBS (pH 7.4, Gibco). The samples were placed on a 3D shaker inside an incubator at a set temperature of 37°C for 15 days. All the samples were carefully washed with DDW, dried with nitrogen gas, and examined with XPS. In addition, the coating of catechol-conjugates on PU substrates was visualized using FITC-conjugated CHI-C, HSA-C, and HA-C during 20 days of incubation in PBS at 37°C. The fluorescence signals were detected by an image analyzer (Image station 4000 MM, Kodak, New Haven, CT, USA).
The Polytetrafluoroethylene (PTFE) tube was used for the swelling study since it is light-impermeable and because it does not swell in solutions unlike PDMS, PVC, or PU. The tube was sonicated in DDW, 5% RBS-35 detergent solution, and DDW for 15 min each. The tube was immersed in dopamine hydrochloride solution (2 mg/mL Tris buffer, pH 8.5) and then placed on 3D shaker (TW3, FINEPCR, Seoul, Korea) for 3 h. The tube was then sonicated in DDW for 15 min and dried with nitrogen gas. A total of 10 deposition layers of FITC modified CHI-C hydrogels were adsorbed on a polydopamine coated PTFE tube and thoroughly dried with nitrogen gas. A cross-section of the coated tube was sliced with a stainless-steel blade and attached to a glass slide. The dried sample was incubated in an oven for 5 min and imaged with a confocal microscope before and after hydration in DDW for 10 min. Before taking the image, excess water was removed by gently wiping the tube. The thickness of the polymeric hydrogel coatings on PTFE tubing was measured by image analysis software ImageJ (U. S. National Institutes of Health, Bethesda, Maryland, USA) and average was taken from four random locations. Volume change of the hydrogel due to swelling was calculated as , where r = radius of the catheter, h = length of the catheter, x1 = hydrogel thickness in dry condition, x2 = hydrogel thickness after hydration. Since π, r, h remained constant, and the radius of catheter is larger than the hydrogel thickness by three orders of magnitude (Lawrence and Turner, 2005), the equation can be simplified to .
Silver nanoparticles (AgNPs) impregnated hydrogel film was prepared by placing 6 deposition cycles of CHI-C coated PU and PVC in a 10 mM AgNO3 solution for 10 min. The finished samples were washed with DDW and dried with nitrogen gas. Then, 2 mL of ten-fold diluted E. coli (0.06 OD600) and S. aureus (0.15 OD600) were added to unmodified, 6 deposition cycles of CHI-C modified, and CHI-C (6 deposition cycles)+10 mM AgNO3 modified PU and PVC. Medium were autoclaved before use. The samples were placed on a 3D shaker at 37°C for 8 h and the change in the bacteria population was measured at OD600 with Nanodrop spectrophotometer (ND-1000, Thermo Scientific). The number of live and dead bacteria in the solution was confirmed by Live/Dead BacLight Viability Kit (Invitrogen, Carlsbad, CA, USA) using spectrofluorophotometer (RF-5301pc, Shimadzu). To analyze the adherent bacteria on the surface, the samples were carefully washed with 1x PBS (pH 7.4, Gibco), stored at −20°C, and lyophilized. The samples were imaged with SEM (S3400N, Hitachi High-Tech).
To immobilize antibacterial coatings on the elastomers used for urethral catheters, a three-step process was used: (1) surface functionalization using polydopamine, (2) multi-layered polymer deposition using catechol-functionalized biomacromolecules, and (3) loading of antibacterial agents (Figure 1). Most of the elastomers used in urethral catheters such as silicone, PU, and PVC have no functional groups on the surface, and the surface coating should be preceded with toxic chemical functionalization process to introduce functional groups. Additionally, most of these surface-induced functional groups are only transient (e.g., oxidize quickly in air or water) and their density is low, so the coatings deposited based on these chemistries usually have low binding affinity and low stability (Makamba et al., 2003; Koberstein, 2004). In previous studies, the polydopamine coating has been shown to provide robust surface functional groups in high density on almost any type of surfaces including polymeric elastomers (e.g., PDMS, PU, and PVC; Lee et al., 2007; Du et al., 2014). Furthermore, catechol-functionalized polymers can be deposited on the surface in a similar manner, potentially forming multi-layered polymer networks via catechol-based reactions (e.g., catechol-catechol, or catechol-amine reactions). The polydopamine coating was done using a previously reported dip coating method in alkaline buffer (pH 8.5), and catechol-functionalized polymers were chemically immobilized on the surface (Lee et al., 2008). The polymer coatings can form a water layer on the coated surface and therefore their deposition on the urethral catheter can play key roles in lowering friction, hindering bacterial attachments (i.e., passive antibacterial) and providing a delivery depot of antibacterial agents (Sudarshan et al., 1992; No et al., 2002). Furthermore, there are a few polymers that have an active antibacterial effect (i.e., polymer itself can kill bacteria) such as chitosan. Given its excellent antibacterial properties and antifouling effects, chitosan has been widely used in other previous studies to create antibacterial coatings on urinary catheters via covalent functionalization on the substrate often in combination with silver nanoparticles or silver nitrates as additional antibacterial agents. Although this strategy showed potential, the reaction process is often cumbersome and the reaction is limited to the surface forming a very thin layer (e.g., single layer of chitosan brush) limiting the stability and the amount of additional antibacterial agents that can be potentially added to the chitosan layer. Therefore, our key hypothesis is that a hydrogel coating using CHI-C with a micrometer-level thickness could create a more stable, lubricated surface potentially with more room for the additional antibacterial agents. We also believe the catechol-based hydrogel coating strategy can be applied in many other biomacromolecules such as HA and proteins (e.g., albumin), although not yet commonly used for urinary catheter coatings (Brokke et al., 1991; Kinnari et al., 2005; Anisha et al., 2013; Drago et al., 2014; Romanó et al., 2017). To functionalize the polymers with catechol groups, EDC chemistry was used (Figure 1). The degrees of substitution were calculated based on the characteristic UV-Vis absorbance peak of catechol at 280 nm using dopamine (or 3,4-dihydroxy-hydrocinnamic acid) solutions as standards. Significant peak shifts or shoulder peaks were not observed, indicating that the catechol groups remained unoxidized and not polymerized after the synthesis and purification steps (the representative UV-Vis spectrum for CHI-C was included in Figure S1). The degree of catechol substitution for CHI-C was 35.0% (~6.5 catechol groups per polymer chain), and HA-C and HSA-C had the degree of catechol substitution of 15.3% (~5.8 catechol groups per polymer chain), and 11.9% (~8.26 catechol groups per protein), respectively.
Figure 1. Catechol-functionalized polymer hydrogel surface modification for urethral catheters. (A) Schematic illustration of the hydrogel deposition on urinary catheter tubing with low friction, low bacterial adhesion and antibacterial effect with silver nanoparticles (AgNPs). To immobilize antibacterial coatings on the elastomers, a three-step process was used: (1) surface priming using polydopamine coating, (2) polymer deposition using catechol-functionalized biomacromolecules, and (3) loading of antibacterial agents (e.g., AgNPs). (B) Synthesis schemes of catechol functionalized chitosan (CHI-C), hyaluronic acid (HA-C), and human serum albumin (HSA-C) using EDC mediated chemistry.
The catechol-tethered macromolecules were added onto the polydopamine-coated surfaces in alkaline condition, where in the catechol group transforms into quinone, which can react with neighboring proteins via aryl-aryl coupling or with amines via Michael-type addition reactions (Yang et al., 2012; Park et al., 2016), forming a network of polymers (i.e., hydrogel). Our strategy to deposit a polymer hydrogel directly on the substrate using the catechol-based reactions provides benefits over other strategies. The layer-by-layer approach provides only a nano-size single layer per deposition cycle and only works with two different polymers. Therefore, opposite charges neutralize to become less hydrophilic, which is not desirable for a hydrogel-based lubricated surface. Another hydrogel film coating strategy is to fabricate a separate hydrogel film and then attach it to the substrate. However, when the hydrogel is fabricated, the crosslinked polymers leave minimal binding functional groups and water layer on the surface leading to minimal surface functional groups available to react with the substrate and potential mismatch of mechanical properties with the substrate. To evaluate the growth kinetics of the hydrogel layer, the thickness of the hydrogel was measured in different time points of the deposition reaction process. When the CHI-C was dissolved in the pH 8.5 Tris buffer solution, the catechol groups are converted into their quinone forms to further react with the adjacent functional groups (e.g., other quinones or amines) on the polydopamine layer or other CHI-C polymers already attached to the substrate. As shown in Figure 2A, the deposition thickness in the first deposition cycle was saturated around 4 h while there are significantly more precipitates from aggregation after 4 h (Figure S2) suggesting that extensive inter-molecular crosslinking between CHI-C polymers was induced in the fabrication process, contributing to the drastic increase of the hydrogel thickness. We further explored using multiple deposition cycles to create a hydrogel coating (Figure 2B). For subsequent studies, 4 h was selected for one deposition cycle and to deposit the next layer a fresh polymer solution with no aggregates was used. In an experiment to deposit CHI-C on PU and PVC substrates, the thicknesses were saturated within 3–6 deposition cycles depending on the substrate and the presence or absence of polydopamine layer. To normalize the deposition cycles for all the samples, we used 6 layers or more for all the studies. In addition, we performed an experiment to show the changes in thickness during the deposition of CHI-C on PU and PVC without polydopamine pre-treatment (PD). Interestingly, the PU substrate was less dependent on the initial polydopamine layer whereas the thickness of CHI-C on the PVC substrate without the initial layer was significantly thinner than the layer formed on the PVC with the polydopamine layer. This is likely due to the higher capacity of hydrogen bonds of PU with catechol groups or the release of plasticizers from the PVC surface, although more detailed study would be required. This result suggests that PU can be directly coated with CHI-C hydrogels and other substrates with less affinity to CHI-C can be also used with additional polydopamine coating. Further SEM image analysis showed that after one deposition cycle for 4 h, the CHI-C formed a thick but rough layer leaving micrometer-scale pores on the hydrogel surface (Figure 2C, left image). After six deposition cycles, CHI-C on polydopamine-treated PU (PU_PD) formed a continuous hydrogel layer on the substrate surface (Figure 2C, middle image). These results suggest that in the dip coating method in an oxidative environment (i.e., pH 8.5 Tris buffer solution), the thickness of the hydrogel is determined in the initial phase of the coating providing a porous scaffold that is filled in with additional deposition cycles (Figure 2D), rather than deposited in a layer-by-layer fashion. The deposition of HSA-C hydrogel showed similar behavior during multiple deposition cycles, although HSA-C formed more discrete aggregates rather than interconnected network even after 6 deposition cycles (Figure S3), potentially due to the higher inter-molecular reactions and tendency for self-oligomerization compared to CHI-C (Ortega-Vinuesa et al., 1998; Bhattacharya et al., 2014). When the CHI-C hydrogel layer after 6 deposition cycles was treated with silver nitrate (AgNO3), silver nanoparticles (AgNPs) were formed on the surface through catechol-based reduction of silver ions into Ag(s) (Figure 2C, right image) indicating the presence of catechol groups on the surface, which potentially can serve as functional groups for further surface functionalization (e.g., immobilization of antibacterial agents, etc.). In XPS analysis, two peaks at the binding energy of 367.3 and 373.3 eV were observed, which are ascribed to Ag 3d5/2 and Ag 3d3/2 signals, respectively. The splitting of Ag 3d doublet is about 6.0 eV, indicating the synthesized AgNPs are in their metallic forms [Ag(s), see Figure S4].
Figure 2. Fabrication and surface morphology of CHI-C hydrogel coatings on urinary catheter materials. (A,B) Changes in the thickness of the CHI-C hydrogel coating during (A) the first 4-h deposition cycle (B) during 6 deposition cycles (4 h each). (C) SEM images of the CHI-C hydrogel coated PU substrates fabricated using 1 deposition cycle (left panel), 6 deposition cycles (middle panel), and 6 deposition cycles treated with AgNO3 to form AgNPs (right panel). (D) Schematic representations of the CHI-C hydrogel coating formation using the dip coating method in oxidative environment.
We further examined how the hydrogel coatings can change the hydrophilicity and water absorption on the elastomer surfaces. The PDMS, PVC and PU substrates with polymer networks were more hydrophilic, as indicated by a decrease in contact angle, most likely through a synergistic effect of the catechol moiety and the macromolecules (Figure 3A). After six deposition cycles of CHI-C coating, the water contact angles of PDMS, PVC and PU substrates were decreased from 87.53 ± 5.87°, 57.86 ± 3.78°, and 76.95 ± 3.57°, to 27.90 ± 6.65°, 23.0 ± 5.4°, and 28.6 ± 9.7°, respectively, indicating the CHI-C coating can enhance the wetting on the surface of the elastomeric materials. Similarly, the six deposition cycles of HA-C, and HSA-C on different substrates also showed decreased water contact angles suggesting that the hydrogel coating strategy can be used for other types of polymers. The HA-C and HSA-C coatings on PDMS resulted in the water contact angle decrease from 87.53 ± 5.87° to 29.73 ± 4.76°, and 25.94 ± 1.77°, respectively, those coated on PVC resulted in contact angle decrease from 57.86 ± 3.78° to 22.2 ± 6.8°, and 18.4 ± 3.2°, respectively, and those coated on PU resulted in the contact angle decrease from 76.95 ± 3.57° to 21.5 ± 2.8°, and 24.7 ± 1.6°, respectively. To evaluate the water absorption of the CHI-C hydrogel coating, A PTFE tube was coated with FITC-conjugated CHI-C following the protocols established above. Opaque PTFE tubes were used instead of PVC or PU that are transparent to avoid fluorescence from entering the polymer tube. To ensure the hydrogel layer is fully saturated into a continuous layer, the PTFE tubes were coated using 10 deposition cycles after polydopamine treatment. The coating was imaged using fluorescent microscope and the sectional thickness was measured using image analysis software. The average thickness of the fully dried CHI-C hydrogel coating was 10.4 ± 1.7 μm (Figure 3B). When they were hydrated with PBS (pH 7.4), their thickness increased to 17.4 ± 2.1 μm, and the volume change after hydration (i.e., water content) was 67%. This increase in thickness indicated that the polymer networks were formed to stably contain water (i.e., hydrogel), which is beneficial to form low-friction anti-biofouling surfaces.
Figure 3. Wettability of PDMS, PVC, and PU substrates with polymeric hydrogel coatings after 6 deposition cycles. (A) Measurement of water contact angles of substrates coated with hydrogel (n = 3). (B) The side view of dry and water swollen FITC-conjugated CHI-C hydrogel coating on PTFE tube imaged with confocal microscope before and after immersion in PBS buffer (pH 7.4) for 10 min (scale bar = 20 μm) and the thickness of the CHI-C hydrogel coating.
The hydrated hydrogel coating could significantly lower the friction coefficient by generating a repulsive force from the surface tension of water molecules entrapped within hydrogel film (Kang et al., 2016; Dedinaite and Claesson, 2017). The lateral force mode of SPM was used to calculate the friction coefficients of hydrated substrates after immersion in PBS (Figure 4A). When lateral voltage (VA−B) was measured against normal voltage (VLFM), its slope can be used to find the relative friction coefficient of the surfaces. The CHI-C hydrogel coating on PDMS, PVC, and PU substrates lowered the friction coefficient from 0.318 ± 0.045, 0.271 ± 0.024, and 0.843 ± 0.206 to 0.103 ± 0.006, 0.089 ± 0.011, and 0.243 ± 0.146, respectively (Figure 4B). The coatings using HA-C and HSA-C on PDMS substrate resulted in a decrease of friction coefficient from 0.318 ± 0.045 to 0.085 ± 0.021, and 0.116 ± 0.010 respectively, for those on PVC substrate resulted in a decrease from 0.271 ± 0.024 to 0.181 ± 0.010, and 0.105 ± 0.003, and for those on PU substrate resulted in a decrease from 0.843 ± 0.206 to 0.104 ± 0.030 and 0.422 ± 0.051 (Figure 4B). HSA coating in general showed higher friction coefficients, potentially due to its tendency to form aggregates and rough surface deposition (Figure S3). The relative friction coefficient decreased in all the polymer-coated substrates, which was calculated by dividing the modified substrate's coefficient by the bare substrate (Figure 4C). Given that surface friction contributes to the adhesion of bacteria in fluidic conditions, which then leads to the development of biofilm that is resistant to antimicrobial treatment (Katsikogianni and Missirlis, 2004; Swartjes et al., 2014), the catechol-functionalized hydrogel coatings with significant reductions in the friction coefficients may offer a preventive strategy against the initial biofilm formation and its progression, in addition to providing lubricity to catheter surface.
Figure 4. Friction coefficient of PDMS, PVC, and PU substrates after modification with catechol-conjugated hydrogels CHI-C, HA-C, and HSA-C. (A) Lateral voltage (VA−B) vs. normal voltage (VLFM) of unmodified and coated substrates (n = 3). (B) Friction coefficient (VL/VA−B) and relative friction coefficient (μ) of coated substrates compared to bare surface. (C) The percentage of relative friction coefficient of coated substrates compared to unmodified substrates (n = 3, *p < 0.05 and **p < 0.01 vs. Bare).
Stability of hydrogel coatings is critical for clinical applications especially for those exposed to high shear stress. Hydrogel coatings with low stability could lead to surface delamination, cracking, and the loss of anti-fouling properties within hours (Cloutier et al., 2015; Fischer et al., 2015). We anticipated that a micrometer-scale hydrogel could prolong the anti-fouling properties by providing a thicker layer of water-containing coating. The stability of the CHI-C hydrogel coatings on PU and PVC was evaluated using XPS, to assess whether cracks or delamination, that would expose bare substrate susceptible to bacterial attachment, would occur. PVC and PU substrates were coated with the CHI-C hydrogel using 6 deposition cycles and incubated in PBS buffer (pH 7.4) for 15 days with continuous agitation in a 3D shaker. After deposition of CHI-C hydrogel onto the PU surface, the N 1s peak at the binding energy 398 eV (Hendrickson et al., 1969) was increased for all groups (bare PU: 0.95% and CHI-C hydrogel coating: 6.15%) (Figure 5A, also see Figure S5A), and after 15 days of incubation with continuous agitation, the N 1s peak was 6.04% indicating that the CHI-C layer is still the outermost layer. On the PVC surface, the organic chloride (Cl 2p) peak at the binding energy 198 eV (Ebner et al., 1976) decreased from 14.99% in unmodified PVC to 1.93% in CHI-C hydrogel coated PVC, and after 15 days of incubation with continuous agitation, the Cl 2p peak was 3.94% (Figure 5A, Figure S5B). Given that the Cl 2p peak of CHI-C hydrogel coatings on PU with no Cl on substrate also increased in similar levels (about 5%), the small peaks are likely from the trace amount of chloride ions from buffer solutions used for the deposition (i.e., Tris buffer) and the incubation (i.e., PBS buffer), and not from the bare substrate (i.e., 14.99% Cl 2p). In addition, when the FITC-conjugated CHI-C and other polymers (i.e., HA-C and HSA-C) were deposited on PU, the FITC fluorescence signals were maintained for 20 days with no crack or delamination observed (Figure 5B). These results show that the CHI-C hydrogel provides a stable coating of the substrate over a few weeks allowing for prolonged surface lubricity and anti-fouling properties potentially in combination with additional antibacterial agents (e.g., AgNPs).
Figure 5. Assessment of stability of the catechol-functionalized hydrogel coatings with 6 deposition cycles on materials used in urethral catheters. (A) XPS peaks of PU and PVC substrates with unmodified surfaces or with CHI-C and quantifications of surface atomic compositions [nitrogen (N 1s) and chloride (Cl 2p)]. (B) Fluorescent images of FITC modified CHI-C, HA-C, and HSA-C coatings on PU substrates after immersion in PBS for 20 days.
The antibacterial effect of the CHI-C hydrogel coating was further evaluated. The low friction of the hydrogel coated surface can reduce the microbial adhesion in the initial phase of exposure and its stability can help prolong the surface characteristics to prevent biofilm formation, which is intrinsically resistant to antibiotics (Jones et al., 2008; Milo et al., 2016). To test if the coating can reduce the bacterial adhesion, PU and PVC substrates with the CHI-C hydrogel coating treated with 10 mM AgNO3 to form AgNPs were used to evaluate the reduced adherence of E. coli and S. aureus, which are the most common gram negative and gram positive bacteria responsible for biofilm formation associated with the use of indwelling medical devices (Foster and Höök, 2000; Donlan, 2001). The CHI-C hydrogel was used for the study due to the antimicrobial properties of chitosan itself (Raafat et al., 2008; Goy et al., 2009; Kara et al., 2014) and its excellent lubricity, stability and capability to form AgNPs by catechol-based reduction of silver ions. Silver is also one of the few antimicrobial agents for urethral catheter coatings, along with other medical devices, that is approved by the US Food and Drug Administration (FDA). To evaluate the antibacterial properties of the CHI-C hydrogel coated PU and PVC, a variant of the ISO 22196 standard for measurement of antibacterial activity on plastics and other non-porous surfaces, in which E. coli and S. aureus are common choices of bacteria strands, was used (ISO22196:2011, 2011). Briefly, the bacteria were cultured on the substrate surface for 8 h at 37 °C in hydrated condition within a 3D shaker, and bacterial adherence was measured after washing and lyophilization of the substrate. The SEM analysis and quantitative data showed that during the initial adhesion period, the population of live E. coli cells significantly decreased in the CHI-C hydrogel coated PU substrate, and further decreased in the AgNPs-CHI-C PU group, compared to bare substrate (bare: 85.23 ± 0.95%, CHI-C hydrogel coating: 48.32 ± 3.80%, CHI-C hydrogel coating with AgNPs: 4.70 ± 0.95%) (Figures 6A,B). Similarly, a significant decrease in the population of live S. aureus cells was observed in the CHI-C coated PU substrate and the CHI-C hydrogel coating with AgNPs on PU further decreased the population (bare: 100 ± 18%, CHI-C hydrogel coating : 73.72 ± 1.03%, CHI-C hydrogel coating with AgNPs: 59.12 ± 5.16%). These results indicate the multilayer hydrogel coating with silver could lower the initial attachment of bacteria, which potentially can further significantly impair biofilm formation. In contrast, the CHI-C hydrogel coating with AgNPs on PVC substrate only exhibited a reduction of the E. coli culture but showed minimal antibacterial effect against S. aureus (Figures 6A,B) suggesting that the type of substrates can also impact the antibacterial effect. Previous studies have shown that PVC catheter can support the survival and growth of Pseudomonas aeruginosa while PU catheter remained inert most likely due to the use of plasticizers and stabilizers in PVC catheters, which can be released into the surrounding solutions and also support the survival of bacteria (Martinez-Martinez et al., 1990).
Figure 6. Enhanced antibacterial adhesion effects by 6 deposition cycles of CHI-C and silver impregnated CHI-C onto the PU and PVC substrates. (A) SEM analysis was performed to validate the antibacterial adhesion effects 8 h after cell seeding. The white arrows indicate E. coli. Scale bar indicates 2 μm. (B) The adhesion of live Escherichia coli (E. coli) and Staphylococcus aureus (S. aureus) were quantified by Live/Dead BacLight Viability assay (n = 3 *p < 0.05 and **p < 0.01 vs. each bare substrate, ##p < 0.01 vs. each CHI-C substrate).
Overtime, the interaction between biofilm and urine leads to mineral precipitation around the catheters that may cause blockage of the inner lumen and demand medical attentions (Donlan, 2001; Wang et al., 2019). Eradication of biofilm after its formation is practically impossible to achieve due to increased risk of polymicrobial infection and the multiplied population's resistance to antibiotics (Singha et al., 2017). Instead, more emphasis should be put on the prevention of biofilm formation, starting with the reduction in bacterial adhesion, which is correlated to reduced progression into biofilm. It has been shown that catechol-functionalized hydrogel can endorse the sustained release of a variety of biological therapeutics, including silver nanoparticles, because of the latent activities of the catechol groups, which also contributed to enhanced stability in physiological conditions (Min and Hammond, 2011; Fullenkamp et al., 2012). As for the hydrogel coating approach outlined in this report, it would be highly desirable to investigate its compatibility with other antimicrobial or biocidal agents and optimize the assembly techniques for sustained release. It can also be expected that multilayer depositions on other biomaterials or biomedical devices can be efficiently harvested from this technology and further developed for incorporating additional functional moieties.
In summary, we have demonstrated the feasibility of using bioinspired catechol conjugates to reduce infections associated with the use of urethral catheters. Catechol conjugation allowed the hydrogels to be simply coated onto the substrates, which obviates the need for aggressive regimens in traditional coating methods for hydrophobic substrates and also increases the homogeneity of the film morphology. The modified surfaces presented higher hydrophilicity due to water adhesion in the hydrogel complex, which can contribute to the desired lubricity and anti-microbial fouling effect for catheter coating. In addition, the hydrogel coatings were also shown to be stable on both PVC and PU surfaces, after 20 days of incubation in hydrated conditions. Especially, the catechol-conjugated chitosan and embedded AgNPs on the PU substrate reduced the adhesion of E. coli and S. aureus and can possibly prevent the concerning biofilm formation on the materials used for urethral catheters. Therefore, the easy-to-fabricate, catechol-mediated hydrogel assembly has demonstrated its robust properties and feasibility as a novel urethral catheter surface coating with enhanced lubricity and anti-biofouling effects.
All datasets for this study are included in the article/Supplementary Material.
KY, KK, TP, and YL developed the concept and designed experiments. KK, KY, and YL conducted the experiments. KY, KK, EL, SL, TP, HL, JK, and YL analyzed the data. KY, KK, EL, SL, HL, JK, and YL, wrote the manuscript. All authors provided critical comments on the manuscript.
This work was supported by the Basic Science Research Program through the National Research Foundation of Korea (NRF) funded by the Ministry of Education of Korea (2012R1A6A3A03041166) and Korea Institute for Advancement of Technology (N0002123) to YL. It also was funded by the Saudi National Industrial Development and Logistics Program (NIDLP) in the form of the Health Initiative and the Technology Leader Program Initiative, projects No. 20-0103 and No. 20-0051.
The authors declare that the research was conducted in the absence of any commercial or financial relationships that could be construed as a potential conflict of interest.
We thank Jong Seung Lee in Yonsei University for the assistance in XPS analysis. We would like to dedicate this article to the late Professor Tae Gwan Park.
The Supplementary Material for this article can be found online at: https://www.frontiersin.org/articles/10.3389/fmats.2019.00274/full#supplementary-material
An, R., Dong, Y., Zhu, J., and Rao, C. (2017). Adhesion and friction forces in biofouling attachments to nanotube- and PEG- patterned TiO2 surfaces. Colloids Surf. B Biointerfaces 159, 108–117. doi: 10.1016/j.colsurfb.2017.07.067
Anisha, B. S., Biswas, R., Chennazhi, K. P., and Jayakumar, R. (2013). Chitosan-hyaluronic acid/nano silver composite sponges for drug resistant bacteria infected diabetic wounds. Int. J. Biol. Macromol. 62, 310–320. doi: 10.1016/j.ijbiomac.2013.09.011
Bhattacharya, A., Prajapati, R., Chatterjee, S., and Mukherjee, T. K. (2014). Concentration-dependent reversible self-oligomerization of serum albumins through intermolecular β-sheet formation. Langmuir 30, 14894–14904. doi: 10.1021/la5034959
Brokke, P., Dankert, J., Carballo, J., and Feijen, J. (1991). Adherence of coagulase-negative staphylococci onto polyethylene catheters in vitro and in vivo: a study on the influence of various plasma proteins. J. Biomater. Appl. 5, 204–226. doi: 10.1177/088532829100500305
Cloutier, M., Mantovani, D., and Rosei, F. (2015). Antibacterial coatings: challenges, perspectives, and opportunities. Trends Biotechnol. 33, 637–652. doi: 10.1016/j.tibtech.2015.09.002
Dallas, P., Sharma, V. K., and Zboril, R. (2011). Silver polymeric nanocomposites as advanced antimicrobial agents: classification, synthetic paths, applications, and perspectives. Adv. Colloid Interface Sci. 166, 119–135. doi: 10.1016/j.cis.2011.05.008
Dedinaite, A., and Claesson, P. M. (2017). Synergies in lubrication. Phys. Chem. Chem. Phys. 19, 23677–23689. doi: 10.1039/C7CP03517A
Dellimore, K. H., Helyer, A. R., and Franklin, S. E. (2013). A scoping review of important urinary catheter induced complications. J. Mater. Sci. Mater. Med. 24, 1825–1835. doi: 10.1007/s10856-013-4953-y
Donlan, R. M. (2001). Biofilms and device-associated infections. Emerg. Infect. Dis. 7, 277–281. doi: 10.3201/eid0702.010226
Drago, L., Cappelletti, L., De Vecchi, E., Pignataro, L., Torretta, S., and Mattina, R. (2014). Antiadhesive and antibiofilm activity of hyaluronic acid against bacteria responsible for respiratory tract infections. APMIS. 122, 1013–1019. doi: 10.1111/apm.12254
Du, J., Liu, X., Liu, W., Wu, Z., and Chen, H. (2014). One-step preparation of vinyl-functionalized material surfaces: a versatile platform for surface modification. Sci. China Chem. 57, 654–660. doi: 10.1007/s11426-014-5067-1
Ebner, J. R., McFadden, D. L., Tyler, D. R., and Walton, R. A. (1976). X-ray photoelectron spectra of inorganic molecules. 12. Chlorine 2p binding energies of dinuclear chloro anions and mononuclear chloro cations of the transition metals. Inorg. Chem. 15, 3014–3018. doi: 10.1021/ic50166a014
Fischer, M., Vahdatzadeh, M., Konradi, R., Friedrichs, J., Maitz, M. F., Freudenberg, U., et al. (2015). Multilayer hydrogel coatings to combine hemocompatibility and antimicrobial activity. Biomaterials 56, 198–205. doi: 10.1016/j.biomaterials.2015.03.056
Foster, T. J., and Höök, M. (2000). “Molecular basis of adherence of staphylococcus aureus to biomaterials,” in Infections Associated with Indwelling Medical Devices, 3rd Edn, eds F. A. Waldvogel and A. L. Bisno (Washington, DC: ASM Press).
Fullenkamp, D. E., Rivera, J. G., Gong, Y. K., Lau, K. H. A., He, L., Varshney, R., et al. (2012). Mussel-inspired silver-releasing antibacterial hydrogels. Biomaterials 33, 3783–3791. doi: 10.1016/j.biomaterials.2012.02.027
Gerard, L. L., Cooper, C. S., Duethman, K. S., Gordley, B. M., and Kleiber, C. M. (2003). Effectiveness of lidocaine lubricant for discomfort during pediatric urethral catheterization. J. Urol. 170(2 Pt 1), 564–567. doi: 10.1097/01.ju.0000068720.10881.b3
GhavamiNejad, A., Park, C. H., and Kim, C. S. (2016). In situ synthesis of antimicrobial silver nanoparticles within antifouling zwitterionic hydrogels by catecholic redox chemistry for wound healing application. Biomacromolecules 17, 1213–1223. doi: 10.1021/acs.biomac.6b00039
Goy, R. C., Britto, D. D., and Assis, O. B. G. (2009). A review of the antimicrobial activity of chitosan. Polímeros 19, 241–247. doi: 10.1590/S0104-14282009000300013
Hendrickson, D. N., Hollander, J. M., and Jolly, W. L. (1969). Nitrogen ls electron binding energies. Correlations with molecular orbital calculated nitrogen charges. Inorg. Chem. 8, 2642–2647. doi: 10.1021/ic50082a020
Hong, S., Yang, K., Kang, B., Lee, C., Song, I. T., Byun, E., et al. (2013). Hyaluronic acid catechol: a biopolymer exhibiting a pH-dependent adhesive or cohesive property for human neural stem cell engineering. Adv. Funct. Mater. 23, 1774–1780. doi: 10.1002/adfm.201202365
ISO22196:2011 (2011). Measurement of Antibacterial Activity on Plastics and Other Non-Porous Surfaces. International Organization for Standardization.
Jones, D. S., Lorimer, C. P., McCoy, C. P., and Gorman, S. P. (2008). Characterization of the physicochemical, antimicrobial, and drug release properties of thermoresponsive hydrogel copolymers designed for medical device applications. J. Biomed. Mater. Res. Part B Appl. Biomater. 85B, 417–426. doi: 10.1002/jbm.b.30960
Kang, T., Banquy, X., Heo, J., Lim, C., Lynd, N. A., Lundberg, P., et al. (2016). Mussel-inspired anchoring of polymer loops that provide superior surface lubrication and antifouling properties. ACS Nano 10, 930–937. doi: 10.1021/acsnano.5b06066
Kara, F., Aksoy, E. A., Yuksekdag, Z., Hasirci, N., and Aksoy, S. (2014). Synthesis and surface modification of polyurethanes with chitosan for antibacterial properties. Carbohydr. Polym. 112, 39–47. doi: 10.1016/j.carbpol.2014.05.019
Katsikogianni, M., and Missirlis, Y. F. (2004). Concise review of mechanisms of bacterial adhesion to biomaterials and of techniques used in estimating bacteria-material interactions. Eur. Cell Mater. 8, 37–57. doi: 10.22203/eCM.v008a05
Kinnari, T. J., Peltonen, L. I., Kuusela, P., Kivilahti, J., Kononen, M., and Jero, J. (2005). Bacterial adherence to titanium surface coated with human serum albumin. Otol. Neurotol. 26, 380–384. doi: 10.1097/01.mao.0000169767.85549.87
Koberstein, J. T. (2004). Molecular design of functional polymer surfaces. J. Polym. Sci. B Polym. Phys. 42, 2942–2956. doi: 10.1002/polb.20157
Lawrence, E. L., and Turner, I. G. (2005). Materials for urinary catheters: a review of their history and development in the UK. Med. Eng. Phys. 27, 443–453. doi: 10.1016/j.medengphy.2004.12.013
Lee, H., Dellatore, S. M., Miller, W. M., and Messersmith, P. B. (2007). Mussel-Inspired Surface Chemistry for Multifunctional Coatings. Science 318, 426–430. doi: 10.1126/science.1147241
Lee, H., Lee, Y., Statz, A. R., Rho, J., Park, T. G., and Messersmith, P. B. (2008). Substrate-independent layer-by-layer assembly by using mussel-adhesive-inspired polymers. Adv. Mater. 20, 1619–1623. doi: 10.1002/adma.200702378
Li, L., Yan, B., Yang, J., Huang, W., Chen, L., and Zeng, H. (2017). Injectable self-healing hydrogel with antimicrobial and antifouling properties. ACS Appl. Mater. Interfaces 9, 9221–9225. doi: 10.1021/acsami.6b16192
Makamba, H., Kim, J. H., Lim, K., Park, N., and Hahn, J. H. (2003). Surface modification of poly(dimethylsiloxane) microchannels. Electrophoresis 24, 3607–3619. doi: 10.1002/elps.200305627
Martinez-Martinez, L., Pascual, A., and Perea, E. J. (1990). Effect of three plastic catheters on survival and growth of Pseudomonas aeruginosa. J. Hosp. Infect. 16, 311–318. doi: 10.1016/0195-6701(90)90003-7
Milo, S., Thet, N. T., Liu, D., Nzakizwanayo, J., Jones, B. V., and Jenkins, A. T. A. (2016). An in-situ infection detection sensor coating for urinary catheters. Biosens. Bioelectron. 81, 166–172. doi: 10.1016/j.bios.2016.02.059
Min, Y., and Hammond, P. T. (2011). Catechol-modified polyions in layer-by-layer assembly to enhance stability and sustain release of biomolecules: a bioinspired approach. Chem. Mater. 23, 5349–5357. doi: 10.1021/cm201801n
Ng, V. W. L., Chan, J. M. W., Sardon, H., Ono, R. J., García, J. M., Yang, Y. Y., et al. (2014). Antimicrobial hydrogels: a new weapon in the arsenal against multidrug-resistant infections. Adv. Drug Deliv. Rev. 78, 46–62. doi: 10.1016/j.addr.2014.10.028
No, H. K., Park, N. Y., Lee, S. H., and Meyers, S. P. (2002). Antibacterial activity of chitosans and chitosan oligomers with different molecular weights. Int. J. Food Microbiol. 74, 65–72. doi: 10.1016/s0168-1605(01)00717-6
Ortega-Vinuesa, J. L., Tengvall, P., and Lundström, I. (1998). Aggregation of HSA, IgG, and fibrinogen on methylated silicon surfaces. J. Colloid Interface Sci. 207, 228–239. doi: 10.1006/jcis.1998.5624
Park, H.-J., Jin, Y., Shin, J., Yang, K., Lee, C., Yang, H. S., et al. (2016). Catechol-functionalized hyaluronic acid hydrogels enhance angiogenesis and osteogenesis of human adipose-derived stem cells in critical tissue defects. Biomacromolecules 17, 1939–1948. doi: 10.1021/acs.biomac.5b01670
Raafat, D., von Bargen, K., Haas, A., and Sahl, H.-G. (2008). Insights into the mode of action of chitosan as an antibacterial compound. Appl. Environ. Microbiol. 74, 3764–3773. doi: 10.1128/AEM.00453-08
Romanó, C. L., De Vecchi, E., Bortolin, M., Morelli, I., and Drago, L. (2017). Hyaluronic acid and its composites as a local antimicrobial/antiadhesive barrier. J. Bone Jt. Infect. 2, 63–72. doi: 10.7150/jbji.17705
Saint, S. (2000). Clinical and economic consequences of nosocomial catheter-related bacteriuria. Am. J. Infect. Control 28, 68–75. doi: 10.1016/S0196-6553(00)90015-4
Saint, S., Wiese, J., Amory, J. K., Bernstein, M. L., Patel, U. D., Zemencuk, J. K., et al. (2000). Are physicians aware of which of their patients have indwelling urinary catheters? Am. J. Med. 109, 476–480. doi: 10.1016/S0002-9343(00)00531-3
Shackley, D. C., Whytock, C., Parry, G., Clarke, L., Vincent, C., Harrison, A., et al. (2017). Variation in the prevalence of urinary catheters: a profile of National Health Service patients in England. BMJ Open 7:e013842. doi: 10.1136/bmjopen-2016-013842
Siderias, J., Gaudio, F., and Singer, A. J. (2004). Comparison of topical anesthetics and lubricants prior to urethral catheterization in males: a randomized controlled trial. Acad. Emerg. Med. 11, 703–706. doi: 10.1111/j.1553-2712.2004.tb02419.x
Singha, P., Locklin, J., and Handa, H. (2017). A review of the recent advances in antimicrobial coatings for urinary catheters. Acta Biomater. 50, 20–40. doi: 10.1016/j.actbio.2016.11.070
Such, G. K., Johnston, A. P. R., and Caruso, F. (2010). Engineered hydrogen-bonded polymer multilayers: from assembly to biomedical applications. Chem. Soc. Rev. 4, 19–29. doi: 10.1039/C0CS00001A
Sudarshan, N. R., Hoover, D. G., and Knorr, D. (1992). Antibacterial action of chitosan. Food Biotechnol. 6, 257–272. doi: 10.1080/08905439209549838
Swartjes, J. J. T. M., Veeregowda, D. H., van der Mei, H. C., Busscher, H. J., and Sharma, P. K. (2014). Normally oriented adhesion versus friction forces in bacterial adhesion to polymer-brush functionalized surfaces under fluid flow. Adv. Funct. Mater. 24, 4435–4441. doi: 10.1002/adfm.201400217
Voccia, S., Ignatova, M., Jérôme, R., and Jérôme, C. (2006). Design of antibacterial surfaces by a combination of electrochemistry and controlled radical polymerization. Langmuir 22, 8607–8613. doi: 10.1021/la0606087
Wang, L., Zhang, S., Keatch, R., Corner, G., Nabi, G., Murdoch, S., et al. (2019). In vitro antibacterial and anti-encrustation performances of silver-polytetrafluoroethylene nanocomposite coated urinary catheter. J. Hosp. Infect. 103, 55–63. doi: 10.1016/j.jhin.2019.02.012
Wang, R., Neoh, K. G., Kang, E.-T., Tambyah, P. A., and Chiong, E. (2015). Antifouling coating with controllable and sustained silver release for long-term inhibition of infection and encrustation in urinary catheters. J. Biomed. Mater. Res. Part B Appl. Biomater. 103, 519–528. doi: 10.1002/jbm.b.33230
Yang, K., Lee, J. S., Kim, J., Lee, Y. B., Shin, H., Um, S. H., et al. (2012). Polydopamine-mediated surface modification of scaffold materials for human neural stem cell engineering. Biomaterials 33, 6952–6964. doi: 10.1016/j.biomaterials.2012.06.067
Yong, Y., Qiao, M., Chiu, A., Fuchs, S., Liu, Q., Pardo, Y., et al. (2019). Conformal hydrogel coatings on catheters to reduce biofouling. Langmuir 35, 1927–1934. doi: 10.1021/acs.langmuir.8b03074
Keywords: urethral catheter, surface coating, catechol, hydrogel, lubricity, anti-bacterial adhesion
Citation: Yang K, Kim K, Lee EA, Liu SS, Kabli S, Alsudir SA, Albrahim S, Zhou A, Park TG, Lee H, Almalik AM, Karp JM, Alhasan AH and Lee Y (2019) Robust Low Friction Antibiotic Coating of Urethral Catheters Using a Catechol-Functionalized Polymeric Hydrogel Film. Front. Mater. 6:274. doi: 10.3389/fmats.2019.00274
Received: 20 June 2019; Accepted: 18 October 2019;
Published: 10 December 2019.
Edited by:
Bruce P. Lee, Michigan Technological University, United StatesReviewed by:
Ameya Narkar, University of Central Florida, United StatesCopyright © 2019 Yang, Kim, Lee, Liu, Kabli, Alsudir, Albrahim, Zhou, Park, Lee, Almalik, Karp, Alhasan and Lee. This is an open-access article distributed under the terms of the Creative Commons Attribution License (CC BY). The use, distribution or reproduction in other forums is permitted, provided the original author(s) and the copyright owner(s) are credited and that the original publication in this journal is cited, in accordance with accepted academic practice. No use, distribution or reproduction is permitted which does not comply with these terms.
*Correspondence: Yuhan Lee, eWxlZTIxQGJ3aC5oYXJ2YXJkLmVkdQ==
†These authors have contributed equally to this work
Disclaimer: All claims expressed in this article are solely those of the authors and do not necessarily represent those of their affiliated organizations, or those of the publisher, the editors and the reviewers. Any product that may be evaluated in this article or claim that may be made by its manufacturer is not guaranteed or endorsed by the publisher.
Research integrity at Frontiers
Learn more about the work of our research integrity team to safeguard the quality of each article we publish.